Commentary: Mesenchymal stem cells in the treatment of spinal cord injury: mechanisms, current advances and future challenges
- 1Department of Spine Surgery, The First Hospital of Jilin University, Changchun, China
- 2Cancer Center, The First Hospital of Jilin University, Changchun, China
Spinal cord injury (SCI) has considerable impact on patient physical, mental, and financial health. Secondary SCI is associated with inflammation, vascular destruction, and subsequent permanent damage to the nervous system. Mesenchymal stem cells (MSCs) have anti-inflammatory properties, promoting vascular regeneration and the release neuro-nutrients, and are a promising strategy for the treatment of SCI. Preclinical studies have shown that MSCs promote sensory and motor function recovery in rats. In clinical trials, MSCs have been reported to improve the American Spinal Injury Association (ASIA) sensory and motor scores. However, the effectiveness of MSCs in treating patients with SCI remains controversial. MSCs promote tumorigenesis and ensuring the survival of MSCs in the hostile environment of SCI is challenging. In this article we examine the evidence on the pathophysiological changes occurring after SCI. We then review the underlying mechanisms of MSCs in the treatment of SCI and summarize the potential application of MSCs in clinical practice. Finally, we highlight the challenges surrounding the use of MSCs in the treatment of SCI and discuss future applications.
1 Introduction
Spinal cord injury (SCI) is the most serious complication of spinal injury and usually results in transient or permanent loss of sensory, motor, and autonomic nerves below the level of injury (1). The overall global incidence of SCI is 10.5 per 100,000 persons (2). Although 94% of patients with acute traumatic SCI survive treatment, survival is greatly reduced due to post-injury complications (3). Current therapeutic approaches for SCI that are part of standard care include the reduction of fractures, surgical decompression of the spinal canal and stabilization of the spine, and rehabilitation. However, standard treatment does not directly promote neuroregeneration, but may only reduce the effects of secondary injury and improve the conditions under which the endogenous mechanisms of repair act. The ideal result is to achieve rapid recovery of neurological function after the release of compression by medical and surgical intervention (4). However, there are currently no optimal treatment strategies to repair damaged nerve cells.
Regenerative medicine strategies based on cell therapy have attracted interest in recent years (5). Mesenchymal stem cells (MSCs) have the ability to differentiate and self-proliferate, and there has been an increasing focus on their use as potential therapeutic agents for a variety of diseases. MSCs release cytokines and exosomes to reduce inflammation at the site of injury. MSCs also release vascular endothelial growth factor (VEGF), nerve growth factor (NGF), glia-derived neurotrophic factor (GDNF) and brain-derived neurotrophic factor (BDNF) to promote nerve cells regeneration and inhibit glial scarring (6, 7). The use of MSCs represents a promising approach for SCI cell therapy. MSC transplantation therapy for SCI has been clinically proven to promote sensory and functional recovery in patients with SCI (8–11). However, stem cells have the ability to differentiate into multiple cell types and transplantation has been associated with tumour regeneration. Tumour regeneration is a reason why stem cell transplantation investigated with caution (12). In addition, the low survival rate of stem cells under the hostile conditions of injury is also one of the main factors limiting their clinical application (13). In this paper, we describe the microenvironment alterations of the spinal cord after injury. Second, we summarize the role of MSCs in SCI. Finally, we highlight the challenges surrounding the use of MSCs in the treatment of SCI and discuss future applications.
2 Pathophysiology of spinal cord injury
2.1 Primary spinal cord injury
Primary SCI is usually caused by trauma resulting in fracture or dislocation of the vertebrae and compression, tear, or transection of the spinal cord (14). Spinal cord compression is the most common mechanism and is often accompanied by disruption of the vasculature and damage to the blood-spinal cord barrier (BSCB) (3). In the healthy spinal cord, the BSCB is essential to maintain spinal cord homeostasis of spinal cord with stability (15). The concept that the spinal cord is privileged from normal immune surveillance is derived from the fact that in the healthy central nervous system there are no peripheral immune cells (16). The BSCB can resist foreign immune cells and toxic metabolites and maintain the stability of the spinal cord microenvironment. When the BSCB is destroyed, pro-inflammatory cytokines (TNF-α, IL-β, and IL-6), free radicals, and toxic substances can enter the area of injury, aggravating neuronal damage.
2.2 Secondary spinal cord injury
Secondary SCI is characterized by a series of cellular and molecular changes that begin within minutes of the primary injury. Secondary injury consists of three consecutive and overlapping phases: acute (within 48 hours of injury), subacute (48 hours to two weeks after injury), and chronic (lasting up to six months after injury) (17). Secondary injuries usually worsen the injury and cause permanent harm to the patient.
Disruption of the microvascular supply aggravates cell death a few minutes after SCI. When spinal cord cells are destroyed, damage-associated molecular patterns (DAMPs) are released, which induce a potent inflammatory response (18). With the activation of pattern recognition receptors (PRRs), resident and peripheral immune cells are recruited at the lesion site (19). Resident microglia, astrocytes, and peripheral-derived immune cells upregulate the expression of inflammatory factors TNF, IL-1, and IL-6 (20). The upregulation of inflammatory factors further aggravates the extensive infiltration of immune cells, which is the main cause of neurodegeneration (21). Neutrophils are known to be the first peripheral cells recruited at the site of injury (22). Reactive oxygen species (ROS) and matrix metalloproteinases (MMPs), released by neutrophils when they engulf necrotic matter and debris, lead to secondary tissue damage (23). Unlike other immune cells, the neutrophil response begins within one hour of injury and the increase in neutrophils persists at the site of injury for 3 days (24).
Microglia and monocytes-derived macrophages (MDMs) are the main cell types that trigger neuroinflammatory responses (25). Microglia are resident immune cells in the central immune system and have immune defence functions and maintain the stability of the nervous system (26). In the healthy spinal cord microenvironment, microglia are in a stable state. In the early stages of injury, the phagocytic ability of microglia allows removal of the surrounding necrotic tissue and debris (27). The positive effect of microglia at the site of injury lasts only one week, and subsequently, activated microglia and macrophages also release ROS and pro-inflammatory factors, leading to secondary damage (27, 28). Microglia, astrocytes, and oligodendrocytes form dense boundary structures of glial scars near the damaged tissue (29). The formation of glial scarring prevents immune cell infiltration and reduces inflammation (30). However, glial scarring is also an important barrier to neuronal regeneration after SCI (27). Microglia are macrophages residing in the central system, and after SCI, microglia are activated before bloodborne macrophages (31). Activated microglia then recruit bloodborne macrophages. Studies have shown that after SCI, M1 macrophages are mainly derived from microglia, and a small number of these are derived from blood-derived macrophages (32). In SCI, activated microglia secrete inflammatory factors such as IL-β, TNF-α, and IL-6 to promote the infiltration of blood-derived macrophages into the surrounding damaged tissue (33). Bloodborne macrophages include pro-inflammatory M1 and anti-inflammatory M2. Compared with microglia, M1 macrophages have a stronger phagocytic ability (34). In the subacute phase of SCI, M1 macrophages release IL-β, TNF-α, IL-6, interferon-γ, NO, and ROS, resulting in vascular endothelial damage and axonal damage. M2 macrophages release anti-inflammatory cytokines and neurotrophic factors (IL-4, IL-10, IL-13, transforming growth factor β (TGF-β) and insulin-like growth factor (IGF)) to provide the environment for neural regeneration (35). The SCI microenvironment is complex and changeable, and the regeneration of damaged neurons and axons cannot be achieved by self-regulation alone (36). Therefore, it is necessary to reverse the hostile SCI microenvironment to promote SCI recovery.
3 Mesenchymal stem cells regulate spinal cord injury
MSCs derive from a wide range of sources and have self-proliferation and multidirectional differentiation capabilities (37). MSCs are widely used in cell therapy and regenerative medicine because of their immunomodulatory and tissue repair effects (5, 38). MSCs can be isolated from almost all tissues, including bone marrow, adipose tissue, amniotic fluid, umbilical cord, liver, and heart (39). MSCs isolated from various tissues exhibit various cell surface markers that can be used for a range of treatment options. MSCs are nonimmunogenic, highly viable and are known to provide structural support in SCI (40). The advantage of MSCs is that they are easy to isolate and preserve, and do not raise specifically ethical issues (41). The most commonly used types of MSCs in clinical practice are bone marrow mesenchymal stem cells (BM-MSCs), human umbilical cord mesenchymal stem cells (HUC-MSCs), and fat-derived mesenchymal stem cells (AD-MSCs) (42).
BMSCs have strong differentiation potential under various induction conditions and can be divided into different subtypes of osteoblasts, chondrocytes, adipocytes, fibroblasts, and neurons and glial cells (43). BMSCs mainly play a role in inhibiting immunity against inflammation, promoting the transition of M1 macrophages to M2 type and releasing neurotrophic factors (7). The disadvantage of BMSCs is that they require patients to undergo bone marrow aspiration under local anaesthesia. AD-MSCs are derived from adipose tissue. A key feature of AD-MSCs is that they can be obtained in large quantities without causing extensive damage. Compared to BMSCs, AD-MSCs release growth factors, extracellular matrix molecules, and proteases to promote angiogenesis and wound healing (44). HUC-MSCs have stronger differentiation and proliferation capabilities than BMSCs (45). In addition, HUC-MSCs are small in size, pass through the BSCB system more easily, and do not cause fat embolism and vascular embolism (46). The role of MSCs in SCI can be broadly summarized as suppressing immunity against inflammation, releasing nutritional factors to promote neurological recovery, and stimulating angiogenesis to remodel BSCBs.
3.1 Anti-inflammatory role
The most attractive aspect of MSCs in regulating regeneration is their unique immunomodulatory ability (47). The immunomodulatory mechanism of MSCs is mainly mediated by direct contact between cells and immune cells and paracrine activity (48). When SCI occurs, microglial activation and macrophage activation release a large number of cytokines and growth factors, including TGF-β, basic fibroblast growth factor, IL-6, andIL-1 (49). Transplantation of MSCs reduces the inflammatory response after SCI (Figure 1). The inhibition of inflammation by MSCs is more dependent on the realization of exosomes produced by their paracrine effect. Exosomes are membrane vesicular structures approximately 30-150 nm in diameter produced by MSCs through the paracrine pathway. As a novel intercellular communication substance, extracellular vesicles (EVs) are involved in cell proliferation and inhibit apoptosis and inflammation to mediate tissue repair (50). EVs can act as a vehicle to target inflammatory regions in vivo, releasing biomolecular cargoes to regulate the inflammatory response at SCI sites (51). Transplantation of BMSCs into a SCI rat contusion model significantly upregulated the number of M2 macrophages at the injury site and downregulated the number of M1 macrophages, while the levels of IL-4 and IL-13 increased, and the levels of TNF-a and IL-6 decreased (52).
