- 1Center for Musculoskeletal Surgery, Clinic for Orthopedics, Charité University Hospital, Berlin, Germany
- 2Department of Orthopedics, Auckland City Hospital, Auckland, New Zealand
- 3BIH Charité Clinician Scientist Program, BIH Biomedical Innovation Academy, Berlin Institute of Health, Charité — Universitätsmedizin Berlin, Berlin, Germany
The immune system is closely linked to bone homeostasis and plays a pivotal role in several pathological and inflammatory conditions. Through various pathways it modulates various bone cells and subsequently sustains the physiological bone metabolism. Myeloid-derived suppressor cells (MDSCs) are a group of heterogeneous immature myeloid-derived cells that can exert an immunosuppressive function through a direct cell-to-cell contact, secretion of anti-inflammatory cytokines or specific exosomes. These cells mediate the innate immune response to chronic stress on the skeletal system. In chronic inflammation, MDSCs act as an inner offset to rebalance overactivation of the immune system. Moreover, they have been found to be involved in processes responsible for bone remodeling in different musculoskeletal disorders, autoimmune diseases, infection, and cancer. These cells can not only cause bone erosion by differentiating into osteoclasts, but also alleviate the immune reaction, subsequently leading to long-lastingly impacted bone remodeling. In this review, we discuss the impact of MDSCs on the bone metabolism under several pathological conditions, the involved modulatory pathways as well as potential therapeutic targets in MDSCs to improve bone health.
1 Introduction
Bone is a versatile organ that is an essential component for the ambulatory ability and is host to essential cell lineages such as hematopoietic stem cells, as well as bone cells and immune cells. The solid bone matrix is constantly being remodeled in response to changes in physical stress (1). This self-regulated biological remodeling process is mainly driven by bone resorption and formation. While osteoclasts (OCs) eliminate damaged or aged bone tissue, osteoblasts (OBs) are responsible for secretion of new bone matrix and mediation of matrix calcification (2). Both cell types are vital for responding to biomechanical or metabolic changes, remodeling the microstructure of the bone accordingly, and maintaining bone homeostasis.
This equilibrium is governed by several cells and mediating cytokines (3). In particular, the immune system interacts tightly with the bone metabolism (4–6). However, in various pathologies such as tumor metastasis or local inflammation, this delicate equilibrium is distorted (7, 8). Besides focusing on the causative disease, recent research has also focused on identifying key regulatory players to influence bone homeostasis (9, 10). Myeloid derived suppressor cells (MDSCs), a group of immature cells of the myeloid lineage, represent a cell type with immune regulatory function through interaction with effector or regulatory lymphocytes. These cells are activated and proliferate in diseases, including chronic bacterial infection, autoimmune diseases, and cancer (11–15).
Recent studies have described the role of MDSCs in bone-related disease. Bone lesions ranging from systemic bone loss (osteoporosis, autoimmune diseases) to local destruction (osteomyelitis, implant related infection, bone fracture and bone metastasis of tumor) can create a long-lasting inflammatory environment (4, 6, 16, 17). These signals play a key role in myeloid lineage cell activation and differentiation to MDSCs, which in turn impact disease progression and the regenerative capabilities of bone. MDSCs can interact with nearby lymphocytes in the bone, indirectly influencing the bone metabolism through stimulation of the immune system. Additionally, MDSCs were found to impact bone directly, i.e., by differentiating into osteoclasts, or secreting cytokines. In this review, we aim to illustrate how MDSCs can affect bone health and their role in musculoskeletal morbidities.
2 Bone remodeling and its interaction with the immune system
Bone serves as one of the most important immune organs as the origin of several immune cells is the bone cavity and its metabolic activity is closely linked to the immune system. The recently coined term “osteoimmunology” connects the metabolic activity of the bone with the immune system (18). The bone forms a relatively closed space that supplies a suitable cradle for the reciprocal interactions of immune cells and bone cells.
Mediators secreted by bone cells can either stimulate or obstruct processes of immune development. Bone cells contribute to the maturation and expansion of various immune cells derived from hematopoietic stem cells (HSCs). Mesenchymal stromal cells expressing the C-X-C motif chemokine-12 (CXCL-12) are required for HSC maintenance (19). Additionally, OBs are essential in maintaining common lymphoid progenitors (CLPs) through expression of IL-7 and CXCL-12 (20). Ablation of OBs results in severely decreased hematopoiesis in the bone marrow, in particular the generation of B cells (21). Osteocytes also support the lymphocyte development and show positive impact on B cell generation (22, 23). Moreover, OCs are fundamental to create bone marrow cavities sufficient in size for HSCs to sustain their physiological capabilities and indirectly support HSCs by recruiting osteoblasts (24). They are also engaged in establishing a livable milieu in the bone to induce HSC homing and niche formation (25).
At the same time the immune system has significant impact on bone homeostasis (26). Over- or under-regulation of the immune system results in abnormal bone mineralization through different mechanisms. Different T cell populations including CD8+, CD4+ T helper cells (Th), and regulatory T cells (Treg) impact the bone metabolism through secretion of various cytokines. CD8+ T cells and Th17 favor osteoclastogenesis by secretion of tumor necrosis factor-α (TNF-α) and IL-17 (17, 27). B cells, as supportive regulators of osteoclasts, limit bone remodeling (28, 29). Macrophages are characterized into two phenotypes, proinflammatory M1 and anti-inflammatory M2, which support and hinder bone regeneration, respectively. Besides their phagocytic function, these cells also differentiate into osteoclasts and secrete TNF-α and various ILs balancing bone formation and resorption (30, 31).
In this regard, MDSCs, a type of immature myeloid cells, have recently started to attract attention due to their impact on the bone metabolism and their immunosuppressive capacities. First described as a key modulator in tumor microenvironment, the role of MDSCs is becoming undeniably important during disease progression due to their potential to regulate immune balance and crosstalk with the bone system.
3 MDSCs are induced in a chronic inflammatory setting
MDSCs were first discovered in a tumor mouse model. Aggregation of these cells around the tumor site lead to suppression of T-cell induced immunity and boosted cancer metastasis (32). While MDSC has become a comprehensive term to describe a specific origin, phenotype, and immunosuppressive capacities, it covers a heterogeneous group of distinct subphenotypes (33). Since several years, interest in MDSC-related immune regulation has been soaring in different disease settings, including chronic inflammatory diseases, infection and obesity (13). Deepening the understanding of the stimulating factors affecting differentiation of MDSCs may offer novel therapeutic targets.
Together with neutrophils and macrophages, MDSCs derive from the myeloid lineage but gain distinguished immunosuppressive functions during differentiation (34–36). Circulating MDSCs have been found in tumor, autoimmune, and septic patients but not or in very limited quantities in healthy individuals (37). In these chronic inflammatory environments, continuous low-grade stimulation of IMCs skews differentiation to increased generation of MDSCs (13). MDSCs generated under these conditions are poorly phagocytic and display potent immune-suppressive potential. Key factors involved in the differentiation of IMCs are granulocyte-macrophage colony stimulating factor (GM-CSF), G-CSF, and M-CSF (38–41), as well as inflammatory cytokines TNF-α, IL-1β, and IL-6 (41–43). These effectors from the microenvironment stimulate and regulate several intracellular pathways involving various key nodes that are crucial for the survival and immunosuppressive function of MDSCs (44–46).
MDSCs are commonly classified as granulocytic (G-MDSC, also known as polymorphonuclear MDSC, PMN-MDSC), monocytic (M-MDSC), and other subgroups such as early-stage MDSC (e-MDSC) and fibrocytic MDSC (F-MDSC) (47, 48). In humans, MDSCs expresses CD11b and CD33–markers related to immunosuppressive functions, while in mice, CD11b, Ly6C and Ly6G were defined as phenotypic markers (34, 49). Additionally, expression of CD84 has been recently identified on MDSCs in tumor settings (36). However, these markers alone cannot sufficiently phenotype all MDSC subpopulations (34). Besides their shared suppressive capabilities against adaptive immunity, their immunosuppressive capability differs in various nuances. In patients with head and neck cancer, PMN-MDSCs displayed the most prominent immunosuppressive features and have been associated with poor clinical outcome (50), while in a tumor mouse model, MDSCs with monocytic features showed heightened suppressive capability and blocked the T cell responses (51, 52).
4 Potential interactions of MDSCs with osteoclasts
Osteolysis occurs in several disease including osteoporosis, autoimmune arthritis, bone infection, and bone metastasis, where osteoclasts surpass the speed of regeneration of osteoblasts (7, 53). Related to the destruction of the cancellous bone microstructure, the trabeculae become thinner and more fragile with larger trabecular separation, subsequently manifesting in reduced bone volume (54, 55). MDSCs are osteoclast progenitors that can break the dynamic balance of bone remodeling in disease.
In inflammation, overactivated osteoclastogenesis can be observed, where monocytes and macrophages are functionally calibrated by various cytokines leading to activation of the receptor activator of nuclear factor kappa-B ligand (RANKL) pathway and receptor osteoprotegerin (OPG). T cells bind to RANK, the receptor of RANKL expressed on osteoclast progenitor cells, while OPG competitively binds to RANKL to hinder the stimulating effect of RANK (18). Other inflammatory components including TNF-α, IL-1, and IL-6 also disrupt the bone metabolism by triggering RANKL expression of osteoblasts, cell fusion, multinucleation, and functional activation of osteoclasts (56–58). The inflammatory cytokines stimulate osteoclasts to eliminate defective bone tissue. At the same time, bone regeneration is inhibited by interfering cells supporting the bone metabolism, particularly osteoblasts, osteocytes, and bone marrow mesenchymal stromal cells (BMSCs). Elevated levels of TNF-α, IL-1α, and IL-7 usually found in chronic inflammatory settings lead to osteoblast apoptosis, negatively affecting the osteogenic capacity of osteoblasts and differentiation of BMSCs (7, 59). Additionally, osteoblasts and osteocytes not only sustain the normal bone mineralization process, but also regulate osteoclast differentiation through secreting soluble proteins, inflammatory cytokines, and through direct cell-cell interactions (2, 60).
MDSCs mainly generate where myelopoiesis takes place including the bone marrow, spleen, and other lymphatic organs, but they can be also reprogrammed from mature myeloid cells in the periphery (37). Besides their immune modulatory ability, MDSCs can differentiate into mature and functional osteoclasts (61–65). An in vitro experiment using murine Gr1+CD11b+ MDSCs showed that a combination of RANKL and M-CSF can initiate differentiation into osteoclasts. Additionally, in a fluorescent mice model osteoclast generation was increased after MDSC injection, indicating MDSCs as an origin of these bone-resorbing cells (61). Likewise, allogenic transfusion can increase osteoclast differentiation in inflammation (62). Recently, obesity was also suggested to promote expansion of M-MDSCs and subsequent differentiation to osteoclasts (64, 65). MDSC-induced osteolysis is linked to chronic pathological diseases (36, 38). However, MDSCs are a heterogenous group consisting of several subgroups with different immune functions and capacity to differentiate to osteoclasts.
