- 1Infection Control Convergence Research Center, College of Medicine, Chungnam National University, Daejeon, Republic of Korea
- 2Department of Medical Science, College of Medicine, Chungnam National University, Daejeon, Republic of Korea
- 3Department of Infection Biology, College of Medicine, Chungnam National University, Daejeon, Republic of Korea
- 4Department of Microbiology, College of Medicine, Chungnam National University, Daejeon, Republic of Korea
- 5Department of Pharmacology, College of Medicine, Chungnam National University, Daejeon, Republic of Korea
Itaconate is a crucial anti-infective and anti-inflammatory immunometabolite that accumulates upon disruption of the Krebs cycle in effector macrophages undergoing inflammatory stress. Esterified derivatives of itaconate (4-octyl itaconate and dimethyl itaconate) and its isomers (mesaconate and citraconate) are promising candidate drugs for inflammation and infection. Several itaconate family members participate in host defense, immune and metabolic modulation, and amelioration of infection, although opposite effects have also been reported. However, the precise mechanisms by which itaconate and its family members exert its effects are not fully understood. In addition, contradictory results in different experimental settings and a lack of clinical data make it difficult to draw definitive conclusions about the therapeutic potential of itaconate. Here we review how the immune response gene 1-itaconate pathway is activated during infection and its role in host defense and pathogenesis in a context-dependent manner. Certain pathogens can use itaconate to establish infections. Finally, we briefly discuss the major mechanisms by which itaconate family members exert antimicrobial effects. To thoroughly comprehend how itaconate exerts its anti-inflammatory and antimicrobial effects, additional research on the actual mechanism of action is necessary. This review examines the current state of itaconate research in infection and identifies the key challenges and opportunities for future research in this field.
1 Introduction
Innate immune responses are the primary host defense to infection. Macrophages participate in innate immunity by recognizing pathogen- or danger-associated molecular patterns. Upon activation, macrophages initiate an intracellular signaling program to activate the expression of numerous genes involved in inflammatory, immune, and antimicrobial responses. Simultaneously, innate immune cells undergo significant metabolic reprogramming depending on their differentiation status (1–3). The metabolites up- or down-regulated during infection act as signals to modulate immune pathways, antimicrobial responses, and homeostasis (4–6).
Itaconate (ITA), a signaling metabolite produced by classically activated macrophages (7), regulates the immune, inflammatory, and oxidative responses to infection (8–10). The intrinsic pathway of endogenous ITA production in macrophages requires immune-responsive gene 1 (IRG1), also known as aconitate decarboxylase 1 (ACOD1), to decarboxylate cis-aconitate (Figure 1) (11). Normally, cis-aconitate does not dissociate from aconitase, the enzyme catalyzing the dehydration of both citrate and isocitrate, and at equilibrium, the substrates of aconitase are present 90% of citrate, 6% of isocitrate, and 4% of cis-aconitate (12–14). The expression of Irg1 encoding ACOD1 shows basal level in nonactivated macrophages, though the gene level is induced upon infection with live pathogens or LPS stimulation (11, 15). Classically activated M1 macrophages undergo dynamic immunometabolic remodeling, manifesting as early accumulation of succinate and ITA, during infection and inflammation, and the accumulation of the two molecules is correlated with each other (7, 16). ITA inhibits succinate dehydrogenase (SDH) competitively based on structural similarity with succinate (Figure 1) (17, 18).
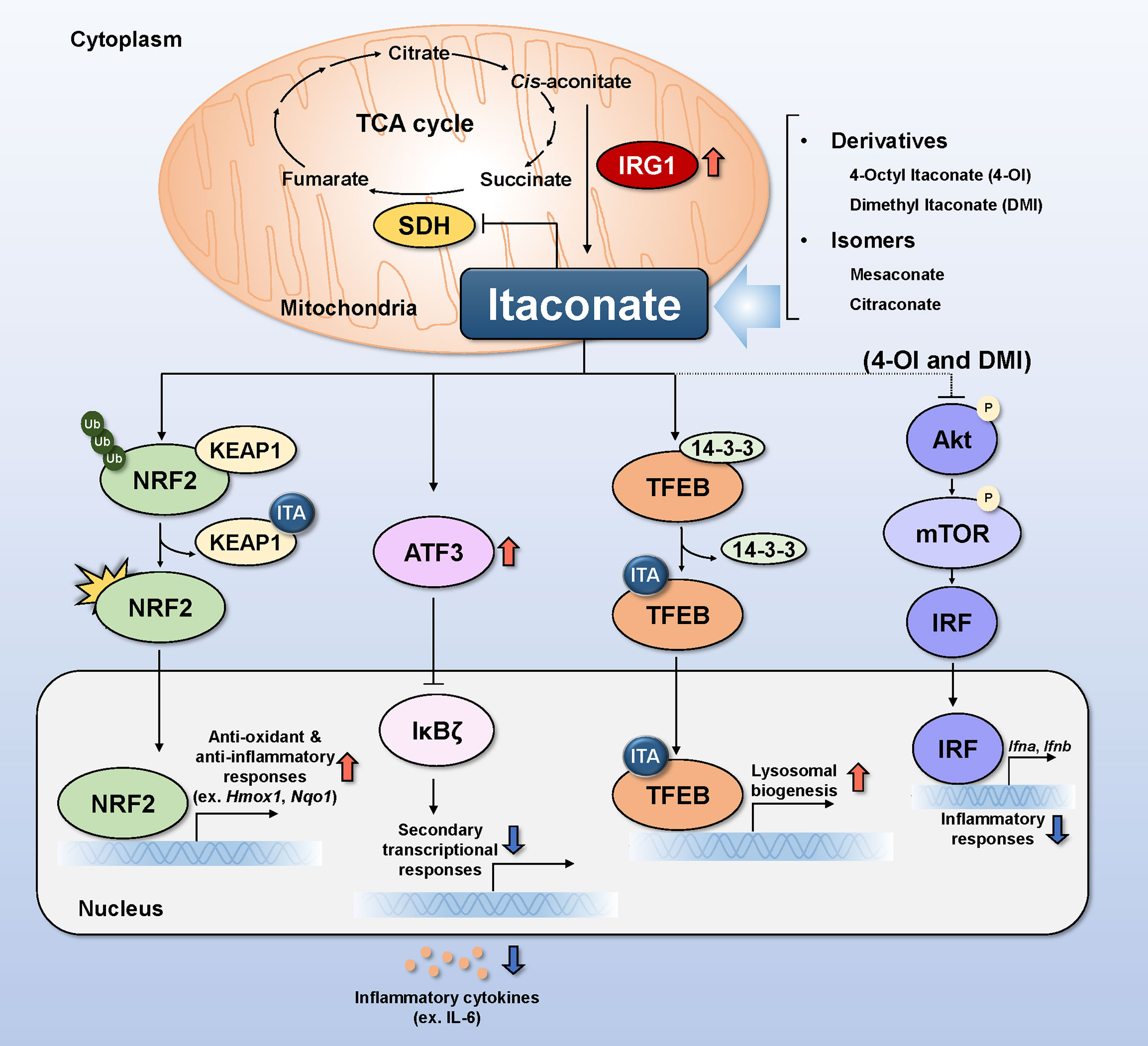
Figure 1 Molecular mechanisms of itaconate and its relatives in infection. Itaconate (ITA) is produced from the decarboxylation of cis-aconitate in mitochondria in response to IRG1 induction. Itaconate modulates the activity of SDH by competing with succinate, thereby regulating the TCA cycle. Itaconate causes KEAP1 to dissociate from the NRF2-KEAP1 complex by alkylating cysteine residues 151,257,288,273, and 297 on KEAP1. Translocation of activated NRF2 to the nucleus increases anti-oxidant and anti-inflammatory gene expression. ATF3 induced by itaconate translocates to the nucleus to inhibit IkBζ, thereby preventing the production and secretion of inflammatory cytokines. TFEB alkylated by itaconate on cysteine residue 212 elicits translocation to the cell nucleus, resulting in the upregulation of genes related to lysosomal biogenesis. DMI and 4-OI decrease Akt phosphorylation, whereas ITA increases it. Inhibiting Akt phosphorylation inhibits mTOR/IRF signaling and the production of type I interferon (Ifna and Ifnb). Alk, alkylation; ATF3, activating transcription factor 3; IκBζ, IkappaB-zeta; IL, interleukin; IRF, interferon-regulated factor; IRG1, immune-responsive gene 1; KEAP1, Kelch ECH associating protein 1; NF-κB, nuclear factor-kappa B; NRF2, nuclear factor erythroid 2-related factor 2; SDH, succinate dehydrogenase; TFEB, transcription factor EB.