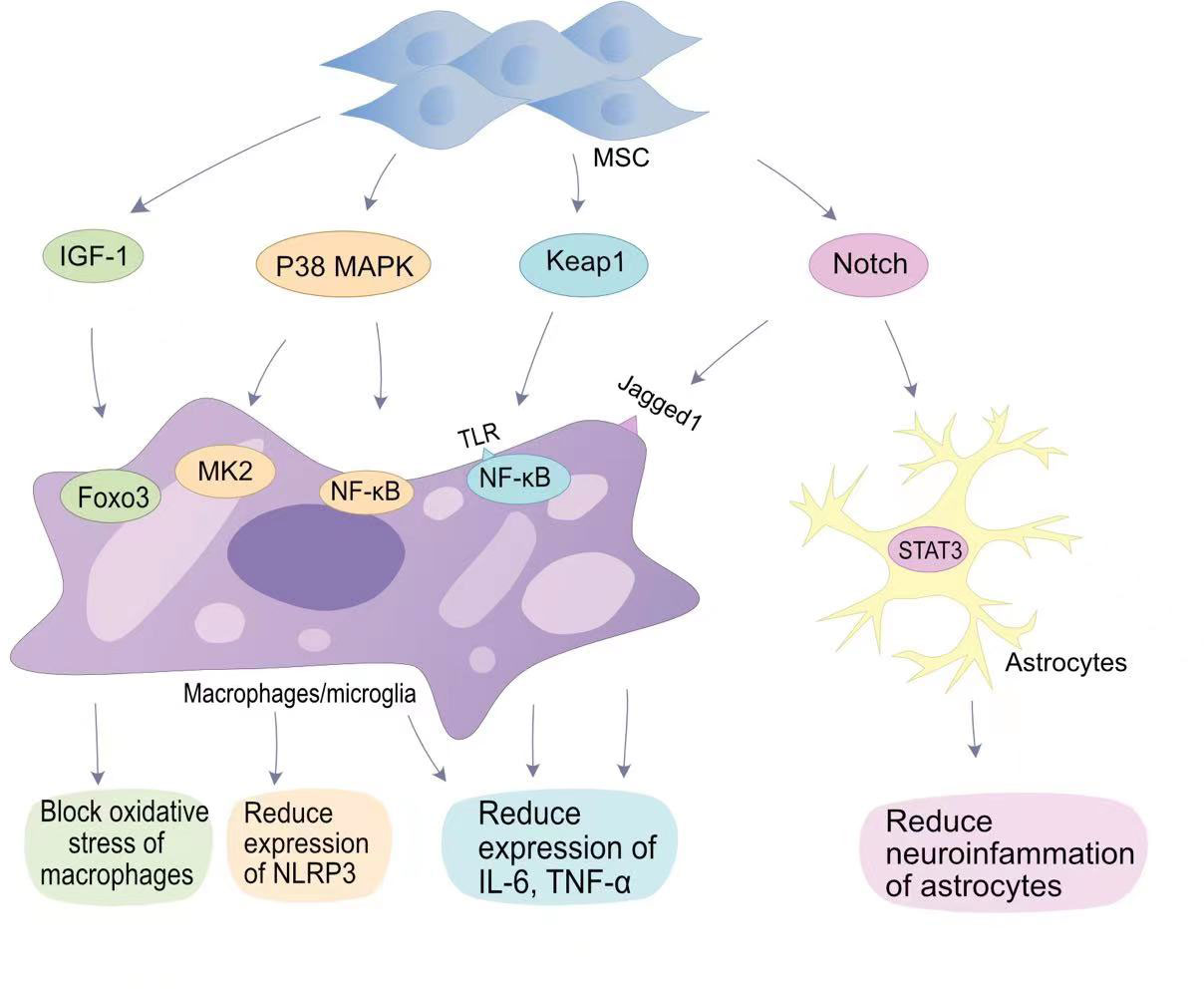
Figure 1 MSCs in anti-inflammatory signaling pathways in SCI. MSCs reduce oxygen partial pressure at the site of injury through the IGF-1-Foxo3 signaling pathway.MSCs reduce NLRP3 expression through the P38 MAPK-MK2 pathway and MAPK-NF-κB pathway. MSCs bind to TLR on the surface of macrophages/microglia via Keap1, thereby reducing IL-6 and TNF-α expression by NF-κB; MSCs reduce neuropathic inflammation through the Notch-STAT3 pathway, which is beneficial for SCI repair.
The classical mitogen-activated protein kinases (MAPKs) pathway (p38 MAPK), also known as stress-associated protein kinases, can be activated during inflammation (53). In SCI, p38 MAPK-mediated inflammatory responses have been demonstrated. Toll-like receptors (TLRs) on macrophages upregulate the expression of iNOS, cyclooxygenase 2, IL-6, and TNF-α in response to a signal by p38 MAPK (54). In addition, p38 MAPK promotes the translation of cytokines in NK cells and T cells by upregulating the MAPK interaction kinase (Mnk) pathway (55). Therefore, p38 can mobilize major SCI-associated pro-inflammatory cytokines in post-traumatic inflammatory processes (53). Li et al. found that HUC-MSCs inhibit p38 activation in microglia and macrophages and reduce SCI central inflammation by inhibiting TLR4 and NF-κB signalling pathways (56). The miRNA delivered by BMSC-EVs targets TLR4 and inhibits the activation of the NF-κB pathway, alleviating the inflammatory response (57–59). Complement (C3/C5) is involved in the NF-κB signalling pathway. Zhao et al. found that BMSCs-EVs inhibit complement mRNA synthesis and release, and inhibit the activation of NF-κB signalling by binding to microglia (60). Blocking the activation of NF-κB signalling reduces the release of pro-inflammatory inflammatory factors.
The Notch pathway is a highly conserved signalling pathway that plays a role in vivo by promoting cell differentiation and stem cell proliferation, and maintaining cell motility (61). The Jagged1/Notch1 signalling pathway plays an important role in endogenous neurogenesis and reducing inflammation at the site of injury, and is considered to be an important target for reducing inflammation after SCI (62). Notch1 was confirmed in SCI mouse models with an initial elevation on the first day after injury which persisted until 14 days after injury. After Zhou et al. blocked the Jagged1/Notch1 signalling pathway using Jagged1 siRNA, the expression of the pro-inflammatory factors IL-1β, IL-6, and TNF-α in SCI mouse models was significantly reduced (63). MSCs transplantation can hinder Jagged1/Notch1 signalling pathway conduction after SCI, resulting in weaker expression of Notch and its downstream factors in microglia/macrophages (63). Astrocytes mainly differentiate into A1 neurotoxic-reactive astrocytes and A2 protective astrocytes in central nervous system diseases (64). The signalling mechanism that regulates astrocyte responsiveness after SCI is an important target for alleviating neuroinflammation. Notch1 can alter STAT3 phosphorylation (65). MSCs blocking the Jagged1/Notch signalling pathway can indirectly inhibit phosphorylation of the JAK/STAT3 signalling pathway in A1 neurotoxic-responsive astrocytes to achieve anti-inflammatory effects (63).
Tristetraprolin (TTP) is a substrate of p38MAPK-activated protein kinase 2 (MK2) (66). The p38/MK2 pathway is a key regulator of TTP expression, stability, and function. Previous studies have shown that MK2 can promote the expression of nucleotide-binding domain-like receptor protein 3 (NLRP3) inflammosomes by activating TTP, thereby promoting the inflammatory response (67). Li et al. showed that HUC- MSCs can reduce the production of NLRP3 inflammasomes by inhibiting the MK2/TTP signalling pathway (68). At the same time, MSCs can reduce spinal neuronal apoptosis by suppressing the expression levels of pro-caspase-1 by inhibiting the MK2/TTP signalling pathway. Mohamadi et al. also demonstrated that MSCs transplantation can reduce the expression of NLRP1 inflammasome components (NLRP1, and caspase-1) and pro-inflammatory factors (IL-1β, IL-18 and TNF-α) (69).. Furthermore, Huang et al. confirmed that fat-derived MSCs exosomes can target the elimination of NLRP3 at the SCI site and reduce inflammatory cytokine production (70). The reduction of peri-SCI inflammation by MSCs-EVs has been widely demonstrated (51).
The nuclear factor erythroid 2 (NFE2)-related factor 2 (Nrf2) signalling pathway is involved in antioxidative stress. Nrf2 can counteract the NF-κB-driven inflammatory response, endoplasmic reticulum stress and autophagy damage, and is an important factor in oxidative stress in the body (71). Keap1 is an endogenous inhibitor of Nrf2, and under basal conditions, Nrf2 is degraded by cytoplasmic Keap1 chelation combined with targeted proteasomes (72). MSCs are able to block the expression of Keap1 and reduce oxidative stress caused by SCI. miR-200a is a small interfering RNA, miR-200a targets the Keap1 3′-untranslated region (3′-UTR), resulting in degradation of the mRNA encoding Keap1 (73). Wang et al. found that the miR-200a released by MSCs can target Keap1 and inhibit the expression of Keap1, thereby improving the level of oxidative stress in SCI (74).
3.2 Promotion of axon regeneration
The goal of treatment after SCI is to repair the damaged nerve cells and restore patient nerve function. Neuronal destruction and loss of nerve cells after SCI are major factors in neurological dysfunction. Although the differentiation of MSCs into neurons in vivo remains controversial, BMSCs secrete brain-derived neurotrophic factor (BDNF) and β nerve growth factor (β-NGF) to promote neuronal survival and axon regeneration (Figure 2) (75, 76). Studies have found that oligodendrocytes are missing with demyelination and irreversible damage to the central nervous system (77). Muniswami et al. observed that MSCs spontaneously expressed neuromarkers at SCI sites, including β III tubulin, enolase 2, and microtubule associated protein 1b (MAP1b) (78). β III tubulin is the main component of neuronal microtubules and plays a key role in axon orientation, maturation, and maintenance (79). At 14 days after SCI, an increase in the number of viable neurons and oligodendrocytes was detected. Park et al. genetically engineered MSCs to express oligodendrocyte lineage transcription factor 2 (Olig2) (80). Transplantation of engineered MSCs to the site of injury one week after SCI in a rat model allowed recovery of rat neurological function. Zhou et al. confirmed that MSC-EVs can be internalized by neuronal cells, activate MEK/ERK pathway signalling, and enhance tubulin expression (81).