MDSCs and osteoclasts derive from the myeloid lineage, as do monocytes, macrophages, and dendritic cells. Both, MDSCs and osteoclasts share some common intracellular signaling pathways related to differentiation, proliferation, and osteoclastic cell functions. The osteoclastogenic capability of both cell types are repressed after treatment with bisphosphonates, suggesting a shared pathway in MDSCs and osteoclasts (63). Osteoclast differentiation of MDSCs is initiated by activation of the RANKL and NF-κB pathway (62). RANKL also activates the immune regulatory functions of MDSCs and promotes the expansion of M-MDSCs (66). The role of other pathways that have interactions with RANKL/RANK in osteoclast differentiation is of ongoing investigation (67). Additionally, MDSCs and OCs share similar immunosuppressive functions through secretion of the immunosuppressive cytokines IL-10 and transforming growth factor (TGF-β) (3). Both cell types are also capable of inhibiting the T cell mediated immune response. However, they also share immune regulatory features with mature myeloid cells that support the inflammatory environment. They have been shown to be able to sustain a proinflammatory environment under pathological conditions by presentation of antigens, secretion of proinflammatory cytokines, and inducing proliferation of T effector cells (3, 68).
5 MDSCs are a link between the immune and skeletal system
MDSCs also regulate other immune cell types which directly affect the musculoskeletal system. They modulate macrophage polarization from M1 to M2. Anti-inflammatory M2 macrophages stimulate the osteogenic capacity of BMSCs (69, 70). Interaction between MDSCs and regulatory B cells (Bregs) positively impact the bone metabolism (71, 72). Additionally, MDSCs stimulate the proliferation of Tregs that act as key helpers in prolonging osteoblast survival (73). This indicates a complicated interaction triangle among MDSCs, the bone, and components of immune system. Figure 1 summarizes an overview of the interaction among MDSCs, immune cells and skeletal system.
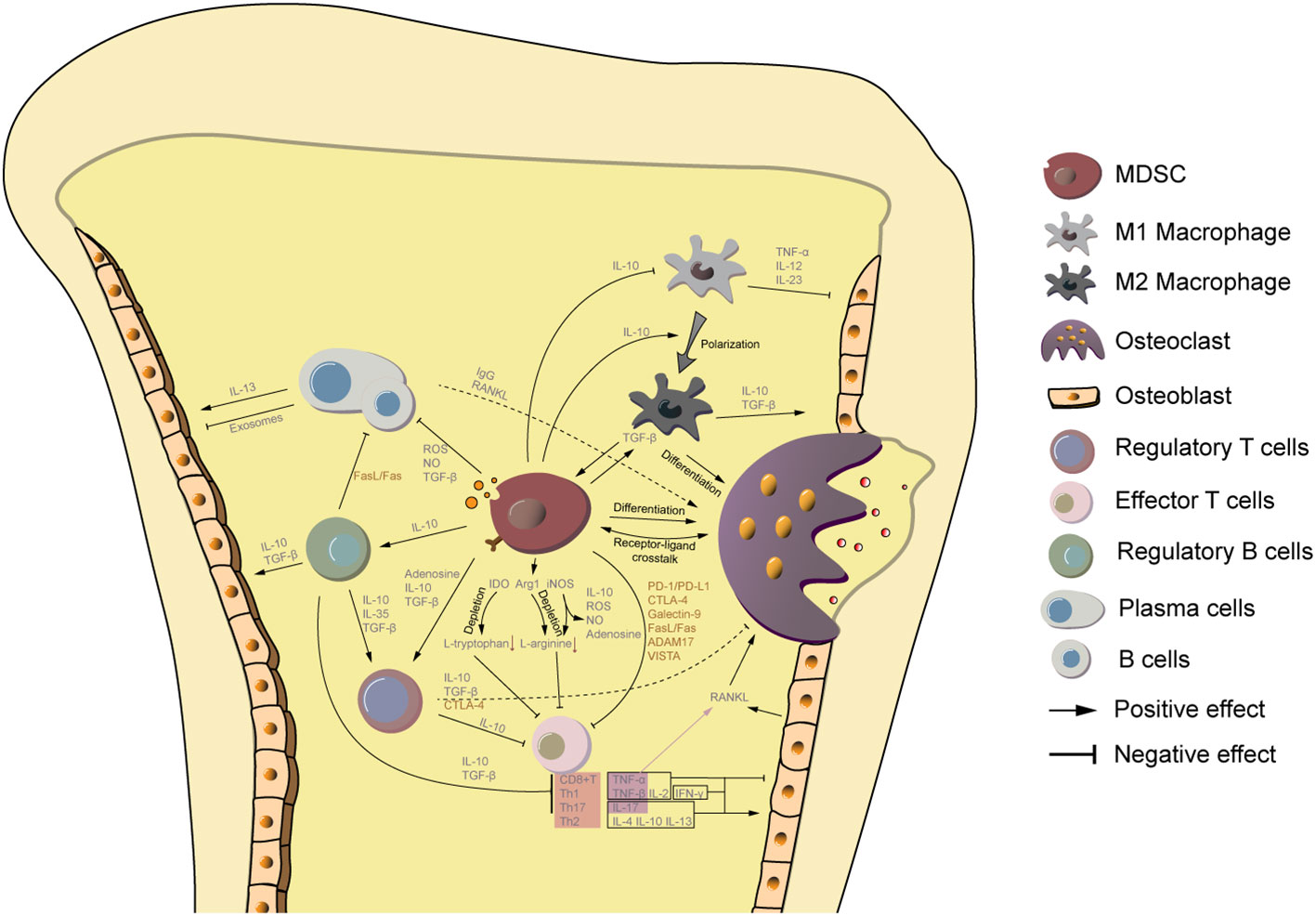
Figure 1 MDSCs are a key link between the bone metabolism and immune system. MDSCs are immature cells of the myeloid lineage that can differentiate to osteoclasts. Additionally, they secret IL-10 to promote macrophage polarization from M1 to M2, of which the latter one is also capable to differentiate to osteoclasts. MDSCs are also involved in the regulation of other counterparts of the immune system. Small molecules from MDSCs, including TGF-β, IL-10, adenosine, and ROS/NO hamper the immune reaction directly or indirectly by supporting proliferation of regulatory T cells, regulatory B cells, M2 macrophages, and inhibiting the activity of effector T cells, B cells, plasma cells, and M1 macrophages. Among them, regulatory T/B cells and M2 macrophage support osteogenic processes. Cytokines from M1 macrophage, CD8+ T cells, and Th1 cells limit osteoblast function, while Th2 and Th17 promote osteogenesis. The effect of plasma cells and B cells on osteoblast activity is controversial and depends on different biological settings. Moreover, immunosuppressive ligands and surface receptors on MDSCs interact with lymphocytes and osteoblasts to regulate their function.
5.1 Soluble factors from MDSCs
A broad range of secreted factors are related to the function of MDSCs, some of which were described immunosuppressive that can prolong the chronicity. However, they also play a versatile role in osteogenesis. TGF-β and IL-10 are two of the most important factors supporting proliferation of Tregs (51, 74), and also play a key role in the generation of osteoblasts (75). Additionally, adenosine which is generated by CD39 and CD73 on the surface of MDSCs can lead to activation of the A2A receptor subsequently increasing production of Tregs (73, 76). Adenosine also has a direct proliferative effect on BMSCs and osteoblasts by activation of the A2B receptor, and therefore contributes to bone regeneration (77, 78). Moreover, other molecules secreted by MDSCSs such as S100A8/A9 and NO have also been shown to positively impact osteoblast differentiation (79, 80).
5.2 Immunosuppressive surface markers on MDSCs
Cell-cell contact through immunosuppressive ligands and receptors plays a key in immune dysregulation. Previous studies have suggested a variety of immunoregulatory surface functional molecules to be found on MDSCs (81). These membrane proteins can directly interact with T effector cells, promote the expansion of Tregs and Bregs, and thus regulate systemic immunity in viral infections, autoimmune diseases, and cancer. There are few studies on the direct contact of MDSCs to osteoblasts, but several studies discussed how these surface markers can affect their fate. In particular, the PD-1/PD-L1 axis might have regulatory effect on bone remodeling by limiting osteoclastogenesis (82). Additionally, Galectin-9 is widely expressed in various tissues that were reported to induce osteoblast differentiation (83). CD155, an important receptor mediating cell adhesion, was reported to be expressed on osteoclast precursors and regulate differentiation processes (84). CD276 is membrane-bound but can be also released from the surface as a soluble molecule. Deficiency of CD276 results in lower osteoblastic activity and reduced mineralization (85). Research on ADAM17 demonstrated its role in stimulating osteoclastogenesis by degrading interferon (IFN)-γ (86) and inhibiting osteoblast differentiation through interaction with RUNX2 (87).
5.3 MDSC-derived exosome and immune response
Exosomes are a group of lipid bilayer vesicles with nanoscale size (usually 30-100nm), shed by various types of cells during the intercellular communication and regulation. Compared to bone marrow from healthy individuals, exosomes of MDSCs in a tumor environment are excreted in larger numbers and contain more cytokines related to tumor invasion, angiogenesis, and myeloid cell activation or function (88), as well as mRNAs, microRNAs, and other protein molecules involved in immune modulation (89–91). Through proteomic analysis, several typical surface markers on exosomes were found to be representative of their parental MDSCs and beneficial for MDSC migration (92). MDSCs secret exosomes to interfere with their neighborhood in response to changing immune circumstances. CD8+ T cells treated with these small vesicles display a trend towards anergy, while Tregs increase their regulatory activity (88). G-MDSCs were reported to attenuate immune responses of Th1 and Th17 cells and thus reduce the severity of autoimmune arthritis by releasing exosomes (93). Additionally, TGF-β and IL-10 have been found in MDSC-exosomes – two molecules involved in inhibition of autoimmunity and stimulation of osteoblastic growth (94).
6 Role of MDSCs in skeletal diseases
MDSCs are activated by inflammation to limit the immune response and to protect against tissue damage. However, in a tumor or chronic bacterial infection environment the immunosuppressive function of MDSCs contribute to disease progression and prolongation. In the skeletal system, MDSCs can not only dampen immune activity, but also cause bone erosion by differentiating to OCs. Despite their importance for bone health, knowledge on their involvement in various different skeletal diseases remains limited.
6.1 Ageing and osteoporosis
Osteoporosis is a chronic disease featuring low bone mineral density, pronounced bone loss, bone fragility, and subsequently increased risk for fracture with or without external force. Aging, female gender, genomics, lack of nutrients and other comorbidities are important pathogenic factors impairing bone health and causal to the development of osteoporosis.
