- Department of Radiation Oncology, Peking University Third Hospital, Beijing, China
Recent studies have revealed that tumor-associated macrophages are the most abundant stromal cells in the tumor microenvironment and play an important role in tumor initiation and progression. Furthermore, the proportion of macrophages in the tumor microenvironment is associated with the prognosis of patients with cancer. Tumor-associated macrophages can polarize into anti-tumorigenic phenotype (M1) and pro-tumorigenic phenotype (M2) by the stimulation of T-helper 1 and T-helper 2 cells respectively, and then exert opposite effects on tumor progression. Besides, there also is wide communication between tumor-associated macrophages and other immune compositions, such as cytotoxic T cells, regulatory T cells, cancer-associated fibroblasts, neutrophils and so on. Furthermore, the crosstalk between tumor-associated macrophages and other immune cells greatly influences tumor development and treatment outcomes. Notably, many functional molecules and signaling pathways have been found to participate in the interactions between tumor-associated macrophages and other immune cells and can be targeted to regulate tumor progression. Therefore, regulating these interactions and CAR-M therapy are considered to be novel immunotherapeutic pathways for the treatment of malignant tumors. In this review, we summarized the interactions between tumor-associated macrophages and other immune compositions in the tumor microenvironment and the underlying molecular mechanisms and analyzed the possibility to block or eradicate cancer by regulating tumor-associated macrophage-related tumor immune microenvironment.
1 Introduction
With the advancement of tumor immunology research, increasing cell subtypes have been identified in the tumor nest, and then the roles of the tumor microenvironment (TME) have attracted extensive attention (1). With the development of single-cell technologies, there is a new understanding of the importance of TME for tumor initiation and progression (2, 3). TME is a complex environment that is mainly composed of tumor cells and various immune cells, such as T cells, tumor-associated macrophages (TAMs), natural killer (NK) cells, neutrophils, dendritic cells (DCs), B lymphocytes and cancer-associated fibroblasts (CAFs) (4–9). Previous studies revealed that the interactions among various immune cells in the TME play an important role in tumor progression. The underlying mechanisms include gap junctions (10), receptors (11), release of small molecules (12), tunneling nanotubes (13), vesicles (14) and mechanical forces (15, 16). Furthermore, recent clinical trials have found that immunotherapy, which mainly relies on the activation of immune effector cells within TME by inhibiting immune checkpoints, has achieved a great success to improve the prognosis of patients with malignant tumors (17–19). Particularly, several immune checkpoint inhibitors to PD-1 and PD-L1 have been supplemented into the first-line treatment for some malignancies (20, 21).
TAMs are one of the most abundant cell types present in the TME of various cancers (22) and are tightly associated with other tumor infiltrated immune cells. Recent studies found that regulators of TAMs polarization and function can effectively modulate tumor progression (23). PD-1/PD-L1 and cytotoxic T lymphocyte antigen 4 (CTLA4) remain the most widely used targets of immune checkpoint inhibitors, which mainly regulating the immune functions of T cells (24–26), and increasing studies revealed that the number, activation status and polarization direction of TAMs are closely associated with the therapeutic efficacy (27–29). These findings suggest that TAMs have an irreplaceable effect in immunotherapy for cancers. In addition, chemotherapy coupled to macrophage-targeting strategies induces a more strong anti-tumor effect and achieves more tumor regressions in triple-negative breast cancer (29), pancreatic adenocarcinoma (30) and non-Hodgkin lymphoma (31). Meanwhile, macrophages inhibition combined with radiotherapy can also enhance anti-tumor effects (32, 33). Therefore, macrophages-based therapy may represent a novel approach for treating cancer. In this review, we summarized the characteristics of TAMs and the interactions between TAMs and other infiltrated immune cells, hoping to contribute to the understanding of TAMs and suggest effective ways related to TAMs-based modulation to block or eradicate cancers.
2 The characteristics of macrophage
2.1 The origin of macrophage
Although the exact mechanism of macrophage formation remains controversial, two distinct lineages, bone marrow-derived macrophages, and tissue-resident macrophages have been widely recognized (34–38). Tissue-resident macrophages are embryonically derived and self-maintain locally (39), while bone marrow-derived macrophages are differentiated from monocytes originating from progenitors in the bone marrow which migrate from the bloodstream into tissues both in homeostasis and inflammation, following the stimulation of local growth factors, pro-inflammatory cytokines, and microbial products (Figure 1). Besides, these macrophage populations have a distinct temporal and spatial distribution in the TME (40). Tissue-resident macrophages spread to surrounding tumor cells early in the initial stages to promote epithelial-mesenchymal transition (EMT) and enhance the invasion, and they increase the number of regulatory T cells to promote the immune escape of tumor cells (40). Thus, the tissue-resident macrophages may be a novelly potential target for tumor therapy (38, 41). Phenotypically, different subtypes of macrophages can be identified by a set of overlapping and unique markers (Figure 1) and we have summarized them in Table 1.