To circumvent the low plasma membrane permeability, ITA is esterified and investigated as 4-octyl ITA (4-OI) or dimethyl ITA (DMI) (17, 19, 20). ITA, its esterified derivatives (4-OI and DMI), and its naturally occurring isomers (mesaconate and citraconate) make considerable contributions to infectious and inflammatory diseases. Indeed, the anti-infective and anti-inflammatory roles of ITA and its isomers and esterified derivatives have been discussed (8–10, 21–23). Here, we review the regulation of endogenous ITA production in terms of immunometabolic networks and the functions of ITA and its relatives during infection. We also focus on the molecular mechanisms by which ITA and its related members regulate innate and inflammatory responses in infection and immunity.
2 Immune regulation via the IRG1-ITA pathway during infection
Classical activation of macrophages toward the M1 phenotype drives metabolic reprogramming, leading to upregulated glycolysis, disruption of the TCA cycle, and ITA accumulation (24). In Mycobacterium tuberculosis (Mtb) infection, the metabolite glutamine drives M1 macrophage responses via immunometabolic remodeling in which the biosynthetic precursor ITA is generated (25). In turn, ITA functions as a feedback inhibitory regulator by TCA-cycle reprogramming in macrophages (26). That is, ITA inhibits isocitrate dehydrogenase 2 (IDH2), thereby altering the mitochondrial NADP+/NADPH ratio and inhibiting SDH (26). In addition, interleukin (IL)-33-mediated metabolic rewiring in macrophages upregulates ITA production, and ITA promotes the GATA3-mediated polarization of alternatively activated macrophages, thereby contributing to tissue repair and the resolution of inflammation (27). Moreover, 4-OI suppresses aerobic glycolysis by directly alkylating Cys22 of GAPDH, thus inhibiting inflammatory responses in activated macrophages (28).
Macrophage stimulation by toll-like receptor (TLR) ligands activates ITA production (29). In human monocytic THP-1 cells, lipopolysaccharide (LPS) stimulation upregulates IRG1 mRNA via cyclin-dependent kinase 2 (CDK2)-mediated JUN activation and IRG1 accumulation, thereby robustly activating the pro-inflammatory tumor necrosis factor-α (TNF-α) signaling pathway (30). In addition, the host TLR2, myeloid differentiation primary response 88 (MyD88), nuclear factor-kappa B (NF-κB), stimulator of interferon genes (STING), and type I interferon (IFN) receptor signaling pathways induce IRG1 expression during Mtb infection (31). Signals from phagocytosis and endosomal acidification are needed to induce IRG1 expression in bone marrow-derived macrophages (BMDMs) (31). In Brucella infection, MyD88 signaling is required for ITA production and ITA-mediated antibacterial responses to B. melitensis in macrophages (32). By contrast, IRG1-mediated ITA production is suppressed by the induction of β-glucan-mediated trained immunity, thus modulating immunoparalysis during sepsis (33).
Type I and II IFNs trigger the expression of IRG1 and ITA to exert bactericidal functions against Legionella pneumophila, and extracellular multidrug-resistant gram-positive and negative bacteria (34). The pro-inflammatory cytokines TNF-α and IL-6 activate ITA-mediated direct antimicrobial responses in M. avium-infected macrophages (35). TNF-α and IL-6 activate paracrine signaling to promote the IRF1/IRG1 pathway and the repositioning of mitochondrial to bacterial phagosomes during M. avium infection (35). Therefore, the inflammatory responses of bystander cells at infection sites may contribute to endogenous ITA production, thereby amplifying antimicrobial responses during infection.
3 Roles of ITA in infection
3.1 ITA-induced protection
In most infection models, ITA and its family members are considered antimicrobial metabolites, because they target isocitrate lyase of the glyoxylate shunt during Salmonella enterica and Mtb infections (11). In vivo, the IRG1-ITA pathway ameliorates neutrophil-mediated pathologic inflammation to promote antimicrobial responses against Mtb infection (36). Also, the endogenous ITA-mediated restriction of intracellular bacteria such as S. Typhimurium depends on the guanosine triphosphatase Rab32, which interacts with IRG1 to deliver the antimicrobial factor ITA to the Salmonella-containing vacuole (37). In addition, the T helper cell 1 (Th1)-induced cytokine IFN-γ stimulates the production of ITA, which inhibits mitochondrial complex II to increase bactericidal activity against Francisella tularensis (38).