Osteoporosis is characterized by gradual degradation of bone tissue with aging. Besides impaired osteoblast function and increasing number of osteoclasts, immune dysfunction has been shown to play a significant role in osteoporosis (6). The aging process of the immune system that is accompanied by progressive immune dysfunction affecting both lymphogenesis and myelogenesis is called “immunosenescence” (95). Specifically, with increasing age there is a gradual decline of T- and B- cells, increased generation of cells from the myeloid lineage, and upregulation of proinflammatory cytokines including IL-6 and TNF-α from senescent cells. The phenomenon of these inflammatory changes within an aging body is called “inflammageing” (96). The resulting chronic proinflammatory environment forms a suitable milieu for proliferation and expansion of MDSCs in bone of the elderly (97–99). Additionally, MDSCs are stimulated towards osteoclast differentiation in inflammageing. Aged individuals show increased MDSC-dependent osteoclast differentiation (99, 100). These changes are driven by increased production of reactive oxygen species (ROS) and nitric oxide (NO). ROS are a set of oxygen-containing molecules aggravating oxidative stress and aging process (101, 102), while NO is synthesized from precursor L-arginine. These molecules damage biologically active molecules, such as DNA, RNA, and enzymes relevant for repairing DNA and cell mitosis (103). In aged individuals, ROS and NO are a potential pathomechanism for enhanced osteoclastogenesis (99, 100). Studies in a murine model of osteoporosis suggest that the resulting bone loss can be alleviated by treatment against these products of oxidative stress (104, 105). Besides being inducers of osteoclastogenesis, ROS and NO function as immune modulators produced by G-MDSCs and M-MDSCs, that suppress T cell generation and function.
Proinflammatory IL-1β, IL-6, and TNF-α, as well as growth factor M-CSF are key regulators in age-related osteoporosis (96, 100). Long-term stimulation by these cytokines leads to increased osteoclastogenesis of MDSCs by upregulation of RANKL – an important regulator of expansion and survival of MDSCs. With increasing age, MDSCs gain more sensitivity to RANKL and are subsequently more stimulated and activated (100). Inhibition of RANKL significantly lowers the proportion of MDSCs vice versa (106). Additionally, chronic NF-κB pathway activation in aged individuals contributes to differentiation of MDSCs (97). The severity of bone loss in osteoporosis is closely related to the activity of the NF-κB pathway (107).
Commonly, bisphosphonates are used to treat age-related osteoporosis. These molecules can dose-dependently abrogate expansion of MDSCs and limit their osteoclastic ability by inhibition of protein prenylation (63), suggesting MDSCs play an essential role in this pathology. Given the impact of MDSCs on the bone metabolism, targeting this cell population is a potential novel therapeutic target against osteoporosis (108).
6.2 Autoimmune arthritis and bone destruction
Autoimmune diseases are a range of morbidities characterized by abnormal generation of self-reactive antibodies (4). In contrast to autoinflammatory diseases caused by the innate immune system, adaptive immune cells are responsible for the development of autoimmune diseases. However, both morbidities share inflammation as a common feature. This proinflammatory environment increases osteoclast differentiation and subsequently causes bone erosion as a discernable sign of autoimmune diseases compared to degenerative arthritis.
In autoimmune diseases, MDSCs have been pointed out to be deleterious to bone formation. Charles et al. first described a group of M-MDSC-like myeloid cells with CD11b-/lowLy6Chi phenotype with high differentiation potential and myeloid suppressor function in a rheumatoid arthritis (RA) mice model (109). Zhang et al. later identified that co-stimulation of MDSCs with M-CSF and RANKL contributes to bone erosion in a collagen induced arthritis (CIA) model (62). Similar, in another murine autoimmunity model (MFG-E8 knockout mice), bone mass was compromised by enhanced inflammation due to increased osteoclast differentiation of MDSCs (110). In humans, Chen et al. found a strong correlation of M-MDSCs and Th17 cells with osteolysis. Th17 cells can switch to a pro-osteoclastogenic phenotype with high expression of RANKL and reciprocally induce M-MDSCs differentiating into OCs (111). Of note, M-MDSCs were found to secret Arg-1 instead of NO to regulate RANKL expression on Th17 cells (111), which contrasts previous findings that M-MDSCs usually secret NO to modulate the immune responses (13).
Besides their impact on the bone, MDSCs can actively regulate the activity of autoimmune diseases by interacting with T and B effector cells. The immunosuppressive ability of MDSCs has been described in various diseases prone to arthritic lesions, including RA, systemic lupus erythematosus (SLE), and ankylosing spondylitis and the adoptive transfer of allogenic MDSCs has been shown to be a novel treatment approach in affected patients (93, 112). In an autoimmune arthritis model, adoptive transfer of MDSCs skewed the T cell population toward Treg generation, reduced the Th1 and Th17 cell population, and decreased the expression of inflammatory cytokines (113). Similar, transfusion of PD-L1 expressing MDSCs resulted in expansion of regulatory T and B cells and subsequent down-regulation of overactive autoimmunity in a murine SLE model (114).
In contrast to these findings, MDSCs have been reported to prolong or even exaggerate inflammation and thus enhance disease activity. In several reports on the adoptive MDSC transfer in SLE, MDSCs increased disease severity by secreting Arg-1 stimulating Th17 cell differentiation (14, 74). Similar, some reports found higher expression of TNF-α and IL-1β and subsequently increased diseases progression in autoimmune arthritis after MDSC transfer (115, 116). This effect may be caused by selecting MDSCs using Gr-1 and CD11b which can also be found on potentially proinflammatory mature myeloid cells. Another potential mechanism responsible for increased inflammation may be MDSCs potential to differentiate to macrophages or neutrophils depending on the local complex inflammatory environment (117). In addition to an adverse immune response, MDSCs are potential osteoclast precursors when transferred into an autoimmune condition and may deteriorate affected bony structures further.
6.3 Orthopedic implant-related infection
Despite increased use of antibiotics and improved aseptic surgical techniques, orthopedic implant-associated infections still remain one of the most challenging complications in orthopedics for patients, physicians, and the health care system alike (118, 119). Chronic inflammation at the bone-implant interface can impact healing and subsequently lead to septic loosening. Once osteolysis sets on, the bone quality decreases over time and the risk for fracture or implant failure significantly increases (118).
In chronic implant-related infection, low virulent bacteria form a layer of biofilm to protect themselves against the immune system and antibiotics (120). Inside the biofilm, bacteria form communities with a reduced metabolic rate, described as a “dormant state” (121, 122). This biofilm gradually elicits the immunosuppressive function of local reactive leukocytes, and therefore prolongs bacteria survival, further complicating successful treatment (16). Additionally, the proinflammatory environment attracts MDSCs to accumulate in the bone niche and attenuate the antibacterial function of polymorphonuclear cells (123).
MDSCs were recently revealed to be involved in the pathogenesis of periprosthetic joint infections. Besides elevated local cell prevalence, their presence in the peripheral blood persists over a long period of time, suggesting a systemic process potentially affecting other organs. However, despite their assumed role in disease progression, knowledge on the impact of MDSCs in implant-associated infections remains severely limited. Their immunosuppressive function has been shown to prolong infection by inhibiting the immune responses mediated by T cells, B cells, and natural killer cells (16, 124, 125). Compared to other myeloid derived cells or lymphocytes, prevalence of MDSCs was particularly high and increased over time in chronic infections (124, 126). Additionally, there has been large numbers of MDSCs observed infiltrating the biofilm, accounting for nearly half of the detectable MDSC population (16). G-MDSCs have been shown to be particularly relevant for heightened bacterial resistance (11). They produce IL-10 leading to increased bacterial persistence (11, 127) and susceptibility to infections (128). After antibody depletion of the G-MDSC population by targeting Ly6G, Ly6C+ monocytes and macrophages expand and regain proinflammatory function essential for clearing bacterial infection (124). Besides G-MDSC, M-MDSC are found around the biofilm albeit in much smaller numbers (16). At the biofilm, M-MDSCs differentiate to anti-inflammatory M2 macrophages that hinder T-cell mediated immunity and thus also contribute to infection persistence (129). Employment of anti-bacterial additions to implants can significantly reduce the number of MDSCs, limit their anti-inflammatory function, and increase efficiency of antibiotics (130, 131). Additionally, successful treatment can positively impact the bone metabolism, as MDSCs differentiate to OCs in infection (132). After surgical addressing of the biofilm, the septic bone destruction recovers significantly (131).
The relationship of the pathogenesis of orthopedic infection and MDSCs is reciprocal. Increased prevalence of MDSCs is linked to heightened risk of infection. Of note, in one in vivo human study, the number of G-MDSCs was elevated after aseptic orthopedic surgeries while relative occurrence of total leukocytes and MDSCs remained the same (128). These results suggest during and immediately after surgery risk for bacterial infection may be highest and targeting MDSCs may be a viable prophylactic treatment.
The PD-1/PD-L1 signaling axis has been suggested as a potential target. MDSCs down-regulate T-cell induced pathogen elimination through PD-1/PD-L1 signaling (133, 134). Additionally, in vivo experiments suggest a crucial role of PD-1 in differentiation of MDSCs to OCs. PD-1 knockout in osteoporotic mice halved the number of OCs and led to a 2-fold increase in bone volume (82). Inhibition of PD-1 using immune checkpoint inhibitors interrupts OC precursor cell differentiation in areas with bone lesions involving downregulation of CC-chemokine ligand 2/CC-chemokine receptor 2 (CCL2/CCR2) pathway, whereas it exerts no effect on physiological bone structures (135). Conversely, targeting the PD-1/PD-L1 axis may improve clinical outcome, yet can also aggravate inflammation and disrupt the bone metabolism (136). Similar, bisphosphonate can dampen the osteolytic effects of OCs and inhibit MDSC differentiation, however, they have been associated with higher bacterial burden and increased risk for infection (53, 137). Promising novel strategies such as using bisphosphonate as carrier for antibiotics still have to prove effective in a clinical setting (138).
6.4 Bone fracture
A traumatic fracture is described as partially or completely disrupted continuity of the bone potentially leading to persisting pain, immobility, and even death due to blood loss (139). However, the bone tissue possesses the potential to fully recover from if treated appropriately. Despite adequate conservative or surgical treatment around 5-10% of affected patients develop mal- or non-union fractures and need additional intervention (140).
Fracture union encompasses consecutive and overlapping phases, from formation of hematoma, soft callus, fibrous tissue to hard callus, and finally remodeled bone (9). The metabolic phases during bone healing interact with the innate and adaptive immune system. The processes involved promote angiogenesis and osteoblast differentiation from BMSCs (9). Dysregulation of the immune response can retard the fracture healing process and is a significant risk factor for mal- or non-union fracture healing. Thus, restoring the physiological immune environment in general and targeting MDSCs in particular is a promising novel therapeutic approach in affected individuals (17, 31).