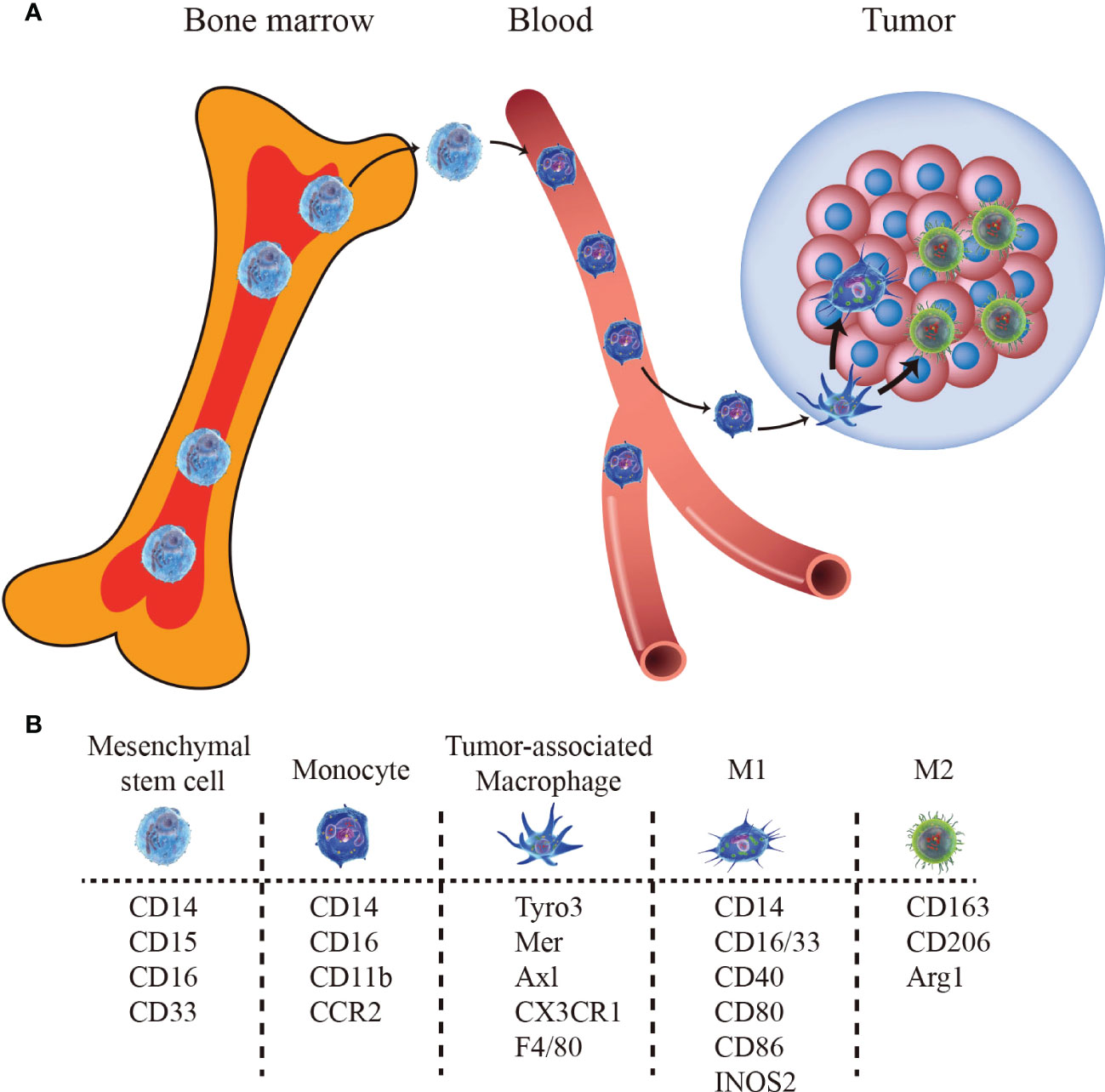
Figure 1 The bone marrow-derived macrophages pathway and biomarkers. (A) Monocytes egress from bone marrow and migrate to tumor sites, differentiating into TAMs. (B) Markers for mesenchymal stem cells, monocytes, TAM, M1-type TAM, and M2-type TAM.
2.2 Macrophage polarization
Usually, macrophages are mainly polarized into two distinct subtypes to exert functions in regulating tumor progression. Lipopolysaccharide (LPS) together with pro-inflammatory cytokines such as Interferon-γ (IFN-γ) and tumor necrosis factor-α (TNF-α) assists the polarization of macrophages to the M1 phenotype (42, 43). Th1 cells are shown to be the major source of IFN-γ and TNF-α in the TME general inflammation. The alternatively activated macrophages (M2) are mainly activated by Th2 cytokines interleukin (IL)-4 and IL-13. The M1 macrophages secrete pro-inflammatory cytokines TNF-α, IFN-γ, IL-1β and IL-8 and exert pro-inflammatory and anti-tumor functions. While M2 macrophages mediate anti-inflammatory and tumorigenesis actions through producing anti-inflammatory factors transforming growth factor-beta (TGF-β), arginase 1 (Arg-1) and IL-10.
Moreover, recent findings revealed that M2 macrophages can be further divided into M2a, M2b, M2c and M2d subsets with distinct functions (Table 2). M2a-subset macrophages activated by IL-4 or IL-13 play an essential role in fibrosis, parasite killing and allergy. Both positive of CD206 and CD68 (CD206+/CD68+) is the character of M2a-subset macrophages. M2b-subset macrophages induced by immune complexes in combination with IL-1β or LPS play a vital role in immune response and are characterized by the expression of CD86 receptors (44, 45). M2c macrophages induced by IL-10, TGFβ or glucocorticoids exert a key role in anti-inflammatory and are characterized by the expression of CD163 receptors (46, 47). The M2d macrophages play an important role in tumor progression and are characterized by increased IL-10 and VEGF secretion and decreased expression of IL-12 and TNF-α, however, the specific mechanism underlying programming the M2d macrophages remains controversial (48, 49).
3 Macrophages regulate tumor progression
Different directions of TAM polarization result in opposite functions in cancer progression. At the initial stages of tumor formation, macrophages mainly play a proinflammatory role and suppress tumor development, although the related evidence is still limited (50). As the tumor grows, macrophages in the TME are “educated” to a protumor phenotype by Th2 cells. Then, cytotoxic macrophages become tumor-supportive macrophages and promote tumor progression (51). A growing number of evidence suggested that TAMs exerted modulatory functions on tumorigenesis, progression, metastasis, angiogenesis and chemo-resistance (52) (Figure 2). For example, colony stimulating factor-1 (CSF-1) promotes malignant transformation in mammary cancer by recruiting macrophages (53). TAMs can also facilitate tumor cell intravasation and extravasation by secreting epidermal growth factor (EGF) (54) and vascular endothelial growth factor (VEGF) (55). EGF secreted by TAMs promotes tumor cell intravasation into blood vessels, while VEGF triggers endothelial cell barrier disruption by destroying adherens junctions. In addition, TAMs modulate tumor metastasis by regulating the EMT process through STAT/miR-506-3p/FoxQ1 signaling and TAT/miR-506-3p/FoxQ1 pathway and promote extracellular matrix degradation via secreting matrix metalloproteinases and C-C motif chemokine ligand 18 (CCL18) (56–60). Besides, M1-type TAMs can exert a direct killing effect on tumor cells once activated by IFN-γ or mediate adaptive immunity by recruiting and activating CD8+ T cells and NK cells after presenting tumor antigens and producing chemokines and cytokines (50). Majority of the TAM’s in the TME tends to be M2 but not M1 thereby shifting the antitumor microenvironment to an immunosuppressive milieu (61). However, several studies reached opposite conclusions. For example, M2 macrophages may have a partial limiting effect on colorectal cancer metastasis (62), whereas M1 macrophages promote tumor progression (63, 64). Therefore, the characteristics of TAMs contribute to better understanding the cancer states and exploring new ways to block or eradicate cancers.