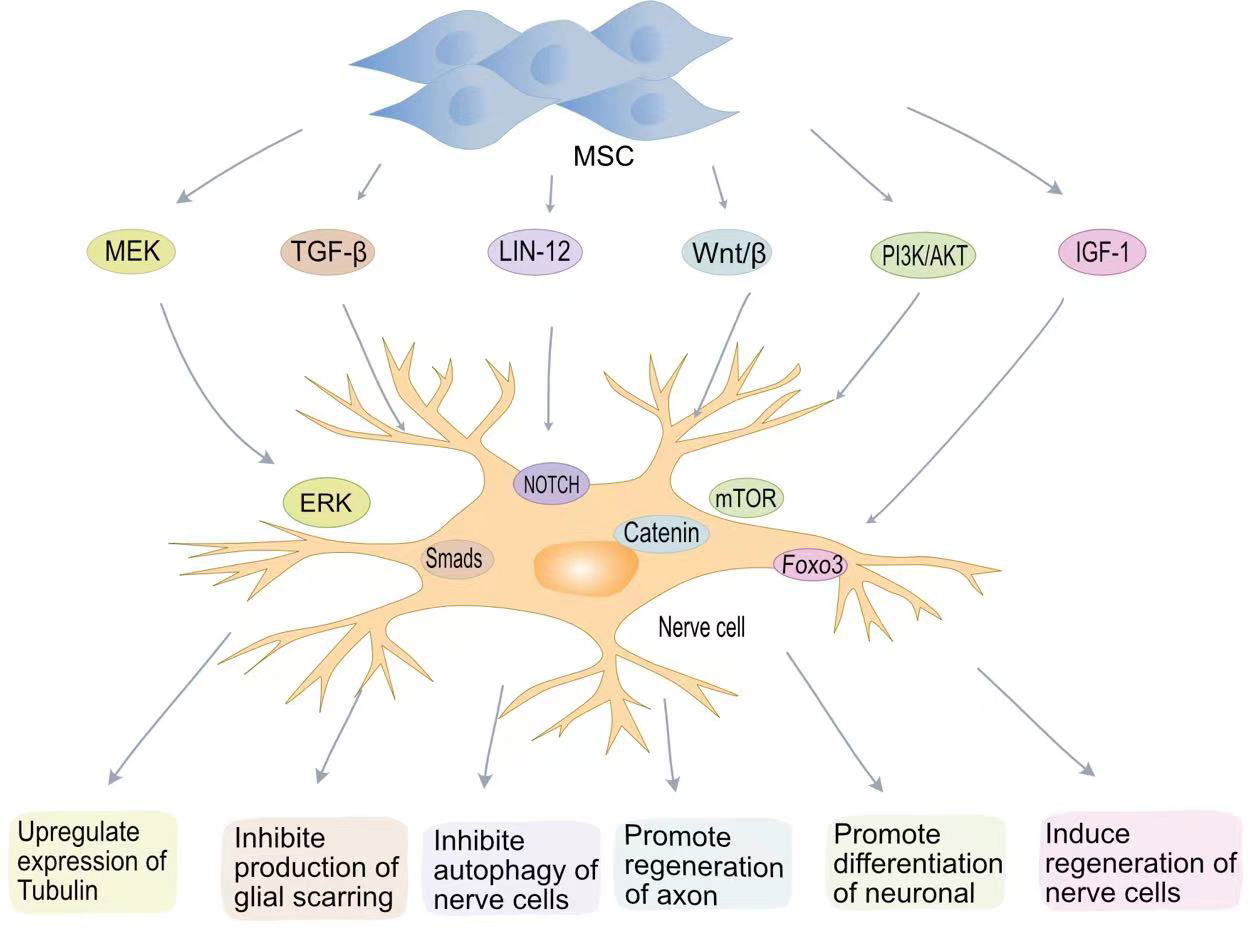
Figure 2 MSCs promote nerve regenerative signaling pathways in SCI. MSCs promote tubulin expression through the MEK-EPR signaling pathway; MSCs inhibit TGF-β to produce glial scarring; MSCs inhibit neurocyte autophagy via the Lin12-Notch pathway; MSCs promote axon regeneration through Wnt/β-catenin; MSCs induce neuronal cell differentiation via the PI3K-mTOR pathway. MSCs induce neural regeneration via the IGF-1-Foxo3 signaling pathway.
The LIN-12/NOTCH signalling pathway is a key pathway controlling the proliferation and differentiation of neural stem cells (82). Autophagy is a conserved cellular process in cellular evolution that allows the degradation of damaged cytoplastic materials and organelles. After nerve cells damage, LIN-12 restricts nerve axon regeneration through autophagy (83, 84). Chen et al. found that MSCs can inhibit the NOTCH signalling pathway and promote the differentiation of neurons (85). The Wnt/β-catenin pathway can also promote neuronal differentiation (86). The Wnt family is a class of glycoproteins widely involved in neurodevelopment, axon guidance, cell proliferation, and nerve cells survival (87). Previous studies have shown that activation of the Wnt/β-catenin signalling pathway plays a key role in functional recovery and axon regeneration after SCI (88). Yin et al. found that MSCS transplantation can enhance axon regeneration after SCI by enhancing the expression of Wnt3a protein (89).
Phosphatidylinositol 3-kinase (PI3K) is a lipid kinase involved in various cellular functions, including cell proliferation, growth, differentiation, migration, and survival (90). The mTOR protein encoded by the mammalian target of rapamycin (mTOR) gene belongs to the family of serine-threonine kinases that control cellular responses to stressors such as growth factors, nutrient deprivation, and DNA damage (91). The PI3K/AKT/mTOR signalling pathway is one of the main pathways by which mammals regulate growth (91). The PI3K/AKT/mTOR signalling pathway is associated with cancer development (92). In vitro experiments have confirmed that blocking the PI3K/AKT/mTOR pathway aggravates neuronal damage (36). BMSCs transplantation effectively restores motor function after SCI by activating the PI3K/AKT/mTOR pathway (93). Fan et al. developed a hydrogel loaded with BMSCs-exosomes (36). The miRNA contained in BMSC-exosomes significantly reduces mRNA expression levels in iNOS, IL-6 and TNF-α and promotes macrophage M2 polarization through the NF-κB pathway. BMSC-exosomes also promote neuronal differentiation and axon regeneration by activating the PI3K/AKT/mTOR pathway.
Insulin-like growth factor 1 (IGF-1) is a protein hormone that is an important growth factor involved in the development of the central nervous system and in promoting recovery after injury or pathological processes (94). BMSCs can secrete IGF-1, and the increase in IGF-1 can maintain the BMSC survival. IGF-1 suppresses oxidative stress at the SCI site, decreasing harmful substances such as ROS. Studies have shown that IGF-1 can actively target ROS around the injury site, blocking cellular oxidative stress processes by inhibiting the transcription factor Foxo3 (95). IGF plays an important role in nerve cells regeneration. IGF-1 binds to IGF-1 receptors on neural stem cells to promote neural stem cell recruitment and differentiation (96, 97).
The presence of glial scars can resist the invasion of inflammatory factors to a certain extent, reducing the inflammatory response after SCI. However, the presence of glial scars also hinders axon regeneration and is detrimental to nervous system recovery (98). Astrocytes are the most abundant glial cells in the central nervous system and play an important role in regulating blood flow, maintaining integrity, and maintaining neuronal homeostasis (99). After SCI, activated microglia transform astrocytes into a neurotoxic state. Chondroitin sulphate proteoglycans (CSPGs) released by astrocytes are strongly associated with glial scar formation (100). Due to the presence of glial scars, the regenerated axons cannot penetrate the colloidal scar structure and therefore cannot build neural networks. MSC-EVs inhibit the activation of astrocytes and reduce apoptosis in neuronal cells (101). MSCs transplanted into SCI rats inhibited the formation of glial scarring and also changed the morphology of astrocytes, providing a supportive microenvironment for axon regeneration and functional recovery (102). MSCs can also inhibit glial scarring by lowering cytokine levels. Studies have shown that TGF-β can mediate glial scarring by activating Smads signalling in astrocytes (103). Lev et al. showed that BMSCs can reduce glial scarring and promote axon regeneration by inhibiting the TGF-β/Smads signalling pathway (104).
3.3 Promotion of vascular repair
Traumatic SCI usually results in the direct destruction of the blood vessels around the spinal cord. Destruction of the blood vessels results in ischemic necrosis, and secondary injury leads to cascade enlargement (105). Vascular recovery contributes to the recovery of motor function in patients with SCI, so promoting SCI vascular recovery is a new target for the treatment of SCI (106). Menezes et al. demonstrated that fat-derived MSCs secrete a large number of angiogenic factors such as vascular endothelial growth factor (VEGF), fibroblast growth factor (FGF), platelet-derived growth factor (PDGF), and IGF-1 (107). These angiogenic factors can promote pericyte recruitment, which is considered a critical step in vascular maturation (108). The migration of MSCs to the SCI site also induces endogenous cell differentiation into pericyte, promoting new angiogenesis (108). Zhang et al. confirmed that post-SCI vascular endothelial cells can absorb MSC-EVs, while normal vascular endothelial cells cannot (109). Although the underlying mechanism is remains unclear, necrotic vascular endothelial cells may be in a specific state conducive to receiving MSC-EVs. The authors reported that 57% of the vascular endothelial cells around the mouse SCI showed a vascular regeneration effect after receiving MSC-EVs and an extensive vascular network was formed around the injury over 28 days (109). Recovery of the vascular endothelium also engulfs myelin debris and inhibits fibrotic scar formation (110).
MMP released after SCI damages the BSCB by degrading the extracellular matrix (ECM) (111). MMP causes destruction of the BSCB, and penetration of inflammatory factors and neurotoxic substances aggravates secondary damage (112). Although peripheral astrocytes can rely on the glucose-1 transporter (Glut-1) to cause new angiogenesis, this weak vascular regeneration is not sufficient to reconstruct the damaged vascular system (112). Wang et al. confirmed that MSC-EVs can maintain the integrity of the BSCB by inhibiting the expression of MMP and promoting the expression of tight junction proteins and adhesion junctions (18). Cao et al. used urine-derived MSC-EVs as a vehicle to deliver angiogenesis-related proteins to the SCI site (113). EVs produced by MSCs through the paracrine pathway have been shown to promote post-SCI angiogenesis and SCI recovery.
In summary, MSCs mainly repair SCI through anti-inflammatory effects and by promoting nerve axon regeneration and vascular regeneration. MSCs promote SCI recovery by modulating multiple pathways. Although the mechanism underlying the effect of MSCs is still not fully understood, the role of MSCs has been demonstrated.
4 Clinical application of MSCs in the treatment of SCI
MSCs have been shown to promote SCI recovery in clinical trials. The effectiveness of MSCs on SCI recovery is influenced by a variety of conditions: mode of transplantation, dose and frequency of MSCs, timing of SCI, and type of SCI (114). At present, the commonly used methods of MSC transplantation are subarachnoid space transplantation, intravenous injection, and local injection into the injured area (115). Intravenous MSCs are prone to pulmonary embolism, and intrathecal MSCs require larger doses because the arachnoid membrane adsorbs a large number of stem cells, which is not conducive to stem cell migration (116). Transplantation (intramedullary injection) can deliver MSCs directly to the site of injury but there is a risk of increased tissue pressure and damage to the normal spinal cord. Most clinical studies have reported delivery of MSCs via intrathecal injection and orthotopic injection (117). Yoon et al. reported that transplantation in the acute and subacute phases could improve neurological function in 33.3% patients with SCI improved from American Spinal Injury Association (ASIA) Impairment Scale score (AIS score) A to B or C), while transplantation in the chronic phase (5.2% of patients improved from AIS score A to B or C) did not result in a significant improvement of the outcomes (118). This may be due to the fact that during the chronic phase of SCI, the formation of glial scarring acts as a physical barrier that interferes with axon regeneration (116). Although Yoon et al. demonstrated that MSC transplantation in the acute phase can promote the recovery of extension function in patients with SCI, transplantation of MSCs in the acute phase creates a cytotoxic environment for implanted stem cells due to the presence of ROS, excitatory transmitters, and inflammatory molecules (119). Transplantation in the subacute phase of SCI appears to be the optimal time for transplantation (120). In the subacute phase, the level of inflammatory factors at the SCI site is lower than that in the acute phase and glial scar formation has not yet occurred. Dai et al. injected autologous BMSCs into a chronic SCI site (121). After 6 months, the ASIA sensory and motor scores were tested, and improvement in ASIA sensory (5.4 ± 8.22) and motor scores (0.9 ± 1.07) was observed in 10 of the 20 patients who underwent MSC transplantation. There was no significant improvement in ASIA sensory (0.25 ± 0.44) and motor scores (0.10 ± 0.31) in the control group. However, this improvement in neurological function may be explained by the large number of stem cells transplanted (122–124). In the current study, the dosage of stem cells transplanted was mainly between 1×106-5×108 cells/kg (41).