Currently, there exist conflicting evidence on the role of MDSCs in the bone healing process. Traumatic injury leads to increased cytokine production of IL-1β, IL-6, and G-CSF prompting accumulation of MDSCs (141). Cheng et al. described a long-term dysregulated immune pattern in delayed bone healing (142). By computational analysis, they found a negative correlation of circulating MDSCs and bone healing. MDSCs indirectly suppress the regenerative capability of BMSCs by inhibition of B cell differentiation and elevated IL-10 expression (72). Conversely, MDSCs show a protective effect on injured bone tissue and can even support tissue remodeling (143, 144). After arthroplasty, there is a high concentration of MDSCs that support development of new blood vessel at the polymethyl methacrylate induced periosteal membrane. Local transplantation of MDSCs enhances the formation of these capillaries around the membrane (145). In traumatic fracture healing, significantly elevated number of MDSCs were observed in the transitional area, facilitating the recovery of the bone injury by suppressing local inflammation to stimulate osteoblast differentiation and function (146). However, while MDSCs promote bone regeneration by improving angiogenesis and limiting the inflammatory response, continuous presence of MDSCs pose a risk for infection due to their immunosuppressive capabilities (142).
6.5 Bone malignancy and metastasis
Cancer growth depends on both the vigorousness of the tumor itself and a compromised anti-tumor ability of the immune system. MDSCs can facilitate tumor growth through their immunosuppressive capabilities. Research on MDSCs and their involvement in tumor progression has been a main focus and inspires hope for novel therapeutic approaches.
Osteosarcoma (OS) is one of the most prevalent primary bone malignancies in children and teenagers. Both surgical intervention and chemotherapy are employed to enhance quality of life and overall survival. A better understanding of the role MDSCs in supporting growth of OS may open up new treatment options. In the tumor microenvironment, MDSCs, most of them PMN-MDSCs, accumulate and inhibit the T-cell mediated immune responses induced by high expression of IL-18 and CXCL12 (147, 148). Blocking these inducive factors has been shown to sharpen the anti-PD-1 treatment efficacy in mice indicating the importance of the PD-1/PD-L1 axis in MDSCs during the growth of OS (147–149). Activation of the PI3K/Akt pathway was also found to be pivotal in OS tumor growth (148, 149). Additionally, the STAT3 pathway has been related to immunosuppression in this tumor pathology. Inhibition of STAT3 and PI3K/Akt signaling can reverse the suppressive effects on local immunity and reduce tumor size (148–150).
Besides primary bone tumors, the skeletal system is much more commonly affected by metastasis of several types of cancer. In cases of bone metastasis, a variety of growth factors and chemokines produced by the bone and immune regulating cells facilitate the proliferation and expansion of MDSCs (151, 152). At tumor site, malignant cells can precondition the immunosuppressive behavior of BMSCs. These cells subsequently promote the expansion of MDSCs and can attract cancer cells to migrate from the blood into the bone (153). Additionally, MDSCs contribute to epithelial-mesenchymal transition (EMT), thus enhancing mobility, invasion, and resistance to apoptotic stimuli of cancer cells. CXCR2+PMN-MDSCs were found to be a major regulator and initiator of EMT through releasing IL-6 during breast cancer progression (154). M-MDSCs can also modulate EMT by secretion of nitric oxide synthase modulate (155). Moreover, MDSCs are involved in the formation of the pre-metastatic niche (PMN). They aggregate at the PMN where they support the construction of the nutritious “soil” for tumor metastases to “plant in” by promoting neovascularization (12, 156) and increasing the activity of neutrophil extracellular traps that can catch circulating tumor cells to colonize (157, 158). Lastly, MDSCs enhance direct differentiation to M2 macrophages (159, 160) and facilitate the differentiation of M1 to tumor-supportive M2 macrophages (161).
The cancer-driven accumulation of MDSCs also has impact on the bone metabolism by differentiating to OCs. This hinders bone regeneration both at the site of osteolytic bone metastases and by dissemination to the bone site via blood stream (61). Of note, osteoclast differentiation is MDSC-dependent in bone metastasis, signifying the essential crosstalk between tumor cells and myeloid progenitors in the bone microenvironment (162). Once tumor cells spread to the bone and meet the primed MDSCs they start a continuous stimulate each other reciprocally challenging the bone health. In multiple myeloma, the impact on the bone is even more severe as this malignancy originates from the bone marrow (63). Additionally, the generated OCs enhance tumor immune evasion of multiple myeloma cells from T cell surveillance via PD-L1, galectin-9, and CD200 (163, 164). Treatment with immune checkpoint blockers targets this mechanism to revert the MDSC-driven anti-tumor immunosuppression (147).
7 Conclusion
The delicate balance of bone resorption and regeneration interacts with and is influenced by the regulatory immune system both physiologically and in disease. In this review, we discuss the impact of MDSCs on the bone metabolism under several pathological conditions, the involved modulatory pathways as well as potential therapeutic targets in MDSCs to improve bone health. MDSCs have a regulatory function on the immune system and can significantly and lastingly impact the process of bone remodeling through differentiation into osteoclasts. In chronic inflammatory conditions, generation of MDSCs is induced. MDSCs have previously been identified in several diseases affecting the bone including tumor, autoimmune diseases, fractures, and infection. They are part of a complex network in which they interact with and regulate other immune cells by releasing soluble proteins, exosomes, and through surface protein-receptor interactions. However, there remains paucity on several of the involved pathways linking MDSCs to osteoclast differentiation and function as well as osteoblast activity and behavior. Emerging evidence suggests a key role of MDSCs in these diseases making them a promising target for novel therapeutic approaches in several diseases.
Author contributions
Conceptualization, YR. Project administration, MM. Resources, MM. Supervision, AK and MM. Visualization, YR. Writing – original draft, YR, HB, and AK. Writing – review & editing, YR, HB, AK, and MM. All authors contributed to the article and approved the submitted version.
Acknowledgments
Dr. AK is participant in the BIH-Charité Junior Clinician Scientist Program funded by the Charité — Universitätsmedizin Berlin and the Berlin Institute of Health.
Conflict of interest
The authors declare that the research was conducted in the absence of any commercial or financial relationships that could be construed as a potential conflict of interest.
Publisher’s note
All claims expressed in this article are solely those of the authors and do not necessarily represent those of their affiliated organizations, or those of the publisher, the editors and the reviewers. Any product that may be evaluated in this article, or claim that may be made by its manufacturer, is not guaranteed or endorsed by the publisher.
References
1. Feng X, McDonald JM. Disorders of bone remodeling. Annu Rev Pathol (2011) 6:121–45. doi: 10.1146/annurev-pathol-011110-130203
2. Kim JM, Lin C, Stavre Z, Greenblatt MB, Shim JH. Osteoblast-osteoclast communication and bone homeostasis. Cells (2020) 9(9):2073. doi: 10.3390/cells9092073
3. Li H, Hong S, Qian J, Zheng Y, Yang J, Yi Q. Cross talk between the bone and immune systems: Osteoclasts function as antigen-presenting cells and activate CD4+ and CD8+ t cells. Blood (2010) 116(2):210–7. doi: 10.1182/blood-2009-11-255026
4. Okamoto K, Nakashima T, Shinohara M, Negishi-Koga T, Komatsu N, Terashima A, et al. Osteoimmunology: The conceptual framework unifying the immune and skeletal systems. Physiol Rev (2017) 97(4):1295–349. doi: 10.1152/physrev.00036.2016
5. El Khassawna T, Serra A, Bucher CH, Petersen A, Schlundt C, Könnecke I, et al. T lymphocytes influence the mineralization process of bone. Front Immunol (2017) 8:562. doi: 10.3389/fimmu.2017.00562
6. Pietschmann P, Mechtcheriakova D, Meshcheryakova A, Föger-Samwald U, Ellinger I. Immunology of osteoporosis: A mini-review. Gerontology (2016) 62(2):128–37. doi: 10.1159/000431091
7. Mbalaviele G, Novack DV, Schett G, Teitelbaum SL. Inflammatory osteolysis: A conspiracy against bone. J Clin Invest (2017) 127(6):2030–9. doi: 10.1172/jci93356
8. Ono T, Nakashima T. Recent advances in osteoclast biology. Histochem Cell Biol (2018) 149(4):325–41. doi: 10.1007/s00418-018-1636-2
9. Einhorn TA, Gerstenfeld LC. Fracture healing: Mechanisms and interventions. Nat Rev Rheumatol (2015) 11(1):45–54. doi: 10.1038/nrrheum.2014.164
10. Delaisse JM. The reversal phase of the bone-remodeling cycle: Cellular prerequisites for coupling resorption and formation. Bonekey Rep (2014) 3:561. doi: 10.1038/bonekey.2014.56