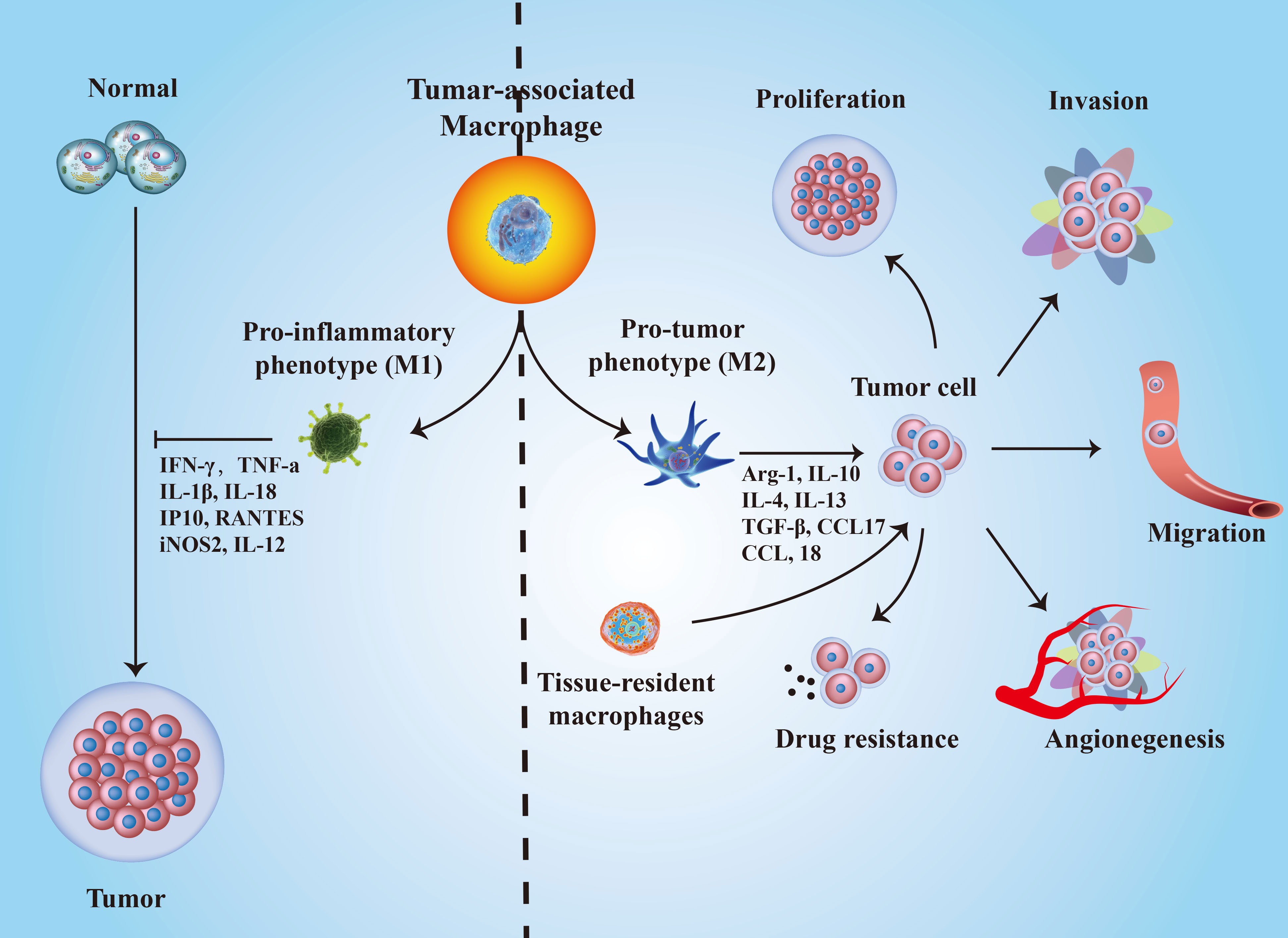
Figure 2 The roles of TAMs polarization in cancer progression. M1-type TAMs inhibit tumorigenesis by secreting IFN-γ, TNF-α, IL-18, IP10, IL-12, and iNOS2; while M2-type TAMs promote cancer development through several biological molecules, such as Arg-1, IL-10, IL-4, IL-13, TGF-β, CCL17, CCL-18, and so on.
4 Interactions between TAMs and other cell components in the TME
Recently, substantial studies revealed that TAMs play a pivotal role in regulating tumor development through interacting with various immune cells in TME (50). For example, M2-phenotype TAMs gradually becomes the major TAM under the stimulation of Th2 cells, and then the antitumor functions of TAMs are diminished (61). In addition, M1-type TAMs can exert a killing effect on tumor cells once activated by IFN-γ (50). TAMs can also express T-cell immune checkpoint ligands, such as PD-L1, CD80 and CD86, to inhibit T-cell functions (50, 65). Therefore, it is important to better understand the interactions between TAMs and other immune cells in the TME. The detailed interactions between other TME contents and TAMs were further described as follows (Figure 3).