Brucella infection upregulates IRG1, which is critical for the control of Brucella growth, in murine alveolar macrophages (39). Notably, ITA and DMI exhibit direct antimicrobial effects against Brucella by targeting isocitrate lyase of B. abortus (39). Similarly, in a Vibrio infection model, ITA suppresses the growth of Vibrio sp. DO1 (40). Moreover, ITA reduces intracellular Escherichia coli at later time points in macrophages, at least in part by increasing phagocytosis and bactericidal activity (41). In addition, DMI suppresses intracellular growth of Mtb, M. avium, even of multidrug resistant Mtb in macrophages, partly associated with the induction of autophagy (22). In Zika virus infection of neurons, receptor interacting protein kinase 1 (RIPK1) and RIPK3 signaling suppresses viral replication via IRG1-mediated ITA production (42). In addition, IRG1 is essential for the restriction of Coxiella burnetii infection, which causes zoonotic Q fever, in macrophages and intratracheal or intraperitoneal infection models. IRG1 deficiency amplifies inflammatory responses—including the expression of Il6, Ifng, Nos2, and Gbp1—in the lungs of infected mice. Interestingly, exogenous ITA reduces the bacterial burden, and the physiologic concentration of ITA is sufficient to control C. burnetii replication (43). Furthermore, in chronic infection with Toxoplasma gondii, which impairs cognitive functions, treatment of infected mice with DMI improves behavioral performance and ameliorates microglial inflammation (44).
There are few reports on the clinical relevance of ITA in human infectious diseases. Interestingly, multidrug-resistant tuberculosis (TB) patients show an inflammatory metabolic response, which manifests as upregulated succinate and downregulated ITA, which is increased in patients on appropriate anti-TB treatment (45). Therefore, host metabolic remodeling accompanied by decreased ITA drives immunopathological responses in human TB.
3.2 ITA pathological functions
Some findings indicate a pathologic or insufficiently protective role for the IRG1-ITA pathway in infection. Respiratory syncytial virus (RSV) infection triggers IRG1 expression to promote reactive oxygen species (ROS) generation in human A549 cells, immune cell infiltration, and lung injury in vivo (46). In addition, the dysfunctional complex of phosphatase and tensin homolog deleted on chromosome 10 (PTEN) with the cystic fibrosis (CF) transmembrane conductance regulator (CFTR), which is associated with the pathogenesis of cystic fibrosis, increases the production of succinate and IRG1-ITA (47). Nevertheless, these metabolic changes are not sufficient to clear Pseudomonas aeruginosa due to impaired PTEN activity and excessive oxidative stress (47). IRG1 and ITA are required for bacterial persistence and host tolerance during infection with Klebsiella pneumoniae sequence type 258 (Kp ST258) (48). Kp ST258 infection drives host metabolic pathways towards glutaminolysis, fatty acid oxidation, and accumulation of ITA, resulting in anti-inflammatory M2-type responses and disease-tolerant immune responses (48). Together, these recent studies raise the question of how the IRG1-ITA pathway contributes to host detrimental responses rather than protection in certain types of infection.
Intracellular microbes and parasites can distort the IRG1/ITA axis and use ITA during infection (44). Influenza A virus (IAV) infection increases Irg1 mRNA expression in M2-type human macrophages and undifferentiated peripheral blood mononuclear cells (PBMCs) (49). In a rabbit model of P. aeruginosa (PAO1) infection, IRG1 induction and ITA production in host cells may contribute to bacterial adaptation and biofilm formation by enabling use of ITA as a carbon source in the acute phase of wound infection (49). Some bacteria such as P. aeruginosa clinical isolates can establish infection and replicate in host cells by using ITA as their major carbon source (50). Upon exposure to ITA, P. aeruginosa produces extracellular polysaccharides (EPS), which stimulate the production of ITA in host cells (50). In addition, ITA inhibits glycolysis in Staphylococcus aureus, a pathogen easily adaptable to the host immunometabolic environment, and increases the synthesis of extracellular polysaccharide and biofilm formation (51). Furthermore, in a vaccine model of Francisella tularensis infection, Irg1 deficiency increases resistance to secondary challenge by promoting CD4+ and CD8+ T cell responses (52). Together, these results suggest that pathogens can use endogenous ITA as a nutrient to establish persistent infection by modulating host immune pathways. Further studies are needed to determine how pathogens manipulate the IRG1-ITA axis to influence innate and adaptive immune pathways.
4 Mechanisms by which ITA and its family members control infection
There are several mechanisms by which ITA and its family members exert antimicrobial effects during infection; we briefly discuss the four major mechanisms, i.e., nuclear factor erythroid 2-related factor 2 (NRF2), activating transcription factor 3 (ATF3), transcription factor EB (TFEB), and Akt, below. And these are summarized in Figure 1 and Table 1.
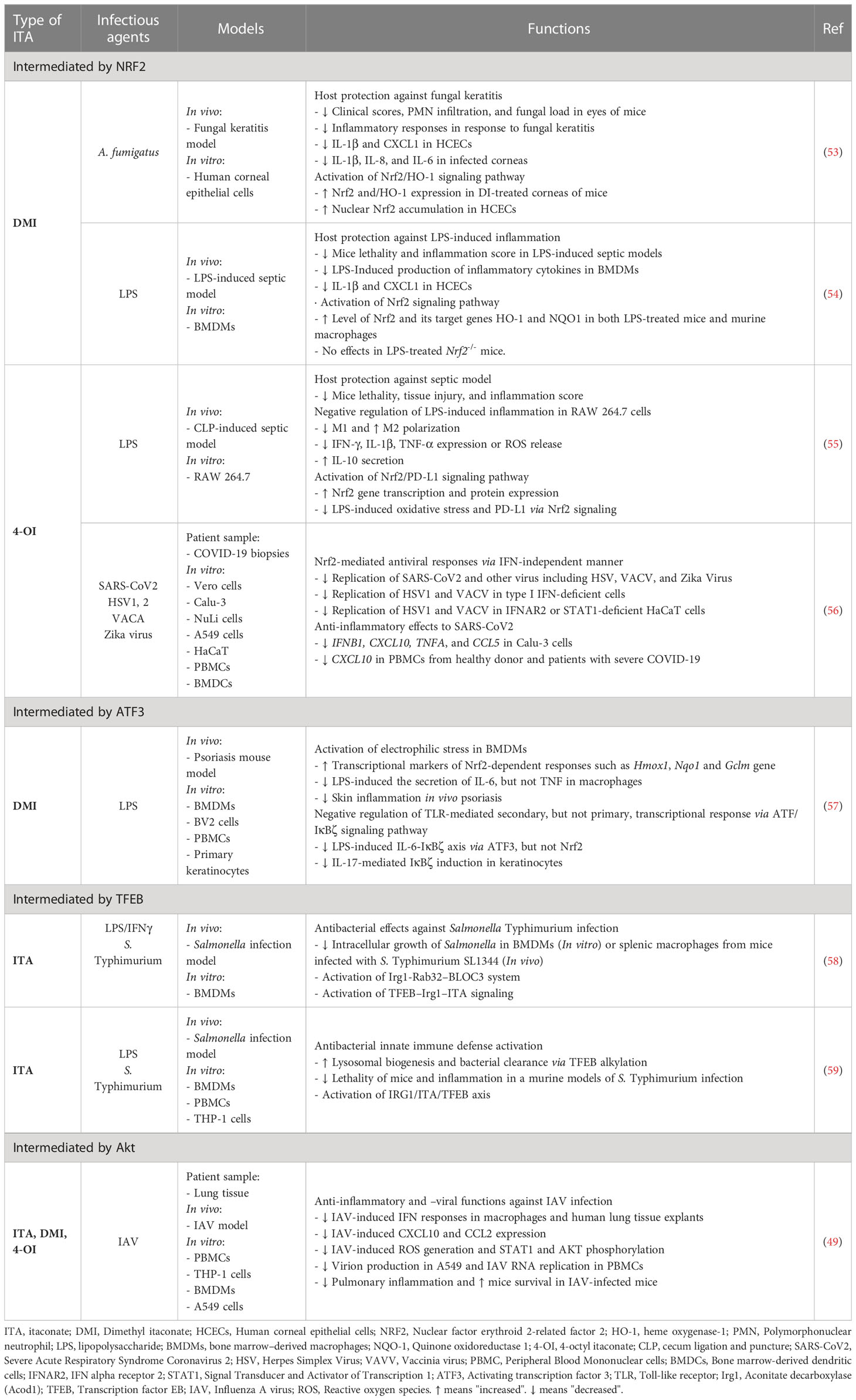
Table 1 Host protective functions of itaconate and its relatives as therapeutic candidates in infectious/inflammatory diseases.