11. Heim CE, Vidlak D, Odvody J, Hartman CW, Garvin KL, Kielian T. Human prosthetic joint infections are associated with myeloid-derived suppressor cells (MDSCs): Implications for infection persistence. J Orthop Res (2018) 36(6):1605–13. doi: 10.1002/jor.23806
12. Vetsika EK, Koukos A, Kotsakis A. Myeloid-derived suppressor cells: Major figures that shape the immunosuppressive and angiogenic network in cancer. Cells (2019) 8(12):1647. doi: 10.3390/cells8121647
13. Veglia F, Perego M, Gabrilovich D. Myeloid-derived suppressor cells coming of age. Nat Immunol (2018) 19(2):108–19. doi: 10.1038/s41590-017-0022-x
14. Wu H, Zhen Y, Ma Z, Li H, Yu J, Xu ZG, et al. Arginase-1-dependent promotion of TH17 differentiation and disease progression by MDSCs in systemic lupus erythematosus. Sci Transl Med (2016) 8(331):331ra40. doi: 10.1126/scitranslmed.aae0482
15. Venet F, Monneret G. Advances in the understanding and treatment of sepsis-induced immunosuppression. Nat Rev Nephrol (2018) 14(2):121–37. doi: 10.1038/nrneph.2017.165
16. Heim CE, West SC, Ali H, Kielian T. Heterogeneity of Ly6G(+) Ly6C(+) myeloid-derived suppressor cell infiltrates during staphylococcus aureus biofilm infection. Infect Immun (2018) 86(12):e00684-18. doi: 10.1128/iai.00684-18
17. Schlundt C, Reinke S, Geissler S, Bucher CH, Giannini C, Märdian S, et al. Individual Effector/Regulator t cell ratios impact bone regeneration. Front Immunol (2019) 10:1954. doi: 10.3389/fimmu.2019.01954
18. Arron JR, Choi Y. Bone versus immune system. Nature (2000) 408(6812):535–6. doi: 10.1038/35046196
19. Greenbaum A, Hsu YM, Day RB, Schuettpelz LG, Christopher MJ, Borgerding JN, et al. CXCL12 in early mesenchymal progenitors is required for haematopoietic stem-cell maintenance. Nature (2013) 495(7440):227–30. doi: 10.1038/nature11926
20. Terashima A, Okamoto K, Nakashima T, Akira S, Ikuta K, Takayanagi H. Sepsis-induced osteoblast ablation causes immunodeficiency. Immunity (2016) 44(6):1434–43. doi: 10.1016/j.immuni.2016.05.012
21. Visnjic D, Kalajzic Z, Rowe DW, Katavic V, Lorenzo J, Aguila HL. Hematopoiesis is severely altered in mice with an induced osteoblast deficiency. Blood (2004) 103(9):3258–64. doi: 10.1182/blood-2003-11-4011
22. Sato M, Asada N, Kawano Y, Wakahashi K, Minagawa K, Kawano H, et al. Osteocytes regulate primary lymphoid organs and fat metabolism. Cell Metab (2013) 18(5):749–58. doi: 10.1016/j.cmet.2013.09.014
23. Yee CS, Manilay JO, Chang JC, Hum NR, Murugesh DK, Bajwa J, et al. Conditional deletion of sost in MSC-derived lineages identifies specific cell-type contributions to bone mass and b-cell development. J Bone Miner Res (2018) 33(10):1748–59. doi: 10.1002/jbmr.3467
24. Lymperi S, Ersek A, Ferraro F, Dazzi F, Horwood NJ. Inhibition of osteoclast function reduces hematopoietic stem cell numbers in vivo. Blood (2011) 117(5):1540–9. doi: 10.1182/blood-2010-05-282855
25. Mansour A, Abou-Ezzi G, Sitnicka E, Jacobsen SE, Wakkach A, Blin-Wakkach C. Osteoclasts promote the formation of hematopoietic stem cell niches in the bone marrow. J Exp Med (2012) 209(3):537–49. doi: 10.1084/jem.20110994
26. Tsukasaki M, Takayanagi H. Osteoimmunology: Evolving concepts in bone-immune interactions in health and disease. Nat Rev Immunol (2019) 19(10):626–42. doi: 10.1038/s41577-019-0178-8
27. Okamoto K, Takayanagi H. Effect of t cells on bone. Bone (2023) 168:116675. doi: 10.1016/j.bone.2023.116675
28. Kolomansky A, Kaye I, Ben-Califa N, Gorodov A, Awida Z, Sadovnic O, et al. Anti-CD20-Mediated b cell depletion is associated with bone preservation in lymphoma patients and bone mass increase in mice. Front Immunol (2020) 11:561294. doi: 10.3389/fimmu.2020.561294
29. Horowitz MC, Fretz JA, Lorenzo JA. How b cells influence bone biology in health and disease. Bone (2010) 47(3):472–9. doi: 10.1016/j.bone.2010.06.011
30. Schlundt C, El Khassawna T, Serra A, Dienelt A, Wendler S, Schell H, et al. Macrophages in bone fracture healing: Their essential role in endochondral ossification. Bone (2018) 106:78–89. doi: 10.1016/j.bone.2015.10.019
31. Lee J, Byun H, Madhurakkat Perikamana SK, Lee S, Shin H. Current advances in immunomodulatory biomaterials for bone regeneration. Adv Healthc Mater (2019) 8(4):e1801106. doi: 10.1002/adhm.201801106
32. Young MR, Aquino S, Young ME. Differential induction of hematopoiesis and immune suppressor cells in the bone marrow versus in the spleen by lewis lung carcinoma variants. J Leukoc Biol (1989) 45(3):262–73. doi: 10.1002/jlb.45.3.262
33. Gabrilovich DI, Bronte V, Chen SH, Colombo MP, Ochoa A, Ostrand-Rosenberg S, et al. The terminology issue for myeloid-derived suppressor cells. Cancer Res (2007) 67(1):425. doi: 10.1158/0008-5472.Can-06-3037
34. Bronte V, Brandau S, Chen SH, Colombo MP, Frey AB, Greten TF, et al. Recommendations for myeloid-derived suppressor cell nomenclature and characterization standards. Nat Commun (2016) 7:12150. doi: 10.1038/ncomms12150
35. Hegde S, Leader AM, Merad M. MDSC: Markers, development, states, and unaddressed complexity. Immunity (2021) 54(5):875–84. doi: 10.1016/j.immuni.2021.04.004
36. Alshetaiwi H, Pervolarakis N, McIntyre LL, Ma D, Nguyen Q, Rath JA, et al. Defining the emergence of myeloid-derived suppressor cells in breast cancer using single-cell transcriptomics. Sci Immunol (2020) 5(44):eaay6017. doi: 10.1126/sciimmunol.aay6017
37. Yaseen MM, Abuharfeil NM, Darmani H, Daoud A. Recent advances in myeloid-derived suppressor cell biology. Front Med (2021) 15(2):232–51. doi: 10.1007/s11684-020-0797-2
38. Condamine T, Mastio J, Gabrilovich DI. Transcriptional regulation of myeloid-derived suppressor cells. J Leukoc Biol (2015) 98(6):913–22. doi: 10.1189/jlb.4RI0515-204R
39. Butterfield LH, Zhao F, Lee S, Tarhini AA, Margolin KA, White RL, et al. Immune correlates of GM-CSF and melanoma peptide vaccination in a randomized trial for the adjuvant therapy of resected high-risk melanoma (E4697). Clin Cancer Res (2017) 23(17):5034–43. doi: 10.1158/1078-0432.Ccr-16-3016
40. Sionov RV, Fridlender ZG, Granot Z. The multifaceted roles neutrophils play in the tumor microenvironment. Cancer Microenviron (2015) 8(3):125–58. doi: 10.1007/s12307-014-0147-5
41. Yang F, Li Y, Wu T, Na N, Zhao Y, Li W, et al. TNFα-induced m-MDSCs promote transplant immune tolerance via nitric oxide. J Mol Med (Berl) (2016) 94(8):911–20. doi: 10.1007/s00109-016-1398-z
42. Horikawa N, Abiko K, Matsumura N, Hamanishi J, Baba T, Yamaguchi K, et al. Expression of vascular endothelial growth factor in ovarian cancer inhibits tumor immunity through the accumulation of myeloid-derived suppressor cells. Clin Cancer Res (2017) 23(2):587–99. doi: 10.1158/1078-0432.Ccr-16-0387
43. Zhang Y, Wilt E, Lu X. Human isogenic cell line models for neutrophils and myeloid-derived suppressor cells. Int J Mol Sci (2020) 21(20):7709. doi: 10.3390/ijms21207709
44. Jing B, Wang T, Sun B, Xu J, Xu D, Liao Y, et al. IL6/STAT3 signaling orchestrates premetastatic niche formation and immunosuppressive traits in lung. Cancer Res (2020) 80(4):784–97. doi: 10.1158/0008-5472.Can-19-2013
45. Marigo I, Bosio E, Solito S, Mesa C, Fernandez A, Dolcetti L, et al. Tumor-induced tolerance and immune suppression depend on the C/EBPbeta transcription factor. Immunity (2010) 32(6):790–802. doi: 10.1016/j.immuni.2010.05.010
46. McClure C, McPeak MB, Youssef D, Yao ZQ, McCall CE, El Gazzar M. Stat3 and C/EBPβ synergize to induce miR-21 and miR-181b expression during sepsis. Immunol Cell Biol (2017) 95(1):42–55. doi: 10.1038/icb.2016.63
47. Mazza EM, Zoso A, Mandruzzato S, Bronte V, Serafini P, Inverardi L, et al. Gene expression profiling of human fibrocytic myeloid-derived suppressor cells (f-MDSCs). Genom Data (2014) 2:389–92. doi: 10.1016/j.gdata.2014.10.018
48. Khan ANH, Emmons TR, Wong JT, Alqassim E, Singel KL, Mark J, et al. Quantification of early-stage myeloid-derived suppressor cells in cancer requires excluding basophils. Cancer Immunol Res (2020) 8(6):819–28. doi: 10.1158/2326-6066.Cir-19-0556
49. Solito S, Falisi E, Diaz-Montero CM, Doni A, Pinton L, Rosato A, et al. A human promyelocytic-like population is responsible for the immune suppression mediated by myeloid-derived suppressor cells. Blood (2011) 118(8):2254–65. doi: 10.1182/blood-2010-12-325753
50. Lang S, Bruderek K, Kaspar C, Höing B, Kanaan O, Dominas N, et al. Clinical relevance and suppressive capacity of human myeloid-derived suppressor cell subsets. Clin Cancer Res (2018) 24(19):4834–44. doi: 10.1158/1078-0432.Ccr-17-3726
51. Huang B, Pan PY, Li Q, Sato AI, Levy DE, Bromberg J, et al. Gr-1+CD115+ immature myeloid suppressor cells mediate the development of tumor-induced t regulatory cells and t-cell anergy in tumor-bearing host. Cancer Res (2006) 66(2):1123–31. doi: 10.1158/0008-5472.Can-05-1299
52. Dolcetti L, Peranzoni E, Ugel S, Marigo I, Fernandez Gomez A, Mesa C, et al. Hierarchy of immunosuppressive strength among myeloid-derived suppressor cell subsets is determined by GM-CSF. Eur J Immunol (2010) 40(1):22–35. doi: 10.1002/eji.200939903
53. Li D, Gromov K, Proulx ST, Xie C, Li J, Crane DP, et al. Effects of antiresorptive agents on osteomyelitis: Novel insights into the pathogenesis of osteonecrosis of the jaw. Ann N Y Acad Sci (2010) 1192(1):84–94. doi: 10.1111/j.1749-6632.2009.05210.x