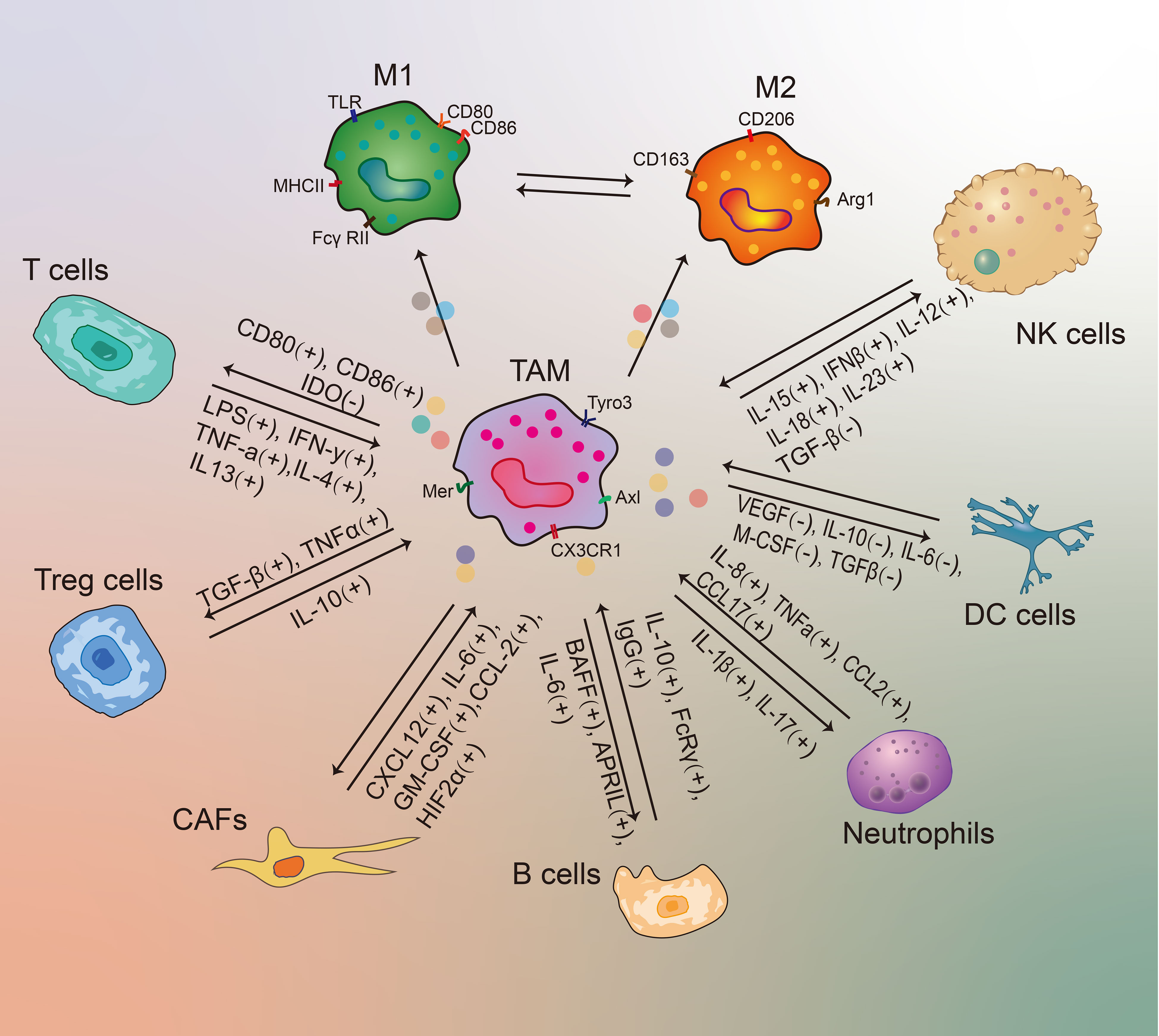
Figure 3 The molecular interactions between TAMs and other immune cells in the TME. A “+” or “-” sign shows a stimulatory or inhibitory interaction.
4.1 TAMs and T cells
Both TAMs and T cells can polarize into different subtypes with different functions under diverse signals’ stimulation. On the one hand, the polarization of TAMs is mainly regulated by T cells, especially Th1 and Th2 cells. On another hand, during antigen presentation, macrophages can also simultaneously activate multiple T cells (66). T cells perceive the stimulatory signals through interacting with macrophages and are eventually activated. Therefore, macrophages play an important role in activating T cells. Different macrophages subtypes may have different functional effects on T cells. For example, the M1 macrophages can activate T cells via upregulating B7 receptors, such as CD80 (B7-1) and CD86 (B7-2), while M2 macrophages are not able to express costimulatory molecules of the B7 family but exert disruption function through binding with T cells (67). FOLR2+ macrophages, which are located in the perivascular spaces in the tumor tissue, can effectively activate the CD8+ T cells in the tumor nest, and then improve the prognosis of breast cancer patients (68). In addition, macrophages also inhibit T cell proliferation in vitro through indoleamine 2,3-dioxygenase (IDO)-induced tryptophan degradation (69). The cavity-resident macrophages with high levels of Tim-4 can weaken the efficacy of anti-PD1 therapy in lung cancer by reducing PD-1 expression levels in CD8+ T cells (70). Moreover, the levels of TAMs-secreted TGF-β are significantly elevated in malignant pleural effusion, which plays an important role in destroying T cell function and promoting cancer progression in lung cancer patients (12).
Recent studies have confirmed that TAMs could produce large amounts of extracellular vesicles to influence the biological function of tumor cells and T cells in TME through extracellular vesicles fusion or cell-cell membrane contact (71). Notably, though TAMs exert immunosuppressive in various cancers, TAMs-derived extracellular vesicles can promote T cells proliferation and activation and exhibit M1 macrophages characteristics in colorectal tumor (71). However, M2 macrophages-derived extracellular vesicles induce CD8+ T cells exhaustion and promote tumor progression in hepatocellular carcinoma (72). Wang et al. introduced the nucleus of tumor cells into M1 macrophages to create chimeric exosomes, the chimeric exosomes can enter lymph nodes and induce T cells activation through direct exosome contact or antigen presenting cells induced immunostimulatory manner (14).
In addition to producing soluble secreted factors and extracellular vesicles, macrophages also modulate functions of T cells through direct interaction. A new research found that there were unique, antigen-specific synaptic interactions between TAMs and CD8+ T cells through using lattice light sheet microscopy (73). These interactions were unable to activate T cells, but result in exhaustion of T cells, which is significantly enhanced under hypoxic conditions (73). Therefore, seeking effective ways to target both macrophages and T cells may be a promising approach to improving the efficacy of immunotherapy.
4.2 TAMs and Tregs
Treg cells are a subset of CD4+ T cells which play a key role in tumor-associated immunosuppression (74). These cells are defined by the characteristic of the expression of transcription factor Foxp3 and IL-12 receptor α-chain (CD25). In inflammation resolution, Tregs stimulate macrophages’ efferocytosis via the production of IL-10 and induce apoptotic cell internalization (75). They can efficiently promote macrophages’ polarization into the M2 phenotype and downregulate the immune response (76, 77). Meanwhile, macrophages can maintain Tregs proliferation to suppress type 2 inflammatory responses (78). Furthermore, Kraaij et al. found that Tregs induced by macrophages are regulated via macrophages-derived reactive oxygen species (ROS) (79).