4.1 NRF2 and antioxidant responses
NRF2, a transcriptional factor with a cytoprotective function, is a focus of research on ITA-associated therapeutics for infection and inflammation. The NRF2 protein level and activity are regulated by ubiquitination and degradation by E3 ligase complexes involving Kelch ECH associating protein 1 (KEAP1) (52, 60). However, the underlying regulatory mechanisms are beyond the scope of this review.
ITA-induced alkylation of KEAP1 activates the NRF2 signaling pathway of antioxidant and anti-inflammatory responses (20, 57, 61). Esterified derivatives of ITA, 4-OI, and DMI, are sufficient to activate the NRF2 signaling pathway. For instance, in a model of Aspergillus fumigatus keratitis, DMI reduces inflammatory responses in human corneal epithelial cells by activating of NRF2/heme oxygenase (HO)-1 signaling (53). An NRF2 signaling pathway is also important for DMI-mediated anti-inflammatory responses to LPS in macrophages, and DMI induces NRF2, HO-1, and NAD(P)H quinone oxydoreductase 1 (NQO-1), expression, downstream signaling factors of NRF2 signaling (54).
4-OI functions in the resolution of wounds in macrophages. 4-OI suppresses TNF-α, but not IL-6, production via NRF2 signaling. 4-OI increases the expression of the immunosuppressive M2 markers TGF-β and CD36, but suppresses collagenase matrix metalloprotease-8 in human monocyte-derived macrophages. In addition, 4-OI alleviates the LPS-induced uptake of fibrous collagen via the NRF2 and p38 MAPK signaling pathways (62). In a sepsis model, 4-OI inhibits inflammatory and oxidative stress factors, but increases anti-inflammatory responses, by activating NRF2 signaling (55). Interestingly, 4-OI exerts an antiviral effect against severe acute respiratory syndrome coronavirus-2 (SARS-CoV-2) infection by suppressing host inflammatory responses via NRF2 signaling (56). 4-OI and dimethyl fumarate exert antiviral effects against herpes simplex viruses-1 and -2, vaccinia virus, and Zika virus by controlling inflammatory responses (56). Moreover, NRF2 activation suppresses STING expression and signaling, an effect mimicked by NRF2 inducers or 4-OI, to affect STING-dependent inflammatory responses (61). The cyclic guanosine monophosphate-adenosine monophosphate (cGAMP) synthase (cGAS)/STING system is a therapeutic target for IFN-related inflammatory and bacterial infections (61, 63). More data are needed to clarify whether ITA and its family members protect against bacterial infections.
4.2 ATF3
ATF3 is a stress-responsive transcription factor of the basic leucine zipper (bZip) family and is essential for controlling physiological functions such as the cell cycle, tumor suppression, and TLR4 signaling (64, 65). An ATF3-mediated signaling pathway regulates the production of inflammatory cytokines, such as IL-6, mediated by ITA and DMI, both of which induce electrophilic stress (57). Whereas TNF is induced by TLR stimulation, IL-6 is produced as a result of secondary transcriptional responses, mainly mediated by IκBζ, which is encoded by Nfkbiz (66). Importantly, DMI-mediated ATF3 upregulation suppresses IL-17–mediated IκBζ signaling pathway activation, thus ameliorating skin pathological inflammation in vitro and in vivo (57). Therefore, the ATF3/IκBζ pathway is a target by which ITA and its derivatives regulate the generation of proinflammatory cytokines.
Mesaconate and citraconic, two isomers of ITA, are immunomodulatory metabolites. They suppress the production of inflammatory cytokines and IFN signaling, and the release of IAV particles from host cells. The anti-inflammatory and antioxidant effects of ITA isomers depend on the NRF2 signaling pathway, and citraconic is the most active NRF2 agonist (67). Mesaconate downregulates glycolysis but does not suppress tricarboxylic acid cycle activity or SDH. Mesaconate significantly reduces the secretion of IL-6 and IL-12 and increases CXCL10 in macrophages. However, this effect is not mediated by NRF2 and ATF3 (68). These data suggest that ITA isomers modulate the NRF2 and ATF3 signaling pathways to influence immune responses in a context-dependent manner.
4.3 TFEB
TFEB is a transcription factor of the microphthalmia (MiT/TFE) family (69) that regulates lysosomal biogenesis and autophagy by binding to the CLEAR (coordinated lysosomal expression and regulation) element, which is found in the promoters of lysosomal genes (70, 71). TFEB activation alters carbon funneling to elevate the level of ITA, thereby suppressing S. Typhimurium, an intracellular pathogen, in macrophages and in vivo (58). Interestingly, S. Typhimurium restricts TFEB activity, however, TFEB activation alone is enough to induce Irg1 and increase the ITA level in macrophages (58). Also, iNOS expression suppresses endogenous ITA synthesis in activated murine macrophages (58). The IRG1-Rab32–BLOC3 pathway is involved in the TFEB-driven ITA transport from mitochondria into Salmonella-containing vacuoles to restrict bacterial growth (58). Zhang et al. reported that ITA produced by LPS-stimulated cells induces the alkylation of human TFEB at Cys212, to drive its nuclear translocation and activation, thus suppressing S. Typhimurium infection (59). Therefore, the TFEB-associated lysosomal function and ITA synthesis could be leveraged to develop therapeutics for intracellular bacteria.