54. Diamond P, Labrinidis A, Martin SK, Farrugia AN, Gronthos S, To LB, et al. Targeted disruption of the CXCL12/CXCR4 axis inhibits osteolysis in a murine model of myeloma-associated bone loss. J Bone Miner Res (2009) 24(7):1150–61. doi: 10.1359/jbmr.090210
55. Xing L, Ebetino FH, Boeckman RK Jr., Srinivasan V, Tao J, Sawyer TK, et al. Targeting anti-cancer agents to bone using bisphosphonates. Bone (2020) 138:115492. doi: 10.1016/j.bone.2020.115492
56. Nakamura I, Jimi E. Regulation of osteoclast differentiation and function by interleukin-1. Vitam Horm (2006) 74:357–70. doi: 10.1016/s0083-6729(06)74015-8
57. Kobayashi K, Takahashi N, Jimi E, Udagawa N, Takami M, Kotake S, et al. Tumor necrosis factor alpha stimulates osteoclast differentiation by a mechanism independent of the ODF/RANKL-RANK interaction. J Exp Med (2000) 191(2):275–86. doi: 10.1084/jem.191.2.275
58. Kwan Tat S, Padrines M, Théoleyre S, Heymann D, Fortun Y. IL-6, RANKL, TNF-alpha/IL-1: Interrelations in bone resorption pathophysiology. Cytokine Growth Factor Rev (2004) 15(1):49–60. doi: 10.1016/j.cytogfr.2003.10.005
59. Amarasekara DS, Kim S, Rho J. Regulation of osteoblast differentiation by cytokine networks. Int J Mol Sci (2021) 22(6):2851. doi: 10.3390/ijms22062851
60. Kitaura H, Marahleh A, Ohori F, Noguchi T, Shen WR, Qi J, et al. Osteocyte-related cytokines regulate osteoclast formation and bone resorption. Int J Mol Sci (2020) 21(14):5169. doi: 10.3390/ijms21145169
61. Danilin S, Merkel AR, Johnson JR, Johnson RW, Edwards JR, Sterling JA. Myeloid-derived suppressor cells expand during breast cancer progression and promote tumor-induced bone destruction. Oncoimmunology (2012) 1(9):1484–94. doi: 10.4161/onci.21990
62. Zhang H, Huang Y, Wang S, Fu R, Guo C, Wang H, et al. Myeloid-derived suppressor cells contribute to bone erosion in collagen-induced arthritis by differentiating to osteoclasts. J Autoimmun (2015) 65:82–9. doi: 10.1016/j.jaut.2015.08.010
63. Zhuang J, Zhang J, Lwin ST, Edwards JR, Edwards CM, Mundy GR, et al. Osteoclasts in multiple myeloma are derived from gr-1+CD11b+myeloid-derived suppressor cells. PloS One (2012) 7(11):e48871. doi: 10.1371/journal.pone.0048871
64. Kwack KH, Zhang L, Sohn J, Maglaras V, Thiyagarajan R, Kirkwood KL. Novel preosteoclast populations in obesity-associated periodontal disease. J Dent Res (2022) 101(3):348–56. doi: 10.1177/00220345211040729
65. Zhang L, Kirkwood CL, Sohn J, Lau A, Bayers-Thering M, Bali SK, et al. Expansion of myeloid-derived suppressor cells contributes to metabolic osteoarthritis through subchondral bone remodeling. Arthritis Res Ther (2021) 23(1):287. doi: 10.1186/s13075-021-02663-z
66. Lian M, Wang Q, Jiang X, Zhang J, Wei Y, Li Y, et al. The immunobiology of receptor activator for nuclear factor kappa b ligand and myeloid-derived suppressor cell activation in immunoglobulin G4-related sclerosing cholangitis. Hepatology (2018) 68(5):1922–36. doi: 10.1002/hep.30095
67. Asagiri M, Takayanagi H. The molecular understanding of osteoclast differentiation. Bone (2007) 40(2):251–64. doi: 10.1016/j.bone.2006.09.023
68. Ibáñez L, Abou-Ezzi G, Ciucci T, Amiot V, Belaïd N, Obino D, et al. Inflammatory osteoclasts prime TNFα-producing CD4(+) t cells and express CX(3) CR1. J Bone Miner Res (2016) 31(10):1899–908. doi: 10.1002/jbmr.2868
69. Zhao SJ, Kong FQ, Jie J, Li Q, Liu H, Xu AD, et al. Macrophage MSR1 promotes BMSC osteogenic differentiation and M2-like polarization by activating PI3K/AKT/GSK3β/β-catenin pathway. Theranostics (2020) 10(1):17–35. doi: 10.7150/thno.36930
70. Chen X, Wang M, Chen F, Wang J, Li X, Liang J, et al. Correlations between macrophage polarization and osteoinduction of porous calcium phosphate ceramics. Acta Biomater (2020) 103:318–32. doi: 10.1016/j.actbio.2019.12.019
71. Sun G, Wang Y, Ti Y, Wang J, Zhao J, Qian H. Regulatory b cell is critical in bone union process through suppressing proinflammatory cytokines and stimulating Foxp3 in treg cells. Clin Exp Pharmacol Physiol (2017) 44(4):455–62. doi: 10.1111/1440-1681.12719
72. Mabilleau G, Delneste Y, Papon N. Predicting Bone Regeneration with a Simple Blood Test. Trends Mol Med (2021) 27(7):622–3. doi: 10.1016/j.molmed.2021.03.006
73. Zheng X, Wang D. The adenosine A2A receptor agonist accelerates bone healing and adjusts Treg/Th17 cell balance through interleukin 6. BioMed Res Int (2020) 2020:2603873. doi: 10.1155/2020/2603873
74. Pang B, Zhen Y, Hu C, Ma Z, Lin S, Yi H. Myeloid-derived suppressor cells shift Th17/Treg ratio and promote systemic lupus erythematosus progression through arginase-1/miR-322-5p/TGF-β pathway. Clin Sci (Lond) (2020) 134(16):2209–22. doi: 10.1042/cs20200799
75. Jann J, Gascon S, Roux S, Faucheux N. Influence of the TGF-β superfamily on Osteoclasts/Osteoblasts balance in physiological and pathological bone conditions. Int J Mol Sci (2020) 21(20):7597. doi: 10.3390/ijms21207597
76. Leone RD, Sun IM, Oh MH, Sun IH, Wen J, Englert J, et al. Inhibition of the adenosine A2a receptor modulates expression of t cell coinhibitory receptors and improves effector function for enhanced checkpoint blockade and ACT in murine cancer models. Cancer Immunol Immunother (2018) 67(8):1271–84. doi: 10.1007/s00262-018-2186-0
77. Stovall KE, Tran TDN, Suantawee T, Yao S, Gimble JM, Adisakwattana S, et al. Adenosine triphosphate enhances osteoblast differentiation of rat dental pulp stem cells via the PLC-IP(3) pathway and intracellular ca (2+) signaling. J Cell Physiol (2020) 235(2):1723–32. doi: 10.1002/jcp.29091
78. Mediero A, Cronstein BN. Adenosine and bone metabolism. Trends Endocrinol Metab (2013) 24(6):290–300. doi: 10.1016/j.tem.2013.02.001
79. Luo G, Tang M, Zhao Q, Lu L, Xie Y, Li Y, et al. Bone marrow adipocytes enhance osteolytic bone destruction by activating 1q21.3(S100A7/8/9-IL6R)-TLR4 pathway in lung cancer. J Cancer Res Clin Oncol (2020) 146(9):2241–53. doi: 10.1007/s00432-020-03277-9
80. Jin Z, Kho J, Dawson B, Jiang MM, Chen Y, Ali S, et al. Nitric oxide modulates bone anabolism through regulation of osteoblast glycolysis and differentiation. J Clin Invest (2021) 131(5):e138935. doi: 10.1172/jci138935
81. Haist M, Stege H, Grabbe S, Bros M. The functional crosstalk between myeloid-derived suppressor cells and regulatory t cells within the immunosuppressive tumor microenvironment. Cancers (Basel) (2021) 13(2):210. doi: 10.3390/cancers13020210
82. Nagahama K, Aoki K, Nonaka K, Saito H, Takahashi M, Varghese BJ, et al. The deficiency of immunoregulatory receptor PD-1 causes mild osteopetrosis. Bone (2004) 35(5):1059–68. doi: 10.1016/j.bone.2004.06.018
83. Tanikawa R, Tanikawa T, Okada Y, Nakano K, Hirashima M, Yamauchi A, et al. Interaction of galectin-9 with lipid rafts induces osteoblast proliferation through the c-Src/ERK signaling pathway. J Bone Miner Res (2008) 23(2):278–86. doi: 10.1359/jbmr.071008
84. Kakehi S, Nakahama K, Morita I. Expression and possible role of PVR/CD155/Necl-5 in osteoclastogenesis. Mol Cell Biochem (2007) 301(1-2):209–17. doi: 10.1007/s11010-007-9413-x
85. Feng P, Zhang H, Zhang Z, Dai X, Mao T, Fan Y, et al. The interaction of MMP-2/B7-H3 in human osteoporosis. Clin Immunol (2016) 162:118–24. doi: 10.1016/j.clim.2015.11.009
86. Kanzaki H, Shinohara F, Suzuki M, Wada S, Miyamoto Y, Yamaguchi Y, et al. A-disintegrin and metalloproteinase (ADAM) 17 enzymatically degrades interferon-gamma. Sci Rep (2016) 6:32259. doi: 10.1038/srep32259
87. Araya HF, Sepulveda H, Lizama CO, Vega OA, Jerez S, Briceño PF, et al. Expression of the ectodomain-releasing protease ADAM17 is directly regulated by the osteosarcoma and bone-related transcription factor RUNX2. J Cell Biochem (2018) 119(10):8204–19. doi: 10.1002/jcb.26832
88. Rashid MH, Borin TF, Ara R, Piranlioglu R, Achyut BR, Korkaya H, et al. Critical immunosuppressive effect of MDSC derived exosomes in the tumor microenvironment. Oncol Rep (2021) 45(3):1171–81. doi: 10.3892/or.2021.7936
89. Deng Z, Rong Y, Teng Y, Zhuang X, Samykutty A, Mu J, et al. Exosomes miR-126a released from MDSC induced by DOX treatment promotes lung metastasis. Oncogene (2017) 36(5):639–51. doi: 10.1038/onc.2016.229
90. Burke M, Choksawangkarn W, Edwards N, Ostrand-Rosenberg S, Fenselau C. Exosomes from myeloid-derived suppressor cells carry biologically active proteins. J Proteome Res (2014) 13(2):836–43. doi: 10.1021/pr400879c
91. Geis-Asteggiante L, Belew AT, Clements VK, Edwards NJ, Ostrand-Rosenberg S, El-Sayed NM, et al. Differential content of proteins, mRNAs, and miRNAs suggests that MDSC and their exosomes may mediate distinct immune suppressive functions. J Proteome Res (2018) 17(1):486–98. doi: 10.1021/acs.jproteome.7b00646
92. Chauhan S, Danielson S, Clements V, Edwards N, Ostrand-Rosenberg S, Fenselau C. Surface glycoproteins of exosomes shed by myeloid-derived suppressor cells contribute to function. J Proteome Res (2017) 16(1):238–46. doi: 10.1021/acs.jproteome.6b00811
93. Zhu D, Tian J, Wu X, Li M, Tang X, Rui K, et al. G-MDSC-derived exosomes attenuate collagen-induced arthritis by impairing Th1 and Th17 cell responses. Biochim Biophys Acta Mol Basis Dis (2019) 1865(12):165540. doi: 10.1016/j.bbadis.2019.165540