The promotion of sensory, motor, and neurological recovery by MSCs following SCI has been widely demonstrated (Table 1) (125–131). Evidence indicates that treatment with MSCs did not cause significant adverse effects (134). Cofano et al. reviewed the clinical treatment of bone marrow mesenchymal stem cells and found no significant adverse effects of stem cell therapy (41). Clinical studies have reported the development of mild fever, gastrointestinal dysfunction, headache, and urinary tract infection in only small numbers of patients after MSCs transplantation (9, 120). Nirmeen et al. reported that of 43 patients with SCI undergoing MSC transplantation, 24 patients developed neuropathic pain within 3 days of transplantation (123). This finding may be related to the frequency of MSC transplantation as the 43 patients received MSCs once a month, while in other studies most patients underwent MSC transplantation only once. There have been no reports on the relationship between MSCs transplantation frequency and adverse effects. Although MSC transplantation appears to improve function in patients with SCI, the effects are not yet clear. Karamouzian et al. reported that only 45.5% of patients in the subacute phase after SCI showed improvement in neurological function after autologous BMC transplantation (120). Park et al. reported that only 3 out of 10 patients with SCI experienced improvements in daily functioning following MSC transplantation (132). Vaquero et al. reported that 44.4% of patients experienced improvement in infralesional muscle reinnervation following MSC administration (133). At present, the effective rate of MSCs in the treatment of SCI does not support the widespread use of MSCs in clinical treatment. Therefore, further research is needed to optimize the effectiveness of MSC treatment. There are many ongoing clinical trials on the use of MSCs for the treatment of SCI (Table 2). In these clinical trials, most MSCs were transplanted at doses of 1×106/kg or 10-100 million per dose. The types of MSCs used in registered clinical trials continued to be BMSCs, AD-MSCs and UC-MSCs. Outcomes observed included ASIA sensory and motor scores, adverse events, and neurologically associated sensory and motor movements. Some trials are at the stage of patient recruitment while others are completed and the publication of data is currently awaited. It is anticipated that these results will provide guidance on the safety and efficacy of MSCs in the treatment of SCI.
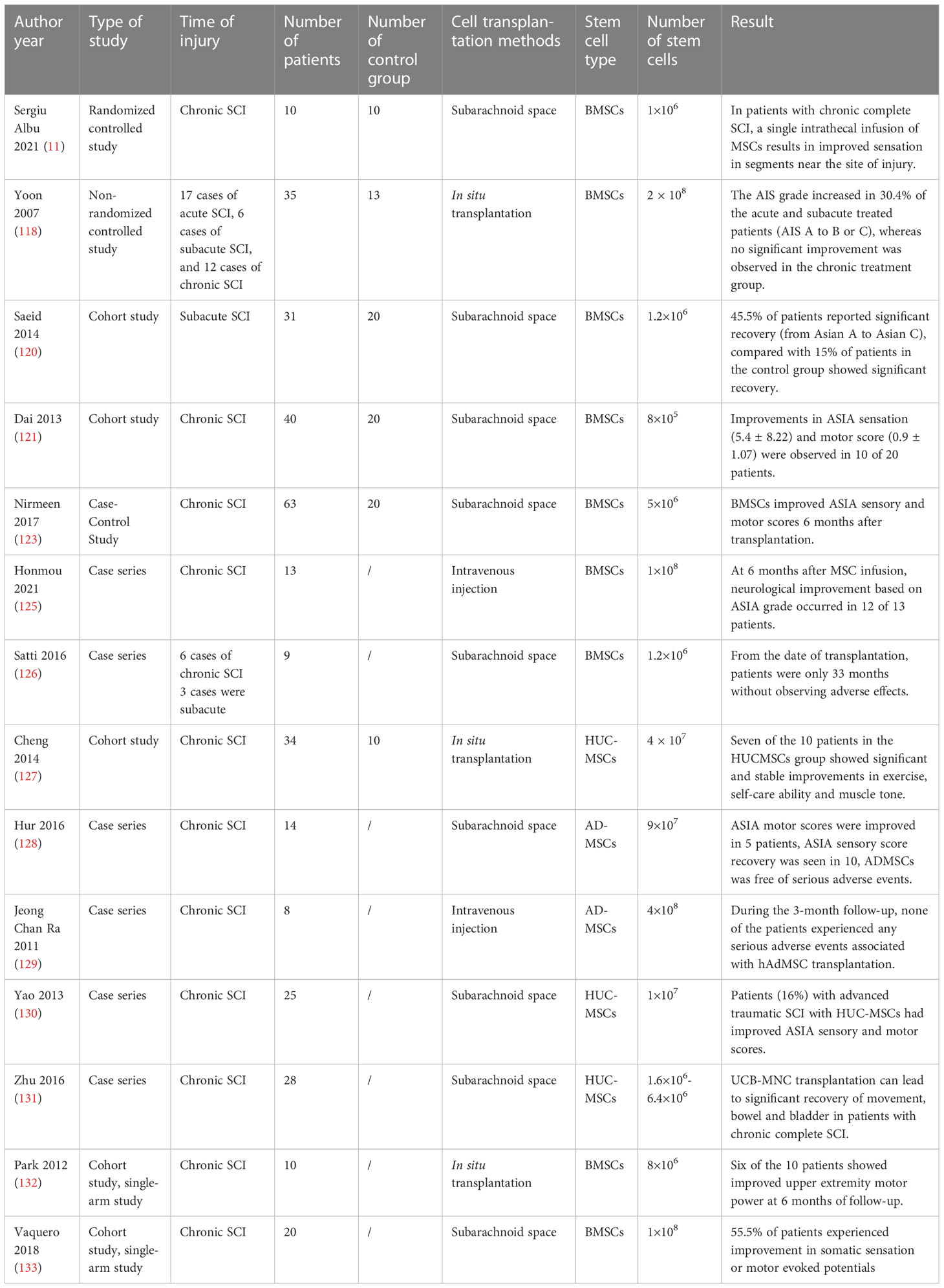
Table 1 Clinical application of mesenchymal stem cells in the treatment of patients with spinal cord injury.
MSCs have the potential for multi-lineage differentiation, easy isolation and preservation, and rapid proliferation and homing to lesions, which have been applied to the treatment of SCI (41). MSCs from different sources have different intrinsic properties. The use of BMSCs, HUC-MSCs, and AD-MSCs has been reported in the treatment of SCI (Table 1). A meta-analysis by Chen et al. showed that autologous BMSCs transplantation significantly improved ASIA motor [MD = 8.01, 95% CI (4.27, 11.76)] and sensory score [MD = 17.98, 95% CI (10.04, 25.91)] (135). Liu et al. analysed 12 studies up to January 30, 2021 with a total of 642 patients and compared BMSCs with UC-MSCs for the treatment of SCI (136). Compared to UC-MSCs, BMSCs were associated with greater improvement in ASIA exercise scores (weighted mean difference [WMD], 6.67; 95% CI, 0.83–12.73) and ASIA sensory scores (WMD, 12.41; 95% CI, 3.42–21.72). This finding may be explained by the greater ability of BMSCs to secrete neuronal phenotypic markers to induce axon regeneration compared to MSCs from other sources (137).
5 Challenges in the clinical application of MSC therapy
MSCs have immunomodulatory abilities and play an important role in the regulation of immune responses and the development of many diseases. Studies have shown that mesenchymal stem cells are involved in the initiation, progression, and metastasis of cancer (138). The suppression of the immune system by MSCs is conducive to immune escape by tumour cells. Although MSCs have tumour homing properties, they can be used as vectors for antitumor drug delivery (139). However, the overall effect of MSCs on tumours is to promote tumour growth more than to inhibit tumour growth (138, 140). In patients treated with stem cells, the patient spontaneously developed gliomas after four years (141). Although there are few reports of tumorigenesis, this remains an aspect of MSC therapy that cannot be ignored. Although MSCs are beneficial to the recovery of neurological function in patients with SCI, the mechanism of action of MSCs and the cellular mechanisms that prevent the recovery of neural circuits after SCI remain unclear (41). Future research needs to focus on understanding the SCI cellular mechanisms and MSC action for use in clinical practice.
The fate of transplanted cells depends primarily on the surrounding environment rather than on the properties of the cells themselves (142). Inflammatory factors and toxic substances in the SCI microenvironment are not conducive to the survival of MSCs. Dead MSCs release toxic substances that exacerbate SCI damage (143). The survival rate and long-term survival of MSCs in hostile environments remain unresolved issues (144). Gels and scaffolds based on stem cell therapy can deliver MSCs to the site of injury and release MSCs slowly and continuously to the site of injury (145–149). Tissue engineering strategies can reduce the number of times that MSCs are administered to the patient and reduce the mechanical damage at the site of puncture. Various types of scaffolds and gels of natural and synthetic biomaterials have been developed to mimic the stem cell microenvironment to maintain stem cell survival (146). The ideal biomaterial should have good biocompatibility and low immunogenicity while being biodegradable. When transplanted to the site of injury, the biomaterial should have ideal mechanical properties for cell adhesion and axon regeneration (150). MSCs with neurotrophic factor co-delivery strategies appear to be more effective therapeutic options (151). However, tissue engineering is currently being applied only in the rat SCI injury model, and its application to clinical practice will require further research. We consider that tissue engineering strategies will address the challenges of delivering MSCs for the treatment of SCI in the future.
6 Conclusion
The amplification of the inflammatory cascade after SCI often causes permanent harm to the patient. Toxic substances and inflammatory factors not only aggravate SCI, but also cause irreparable damage to the nervous system. The role of MSCs in the treatment of SCI is promising. MSCs achieve immunosuppression through direct contact with immune cells or paracrine release signalling molecules to reduce the inflammatory response at SCI. MSCs also release neurotrophic factors such as BDNF and β-NGF to promote axon regeneration. Furthermore, MSCs regulate signalling pathways to inhibit glial scarring. Obstruction of glial scarring facilitates axon regeneration. In addition, MSCs can release angiogenic factors such as VEGF, FGF, PDGF and IGF-1 to promote perispinal angiogenesis and reshape BSCB. MSCs have been shown to be beneficial in SCI mice for motor function recovery in preclinical studies. In clinical practice, MSCs have also been used in the treatment of patients with SCI. The ability of MSCs to improve ASIA sensory and motor scores and promote bladder function recovery and neurological symptom recovery in patients with SCI has been widely demonstrated. After treatment with MSCs, patients mild fever, gastrointestinal dysfunction, headache, and urinary tract infections have been reported, although these did not cause serious adverse reactions. Although MSCs can improve ASIA sensory and motor scores in patients, the effectiveness of MSC therapy reported in the literature is not sufficient to support the widespread clinical use of MSCs and needs to be further improved. In addition, there are many unanswered questions about MSCs in the treatment of SCI. The cell dose and frequency of doses required, the mechanism of action of MSCs, and the cellular processes that prevent neural circuit recovery after SCI remain unclear. Furthermore, the toxic environment associated with SCI is not conducive to MSC survival. Although further clinical studies are required to elucidate these points, the evidence currently available indicates that MSCs are likely to play a major role in the treatment of SCI in the future.