Many studies have established that Tregs promote tumor progression, such as hepatocellular carcinoma (80), breast cancer (81) and esophageal squamous cell carcinoma (82). Studies have also revealed a significant association between macrophages and Tregs in tumor progression (83). For example, TAMs induce the conversion of CD4+ T cells into Tregs through secreting TGF-β and promoting PD-1 expression on CD4+ T cells, resulting in Tregs infiltration in tumors (84). M2 macrophages can activate the TGF-β/Smad signaling pathway by expressing TGF-β, then induce Tregs generation and promote colorectal cancer development (85). In epithelial ovarian cancer, TAMs can upregulate Treg/Th17 ratios and promote tumor progression through releasing exosomes that contain miR-29a-3p and miR-21-5p targeting STAT3 to T cells (86). In addition, Liu et al. found that Tregs facilitate the M2-polarization of macrophages through inhibiting CD8+ T cells expression of IFN-γ and activating M2 macrophages sterol regulatory element-binding protein 1 mediated fatty acid synthesis (87). Thus, targeting Tregs and TAMs interaction may be an effective anti-tumor approach. In addition, radiotherapy is considered one of the most important treatment modalities in clinic. It is well recognized that radiotherapy induces inflammatory cells recruitment into TME, as well as immunosuppressive cells (88). Mondini and colleagues confirmed that radiotherapy can promote the secretion of CCL2 by tumor cells and induce the accumulation of CCR2+ Tregs and CCR2-dependent macrophages which can produce TNF-α, then TNF-α induces Tregs activation and decreases the efficacy of radiotherapy (89). Therefore, CCL2/CCR2 inhibitors in combination with radiotherapy may be an efficient approach for improving the therapeutic effects of radiotherapy in tumor treatment.
In addition to potential therapeutic targets, macrophages and Tregs infiltration can also be used as a prognostic biomarker for tumors. For example, the high level expression of Tregs indicates a better prognosis in early-stage gastric cancer patients, while the opposite results have been found in late-stage patients (90). Meanwhile, M2 macrophages predict a worse prognosis in general, however, high infiltration of M2 macrophages suggests a good prognosis in signet ring cell carcinoma and mucinous adenocarcinoma. The combination of both indicators can improve the prediction accuracy of cancers. In addition, single-cell RNA sequencing (scRNA-seq) revealed that M2 macrophages and Tregs infiltration are adverse prognostic factors for prostate cancer patients (91), colorectal cancer (92) and hepatocellular carcinoma patients (93). Thus, the specific prognostic value of TAMs and Tregs should be investigated in different cancers.
4.3 TAMs and CAFs
Activated fibroblasts in tumors are defined as CAFs (94). Though the origin of CAFs remains controversial, some researchers proposed that CAFs can derive from tissue-resident fibroblast, bone-marrow-derived mesenchymal stem cells (95), pancreatic or hepatic stellate cells (96, 97), adipocytes (98) and endothelial cells (99). CAFs exhibit a wide range of phenotypic and functional heterogeneity, and there is no clear biological marker to identify CAFs at present (100, 101). The functions of CAFs in tumor progression have been widely studied. For example, CAFs have been demonstrated to promote tumorigenesis and metastasis in breast cancer (102), lung cancer (103) and colorectal carcinoma (104). Moreover, CAFs have also been found to exert anti-tumor effects in pancreatic cancer (105).
As TAMs and CAFs are both major components in TME and TAMs infiltration increases in the regions where CAFs are enriched, there might exist a tight correlation between them (106, 107). Studies have revealed that CAFs can regulate TAMs infiltration in TME and induce TAMs to polarize into a pro-tumorigenic phenotype (107). For example, Zhang et al. found that CAFs can induce TAMs infiltration and promote M2 macrophage polarization, which leads to loss of NK cells function and contributes to an immune suppressive environment in colorectal cancer (108). Furthermore, a similar effect of CAFs on TAMs was found in hepatocellular carcinoma through secreting CXCL12 (109). Similarly, CAFs can also stimulate TAMs through other cytokines, such as IL-6, GM-CSF (110) and CCL2 (111). In addition to cytokines, CAFs can also regulate M2 macrophage polarization through expressing hypoxia inducible factor 2α (HIF2α) and promote pancreatic cancer progression (112). Meanwhile, CAFs not only promote TAMs infiltration and polarization but also enhance TAMs expression of PD-1, which leads to decreased phagocytosis and enhanced immunosuppressive functions (113, 114).
TAMs also regulate CAFs functions and activation as well. For example, Tang et al. revealed that TAMs promote CAFs generation via Smads-mediated macrophage-myofibroblast transition (115). Meanwhile, M2 macrophages can enhance CAFs activation by regulating the mesenchymal-mesenchymal transition of fibroblasts (115) and secreting TGF-β (116). Then, activated CAFs further enhance TAMs recruitment and activity, resulting in an immunosuppressive environment. Collectively, the interaction between TAMs and CAFs generates a cancer-promoting phenotype. However, the exact mechanism of CAFs and TAMs interaction remains undefined, further investigation is required for therapeutic exploitation.
4.4 TAMs and B cells
Recently, several studies have revealed that high levels of B cells in the tumor nest indicate a persistent immune activation response and predict a good efficacy of immunotherapy for patients with cancer (117). Furthermore, TAMs and B cells have a close association. For example, subcapsular-sinus macrophages play an important role in accumulating various larger antigens through the expression of sulphated glycoproteins which can preserve the integrity of antigens and then present antigens to the neighboring follicular B cells (118–120). Meanwhile, macrophages can regulate the transportation and retention of B cells in the splenic marginal zone (121).