94. Zöller M, Zhao K, Kutlu N, Bauer N, Provaznik J, Hackert T, et al. Immunoregulatory effects of myeloid-derived suppressor cell exosomes in mouse model of autoimmune alopecia areata. Front Immunol (2018) 9:1279. doi: 10.3389/fimmu.2018.01279
95. Pang WW, Price EA, Sahoo D, Beerman I, Maloney WJ, Rossi DJ, et al. Human bone marrow hematopoietic stem cells are increased in frequency and myeloid-biased with age. Proc Natl Acad Sci U.S.A. (2011) 108(50):20012–7. doi: 10.1073/pnas.1116110108
96. Franceschi C, Campisi J. Chronic inflammation (inflammaging) and its potential contribution to age-associated diseases. J Gerontol A Biol Sci Med Sci (2014) 69 Suppl 1:S4–9. doi: 10.1093/gerona/glu057
97. Flores RR, Clauson CL, Cho J, Lee BC, McGowan SJ, Baker DJ, et al. Expansion of myeloid-derived suppressor cells with aging in the bone marrow of mice through a NF-κB-dependent mechanism. Aging Cell (2017) 16(3):480–7. doi: 10.1111/acel.12571
98. Verschoor CP, Johnstone J, Millar J, Dorrington MG, Habibagahi M, Lelic A, et al. Blood CD33(+)HLA-DR(-) myeloid-derived suppressor cells are increased with age and a history of cancer. J Leukoc Biol (2013) 93(4):633–7. doi: 10.1189/jlb.0912461
99. Zou L, Jiang W, Wang Z, Chen J, Zhu S. Effect of advanced oxidation protein products (AOPPs) and aging on the osteoclast differentiation of myeloid-derived suppressor cells (MDSCs) and its preliminary mechanism. Biochem Biophys Res Commun (2022) 636(Pt 2):87–96. doi: 10.1016/j.bbrc.2022.10.066
100. Li Z, Zhao Y, Chen Z, Katz J, Michalek SM, Li Y, et al. Age-related expansion and increased osteoclastogenic potential of myeloid-derived suppressor cells. Mol Immunol (2021) 137:187–200. doi: 10.1016/j.molimm.2021.07.004
101. Xiao H, Jedrychowski MP, Schweppe DK, Huttlin EL, Yu Q, Heppner DE, et al. A quantitative tissue-specific landscape of protein redox regulation during aging. Cell (2020) 180(5):968–83.e24. doi: 10.1016/j.cell.2020.02.012
102. Capel F, Rimbert V, Lioger D, Diot A, Rousset P, Mirand PP, et al. Due to reverse electron transfer, mitochondrial H2O2 release increases with age in human vastus lateralis muscle although oxidative capacity is preserved. Mech Ageing Dev (2005) 126(4):505–11. doi: 10.1016/j.mad.2004.11.001
103. Davalli P, Mitic T, Caporali A, Lauriola A, D'Arca D. ROS, cell senescence, and novel molecular mechanisms in aging and age-related diseases. Oxid Med Cell Longev (2016) 2016:3565127. doi: 10.1155/2016/3565127
104. Geng Q, Gao H, Yang R, Guo K, Miao D. Pyrroloquinoline quinone prevents estrogen deficiency-induced osteoporosis by inhibiting oxidative stress and osteocyte senescence. Int J Biol Sci (2019) 15(1):58–68. doi: 10.7150/ijbs.25783
105. Manolagas SC. From estrogen-centric to aging and oxidative stress: A revised perspective of the pathogenesis of osteoporosis. Endocr Rev (2010) 31(3):266–300. doi: 10.1210/er.2009-0024
106. Ahern E, Harjunpää H, Barkauskas D, Allen S, Takeda K, Yagita H, et al. Co-administration of RANKL and CTLA4 antibodies enhances lymphocyte-mediated antitumor immunity in mice. Clin Cancer Res (2017) 23(19):5789–801. doi: 10.1158/1078-0432.Ccr-17-0606
107. Tilstra JS, Robinson AR, Wang J, Gregg SQ, Clauson CL, Reay DP, et al. NF-κB inhibition delays DNA damage-induced senescence and aging in mice. J Clin Invest (2012) 122(7):2601–12. doi: 10.1172/jci45785
108. Kirkwood KL, Zhang L, Thiyagarajan R, Seldeen KL, Troen BR. Myeloid-derived suppressor cells at the intersection of inflammaging and bone fragility. Immunol Invest (2018) 47(8):844–54. doi: 10.1080/08820139.2018.1552360
109. Charles JF, Hsu LY, Niemi EC, Weiss A, Aliprantis AO, Nakamura MC. Inflammatory arthritis increases mouse osteoclast precursors with myeloid suppressor function. J Clin Invest (2012) 122(12):4592–605. doi: 10.1172/jci60920
110. Michalski MN, Seydel AL, Siismets EM, Zweifler LE, Koh AJ, Sinder BP, et al. Inflammatory bone loss associated with MFG-E8 deficiency is rescued by teriparatide. FASEB J (2018) 32(7):3730–41. doi: 10.1096/fj.201701238R
111. Chen S, Guo C, Wang R, Feng Z, Liu Z, Wu L, et al. Monocytic MDSCs skew Th17 cells toward a pro-osteoclastogenic phenotype and potentiate bone erosion in rheumatoid arthritis. Rheumatol (Oxford) (2020) 60(5):2409–20. doi: 10.1093/rheumatology/keaa625
112. Liu YF, Zhuang KH, Chen B, Li PW, Zhou X, Jiang H, et al. Expansion and activation of monocytic-myeloid-derived suppressor cell via STAT3/arginase-i signaling in patients with ankylosing spondylitis. Arthritis Res Ther (2018) 20(1):168. doi: 10.1186/s13075-018-1654-4
113. Zhang L, Zhang Z, Zhang H, Wu M, Wang Y. Myeloid-derived suppressor cells protect mouse models from autoimmune arthritis via controlling inflammatory response. Inflammation (2014) 37(3):670–7. doi: 10.1007/s10753-013-9783-z
114. Park MJ, Baek JA, Choi JW, Jang SG, Kim DS, Park SH, et al. Programmed death-ligand 1 expression potentiates the immune modulatory function of myeloid-derived suppressor cells in systemic lupus erythematosus. Front Immunol (2021) 12:606024. doi: 10.3389/fimmu.2021.606024
115. Guo C, Hu F, Yi H, Feng Z, Li C, Shi L, et al. Myeloid-derived suppressor cells have a proinflammatory role in the pathogenesis of autoimmune arthritis. Ann Rheum Dis (2016) 75(1):278–85. doi: 10.1136/annrheumdis-2014-205508
116. Zhang H, Wang S, Huang Y, Wang H, Zhao J, Gaskin F, et al. Myeloid-derived suppressor cells are proinflammatory and regulate collagen-induced arthritis through manipulating Th17 cell differentiation. Clin Immunol (2015) 157(2):175–86. doi: 10.1016/j.clim.2015.02.001
117. Tcyganov E, Mastio J, Chen E, Gabrilovich DI. Plasticity of myeloid-derived suppressor cells in cancer. Curr Opin Immunol (2018) 51:76–82. doi: 10.1016/j.coi.2018.03.009
118. Kienzle A, Walter S, von Roth P, Fuchs M, Winkler T, Muller M. High rates of aseptic loosening after revision total knee arthroplasty for periprosthetic joint infection. JB JS Open Access (2020) 5(3):e20.00026. doi: 10.2106/JBJS.OA.20.00026
119. Kienzle A, Walter S, Palmowski Y, Kirschbaum S, Biedermann L, von Roth P, et al. Influence of gender on occurrence of aseptic loosening and recurrent PJI after revision total knee arthroplasty. Osteology (2021) 1(2):92–104. doi: 10.3390/osteology1020010
120. Arciola CR, Campoccia D, Montanaro L. Implant infections: Adhesion, biofilm formation and immune evasion. Nat Rev Microbiol (2018) 16(7):397–409. doi: 10.1038/s41579-018-0019-y
121. Conlon BP, Rowe SE, Gandt AB, Nuxoll AS, Donegan NP, Zalis EA, et al. Persister formation in staphylococcus aureus is associated with ATP depletion. Nat Microbiol (2016) 1:16051. doi: 10.1038/nmicrobiol.2016.51
122. Walters MC 3rd, Roe F, Bugnicourt A, Franklin MJ, Stewart PS. Contributions of antibiotic penetration, oxygen limitation, and low metabolic activity to tolerance of pseudomonas aeruginosa biofilms to ciprofloxacin and tobramycin. Antimicrob Agents Chemother (2003) 47(1):317–23. doi: 10.1128/aac.47.1.317-323.2003
123. Aldrich AL, Horn CM, Heim CE, Korshoj LE, Kielian T. Transcriptional diversity and niche-specific distribution of leukocyte populations during staphylococcus aureus craniotomy-associated biofilm infection. J Immunol (2021) 206(4):751–65. doi: 10.4049/jimmunol.2001042
124. Heim CE, Vidlak D, Scherr TD, Kozel JA, Holzapfel M, Muirhead DE, et al. Myeloid-derived suppressor cells contribute to staphylococcus aureus orthopedic biofilm infection. J Immunol (2014) 192(8):3778–92. doi: 10.4049/jimmunol.1303408
125. Hofstee MI, Riool M, Gieling F, Stenger V, Constant C, Nehrbass D, et al. A murine staphylococcus aureus fracture-related infection model characterised by fracture non-union, staphylococcal abscess communities and myeloid-derived suppressor cells. Eur Cell Mater (2021) 41:774–92. doi: 10.22203/eCM.v041a49
126. Vantucci CE, Ahn H, Fulton T, Schenker ML, Pradhan P, Wood LB, et al. Development of systemic immune dysregulation in a rat trauma model of biomaterial-associated infection. Biomaterials (2021) 264:120405. doi: 10.1016/j.biomaterials.2020.120405
127. Heim CE, Vidlak D, Kielian T. Interleukin-10 production by myeloid-derived suppressor cells contributes to bacterial persistence during staphylococcus aureus orthopedic biofilm infection. J Leukoc Biol (2015) 98(6):1003–13. doi: 10.1189/jlb.4VMA0315-125RR
128. Heim CE, Yamada KJ, Fallet R, Odvody J, Schwarz DM, Lyden ER, et al. Orthopaedic surgery elicits a systemic anti-inflammatory signature. J Clin Med (2020) 9(7):2123. doi: 10.3390/jcm9072123
129. Peng KT, Hsieh CC, Huang TY, Chen PC, Shih HN, Lee MS, et al. Staphylococcus aureus biofilm elicits the expansion, activation and polarization of myeloid-derived suppressor cells in vivo and in vitro. PloS One (2017) 12(8):e0183271. doi: 10.1371/journal.pone.0183271
130. He W, Liu X, Kienzle A, Muller WE, Feng Q. In vitro uptake of silver nanoparticles and their toxicity in human mesenchymal stem cells derived from bone marrow. J Nanosci Nanotechnol (2016) 16(1):219–28. doi: 10.1166/jnn.2016.10728
131. Peng KT, Chiang YC, Huang TY, Chen PC, Chang PJ, Lee CW. Curcumin nanoparticles are a promising anti-bacterial and anti-inflammatory agent for treating periprosthetic joint infections. Int J Nanomedicine (2019) 14:469–81. doi: 10.2147/ijn.S191504