Author contributions
All authors read and approved the final manuscript. YL wrote the initial manuscript. CF contributed new ideas. JS created the figures. RH, YH and HY revised the manuscript and approved the final version.
Funding
This work was supported by the National Natural Science Foundation of China (Grant Nos. 82071391)
Acknowledgments
We would like to express our appreciation to everyone who was involved in the drafting and preparation of the manuscript. We would like to thank Editage (www.editage.cn) for English language editing.
Conflict of interest
The authors declare that the research was conducted in the absence of any commercial or financial relationships that could be construed as a potential conflict of interest.
Publisher’s note
All claims expressed in this article are solely those of the authors and do not necessarily represent those of their affiliated organizations, or those of the publisher, the editors and the reviewers. Any product that may be evaluated in this article, or claim that may be made by its manufacturer, is not guaranteed or endorsed by the publisher.
References
1. Liu X, Zhang Y, Wang Y, Qian T. Inflammatory response to spinal cord injury and its treatment. World Neurosurg (2021) 155:19–31. doi: 10.1016/j.wneu.2021.07.148
2. Wang H, Xia Y, Li B, Li Y, Fu C. Reverse adverse immune microenvironments by biomaterials enhance the repair of spinal cord injury. Front Bioengineering Biotechnol (2022) 10:812340. doi: 10.3389/fbioe.2022.812340
3. Taylor EC, Fitzpatrick CE, Thompson SE, Justice SB. Acute traumatic spinal cord injury. Adv Emergency Nurs J (2022) 44:272–80. doi: 10.1097/TME.0000000000000428
4. Zipser CM, Cragg JJ, Guest JD, Fehlings MG, Jutzeler CR, Anderson AJ, et al. Cell-based and stem-cell-based treatments for spinal cord injury: evidence from clinical trials. Lancet Neurol (2022) 21:659–70. doi: 10.1016/S1474-4422(21)00464-6
5. Xia Y, Yang R, Hou Y, Wang H, Li Y, Zhu J, et al. Application of mesenchymal stem cell-derived exosomes from different sources in intervertebral disc degeneration. Front Bioengineering Biotechnol (2022) 10:1019437. doi: 10.3389/fbioe.2022.1019437
6. Kim GU, Sung SE, Kang KK, Choi JH, Lee S, Sung M, et al. Therapeutic potential of mesenchymal stem cells (MSCs) and MSC-derived extracellular vesicles for the treatment of spinal cord injury. Int J Mol Sci (2021) 22(24):13672. doi: 10.3390/ijms222413672
7. Shao A, Tu S, Lu J, Zhang J. Crosstalk between stem cell and spinal cord injury: pathophysiology and treatment strategies. Stem Cell Res Ther (2019) 10:238. doi: 10.1186/s13287-019-1357-z
8. Mukhamedshina YO, Gracheva OA, Mukhutdinova DM, Chelyshev YA, Rizvanov AA. Mesenchymal stem cells and the neuronal microenvironment in the area of spinal cord injury. Neural Regeneration Res (2019) 14(2):227–237. doi: 10.4103/1673-5374.244778
9. Saini R, Pahwa B, Agrawal D, Singh PK, Gujjar H, Mishra S, et al. Efficacy and outcome of bone marrow derived stem cells transplanted via intramedullary route in acute complete spinal cord injury - a randomized placebo controlled trial. J Clin Neurosci (2022) 100:7–14. doi: 10.1016/j.jocn.2022.03.033
10. Song H, Suo SQ, Ning C, Zhang Y, Mu WD, Chen S. Bone marrow mesenchymal stem cells transplantation on acute spinal cord injury. J Hard Tissue Biol (2020) 29:91–8. doi: 10.2485/jhtb.29.91
11. Albu S, Kumru H, Coll R, Vives J, Valles M, Benito-Penalva J, et al. Clinical effects of intrathecal administration of expanded Wharton jelly mesenchymal stromal cells in patients with chronic complete spinal cord injury: a randomized controlled study. Cytotherapy (2021) 23:146–56. doi: 10.1016/j.jcyt.2020.08.008
12. Ding DC, Shyu WC, Lin SZ. Mesenchymal stem cells. Cell Transplant (2011) 20:5–14. doi: 10.3727/096368910X
13. Raghav PK, Mann Z, Ahlawat S, Mohanty S. Mesenchymal stem cell-based nanoparticles and scaffolds in regenerative medicine. Eur J Pharmacol (2022) 918:174657. doi: 10.1016/j.ejphar.2021.174657
14. Hamid R, Averbeck MA, Chiang H, Garcia A, Al Mousa RT, Oh S-J, et al. Epidemiology and pathophysiology of neurogenic bladder after spinal cord injury. World J Urol (2018) 36:1517–27. doi: 10.1007/s00345-018-2301-z
15. Chio JCT, Punjani N, Hejrati N, Zavvarian M-M, Hong J, Fehlings MG. Extracellular matrix and oxidative stress following traumatic spinal cord injury: Physiological and pathophysiological roles and opportunities for therapeutic intervention. Antioxid Redox Signaling (2021) 37:184–207. doi: 10.1089/ars.2021.0120
16. Pang QM, Chen SY, Fu SP, Zhou H, Zhang Q, Ao J, et al. Regulatory role of mesenchymal stem cells on secondary inflammation in spinal cord injury. J Inflammation Res (2022) 15:573–93. doi: 10.2147/JIR.S349572
17. He X, Li Y, Deng B, Lin A, Zhang G, Ma M, et al. The PI3K/AKT signalling pathway in inflammation, cell death and glial scar formation after traumatic spinal cord injury: Mechanisms and therapeutic opportunities. Cell Proliferation (2022) 55:e13275. doi: 10.1111/cpr.13275
18. Xin W, Qiang S, Jianing D, Jiaming L, Fangqi L, Bin C, et al. Human bone marrow mesenchymal stem cell–derived exosomes attenuate blood–spinal cord barrier disruption via the TIMP2/MMP pathway after acute spinal cord injury. Mol Neurobiol (2021) 58:6490–504. doi: 10.1007/s12035-021-02565-w
19. Lima R, Monteiro A, Salgado AJ, Monteiro S, Silva NA. Pathophysiology and therapeutic approaches for spinal cord injury. Int J Mol Sci (2022) 23(22):13833. doi: 10.3390/ijms232213833
20. Garcia E, Aguilar-Cevallos J, Silva-Garcia R, Ibarra A. Cytokine and growth factor activation In vivo and In vitro after spinal cord injury. Mediators Inflammation (2016) 2016:9476020. doi: 10.1155/2016/9476020
21. Feng D, Yu J, Bao L, Fan D, Zhang B. Inhibiting RGS1 attenuates secondary inflammation response and tissue degradation via the TLR/TRIF/NF-κB pathway in macrophage post spinal cord injury. Neurosci Lett (2022) 768:136374. doi: 10.1016/j.neulet.2021.136374
22. Zivkovic S, Ayazi M, Hammel G, Ren Y. For better or for worse: A look into neutrophils in traumatic spinal cord injury. Front Cell Neurosci (2021) 15:648076. doi: 10.3389/fncel.2021.648076
23. Trivedi A, Olivas AD, Noble-Haeusslein LJ. Inflammation and spinal cord injury: Infiltrating leukocytes as determinants of injury and repair processes. Clin Neurosci Res (2006) 6:283–92. doi: 10.1016/j.cnr.2006.09.007
24. Fleming JC, Norenberg MD, Ramsay DA, Dekaban GA, Marcillo AE, Saenz AD, et al. The cellular inflammatory response in human spinal cords after injury. Brain J Neurol (2006) 129:3249–69. doi: 10.1093/brain/awl296
25. Sroga JM, Jones TB, Kigerl KA, McGaughy VM, Popovich PG. Rats and mice exhibit distinct inflammatory reactions after spinal cord injury. J Comp Neurol (2003) 462:223–40. doi: 10.1002/cne.10736
26. Xu L, Wang J, Ding Y, Wang L, Zhu YJ. Current knowledge of microglia in traumatic spinal cord injury. Front Neurol (2021) 12:796704. doi: 10.3389/fneur.2021.796704
27. Bellver-Landete V, Bretheau F, Mailhot B, Vallières N, Lessard M, Janelle ME, et al. Microglia are an essential component of the neuroprotective scar that forms after spinal cord injury. Nat Commun (2019) 10:518. doi: 10.1038/s41467-019-08446-0
28. Kohno K, Shirasaka R, Yoshihara K, Mikuriya S, Tanaka K, Takanami K, et al. A spinal microglia population involved in remitting and relapsing neuropathic pain. Sci (New York NY) (2022) 376:86–90. doi: 10.1126/science.abf6805
29. Duncan GJ, Manesh SB, Hilton BJ, Assinck P, Plemel JR, Tetzlaff W. The fate and function of oligodendrocyte progenitor cells after traumatic spinal cord injury. Glia (2020) 68:227–45. doi: 10.1002/glia.23706
30. Li Y, He X, Kawaguchi R, Zhang Y, Wang Q, Monavarfeshani A, et al. Microglia-organized scar-free spinal cord repair in neonatal mice. Nature (2020) 587:613–8. doi: 10.1038/s41586-020-2795-6
31. Brennan FH, Li Y, Wang C, Ma A, Guo Q, Li Y, et al. Microglia coordinate cellular interactions during spinal cord repair in mice. Nat Commun (2022) 13:4096. doi: 10.1038/s41467-022-31797-0
32. Chen J, Chen YQ, Shi YJ, Ding SQ, Shen L, Wang R, et al. VX-765 reduces neuroinflammation after spinal cord injury in mice. Neural Regener Res (2021) 16:1836–47. doi: 10.4103/1673-5374.306096
33. Jeong JW, Jin CY, Kim GY, Lee JD, Park C, Kim GD, et al. Anti-inflammatory effects of cordycepin via suppression of inflammatory mediators in BV2 microglial cells. Int Immunopharmacol (2010) 10:1580–6. doi: 10.1016/j.intimp.2010.09.011
34. Hou C, Mei Q, Song X, Bao Q, Li X, Wang D, et al. Mono-macrophage-Derived MANF protects against lipopolysaccharide-induced acute kidney injury via inhibiting inflammation and renal M1 macrophages. Inflammation (2021) 44:693–703. doi: 10.1007/s10753-020-01368-w
35. Anwar MA, Al Shehabi TS, Eid AH. Inflammogenesis of secondary spinal cord injury. Front Cell Neurosci (2016) 10:98. doi: 10.3389/fncel.2016.00098
36. Fan L, Liu C, Chen X, Zheng L, Zou Y, Wen H, et al. Exosomes-loaded electroconductive hydrogel synergistically promotes tissue repair after spinal cord injury via immunoregulation and enhancement of myelinated axon growth. Adv Sci (Weinheim Baden-Wurttemberg Germany) (2022) 9:e2105586. doi: 10.1002/advs.202105586