Besides, tissue-resident macrophages are not only derived from monocytes but can also differentiate from early pro-B cell/fraction B within the bone marrow, these macrophages’ precursors enter into the systemic circulation and acquire the same transcriptome identical as embryonically derived macrophages (122). These macrophages precursors also gain CD115, F4/80, and CD16/32 after entering inflammation sites, which are very similar to blood monocyte-derived macrophages (122). Thus, pre/pro-B cells may be an additional source of macrophages. It is worth noting that B1 cells can migrate into the inflammatory milieu and differentiate into a macrophage-like cell type in vitro (123). In turn, macrophages can also regulate B cell proliferation via secreting B cells-activating factor (BAFF) and a proliferation-inducing ligand (APRIL) (124). Macrophages have also been verified to support the later B1 cells development via expressing IL-6 (125). Meanwhile, B cells can also regulate the polarization of macrophages. For example, B cells induce peritoneal macrophages to polarize into an M2-like phenotype through secreting IL-10 and this phenomenon is also observed in tumors where B cells reprogram TAMs into the M2 macrophages (126). In addition, Andreu et al. found that B cells promote the M2 macrophage infiltration and induce the proangiogenic and protumorigenic effects of macrophages through activating Fc-gamma (FcRγ) in squamous carcinoma (127). Chemokines receptors trigger B cells migration into lymphoid follicles, such as chemokine (C-X-C motif) receptor 4 (CXCR4) and CXCR5 (128). Liu and colleagues demonstrated that CXCR3+ B cells infiltrate predominantly in hepatocellular carcinoma invading edge and are associated with tumor recurrence, furthermore, CXCR3+ B cells induce TAMs repolarization into M2 macrophages through an IgG-dependent manner and promote hepatocellular carcinoma progression (129).
Collectively, current studies have revealed that TAMs and B cells have associations in their origins and influence each other. However, the detailed mechanisms remain unclear and still require further clarification.
4.5 TAMs and neutrophils
Neutrophils are phagocytic cells that are an important part of the innate immune system and play a pivotal role in the first–line of defense (130). Like TAMs, tumor–associated neutrophils (TANs) can also polarize into anti–tumor (N1) phenotype and pro–tumor (N2) phenotype according to different cytokines stimuli (131). Considering that neutrophils can recruit macrophages via secreting IL–8 and TNF–α in an inflammatory environment and macrophages can in turn regulate neutrophils function, the TAMs and TANs may have close interrelationships during tumor progression (132). For example, TAMs induce IL–17 production through releasing IL–1β, the IL–17 can enhance neutrophils recruitment and promote tumor metastasis in breast cancer (133, 134). Similarly, TANs promote TAMs and T–regulatory cell recruitment in hepatocellular carcinoma via secreting CCL2 and CCL17, leading to tumor growth and drug resistance (135). Meanwhile, both TAMs and TANs can produce matrix metalloproteinase–9, which releases angiogenic factors and VEGF to promote angiogenic (136).
Besides, growing evidence has found that the neutrophils-to-lymphocytes ratio is a prognostic biomarker in patients with pancreatic tumors (137), colorectal cancers (138) and hepatocellular carcinoma (139), and a higher ratio predicts a poor prognosis. The accumulation of TAMs in TME can elevate the neutrophils-to-lymphocytes ratio and confers a poorer prognosis for patients (137). Furthermore, Huang et al. found that a combination of CD163+ TAMs and CD66b+ TANs is an important prognostic marker for gastric cancer patients (140).
4.6 TAMs and DCs
Macrophages and DCs are forefront cells of innate immunity, they are capable of sensing and immediately against invading pathogens (141). Though macrophages and DCs are different cell types and originate from different lineages, they express several same markers and exhibit some similar functions (142). For example, macrophages and DCs are both found in peripheral tissues and accumulate in the areas of pathogen entry (143). Besides, macrophages and DCs can exert a synergetic effect on connecting innate and adaptive immunity through recognizing and presenting the foreign antigens to T cells (144–146). The phagosomal degradation of DCs is lower than macrophages, which retain the antigenic peptides and initiates adaptive immune responses (143).
ScRNA-seq analyses found that macrophages and DCs play a key role in mediating cellular cross-talk in the TME and regulate tumor immunity (147). The potent anti-tumor immune response needs antigen presentation by macrophages and DCs. Immature DCs can get matured and migrate from the periphery to the lymph node and activate T cells when they recognize pathogen-associated molecular patterns (PAMPs) and damage-associated molecular patterns (DAMPs) (148). Whereas, the maturation and function of DCs can be inhibited by several factors secreted by tumor cells and TAMs, such as VEGF, IL-10, IL-6, M-CSF and TGFβ (149–153). Furthermore, a study revealed that the immature or defective DCs results in T cells’ unresponsiveness and immunosuppression in TME (154). DAMPs have been referred to endogenous molecules and fragments from damaged cells and tissues, which were also be recognized as danger signals (155, 156). The adenosine triphosphate (ATP), which is also an important component of TME, is likely to be the prototypical and most widely diffused DAMPs (157). Studies have confirmed that ATP can not only promote DCs migration into lymph nodes and activate T cells, but also regulate TAMs physiology (158–160). Regarding the proteins involved in this ATP-related signaling, there are Connexins and Pannexins channels, which allow contact dependent or independent communication (160–162). Interestingly, Pannexins are differentially expressed during macrophage polarization, which makes them valuable target for therapy (163). In advanced osteosarcoma, Zhou et al. found that monocytes and macrophages make up the majority of total myeloid cells at 70-80%, while DCs only account for less than 5% by scRNA-seq analysis (164). It is still uncovered whether the decreasing proportion of DCs is associated with poor prognosis in tumors. Currently, there are limited studies exploring the interaction of DCs and macrophages on the effects of tumors, targeting the cross-talk between DCs and macrophages may be an effective anti-tumor strategy.
4.7 TAMs and NK cells
NK cells are also an important component of innate immunity which play a pivotal role in the defense against infections and cancer (165). NK cells can also promote TAMs to repolarize into the M1-type macrophages (166). The cross-talk between macrophages and NK cells have been verified as an important part of inflammatory and anti-tumor reactions. Macrophages promote NK cell activation mainly through secreting cytokines, such as IL-15, IFNβ (167), IL-12, IL-18 (168) and IL-23 (169). Once activated, NK cells produce large amounts of IFN-γ to exert cytotoxic effects. Besides, M1 macrophages increase NK cells number and induce NK cell activation to express TNF-related apoptosis-inducing ligand which can promote hepatic stellate cell apoptosis in the fibrotic liver (170). In contrast, TAMs can also inhibit NK cell function through expressing TGF-β (171).