4.4 Akt signaling pathway
Akt/protein kinase B, a downstream serine/threonine protein kinase of phosphoinositide 3-kinase (PI3K), is important in cell growth and survival, cell cycle progression, glucose metabolism, and immune responses (72, 73). Aberrant activation of the Akt pathway contributes to multiple pathological processes during infection, including inflammatory responses, viral propagation (74, 75), and increased intracellular bacterial survival (76, 77). By contrast, the Akt/mTOR-mediated signaling pathway contributes to the non-canonical activation of IFN-dependent antiviral responses (78). IAV-induced pathological inflammation in the lung is increased in IRG1-deficient compared to wild-type mice (49). Importantly, DMI and 4-OI exert the same protective effect as ITA and reduce IFN and inflammatory responses in human PBMCs and lung tissue (49). Mechanistically, both DMI and 4-OI suppress, whereas ITA increases, the phosphorylation of Akt in human monocytic THP1 cells (49). The regulatory effects of ITA and its relatives need to be characterized in terms of Akt signaling modulation and its consequences in viral and bacterial infection.
5 Discussion
The roles of ITA and its family members in infection and inflammation have been investigated extensively, but their roles in host defense and pathogenesis during infection are unclear. ITA and its family members exert antimicrobial effects during viral, bacterial, and parasitic infections. However, the IRG1-ITA pathway promotes the pathogenesis of infection in a context-dependent manner. These findings suggest that the complex immunometabolic environment determines the role of IRG1 and ITA in the modulation of host defense against infection. Several pathogens can use ITA as a carbon source during infection. There is no report that esterified derivatives of ITA (4-OI and DMI) are directly used by intracellular pathogens. Therefore, ITA derivatives with similar activities as endogenous ITA show promise as host-directed therapeutics for infectious diseases.
Although the mechanisms by which ITA and its relatives promote host defense against pathogens are unclear, at least four factors—NRF2, ATF3, TFEB, and Akt—are implicated. The esterified forms (4-OI and DMI) are used to surmount the low cell permeability and mimic the functions of ITA. 4-OI inhibits inflammation by alkylating GAPDH and exerts antiviral properties through NRF2 signaling. DMI elicits NRF2 and ATF3 activation in response to bacterial infection, promoting host defense. The two derivatives decreased the phosphorylation of Akt, whereas ITA increases it. Detailed regulation of cellular signaling and comparisons of preclinical and clinical outcomes will further illuminate the unique function of each derivative. It is likely that additional signaling pathways, metabolic remodeling, and factors are involved and should be investigated in greater depth. Further clinical trials are needed to clarify whether ITA and its relatives contribute to antimicrobial or tolerogenic responses during infection. Such efforts will facilitate the development of ITA-based antimicrobials that enhance host immune responses. Overall, the study of ITA and its family members in the context of host defense against infections represents an intriguing area of research with promising implications for the development of novel therapeutic strategies.
Author contributions
All authors contributed in their order in writing the manuscript. J-MY prepared the table, and E-JP and ISK prepared the figure. All authors wrote this manuscript. E-KJ conceived, wrote this manuscript and is the corresponding author. All authors read and approved the final manuscript.
Funding
This work is supported by a grant of the Korea Health Technology R&D Project through the Korea Health Industry Development Institute (KHIDI), funded by the Ministry of Health & Welfare, Republic of Korea (HI22C1361) and by the National Research Foundation of Korea (NRF) grant funded by the Korea government (MSIT) (No. 2017R1A5A2015385).
Conflict of interest
The authors declare that the research was conducted in the absence of any commercial or financial relationships that could be construed as a potential conflict of interest.
Publisher’s note
All claims expressed in this article are solely those of the authors and do not necessarily represent those of their affiliated organizations, or those of the publisher, the editors and the reviewers. Any product that may be evaluated in this article, or claim that may be made by its manufacturer, is not guaranteed or endorsed by the publisher.
Glossary
References
1. Cameron AM, Lawless SJ, Pearce EJ. Metabolism and acetylation in innate immune cell function and fate. Semin Immunol (2016) 28(5):408–16. doi: 10.1016/j.smim.2016.10.003
2. Mohammadnezhad L, Shekarkar Azgomi M, La Manna MP, Sireci G, Rizzo C, Badami GD, et al. Metabolic reprogramming of innate immune cells as a possible source of new therapeutic approaches in autoimmunity. Cells (2022) 11(10):1663. doi: 10.3390/cells11101663
3. Michaeloudes C, Bhavsar PK, Mumby S, Xu B, Hui CKM, Chung KF, et al. Role of metabolic reprogramming in pulmonary innate immunity and its impact on lung diseases. J Innate Immun (2020) 12(1):31–46. doi: 10.1159/000504344
4. Chen J, Vitetta L. The role of butyrate in attenuating pathobiont-induced hyperinflammation. Immune Netw (2020) 20(2):e15. doi: 10.4110/in.2020.20.e15
5. Iacob S, Iacob DG. Infectious threats, the intestinal barrier, and its Trojan horse: dysbiosis. Front Microbiol (2019) 10:1676. doi: 10.3389/fmicb.2019.01676
6. Cordes T, Michelucci A, Hiller K. Itaconic acid: the surprising role of an industrial compound as a mammalian antimicrobial metabolite. Annu Rev Nutr (2015) 35:451–73. doi: 10.1146/annurev-nutr-071714-034243