37. Liu J, Gao J, Liang Z, Gao C, Niu Q, Wu F, et al. Mesenchymal stem cells and their microenvironment. Stem Cell Res Ther (2022) 13:429. doi: 10.1186/s13287-022-02985-y
38. Alió Del Barrio JL, de la Mata A, De Miguel MP, Arnalich-Montiel F, Nieto-Miguel T, El Zarif M, et al. Corneal regeneration using adipose-derived mesenchymal stem cells. Cells (2022) 11(16):2549. doi: 10.3390/cells11162549
39. Costela-Ruiz VJ, Melguizo-Rodríguez L, Bellotti C, Illescas-Montes R, Stanco D, Arciola CR, et al. Different sources of mesenchymal stem cells for tissue regeneration: A guide to identifying the most favorable one in orthopedics and dentistry applications. Int J Mol Sci (2022) 23(11):6356. doi: 10.3390/ijms23116356
40. Jarocha D, Milczarek O, Wedrychowicz A, Kwiatkowski S, Majka M. Continuous improvement after multiple mesenchymal stem cell transplantations in a patient with complete spinal cord injury. Cell Transplant (2015) 24:661–72. doi: 10.3727/096368915X687796
41. Cofano F, Boido M, Monticelli M, Zenga F, Ducati A, Vercelli A, et al. Mesenchymal stem cells for spinal cord injury: Current options, limitations, and future of cell therapy. Int J Mol Sci (2019) 20(11):2698. doi: 10.3390/ijms20112698
42. Gao L, Peng Y, Xu W, He P, Li T, Lu X, et al. Progress in stem cell therapy for spinal cord injury. Stem Cells Int (2020) 2020:2853650. doi: 10.1155/2020/2853650
43. Luo H, Xu C, Liu Z, Yang L, Hong Y, Liu G, et al. Neural differentiation of bone marrow mesenchymal stem cells with human brain-derived neurotrophic factor gene-modified in functionalized self-assembling peptide hydrogel in vitro. J Cell Biochem (2019) 120:2828–35. doi: 10.1002/jcb.26408
44. Kokai LE, Marra K, Rubin JP. Adipose stem cells: biology and clinical applications for tissue repair and regeneration. Trans Res J Lab Clin Med (2014) 163:399–408. doi: 10.1016/j.trsl.2013.11.009
45. Reyhani S, Abbaspanah B, Mousavi SH. Umbilical cord-derived mesenchymal stem cells in neurodegenerative disorders: from literature to clinical practice. Regenerative Med (2020) 15:1561–78. doi: 10.2217/rme-2019-0119
46. Krupa P, Vackova I, Ruzicka J, Zaviskova K, Dubisova J, Koci Z, et al. The effect of human mesenchymal stem cells derived from wharton’s jelly in spinal cord injury treatment is dose-dependent and can be facilitated by repeated application. Int J Mol Sci (2018) 19(5):1503. doi: 10.3390/ijms19051503
47. Wang Y, Fang J, Liu B, Shao C, Shi Y. Reciprocal regulation of mesenchymal stem cells and immune responses. Cell Stem Cell (2022) 29:1515–30. doi: 10.1016/j.stem.2022.10.001
48. Ankrum JA, Ong JF, Karp JM. Mesenchymal stem cells: immune evasive, not immune privileged. Nat Biotechnol (2014) 32:252–60. doi: 10.1038/nbt.2816
49. Hausmann ON. Post-traumatic inflammation following spinal cord injury. Spinal Cord (2003) 41:369–78. doi: 10.1038/sj.sc.3101483
50. Chang W-H, Cerione RA, Antonyak MA. Extracellular vesicles and their roles in cancer progression. In: Robles-Flores M, editor. Cancer cell signaling: Methods and protocols. New York, NY: Springer US (2021). p. 143–70.
51. Zhang M, Wang L, Huang S, He X. Exosomes with high level of miR-181c from bone marrow-derived mesenchymal stem cells inhibit inflammation and apoptosis to alleviate spinal cord injury. J Mol Histol (2021) 52:301–11. doi: 10.1007/s10735-020-09950-0
52. Pang Q-M, Chen S-Y, Xu Q-J, Fu S-P, Yang Y-C, Zou W-H, et al. Neuroinflammation and scarring after spinal cord injury: Therapeutic roles of MSCs on inflammation and glial scar. Front Immunol. (2021) 12:751021. doi: 10.3389/fimmu.2021.751021
53. Kasuya Y, Umezawa H, Hatano M. Stress-activated protein kinases in spinal cord injury: Focus on roles of p38. Int J Mol Sci (2018) 19(3):867. doi: 10.3390/ijms19030867
54. Kang YJ, Chen J, Otsuka M, Mols J, Ren S, Wang Y, et al. Macrophage deletion of p38α partially impairs lipopolysaccharide-induced cellular Activation1. J Immunol (2008) 180:5075–82. doi: 10.4049/jimmunol.180.7.5075
55. Nagaleekar VK, Sabio G, Aktan I, Chant A, Howe IW, Thornton TM, et al. Translational control of NKT cell cytokine production by p38 MAPK. J Immunol (2011) 186:4140–6. doi: 10.4049/jimmunol.1002614
56. Li J, Huang J, Chen L, Ren W, Cai W. Human umbilical cord mesenchymal stem cells contribute to the reconstruction of bladder function after acute spinal cord injury via p38 mitogen-activated protein kinase/nuclear factor-kappa b pathway. Bioengineered (2022) 13:4844–56. doi: 10.1080/21655979.2022.2036397
57. Nie H, Jiang Z. Bone mesenchymal stem cell-derived extracellular vesicles deliver microRNA-23b to alleviate spinal cord injury by targeting toll-like receptor TLR4 and inhibiting NF-κB pathway activation. Bioengineered (2021) 12:8157–72. doi: 10.1080/21655979.2021.1977562
58. Fan L, Dong J, He X, Zhang C, Zhang T. Bone marrow mesenchymal stem cells-derived exosomes reduce apoptosis and inflammatory response during spinal cord injury by inhibiting the TLR4/MyD88/NF-κB signaling pathway. Hum Exp Toxicol (2021) 40:1612–23. doi: 10.1177/09603271211003311
59. Jiang Z, Zhang J. Mesenchymal stem cell-derived exosomes containing miR-145-5p reduce inflammation in spinal cord injury by regulating the TLR4/NF-κB signaling pathway. Cell Cycle (Georgetown Tex) (2021) 20:993–1009. doi: 10.1080/15384101.2021.1919825
60. Zhao C, Zhou X, Qiu J, Xin D, Li T, Chu X, et al. Exosomes derived from bone marrow mesenchymal stem cells inhibit complement activation in rats with spinal cord injury. Drug Design Dev Ther (2019) 13:3693–704. doi: 10.2147/DDDT.S209636
61. Yu L, Li J. Punicalagin alleviates aged bronchial asthma by inhibiting Th2 differentiation through IL-4/STAT6 and Jagged1/Notch pathways. J Healthc Eng (2022) 2022:1184677. doi: 10.1155/2022/1184677
62. Liu W, Li R, Yin J, Guo S, Chen Y, Fan H, et al. Mesenchymal stem cells alleviate the early brain injury of subarachnoid hemorrhage partly by suppression of Notch1-dependent neuroinflammation: involvement of botch. J Neuroinflamm (2019) 16:8. doi: 10.1186/s12974-019-1396-5
63. Zhou Z, Tian X, Mo B, Xu H, Zhang L, Huang L, et al. Adipose mesenchymal stem cell transplantation alleviates spinal cord injury-induced neuroinflammation partly by suppressing the Jagged1/Notch pathway. Stem Cell Res Ther (2020) 11:212. doi: 10.1186/s13287-020-01724-5
64. Liddelow SA, Guttenplan KA, Clarke LE, Bennett FC, Bohlen CJ, Schirmer L, et al. Neurotoxic reactive astrocytes are induced by activated microglia. Nature (2017) 541:481–7. doi: 10.1038/nature21029
65. Yang J, Xing H, Lu D, Wang J, Li B, Tang J, et al. Role of Jagged1/STAT3 signalling in platinum-resistant ovarian cancer. J Cell Mol Med (2019) 23:4005–18. doi: 10.1111/jcmm.14286
66. Wu W, Li D, Zong Y, Zhu H, Pan D, Xu T, et al. Luteolin inhibits inflammatory responses via p38/MK2/TTP-mediated mRNA stability. Mol (Basel Switzerland) (2013) 18:8083–94. doi: 10.3390/molecules18078083
67. Qin CC, Liu YN, Hu Y, Yang Y, Chen Z. Macrophage inflammatory protein-2 as mediator of inflammation in acute liver injury. World J Gastroenterol (2017) 23:3043–52. doi: 10.3748/wjg.v23.i17.3043
68. Na L, Wang S, Liu T, Zhang L. Ultrashort wave combined with human umbilical cord mesenchymal stem cell (HUC-MSC) transplantation inhibits NLRP3 inflammasome and improves spinal cord injury via MK2/TTP signalling pathway. BioMed Res Int (2020) 2020:3021750. doi: 10.1155/2020/3021750
69. Mohamadi Y, Noori Moghahi SMH, Mousavi M, Borhani-Haghighi M, Abolhassani F, Kashani IR, et al. Intrathecal transplantation of wharton’s jelly mesenchymal stem cells suppresses the NLRP1 inflammasome in the rat model of spinal cord injury. J Chem Neuroanat (2019) 97:1–8. doi: 10.1016/j.jchemneu.2019.01.011
70. Huang J-H, Fu C-H, Xu Y, Yin X-M, Cao Y, Lin F-Y. Extracellular vesicles derived from epidural fat-mesenchymal stem cells attenuate NLRP3 inflammasome activation and improve functional recovery after spinal cord injury. Neurochem Res (2020) 45:760–71. doi: 10.1007/s11064-019-02950-x
71. Bellezza I, Giambanco I, Minelli A, Donato R. Nrf2-Keap1 signaling in oxidative and reductive stress. Biochim Biophys Acta (BBA) Mol Cell Res (2018) 1865:721–33. doi: 10.1016/j.bbamcr.2018.02.010
72. Itoh K, Ishii T, Wakabayashi N, Yamamoto M. Regulatory mechanisms of cellular response to oxidative stress. Free Radical Res (1999) 31:319–24. doi: 10.1080/10715769900300881
73. Eades G, Yang M, Yao Y, Zhang Y, Zhou Q. miR-200a regulates Nrf2 activation by targeting Keap1 mRNA in breast cancer cells*. J Biol Chem (2011) 286:40725–33. doi: 10.1074/jbc.M111.275495
74. Wang X, Ye L, Zhang K, Gao L, Xiao J, Zhang Y. Upregulation of microRNA-200a in bone marrow mesenchymal stem cells enhances the repair of spinal cord injury in rats by reducing oxidative stress and regulating Keap1/Nrf2 pathway. Artif Organs (2020) 44:744–52. doi: 10.1111/aor.13656
75. Luzuriaga J, Polo Y, Pastor-Alonso O, Pardo-Rodríguez B, Larrañaga A, Unda F, et al. Advances and perspectives in dental pulp stem cell based neuroregeneration therapies. Int J Mol Sci (2021) 22(7):3546. doi: 10.3390/ijms22073546
76. Luo Y, Xu T, Liu W, Rong Y, Wang J, Fan J, et al. Exosomes derived from GIT1-overexpressing bone marrow mesenchymal stem cells promote traumatic spinal cord injury recovery in a rat model. Int J Neurosci (2021) 131:170–82. doi: 10.1080/00207454.2020.1734598