132. Su L, Xu Q, Zhang P, Michalek SM, Katz J. Phenotype and function of myeloid-derived suppressor cells induced by porphyromonas gingivalis infection. Infect Immun (2017) 85(8):e00213–17. doi: 10.1128/iai.00213-17
133. Patera AC, Drewry AM, Chang K, Beiter ER, Osborne D, Hotchkiss RS. Frontline science: Defects in immune function in patients with sepsis are associated with PD-1 or PD-L1 expression and can be restored by antibodies targeting PD-1 or PD-L1. J Leukoc Biol (2016) 100(6):1239–54. doi: 10.1189/jlb.4HI0616-255R
134. Ruan WS, Feng MX, Xu J, Xu YG, Song CY, Lin LY, et al. Early activation of myeloid-derived suppressor cells participate in sepsis-induced immune suppression via PD-L1/PD-1 axis. Front Immunol (2020) 11:1299. doi: 10.3389/fimmu.2020.01299
135. Wang K, Gu Y, Liao Y, Bang S, Donnelly CR, Chen O, et al. PD-1 blockade inhibits osteoclast formation and murine bone cancer pain. J Clin Invest (2020) 130(7):3603–20. doi: 10.1172/jci133334
136. Moseley KF, Naidoo J, Bingham CO, Carducci MA, Forde PM, Gibney GT, et al. Immune-related adverse events with immune checkpoint inhibitors affecting the skeleton: a seminal case series. J Immunother Cancer (2018) 6(1):104. doi: 10.1186/s40425-018-0417-8
137. Thompson K, Freitag L, Styger U, Camenisch K, Zeiter S, Arens D, et al. Impact of low bone mass and antiresorptive therapy on antibiotic efficacy in a rat model of orthopedic device-related infection. J Orthop Res (2021) 39(2):415–25. doi: 10.1002/jor.24951
138. Sedghizadeh PP, Sun S, Junka AF, Richard E, Sadrerafi K, Mahabady S, et al. Design, synthesis, and antimicrobial evaluation of a novel bone-targeting bisphosphonate-ciprofloxacin conjugate for the treatment of osteomyelitis biofilms. J Med Chem (2017) 60(6):2326–43. doi: 10.1021/acs.jmedchem.6b01615
139. Grigoryan KV, Javedan H, Rudolph JL. Orthogeriatric care models and outcomes in hip fracture patients: A systematic review and meta-analysis. J Orthop Trauma (2014) 28(3):e49–55. doi: 10.1097/BOT.0b013e3182a5a045
140. Holmes D. Non-union bone fracture: A quicker fix. Nature (2017) 550(7677):S193. doi: 10.1038/550S193a
141. Hüsecken Y, Muche S, Kustermann M, Klingspor M, Palmer A, Braumüller S, et al. MDSCs are induced after experimental blunt chest trauma and subsequently alter antigen-specific t cell responses. Sci Rep (2017) 7(1):12808. doi: 10.1038/s41598-017-13019-6
142. Cheng A, Vantucci CE, Krishnan L, Ruehle MA, Kotanchek T, Wood LB, et al. Early systemic immune biomarkers predict bone regeneration after trauma. Proc Natl Acad Sci U.S.A. (2021) 118(8):e2017889118. doi: 10.1073/pnas.2017889118
143. Saiwai H, Kumamaru H, Ohkawa Y, Kubota K, Kobayakawa K, Yamada H, et al. Ly6C+ Ly6G- myeloid-derived suppressor cells play a critical role in the resolution of acute inflammation and the subsequent tissue repair process after spinal cord injury. J Neurochem (2013) 125(1):74–88. doi: 10.1111/jnc.12135
144. Zhou L, Miao K, Yin B, Li H, Fan J, Zhu Y, et al. Cardioprotective role of myeloid-derived suppressor cells in heart failure. Circulation (2018) 138(2):181–97. doi: 10.1161/circulationaha.117.030811
145. Wang W, Zuo R, Long H, Wang Y, Zhang Y, Sun C, et al. Advances in the masquelet technique: Myeloid-derived suppressor cells promote angiogenesis in PMMA-induced membranes. Acta Biomater (2020) 108:223–36. doi: 10.1016/j.actbio.2020.03.010
146. Kawai H, Oo MW, Tsujigiwa H, Nakano K, Takabatake K, Sukegawa S, et al. Potential role of myeloid-derived suppressor cells in transition from reaction to repair phase of bone healing process. Int J Med Sci (2021) 18(8):1824–30. doi: 10.7150/ijms.51946
147. Guan Y, Zhang R, Peng Z, Dong D, Wei G, Wang Y. Inhibition of IL-18-mediated myeloid derived suppressor cell accumulation enhances anti-PD1 efficacy against osteosarcoma cancer. J Bone Oncol (2017) 9:59–64. doi: 10.1016/j.jbo.2017.10.002
148. Jiang K, Li J, Zhang J, Wang L, Zhang Q, Ge J, et al. SDF-1/CXCR4 axis facilitates myeloid-derived suppressor cells accumulation in osteosarcoma microenvironment and blunts the response to anti-PD-1 therapy. Int Immunopharmacol (2019) 75:105818. doi: 10.1016/j.intimp.2019.105818
149. Shi X, Li X, Wang H, Yu Z, Zhu Y, Gao Y. Specific inhibition of PI3Kδ/γ enhances the efficacy of anti-PD1 against osteosarcoma cancer. J Bone Oncol (2019) 16:100206. doi: 10.1016/j.jbo.2018.11.001
150. Horlad H, Fujiwara Y, Takemura K, Ohnishi K, Ikeda T, Tsukamoto H, et al. Corosolic acid impairs tumor development and lung metastasis by inhibiting the immunosuppressive activity of myeloid-derived suppressor cells. Mol Nutr Food Res (2013) 57(6):1046–54. doi: 10.1002/mnfr.201200610
151. Xu X, Zhang C, Trotter TN, Gowda PS, Lu Y, Ponnazhagan S, et al. Runx2 deficiency in osteoblasts promotes myeloma progression by altering the bone microenvironment at new bone sites. Cancer Res (2020) 80(5):1036–48. doi: 10.1158/0008-5472.Can-19-0284
152. Pioli PD, Casero D, Montecino-Rodriguez E, Morrison SL, Dorshkind K. Plasma cells are obligate effectors of enhanced myelopoiesis in aging bone marrow. Immunity (2019) 51(2):351–66.e6. doi: 10.1016/j.immuni.2019.06.006
153. Sai B, Dai Y, Fan S, Wang F, Wang L, Li Z, et al. Cancer-educated mesenchymal stem cells promote the survival of cancer cells at primary and distant metastatic sites via the expansion of bone marrow-derived-PMN-MDSCs. Cell Death Dis (2019) 10(12):941. doi: 10.1038/s41419-019-2149-1
154. Zhu H, Gu Y, Xue Y, Yuan M, Cao X, Liu Q. CXCR2(+) MDSCs promote breast cancer progression by inducing EMT and activated t cell exhaustion. Oncotarget (2017) 8(70):114554–67. doi: 10.18632/oncotarget.23020
155. Ouzounova M, Lee E, Piranlioglu R, El Andaloussi A, Kolhe R, Demirci MF, et al. Monocytic and granulocytic myeloid derived suppressor cells differentially regulate spatiotemporal tumour plasticity during metastatic cascade. Nat Commun (2017) 8:14979. doi: 10.1038/ncomms14979
156. Yang L, DeBusk LM, Fukuda K, Fingleton B, Green-Jarvis B, Shyr Y, et al. Expansion of myeloid immune suppressor Gr+CD11b+ cells in tumor-bearing host directly promotes tumor angiogenesis. Cancer Cell (2004) 6(4):409–21. doi: 10.1016/j.ccr.2004.08.031
157. Teijeira Á, Garasa S, Gato M, Alfaro C, Migueliz I, Cirella A, et al. CXCR1 and CXCR2 chemokine receptor agonists produced by tumors induce neutrophil extracellular traps that interfere with immune cytotoxicity. Immunity (2020) 52(5):856–71.e8. doi: 10.1016/j.immuni.2020.03.001
158. Alfaro C, Teijeira A, Oñate C, Pérez G, Sanmamed MF, Andueza MP, et al. Tumor-produced interleukin-8 attracts human myeloid-derived suppressor cells and elicits extrusion of neutrophil extracellular traps (NETs). Clin Cancer Res (2016) 22(15):3924–36. doi: 10.1158/1078-0432.Ccr-15-2463
159. Kwak T, Wang F, Deng H, Condamine T, Kumar V, Perego M, et al. Distinct populations of immune-suppressive macrophages differentiate from monocytic myeloid-derived suppressor cells in cancer. Cell Rep (2020) 33(13):108571. doi: 10.1016/j.celrep.2020.108571
160. Biswas S, Mandal G, Roy Chowdhury S, Purohit S, Payne KK, Anadon C, et al. Exosomes produced by mesenchymal stem cells drive differentiation of myeloid cells into immunosuppressive M2-polarized macrophages in breast cancer. J Immunol (2019) 203(12):3447–60. doi: 10.4049/jimmunol.1900692
161. Sinha P, Clements VK, Bunt SK, Albelda SM, Ostrand-Rosenberg S. Cross-talk between myeloid-derived suppressor cells and macrophages subverts tumor immunity toward a type 2 response. J Immunol (2007) 179(2):977–83. doi: 10.4049/jimmunol.179.2.977
162. Sawant A, Deshane J, Jules J, Lee CM, Harris BA, Feng X, et al. Myeloid-derived suppressor cells function as novel osteoclast progenitors enhancing bone loss in breast cancer. Cancer Res (2013) 73(2):672–82. doi: 10.1158/0008-5472.Can-12-2202
163. An G, Acharya C, Feng X, Wen K, Zhong M, Zhang L, et al. Osteoclasts promote immune suppressive microenvironment in multiple myeloma: Therapeutic implication. Blood (2016) 128(12):1590–603. doi: 10.1182/blood-2016-03-707547
Keywords: myeloid derived suppressor cell (MDSC), bone metabolism, osteoclast, osteoblast, immune cells, inflammation, osteoimmunology
Citation: Ren Y, Bäcker H, Müller M and Kienzle A (2023) The role of myeloid derived suppressor cells in musculoskeletal disorders. Front. Immunol. 14:1139683. doi: 10.3389/fimmu.2023.1139683
Received: 07 January 2023; Accepted: 21 February 2023;
Published: 03 March 2023.
Edited by:
Bettina Willie, McGill University, CanadaReviewed by:
Yuanliang Xia, First Affiliated Hospital of Jilin University, ChinaMichael Fuchs, Universitäts- und Rehabilitationskliniken Ulm, Germany
Copyright © 2023 Ren, Bäcker, Müller and Kienzle. This is an open-access article distributed under the terms of the Creative Commons Attribution License (CC BY). The use, distribution or reproduction in other forums is permitted, provided the original author(s) and the copyright owner(s) are credited and that the original publication in this journal is cited, in accordance with accepted academic practice. No use, distribution or reproduction is permitted which does not comply with these terms.
*Correspondence: Arne Kienzle, YXJuZS5raWVuemxlQGNoYXJpdGUuZGU=