7. Seim GL, Britt EC, John SV, Yeo FJ, Johnson AR, Eisenstein RS, et al. Two-stage metabolic remodelling in macrophages in response to lipopolysaccharide and interferon-gamma stimulation. Nat Metab (2019) 1(7):731–42. doi: 10.1038/s42255-019-0083-2
8. Shi X, Zhou H, Wei J, Mo W, Li Q, Lv X. The signaling pathways and therapeutic potential of itaconate to alleviate inflammation and oxidative stress in inflammatory diseases. Redox Biol (2022) 58:102553. doi: 10.1016/j.redox.2022.102553
9. McGettrick AF, O'Neill LA. Two for the price of one: itaconate and its derivatives as an anti-infective and anti-inflammatory immunometabolite. Curr Opin Immunol (2023) 80:102268. doi: 10.1016/j.coi.2022.102268
10. Shi J, Cai C. Research progress on the mechanism of itaconate regulating macrophage immunometabolism. Front Immunol (2022) 13:937247. doi: 10.3389/fimmu.2022.937247
11. Michelucci A, Cordes T, Ghelfi J, Pailot A, Reiling N, Goldmann O, et al. Immune-responsive gene 1 protein links metabolism to immunity by catalyzing itaconic acid production. Proc Natl Acad Sci USA (2013) 110(19):7820–5. doi: 10.1073/pnas.1218599110
12. Dickman SR, Cloutier AA. Factors affecting the activity of aconitase. J Biol Chem (1951) 188(1):379–88. doi: 10.1016/S0021-9258(18)56180-9
13. Thomson JF, Nance SL, Bush KJ, Szczepanik PA. Isotope and solvent effects of deuterium on aconitase. Arch Biochem Biophys (1966) 117(1):65–74. doi: 10.1016/0003-9861(66)90126-3
14. Villafranca JJ, Mildvan AS. The mechanism of aconitase action. II. magnetic resonance studies of the complexes of enzyme, manganese(II), iron(II), and substrates. J Biol Chem (1971) 246(18):5791–8. doi: 10.1016/S0021-9258(18)61874-5
15. Wu R, Chen F, Wang N, Tang D, Kang R. ACOD1 in immunometabolism and disease. Cell Mol Immunol (2020) 17(8):822–33. doi: 10.1038/s41423-020-0489-5
16. Cordes T, Wallace M, Michelucci A, Divakaruni AS, Sapcariu SC, Sousa C, et al. Immunoresponsive gene 1 and itaconate inhibit succinate dehydrogenase to modulate intracellular succinate levels. J Biol Chem (2016) 291(27):14274–84. doi: 10.1074/jbc.M115.685792
17. Lampropoulou V, Sergushichev A, Bambouskova M, Nair S, Vincent EE, Loginicheva E, et al. Itaconate links inhibition of succinate dehydrogenase with macrophage metabolic remodeling and regulation of inflammation. Cell Metab (2016) 24(1):158–66. doi: 10.1016/j.cmet.2016.06.004
18. Cordes T, Metallo CM. Itaconate alters succinate and coenzyme a metabolism via inhibition of mitochondrial complex II and methylmalonyl-CoA mutase. Metabolites (2021) 11(2):117. doi: 10.3390/metabo11020117
19. ElAzzouny M, Tom CT, Evans CR, Olson LL, Tanga MJ, Gallagher KA, et al. Dimethyl itaconate is not metabolized into itaconate intracellularly. J Biol Chem (2017) 292(12):4766–9. doi: 10.1074/jbc.C117.775270
20. Mills EL, Ryan DG, Prag HA, Dikovskaya D, Menon D, Zaslona Z, et al. Itaconate is an anti-inflammatory metabolite that activates Nrf2 via alkylation of KEAP1. Nature (2018) 556(7699):113–7. doi: 10.1038/nature25986
21. Li Y, Gong W, Li W, Liu P, Liu J, Jiang H, et al. The IRG1-itaconate axis: a regulatory hub for immunity and metabolism in macrophages. Int Rev Immunol (2022), 1–15. doi: 10.1080/08830185.2022.2067153
22. Kim YJ, Park EJ, Lee SH, Silwal P, Kim JK, Yang JS, et al. Dimethyl itaconate is effective in host-directed antimicrobial responses against mycobacterial infections through multifaceted innate immune pathways. Cell Biosci (2023) 13(1):49. doi: 10.1186/s13578-023-00992-x
23. Urso A, Prince A. Anti-inflammatory metabolites in the pathogenesis of bacterial infection. Front Cell Infect Microbiol (2022) 12:925746. doi: 10.3389/fcimb.2022.925746
24. Bailey JD, Diotallevi M, Nicol T, McNeill E, Shaw A, Chuaiphichai S, et al. Nitric oxide modulates metabolic remodeling in inflammatory macrophages through TCA cycle regulation and itaconate accumulation. Cell Rep (2019) 28(1):218–30 e7. doi: 10.1016/j.celrep.2019.06.018
25. Jiang Q, Qiu Y, Kurland IJ, Drlica K, Subbian S, Tyagi S, et al. Glutamine is required for M1-like polarization of macrophages in response to Mycobacterium tuberculosis infection. mBio (2022) 13(4):e0127422. doi: 10.1128/mbio.01274-22
26. Heinz A, Nonnenmacher Y, Henne A, Khalil MA, Bejkollari K, Dostert C, et al. Itaconate controls its own synthesis via feedback-inhibition of reverse TCA cycle activity at IDH2. Biochim Biophys Acta Mol Basis Dis (2022) 1868(12):166530. doi: 10.1016/j.bbadis.2022.166530
27. Faas M, Ipseiz N, Ackermann J, Culemann S, Gruneboom A, Schroder F, et al. IL-33-induced metabolic reprogramming controls the differentiation of alternatively activated macrophages and the resolution of inflammation. Immunity (2021) 54(11):2531–46 e5. doi: 10.1016/j.immuni.2021.09.010
28. Liao ST, Han C, Xu DQ, Fu XW, Wang JS, Kong LY. 4-octyl itaconate inhibits aerobic glycolysis by targeting GAPDH to exert anti-inflammatory effects. Nat Commun (2019) 10(1):5091. doi: 10.1038/s41467-019-13078-5
29. Stocks CJ, Schembri MA, Sweet MJ, Kapetanovic R. For when bacterial infections persist: toll-like receptor-inducible direct antimicrobial pathways in macrophages. J Leukoc Biol (2018) 103(1):35–51. doi: 10.1002/JLB.4RI0917-358R
30. Wu R, Liu J, Wang N, Zeng L, Yu C, Chen F, et al. Aconitate decarboxylase 1 is a mediator of polymicrobial sepsis. Sci Transl Med (2022) 14(659):eabo2028. doi: 10.1126/scitranslmed.abo2028
31. Bomfim CCB, Fisher L, Amaral EP, Mittereder L, McCann K, Correa AAS, et al. Mycobacterium tuberculosis induces Irg1 in murine macrophages by a pathway involving both TLR-2 and STING/IFNAR signaling and requiring bacterial phagocytosis. Front Cell Infect Microbiol (2022) 12:862582. doi: 10.3389/fcimb.2022.862582
32. Lacey CA, Ponzilacqua-Silva B, Chambers CA, Dadelahi AS, Skyberg JA. MyD88-dependent glucose restriction and itaconate production control Brucella infection. Infect Immun (2021) 89(10):e0015621. doi: 10.1128/IAI.00156-21