77. Ohori Y, Yamamoto S, Nagao M, Sugimori M, Yamamoto N, Nakamura K, et al. Growth factor treatment and genetic manipulation stimulate neurogenesis and oligodendrogenesis by endogenous neural progenitors in the injured adult spinal cord. J Neurosci Off J Soc Neurosci (2006) 26:11948–60. doi: 10.1523/JNEUROSCI.3127-06.2006
78. Muniswami DM, Kanthakumar P, Kanakasabapathy I, Tharion G. Motor recovery after transplantation of bone marrow mesenchymal stem cells in rat models of spinal cord injury. Ann Neurosci (2019) 25:126–40. doi: 10.1159/000487069
80. Park HW, Oh S, Lee KH, Lee BH, Chang MS. Olig2-expressing mesenchymal stem cells enhance functional recovery after contusive spinal cord injury. Int J Stem Cells (2018) 11:177–86. doi: 10.15283/ijsc18071
81. Zhou W, Silva M, Feng C, Zhao S, Liu L, Li S, et al. Exosomes derived from human placental mesenchymal stem cells enhanced the recovery of spinal cord injury by activating endogenous neurogenesis. Stem Cell Res Ther (2021) 12:174. doi: 10.1186/s13287-021-02248-2
82. Roese-Koerner B, Stappert L, Brüstle O. Notch/Hes signaling and miR-9 engage in complex feedback interactions controlling neural progenitor cell proliferation and differentiation. Neurogenesis (2017) 4:e1313647. doi: 10.1080/23262133.2017.1313647
83. El Bejjani R, Hammarlund M. Notch signaling inhibits axon regeneration. Neuron (2012) 73:268–78. doi: 10.1016/j.neuron.2011.11.017
84. Ko SH, Apple EC, Liu Z, Chen L. Age-dependent autophagy induction after injury promotes axon regeneration by limiting NOTCH. Autophagy (2020) 16:2052–68. doi: 10.1080/15548627.2020.1713645
85. Chen Y, Lian XH, Liao LY, Liu YT, Liu SL, Gao Q. Transplantation of bone marrow mesenchymal stem cells alleviates spinal cord injury via inhibiting notch signaling. Eur Rev Med Pharmacol Sci (2019) 23:31–8. doi: 10.26355/eurrev_201908_18625
86. Hirabayashi Y, Itoh Y, Tabata H, Nakajima K, Akiyama T, Masuyama N, et al. The wnt/beta-catenin pathway directs neuronal differentiation of cortical neural precursor cells. Dev (Cambridge England) (2004) 131:2791–801. doi: 10.1242/dev.01165
87. Zhou H, Liu Y, Sun L, Fu M, Zhao Y. Salvianolic acid b activates wnt/β-catenin signaling following spinal cord injury. Exp Ther Med (2020) 19:825–32. doi: 10.3892/etm.2019.8292
88. Lu GB, Niu FW, Zhang YC, Du L, Liang ZY, Gao Y, et al. Methylprednisolone promotes recovery of neurological function after spinal cord injury: association with wnt/β-catenin signaling pathway activation. Neural Regener Res (2016) 11:1816–23. doi: 10.4103/1673-5374.194753
89. Yoon HH, Lee HJ, Min J, Kim JH, Park JH, Kim JH, et al. Optimal ratio of Wnt3a expression in human mesenchymal stem cells promotes axonal regeneration in spinal cord injured rat model. J Korean Neurosurg Soc (2021) 64:705–15. doi: 10.3340/jkns.2021.0003
90. Hoxhaj G, Manning BD. The PI3K–AKT network at the interface of oncogenic signalling and cancer metabolism. Nat Rev Cancer (2020) 20:74–88. doi: 10.1038/s41568-019-0216-7
91. Zhang Y, Kwok-Shing Ng P, Kucherlapati M, Chen F, Liu Y, Tsang YH, et al. A pan-cancer proteogenomic atlas of PI3K/AKT/mTOR pathway alterations. Cancer Cell (2017) 31:820–832.e3. doi: 10.1016/j.ccell.2017.04.013
92. Iksen, Pothongsrisit S, Pongrakhananon V. Targeting the PI3K/AKT/mTOR signaling pathway in lung cancer: An update regarding potential drugs and natural products. Mol (Basel Switzerland) (2021) 26(13):4100. doi: 10.3390/molecules26134100
93. Sun X, Huang LY, Pan HX, Li LJ, Wang L, Pei GQ, et al. Bone marrow mesenchymal stem cells and exercise restore motor function following spinal cord injury by activating PI3K/AKT/mTOR pathway. Neural Regener Res (2023) 18:1067–75. doi: 10.4103/1673-5374.355762
94. Dyer AH, Vahdatpour C, Sanfeliu A, Tropea D. The role of insulin-like growth factor 1 (IGF-1) in brain development, maturation and neuroplasticity. Neuroscience (2016) 325:89–99. doi: 10.1016/j.neuroscience.2016.03.056
95. Dávila D, Torres-Aleman I. Neuronal death by oxidative stress involves activation of FOXO3 through a two-arm pathway that activates stress kinases and attenuates insulin-like growth factor I signaling. Mol Biol Cell (2008) 19:2014–25. doi: 10.1091/mbc.e07-08-0811
96. Yan Y-P, Sailor KA, Vemuganti R, Dempsey RJ. Insulin-like growth factor-1 is an endogenous mediator of focal ischemia-induced neural progenitor proliferation. Eur J Neurosci (2006) 24:45–54. doi: 10.1111/j.1460-9568.2006.04872.x
97. Allahdadi KJ, de Santana TA, Santos GC, Azevedo CM, Mota RA, Nonaka CK, et al. IGF-1 overexpression improves mesenchymal stem cell survival and promotes neurological recovery after spinal cord injury. Stem Cell Res Ther (2019) 10:146. doi: 10.1186/s13287-019-1223-z
98. Lukacova N, Kisucka A, Kiss Bimbova K, Bacova M, Ileninova M, Kuruc T, et al. Glial-neuronal interactions in pathogenesis and treatment of spinal cord injury. Int J Mol Sci (2021) 22(24):13577. doi: 10.20944/preprints202111.0116.v1
99. Hart CG, Karimi-Abdolrezaee S. Recent insights on astrocyte mechanisms in CNS homeostasis, pathology, and repair. J Neurosci Res (2021) 99:2427–62. doi: 10.1002/jnr.24922
100. Fitch MT, Silver J. Glial cell extracellular matrix: boundaries for axon growth in development and regeneration. Cell Tissue Res (1997) 290:379–84. doi: 10.1007/s004410050944
101. Liu W, Wang Y, Gong F, Rong Y, Luo Y, Tang P, et al. Exosomes derived from bone mesenchymal stem cells repair traumatic spinal cord injury by suppressing the activation of A1 neurotoxic reactive astrocytes. J Neurotrauma (2019) 36:469–84. doi: 10.1089/neu.2018.5835
102. Okuda A, Horii-Hayashi N, Sasagawa T, Shimizu T, Shigematsu H, Iwata E, et al. Bone marrow stromal cell sheets may promote axonal regeneration and functional recovery with suppression of glial scar formation after spinal cord transection injury in rats. J Neurosurg Spine (2017) 26:388–95. doi: 10.3171/2016.8.SPINE16250
103. Hellal F, Hurtado A, Ruschel J, Flynn KC, Laskowski CJ, Umlauf M, et al. Microtubule stabilization reduces scarring and causes axon regeneration after spinal cord injury. Sci (New York NY) (2011) 331:928–31. doi: 10.1126/science.1201148
104. Lv C, Zhang T, Li K, Gao K. Bone marrow mesenchymal stem cells improve spinal function of spinal cord injury in rats via TGF-β/Smads signaling pathway. Exp Ther Med (2020) 19:3657–63. doi: 10.3892/etm.2020.8640
105. Rabinstein AA. Traumatic spinal cord injury. Continuum (Minneapolis Minn) (2018) 24:551–66. doi: 10.1212/CON.0000000000000581
106. Ni S, Cao Y, Jiang L, Luo Z, Lu H, Hu J, et al. Synchrotron radiation imaging reveals the role of estrogen in promoting angiogenesis after acute spinal cord injury in rats. Spine (2018) 43(18):1241–1249. doi: 10.1097/BRS.0000000000002629
107. Menezes K, Rosa BG, Freitas C, da Cruz AS, de Siqueira Santos R, Nascimento MA, et al. Human mesenchymal stromal/stem cells recruit resident pericytes and induce blood vessels maturation to repair experimental spinal cord injury in rats. Sci Rep (2020) 10:19604. doi: 10.1038/s41598-020-76290-0
108. Bergers G, Song S. The role of pericytes in blood-vessel formation and maintenance. Neuro-oncology (2005) 7:452–64. doi: 10.1215/S1152851705000232
109. Zhang C, Zhang C, Xu Y, Li C, Cao Y, Li P. Exosomes derived from human placenta-derived mesenchymal stem cells improve neurologic function by promoting angiogenesis after spinal cord injury. Neurosci Lett (2020) 739:135399. doi: 10.1016/j.neulet.2020.135399
110. Zhou T, Zheng Y, Sun L, Badea SR, Jin Y, Liu Y, et al. Microvascular endothelial cells engulf myelin debris and promote macrophage recruitment and fibrosis after neural injury. Nat Neurosci (2019) 22:421–35. doi: 10.1038/s41593-018-0324-9
111. Zhou Y, Cui Z, Xia X, Liu C, Zhu X, Cao J, et al. Matrix metalloproteinase-1 (MMP-1) expression in rat spinal cord injury model. Cell Mol Neurobiol (2014) 34:1151–63. doi: 10.1007/s10571-014-0090-5
112. Whetstone WD, Hsu JY, Eisenberg M, Werb Z, Noble-Haeusslein LJ. Blood-spinal cord barrier after spinal cord injury: relation to revascularization and wound healing. J Neurosci Res (2003) 74:227–39. doi: 10.1002/jnr.10759
113. Cao Y, Xu Y, Chen C, Xie H, Lu H, Hu J. Local delivery of USC-derived exosomes harboring ANGPTL3 enhances spinal cord functional recovery after injury by promoting angiogenesis. Stem Cell Res Ther (2021) 12:20. doi: 10.1186/s13287-020-02078-8
114. Holmes D. Spinal-cord injury: spurring regrowth. Nature (2017) 552:S49. doi: 10.1038/d41586-017-07550-9
115. Hong EG, Ko HJ, Cho YR, Kim HJ, Ma Z, Yu TY, et al. Interleukin-10 prevents diet-induced insulin resistance by attenuating macrophage and cytokine response in skeletal muscle. Diabetes (2009) 58:2525–35. doi: 10.2337/db08-1261
116. Oh SK, Jeon SR. Current concept of stem cell therapy for spinal cord injury: A review. Korean J Neurotrauma (2016) 12:40–6. doi: 10.13004/kjnt.2016.12.2.40
117. Takahashi Y, Tsuji O, Kumagai G, Hara CM, Okano HJ, Miyawaki A, et al. Comparative study of methods for administering neural stem/progenitor cells to treat spinal cord injury in mice. Cell Transplant (2011) 20:727–39. doi: 10.3727/096368910X536554
118. Yoon SH, Shim YS, Park YH, Chung JK, Nam JH, Kim MO, et al. Complete spinal cord injury treatment using autologous bone marrow cell transplantation and bone marrow stimulation with granulocyte macrophage-colony stimulating factor: Phase I/II clinical trial. Stem Cells (2007) 25:2066–73. doi: 10.1634/stemcells.2006-0807