Notably, NK cells exhibit distinct functions when interacting with different phenotypes of TAMs. For example, activated NK cells can kill M0- and M2-TAMs, while the M1-TAMs are more resistant to lysis than M0- and M2-TAMs due to their high levels of HLA class I molecules (172). Besides, after stimulation with LPS, M0- and M2-TAMs induce the activation of resting NK cells and promote the expression of CD69, CD25 and CCR7.
4.8 TAMs and NKT cells
Natural killer T (NKT) cells are a unique lymphocyte population which can recognize lipid antigens presented by the MHC class I-like molecular CD1d (173). Upon activated by CD1d, NKT cells initiated an essential role in autoimmunity, infection and tumor immunity through secreting a lot of cytokines, including TNF-α, IFN-γ, IL-4, IL-6 and IL-17 (174–177). Furthermore, the activated NKT cells also increase the proportion of M1-type macrophages and reduce M2 macrophages in the TME to exert an antitumor effect (173). In addition, recent study found that TAMs can promote tumor growth through producing IL-6, but accounting for majority of CD1d-expressing cells (178). Further mechanismic research revealed that CD1d-activated NKT cells can recognize TAMs specifically and kill TAMs to suppress tumor growth (178). Therefore, NKT-based therapies that can against both tumor cells and TAMs will be an effective antitumor treatment.
5 Cancer cell therapy by targeting TAMs-based communications among TME
Since the first chimeric antigen receptor (CAR)-T cell therapy (Kymriah) was approved by FDA, the cell therapy field is still expanding and evolving (179). Although CAR-T therapy has achieved remarkable success in hematological malignancies, the efficacy of CAR-T treatment of solid tumors is limited (180, 181). Therefore, it is urgent to find more effective cellular immunotherapeutic strategies. Currently, the unique characteristics of macrophages make it a proper candidates for the treatment of solid tumors (182). CAR macrophages (CAR-M) demonstrated antigen-specific phagocytosis and increase antigen-presentation ability. Meanwhile, CAR-M can also reprogram M2 macrophages to M1 and stimulate the expression of pro-inflammatory cytokines and chemokines to induce a pro-inflammatory microenvironment and enhance T cell-mediated antitumor activity (182, 183). Nevertheless, clinical trials and results about CAR-M have been highly limited, there is still a long way to go for CAR-M therapy (184). Given that the crucial role of TAMs in cancer progression and response to treatment, TAMs-based cell therapies have been well studied and the combination therapeutic strategies in clinical trials are included in Table 3.
5.1 Targeting TAMs and T cells in cancercell therapy
Immune checkpoint inhibitors have demonstrated effective anti-tumor effects by regulating T cell activity. Furthermore, their functions in regulating macrophages have also been revealed. PD-L1 is a significant immune suppressor which can regulate macrophages and T cells interaction in tumors (185). Xiong et al. reported that anti-PD-L1 therapy can not only activate CD8+ T cells expressing a high level of granzyme-B but also reprogram macrophages from anti-inflammatory to a pro-inflammatory phenotype, meanwhile, increasing the CD8+ T/Treg ratio (186). Therefore, targeting both macrophages and T cells is required for synergistic therapy.
The CD47/signal regulatory protein-α (SIRPα) cascade is an important transmembrane protein that functions as a “don’t eat me” signal, which can be delivered to macrophages (187). Depletion of SIRPα on intratumoral macrophages can enhance the therapeutic response of radiotherapy and reshape the TME from anti-inflammatory to pro-inflammatory. Furthermore, the SIRPα-/- can promote high levels of pro-inflammatory factors expression, induce tumor-specific cytotoxic CD8+ T cells expansion and activation, and exert efficient anti-tumor immunity in colorectal and pancreatic tumors (188). Therefore, targeting CD47/SIRPα signaling may play an important role in regulating macrophages and T cells. Furthermore, recent studies have revealed that the combination of anti-PD1/PD-L1 and inhibition of CD47/SIRPα signaling developed more effective cancer immunotherapy through activating macrophages phagocytosis and antitumor effects which can further activate CD8+ T cells (189, 190). In addition to the CD47/SIRPα cascade, CD40 agonists re-educate TAMs into M1 macrophages to restore cancer immune surveillance (191), and the combination therapy of anti-PD1/PD-L1 and anti-CD40 also enhances anti-tumor efficacy (191, 192).
Recently, a novel nanomedicine has been constructed that can activate CD4+ T cells and CD8+ T cells and polarize the M2 macrophages to M1 macrophages, which induced potent anti-tumor immunity and has good clinical application prospects (193). Besides, Wang and colleagues found a novel cryo-thermal therapy that can induce substantial amounts of iron secretion, which promote M1 macrophage polarization through inhibiting ERK phosphorylation and the M1 macrophages can further promote CD4+ T cells differentiation into CD4 cytolytic T lymphocytes (CTL) (194). In addition, blockade of macrophage scavenger receptor common lymphatic endothelial and vascular endothelial receptor-1 (Clever-1) (185), ibuprofen (195), sophoridine (196) and all-trans retinoic (197) have also been demonstrated to activate endogenous antitumor CD8+ T cells and convert the TME from anti-inflammatory to pro-inflammatory state.
Adoptive immunotherapy with CAR-T cells has shown good clinical value on the prognosis of patients with cancer, expecially those with hematologic malignancies (198). Rodriguez and colleagues demonstrated that CAR-T cells specific for human FRβ specifically recognize and delete M2-like FRβ+ TAMs and enhance the anti-tumor efficiency of CAR-T cells (199). However, cytokines, including IL-6 and IL-1β released from macrophages may cause serious adverse effects of CAR-T therapy, such as cytokine release syndrome (CRS) (200, 201). CRS is thought to be the most common severe toxicity of CAR-T therapy which is characterized by high fevers, hypotension, hypoxia, sunus tachycardia and depressed cardiac function and greatly limit the broad use of CAR-T treatment (202–206). Therefore, it is urgent to find effective therapeutic strategy targeting macrophages to reduce the occurrence of CRS. Taken together, these findings suggest that novel strategies targeting both TAMs and T cells can significantly enhance anti-tumor activity.