33. Dominguez-Andres J, Novakovic B, Li Y, Scicluna BP, Gresnigt MS, Arts RJW, et al. The itaconate pathway is a central regulatory node linking innate immune tolerance and trained immunity. Cell Metab (2019) 29(1):211–20 e5. doi: 10.1016/j.cmet.2018.09.003
34. Jankowski J, Kubinska M, Juskiewicz J, Czech A, Zdunczyk Z. The effect of dietary methionine levels on fattening performance and selected blood and tissue parameters of turkeys. Arch Anim Nutr (2016) 70(2):127–40. doi: 10.1080/1745039X.2015.1134399
35. Gidon A, Louet C, Rost LM, Bruheim P, Flo TH. The tumor necrosis factor alpha and interleukin 6 auto-paracrine signaling loop controls Mycobacterium avium infection via induction of IRF1/IRG1 in human primary macrophages. mBio (2021) 12(5):e0212121. doi: 10.1128/mBio.02121-21
36. Nair S, Huynh JP, Lampropoulou V, Loginicheva E, Esaulova E, Gounder AP, et al. Irg1 expression in myeloid cells prevents immunopathology during m. tuberculosis infection. J Exp Med (2018) 215(4):1035–45. doi: 10.1084/jem.20180118
37. Chen M, Sun H, Boot M, Shao L, Chang SJ, Wang W, et al. Itaconate is an effector of a rab GTPase cell-autonomous host defense pathway against salmonella. Science (2020) 369(6502):450–55. doi: 10.1126/science.aaz1333
38. Jessop F, Buntyn R, Schwarz B, Wehrly T, Scott D, Bosio CM. Interferon gamma reprograms host mitochondrial metabolism through inhibition of complex II to control intracellular bacterial replication. Infect Immun (2020) 88(2):e00744-19. doi: 10.1128/IAI.00744-19
39. Demars A, Vitali A, Comein A, Carlier E, Azouz A, Goriely S, et al. Aconitate decarboxylase 1 participates in the control of pulmonary Brucella infection in mice. PloS Pathog (2021) 17(9):e1009887. doi: 10.1371/journal.ppat.1009887
40. Nguyen TV, Alfaro AC, Young T, Green S, Zarate E, Merien F. Itaconic acid inhibits growth of a pathogenic marine Vibrio strain: a metabolomics approach. Sci Rep (2019) 9(1):5937. doi: 10.1038/s41598-019-42315-6
41. O'Callaghan AA, Dempsey E, Iyer N, Stiegeler S, Mercurio K, Corr SC. Intestinal metabolites influence macrophage phagocytosis and clearance of bacterial infection. Front Cell Infect Microbiol (2021) 11:622491. doi: 10.3389/fcimb.2021.622491
42. Daniels BP, Kofman SB, Smith JR, Norris GT, Snyder AG, Kolb JP, et al. The nucleotide sensor ZBP1 and kinase RIPK3 induce the enzyme IRG1 to promote an antiviral metabolic state in neurons. Immunity (2019) 50(1):64–76 e4. doi: 10.1016/j.immuni.2018.11.017
43. Kohl L, Siddique M, Bodendorfer B, Berger R, Preikschat A, Daniel C, et al. Macrophages inhibit Coxiella burnetii by the ACOD1-itaconate pathway for containment of q fever. EMBO Mol Med (2023) 15(2):e15931. doi: 10.15252/emmm.202215931
44. He Y, Xu D, Yan Z, Wu Y, Zhang Y, Tian X, et al. A metabolite attenuates neuroinflammation, synaptic loss and cognitive deficits induced by chronic infection of toxoplasma gondii. Front Immunol (2022) 13:1043572. doi: 10.3389/fimmu.2022.1043572
45. Collins JM, Jones DP, Sharma A, Khadka M, Liu KH, Kempker RR, et al. TCA cycle remodeling drives proinflammatory signaling in humans with pulmonary tuberculosis. PloS Pathog (2021) 17(9):e1009941. doi: 10.1371/journal.ppat.1009941
46. Ren K, Lv Y, Zhuo Y, Chen C, Shi H, Guo L, et al. Suppression of IRG-1 reduces inflammatory cell infiltration and lung injury in respiratory syncytial virus infection by reducing production of reactive oxygen species. J Virol (2016) 90(16):7313–22. doi: 10.1128/JVI.00563-16
47. Riquelme SA, Lozano C, Moustafa AM, Liimatta K, Tomlinson KL, Britto C, et al. CFTR-PTEN-dependent mitochondrial metabolic dysfunction promotes pseudomonas aeruginosa airway infection. Sci Transl Med (2019) 11(499):eaav4634. doi: 10.1126/scitranslmed.aav4634
48. Wong Fok Lung T, Charytonowicz D, Beaumont KG, Shah SS, Sridhar SH, Gorrie CL, et al. Klebsiella pneumoniae induces host metabolic stress that promotes tolerance to pulmonary infection. Cell Metab (2022) 34(5):761–74 e9. doi: 10.1016/j.cmet.2022.03.009
49. Sohail A, Iqbal AA, Sahini N, Chen F, Tantawy M, Waqas SFH, et al. Itaconate and derivatives reduce interferon responses and inflammation in influenza a virus infection. PloS Pathog (2022) 18(1):e1010219. doi: 10.1371/journal.ppat.1010219
50. Riquelme SA, Liimatta K, Wong Fok Lung T, Fields B, Ahn D, Chen D, et al. Pseudomonas aeruginosa utilizes host-derived itaconate to redirect its metabolism to promote biofilm formation. Cell Metab (2020) 31(6):1091–106 e6. doi: 10.1016/j.cmet.2020.04.017
51. Tomlinson KL, Lung TWF, Dach F, Annavajhala MK, Gabryszewski SJ, Groves RA, et al. Staphylococcus aureus induces an itaconate-dominated immunometabolic response that drives biofilm formation. Nat Commun (2021) 12(1):1399. doi: 10.1038/s41467-021-21718-y
52. Roberts LM, Leighton I, Schwarz B, Wehrly TD, Evans TJ, Bosio CM. Itaconate indirectly influences expansion of effector T cells following vaccination with francisella tularensis live vaccine strain. Cell Immunol (2022) 373:104485. doi: 10.1016/j.cellimm.2022.104485
53. Gu L, Lin J, Wang Q, Li C, Peng X, Fan Y, et al. Dimethyl itaconate protects against fungal keratitis by activating the Nrf2/HO-1 signaling pathway. Immunol Cell Biol (2020) 98(3):229–41. doi: 10.1111/imcb.12316
54. Zhang S, Jiao Y, Li C, Liang X, Jia H, Nie Z, et al. Dimethyl itaconate alleviates the inflammatory responses of macrophages in sepsis. Inflammation (2021) 44(2):549–57. doi: 10.1007/s10753-020-01352-4
55. Zhang P, Wang Y, Yang W, Yin Y, Li C, Ma X, et al. 4-octyl itaconate regulates immune balance by activating Nrf2 and negatively regulating PD-L1 in a mouse model of sepsis. Int J Biol Sci (2022) 18(16):6189–209. doi: 10.7150/ijbs.74456