119. Fawcett JW, Curt A, Steeves JD, Coleman WP, Tuszynski MH, Lammertse D, et al. Guidelines for the conduct of clinical trials for spinal cord injury as developed by the ICCP panel: spontaneous recovery after spinal cord injury and statistical power needed for therapeutic clinical trials. Spinal Cord (2007) 45:190–205. doi: 10.1038/sj.sc.3102007
120. Karamouzian S, Nematollahi-Mahani SN, Nakhaee N, Eskandary H. Clinical safety and primary efficacy of bone marrow mesenchymal cell transplantation in subacute spinal cord injured patients. Clin Neurol Neurosurg (2012) 114:935–9. doi: 10.1016/j.clineuro.2012.02.003
121. Dai G, Liu X, Zhang Z, Yang Z, Dai Y, Xu R. Transplantation of autologous bone marrow mesenchymal stem cells in the treatment of complete and chronic cervical spinal cord injury. Brain Res (2013) 1533:73–9. doi: 10.1016/j.brainres.2013.08.016
122. El-Kheir WA, Gabr H, Awad MR, Ghannam O, Barakat Y, Farghali HA, et al. Autologous bone marrow-derived cell therapy combined with physical therapy induces functional improvement in chronic spinal cord injury patients. Cell Transplant (2014) 23:729–45. doi: 10.3727/096368913X664540
123. Kishk NA, Gabr H, Hamdy S, Afifi L, Abokresha N, Mahmoud H, et al. Case control series of intrathecal autologous bone marrow mesenchymal stem cell therapy for chronic spinal cord injury. Neurorehabil Neural Repair (2010) 24:702–8. doi: 10.1177/1545968310369801
124. Mendonça MV, Larocca TF, de Freitas Souza BS, Villarreal CF, Silva LF, Matos AC, et al. Safety and neurological assessments after autologous transplantation of bone marrow mesenchymal stem cells in subjects with chronic spinal cord injury. Stem Cell Res Ther (2014) 5:126. doi: 10.1186/scrt516
125. Honmou O, Yamashita T, Morita T, Oshigiri T, Hirota R, Iyama S, et al. Intravenous infusion of auto serum-expanded autologous mesenchymal stem cells in spinal cord injury patients: 13 case series. Clin Neurol Neurosurg (2021) 203:106565. doi: 10.1016/j.clineuro.2021.106565
126. Satti HS, Waheed A, Ahmed P, Ahmed K, Akram Z, Aziz T, et al. Autologous mesenchymal stromal cell transplantation for spinal cord injury: A phase I pilot study. Cytotherapy (2016) 18:518–22. doi: 10.1016/j.jcyt.2016.01.004
127. Cheng H, Liu X, Hua R, Dai G, Wang X, Gao J, et al. Clinical observation of umbilical cord mesenchymal stem cell transplantation in treatment for sequelae of thoracolumbar spinal cord injury. J Trans Med (2014) 12:253. doi: 10.1186/s12967-014-0253-7
128. Hur JW, Cho TH, Park DH, Lee JB, Park JY, Chung YG. Intrathecal transplantation of autologous adipose-derived mesenchymal stem cells for treating spinal cord injury: A human trial. J Spinal Cord Med (2016) 39:655–64. doi: 10.1179/2045772315Y.0000000048
129. Ra JC, Shin IS, Kim SH, Kang SK, Kang BC, Lee HY, et al. Safety of intravenous infusion of human adipose tissue-derived mesenchymal stem cells in animals and humans. Stem Cells Dev (2011) 20:1297–308. doi: 10.1089/scd.2010.0466
130. Yao L, He C, Zhao Y, Wang J, Tang M, Li J, et al. Human umbilical cord blood stem cell transplantation for the treatment of chronic spinal cord injury: Electrophysiological changes and long-term efficacy. Neural Regener Res (2013) 8:397–403. doi: 10.3969/j.issn.1673-5374.2013.05.002
131. Zhu H, Poon W, Liu Y, Leung GK, Wong Y, Feng Y, et al. Phase I-II clinical trial assessing safety and efficacy of umbilical cord blood mononuclear cell transplant therapy of chronic complete spinal cord injury. Cell Transplant (2016) 25:1925–43. doi: 10.3727/096368916X691411
132. Park JH, Kim DY, Sung IY, Choi GH, Jeon MH, Kim KK, et al. Long-term results of spinal cord injury therapy using mesenchymal stem cells derived from bone marrow in humans. Neurosurgery (2012) 70:1238–47. doi: 10.1227/NEU.0b013e31824387f9
133. Vaquero J, Zurita M, Rico MA, Aguayo C, Bonilla C, Marin E, et al. Intrathecal administration of autologous mesenchymal stromal cells for spinal cord injury: Safety and efficacy of the 100/3 guideline. Cytotherapy (2018) 20:806–19. doi: 10.1016/j.jcyt.2018.03.032
134. Muthu S, Jeyaraman M, Gulati A, Arora A. Current evidence on mesenchymal stem cell therapy for traumatic spinal cord injury: systematic review and meta-analysis. Cytotherapy (2021) 23:186–97. doi: 10.1016/j.jcyt.2020.09.007
135. Chen WC, Liu WF, Bai YY, Zhou YY, Zhang Y, Wang CM, et al. Transplantation of mesenchymal stem cells for spinal cord injury: a systematic review and network meta-analysis. J Trans Med (2021) 19:178. doi: 10.1186/s12967-021-02843-0
136. Liu S, Zhang H, Wang H, Huang J, Yang Y, Li G, et al. A comparative study of different stem cell transplantation for spinal cord injury: A systematic review and network meta-analysis. World Neurosurg (2022) 159:e232–43. doi: 10.1016/j.wneu.2021.12.035
137. Wislet-Gendebien S, Hans G, Leprince P, Rigo JM, Moonen G, Rogister B. Plasticity of cultured mesenchymal stem cells: Switch from nestin-positive to excitable neuron-like phenotype. Stem Cells (2005) 23:392–402. doi: 10.1634/stemcells.2004-0149
138. Galland S, Stamenkovic I. Mesenchymal stromal cells in cancer: a review of their immunomodulatory functions and dual effects on tumor progression. J Pathol (2020) 250:555–72. doi: 10.1002/path.5357
139. Khakoo AY, Pati S, Anderson SA, Reid W, Elshal MF, Rovira II, et al. Human mesenchymal stem cells exert potent antitumorigenic effects in a model of kaposi's sarcoma. J Exp Med (2006) 203:1235–47. doi: 10.1084/jem.20051921
140. Lan T, Luo M, Wei X. Mesenchymal stem/stromal cells in cancer therapy. J Hematol Oncol (2021) 14:195. doi: 10.1186/s13045-021-01208-w
141. Amariglio N, Hirshberg A, Scheithauer BW, Cohen Y, Loewenthal R, Trakhtenbrot L, et al. Donor-derived brain tumor following neural stem cell transplantation in an ataxia telangiectasia patient. PloS Med (2009) 6:e1000029. doi: 10.1371/journal.pmed.1000029
142. Ponticiello MS, Schinagl RM, Kadiyala S, Barry FP. Gelatin-based resorbable sponge as a carrier matrix for human mesenchymal stem cells in cartilage regeneration therapy. J Biomed Mater Res (2000) 52:246–55. doi: 10.1002/1097-4636(200011)52:2<246::AID-JBM2>3.0.CO;2-W
143. Han Y, Li X, Zhang Y, Han Y, Chang F, Ding J. Mesenchymal stem cells for regenerative medicine. Cells (2019) 8(8):886. doi: 10.1155/2019/5432134
144. Xia YL, Yang RH, Wang HY, Li YH, Fu CF. Application of chitosan-based materials in surgical or postoperative hemostasis. Front Mater (2022) 9. doi: 10.3389/fmats.2022.994265
145. Zhang J, Cheng T, Chen Y, Gao F, Guan F, Yao M. A chitosan-based thermosensitive scaffold loaded with bone marrow-derived mesenchymal stem cells promotes motor function recovery in spinal cord injured mice. Biomed Mater (Bristol England) (2020) 15:035020. doi: 10.1088/1748-605X/ab785f
146. Yao S, He F, Cao Z, Sun Z, Chen Y, Zhao H, et al. Mesenchymal stem cell-laden hydrogel microfibers for promoting nerve fiber regeneration in long-distance spinal cord transection injury. ACS Biomater Sci Eng (2020) 6:1165–75. doi: 10.1021/acsbiomaterials.9b01557
147. Boido M, Ghibaudi M, Gentile P, Favaro E, Fusaro R, Tonda-Turo C. Chitosan-based hydrogel to support the paracrine activity of mesenchymal stem cells in spinal cord injury treatment. Sci Rep (2019) 9:6402. doi: 10.1038/s41598-019-42848-w
148. Li J, Ji Z, Wang Y, Li T, Luo J, Li J, et al. Human adipose-derived stem cells combined with nano-hydrogel promote functional recovery after spinal cord injury in rats. Biol (2022) 11(5):781. doi: 10.3390/biology11050781
149. Xia Y, Yang R, Wang H, Hou Y, Li Y, Zhu J, et al. Biomaterials delivery strategies to repair spinal cord injury by modulating macrophage phenotypes. J Tissue Eng (2022) 13:20417314221143059. doi: 10.1177/20417314221143059
150. Katoh H, Yokota K, Fehlings MG. Regeneration of spinal cord connectivity through stem cell transplantation and biomaterial scaffolds. Front Cell Neurosci (2019) 13:248. doi: 10.3389/fncel.2019.00248
Keywords: spinal cord injury, mesenchymal stem cells, inflammatory factors, axon regeneration, macrophages, microglia
Citation: Xia Y, Zhu J, Yang R, Wang H, Li Y and Fu C (2023) Mesenchymal stem cells in the treatment of spinal cord injury: Mechanisms, current advances and future challenges. Front. Immunol. 14:1141601. doi: 10.3389/fimmu.2023.1141601
Received: 10 January 2023; Accepted: 13 February 2023;
Published: 24 February 2023.
Edited by:
Andreas Demetriades, University of Edinburgh, United KingdomReviewed by:
Miltiadis Georgiopoulos, McGill University, CanadaUlises Gomez-Pinedo, Health Research Institute of Hospital Clínico San Carlos, Spain
Copyright © 2023 Xia, Zhu, Yang, Wang, Li and Fu. This is an open-access article distributed under the terms of the Creative Commons Attribution License (CC BY). The use, distribution or reproduction in other forums is permitted, provided the original author(s) and the copyright owner(s) are credited and that the original publication in this journal is cited, in accordance with accepted academic practice. No use, distribution or reproduction is permitted which does not comply with these terms.
*Correspondence: Changfeng Fu, ZnVjZkBqbHUuZWR1LmNu