56. Olagnier D, Farahani E, Thyrsted J, Blay-Cadanet J, Herengt A, Idorn M, et al. SARS-CoV2-mediated suppression of NRF2-signaling reveals potent antiviral and anti-inflammatory activity of 4-octyl-itaconate and dimethyl fumarate. Nat Commun (2020) 11(1):4938. doi: 10.1038/s41467-020-18764-3
57. Bambouskova M, Gorvel L, Lampropoulou V, Sergushichev A, Loginicheva E, Johnson K, et al. Electrophilic properties of itaconate and derivatives regulate the IkappaBzeta-ATF3 inflammatory axis. Nature (2018) 556(7702):501–4. doi: 10.1038/s41586-018-0052-z
58. Schuster EM, Epple MW, Glaser KM, Mihlan M, Lucht K, Zimmermann JA, et al. TFEB induces mitochondrial itaconate synthesis to suppress bacterial growth in macrophages. Nat Metab (2022) 4(7):856–66. doi: 10.1038/s42255-022-00605-w
59. Zhang Z, Chen C, Yang F, Zeng YX, Sun P, Liu P, et al. Itaconate is a lysosomal inducer that promotes antibacterial innate immunity. Mol Cell (2022) 82(15):2844–57 e10. doi: 10.1016/j.molcel.2022.05.009
60. Kobayashi A, Ohta T, Yamamoto M. Unique function of the Nrf2-Keap1 pathway in the inducible expression of antioxidant and detoxifying enzymes. Methods Enzymol (2004) 378:273–86. doi: 10.1016/S0076-6879(04)78021-0
61. Olagnier D, Brandtoft AM, Gunderstofte C, Villadsen NL, Krapp C, Thielke AL, et al. Nrf2 negatively regulates STING indicating a link between antiviral sensing and metabolic reprogramming. Nat Commun (2018) 9(1):3506. doi: 10.1038/s41467-018-05861-7
62. Maassen S, Coenen B, Ioannidis M, Harber K, Grijpstra P, Van den Bossche J, et al. Itaconate promotes a wound resolving phenotype in pro-inflammatory macrophages. Redox Biol (2023) 59:102591. doi: 10.1016/j.redox.2022.102591
63. Liu N, Pang X, Zhang H, Ji P. The cGAS-STING pathway in pacterial infection and bacterial immunity. Front Immunol (2021) 12:814709. doi: 10.3389/fimmu.2021.814709
64. Hai T, Wolford CC, Chang YS. ATF3, a hub of the cellular adaptive-response network, in the pathogenesis of diseases: is modulation of inflammation a unifying component? Gene Expr (2010) 15(1):1–11. doi: 10.3727/105221610X12819686555015
65. Gilchrist M, Thorsson V, Li B, Rust AG, Korb M, Roach JC, et al. Systems biology approaches identify ATF3 as a negative regulator of toll-like receptor 4. Nature (2006) 441(7090):173–8. doi: 10.1038/nature04768
66. Yamamoto M, Yamazaki S, Uematsu S, Sato S, Hemmi H, Hoshino K, et al. Regulation of Toll/IL-1-receptor-mediated gene expression by the inducible nuclear protein IkappaBzeta. Nature (2004) 430(6996):218–22. doi: 10.1038/nature02738
67. Chen F, Elgaher WAM, Winterhoff M, Bussow K, Waqas FH, Graner E, et al. Citraconate inhibits ACOD1 (IRG1) catalysis, reduces interferon responses and oxidative stress, and modulates inflammation and cell metabolism. Nat Metab (2022) 4(5):534–46. doi: 10.1038/s42255-022-00577-x
68. He W, Henne A, Lauterbach M, Geissmar E, Nikolka F, Kho C, et al. Mesaconate is synthesized from itaconate and exerts immunomodulatory effects in macrophages. Nat Metab (2022) 4(5):524–33. doi: 10.1038/s42255-022-00565-1
69. Steingrimsson E, Copeland NG, Jenkins NA. Melanocytes and the microphthalmia transcription factor network. Annu Rev Genet (2004) 38:365–411. doi: 10.1146/annurev.genet.38.072902.092717
70. Sardiello M, Palmieri M, di Ronza A, Medina DL, Valenza M, Gennarino VA, et al. A gene network regulating lysosomal biogenesis and function. Science (2009) 325(5939):473–7. doi: 10.1126/science.1174447
71. Palmieri M, Impey S, Kang H, di Ronza A, Pelz C, Sardiello M, et al. Characterization of the CLEAR network reveals an integrated control of cellular clearance pathways. Hum Mol Genet (2011) 20(19):3852–66. doi: 10.1093/hmg/ddr306
72. Porta C, Paglino C, Mosca A. Targeting PI3K/Akt/mTOR signaling in cancer. Front Oncol (2014) 4:64. doi: 10.3389/fonc.2014.00064
73. Mohseni AH, Casolaro V, Bermudez-Humaran LG, Keyvani H, Taghinezhad SS. Modulation of the PI3K/Akt/mTOR signaling pathway by probiotics as a fruitful target for orchestrating the immune response. Gut Microbes (2021) 13(1):1–17. doi: 10.1080/19490976.2021.1886844
74. Ehrhardt C, Ludwig S. A new player in a deadly game: influenza viruses and the PI3K/Akt signalling pathway. Cell Microbiol (2009) 11(6):863–71. doi: 10.1111/j.1462-5822.2009.01309.x
75. Ye J, Zhang H, He W, Zhu B, Zhou D, Chen Z, et al. Quantitative phosphoproteomic analysis identifies the critical role of JNK1 in neuroinflammation induced by Japanese encephalitis virus. Sci Signal (2016) 9(448):ra98. doi: 10.1126/scisignal.aaf5132
76. Silwal P, Paik S, Kim JK, Yoshimori T, Jo EK. Regulatory mechanisms of autophagy-targeted antimicrobial therapeutics against mycobacterial infection. Front Cell Infect Microbiol (2021) 11:633360. doi: 10.3389/fcimb.2021.633360
77. Robinson KS, Aw R. The commonalities in bacterial effector inhibition of apoptosis. Trends Microbiol (2016) 24(8):665–80. doi: 10.1016/j.tim.2016.04.002
Keywords: itaconate, innate immunity, toll-like receptor, inflammation, host defense
Citation: Yuk J-M, Park E-J, Kim IS and Jo E-K (2023) Itaconate family-based host-directed therapeutics for infections. Front. Immunol. 14:1203756. doi: 10.3389/fimmu.2023.1203756
Received: 11 April 2023; Accepted: 04 May 2023;
Published: 16 May 2023.
Edited by:
Cheng Zhixue, Third Affiliated Hospital of Sun Yat-sen University, ChinaReviewed by:
Xiangmin Lin, Fujian Agriculture and Forestry University, ChinaJinzhou Ye, Shenzhen Bay Laboratory, China
Copyright © 2023 Yuk, Park, Kim and Jo. This is an open-access article distributed under the terms of the Creative Commons Attribution License (CC BY). The use, distribution or reproduction in other forums is permitted, provided the original author(s) and the copyright owner(s) are credited and that the original publication in this journal is cited, in accordance with accepted academic practice. No use, distribution or reproduction is permitted which does not comply with these terms.
*Correspondence: Eun-Kyeong Jo, aGF5b3VuZ2pAY251LmFjLmty