5.2 Targeting TAMs and Tregs in cancer cell therapy
High-level infiltration of Tregs in TME has been demonstrated to be associated with poor prognosis (207), while the depletion of Tregs with anti-CD25 has been used in tumors and achieved preliminary results in melanoma (208), ovarian, breast and lung carcinoma (209). Currently, there is limited evidence for the use of combination therapy between macrophages and Tregs. Liver X receptor (LXR) is a member of the nuclear receptor family of transcription factors (210), studies have found that LXR agonists can obstruct tumor growth in melanoma (211), breast cancer (212), lung cancer and colon cancer (213). However, the exact mechanism of their anti-tumor activities remains undefined. Carbo and colleagues found that LXR agonist T0901317 can reduce infiltration of Tregs in tumors and TAMs expression of chemokine CCL17 which attracts Tregs migration. Furthermore, LXR agonists also inhibit IRF4 expression which further reduces the downstream genes in macrophages, such as CCL17 (214). Thus, activation of LXR might be an effective treatment in regulating the TAMs and Tregs-mediated immunosuppressive in tumors. Macrophage receptor with collagenous structure (MARCO) is a scavenger receptor expressed mainly in macrophages (215), MARCO-expressing TAMs have been demonstrated to induce Tregs proliferation and promote tumor progression in lung cancer, thus targeting MARCO with antibodies decrease Tregs frequencies and activation (216).
5.3 Targeting TAMs and CAFs in cancer cell therapy
Due to the highly heterogeneous of CAFs, it is difficult to target CAFs through unique markers. Thus, it is necessary to investigate the molecules and signaling pathways that affect CAFs activation and function. Studies have demonstrated that NFκB induces CAFs activation and promotes tumor epithelial-mesenchymal transition and induces chemo-resistance by expressing IL-6 and IL-8 (103, 217). Therefore, the NFκB signaling pathway may be a potential target for cancer therapy. CSF1/CSF1R signaling pathway plays a key role in regulating TAMs proliferation and polarization, many studies have confirmed the effectiveness of CSF1R inhibitors in depleting TAMs and targeting tumors (218, 219). However, Kumar et al. found that CAFs can promote polymorphonuclear myeloid-derived suppressor cells migrating into tumor tissues through secreting CXCL1 and weaken the anti-tumor effect of CSF1R inhibitors (220). Therefore, a combination of CSF1R inhibitor with blockade of macrophage recruitment may improve treatment efficacy. Furthermore, a synergistic anti-tumor effect was observed when combined anti-PD-1 with these two inhibitors.
5.4 Targeting TAMs and B cells in cancer therapy
The relationship between TAMs and B cells in inflammation and tumor has been reported, however, the related applications in treatment have been poorly analyzed. Affara et al. found that clearance of B cells can regulate TAMs reprogram into the M1 macrophages by using B cells-specific deletion mice, induce macrophages to express anti-tumor chemokines and activate CD8+ T cells in squamous carcinomas. In addition, αCD20 monoclonal antibodies, which can deplete B cells, have also been demonstrated to promote TAMs to express high levels of angiostatic and CCR chemokines, such as CXCL10, CXCL11, and CCR5, which can elevate CD8+ T cells infiltration and improve the response to chemotherapy. Thus, the interaction of B cells and macrophages may serve as a target for cancer cell therapy.
It is well known that CD40 agonist antibodies can reprogram M2 macrophages into M1 macrophages. Furthermore, Inoue and colleagues found that CD40-CD40L interaction can down-regulate the immunosuppressive effects of B cells on T cells and NK cells and stimulate IFN-γ production to exert anti-tumor immune response (221). Therefore, depletion of B cells and reprogramming macrophages via CD40 agonist antibody may have potential use in cancer treatment. Studies have confirmed that TAMs have an important role in the progression of B-cell lymphomas, such as classic Hodgkin’s lymphoma (222) and chronic lymphocytic leukemia (223). Considering the CSF1/CSF1R signaling pathway as an effective therapeutic target for depleting and reprogramming TAMs, blockade of CSF1/CSF1R signaling has been demonstrated to effectively deplete neural-like cells and control the progression of chronic lymphocytic leukemia (223). However, TAMs depletion not only induces leukemic cell death mainly through the TNF pathway, but also increases CD20+ leukemic cell infiltration (224). Therefore, a combination targeting TAMs and anti-CD20 mAbs may provide an effective strategy for chronic B lymphocytic leukemia. In conclusion, targeting the TAMs and B cells is also a promising therapeutic strategy for malignant tumors.
5.5 Targeting TAMs and neutrophils in cancer cell therapy
Dual targeting of TAMs and TANs might be an effective anti-tumor therapy strategy. The CSF-1R blockade can significantly deplete TAMs infiltration and stimulate intratumoral type I interferon signaling, which further targets the immunosuppressive TANs and elevate anti-tumor immune response during cisplatin therapy (225). In addition, voets et al. found that selective pan-allele anti-SIRPα antibody ADU-1805 has also been demonstrated to increase macrophages phagocytosis and enhance neutrophils trogocytosis, but not impact T cells activation (226). Furthermore, Ring and colleagues have revealed a new anti-human SIRPα antibody, KWAR23, which can elevate both neutrophils and macrophages’ anti-tumor activity in vitro and in vivo (227). Currently, the optimal treatment for cancers has not yet been defined. Therefore, discovering effective therapeutics targeting both macrophages and neutrophils is important for tumor patients.
IL-23 promotes M2 macrophages and neutrophils infiltration and releases immunosuppressive cytokines, such as TGF-β, IL-10 and VEGF, which reduce CD8+ T cells proliferation and suppress anti-tumor responses (228). Therefore, IL-23 could be a potential target for new therapeutic strategies by regulating macrophages and neutrophils simultaneously. In addition to IL-23, phospholipase D-2 (PLD2) has also been found to play a significant role in tumor progression and metastasis, and PLD was also identified to modulate macrophages and neutrophil signaling pathways (229, 230). In addition, a study found that PLD-specific inhibitors can reduce TAMs and TANs infiltration in tumors and decrease tumor growth in breast cancer, which may implicate PLD as a potential therapeutic target in the treatment of cancers (231). Besides, DKK1 was also found to inhibit TAMs and TANs infiltration in lung metastases (232).
5.6 Targeting TAMs and DCs in cancer cell therapy
Tumor immunotherapy with DCs vaccinations are being extensively investigated in recent years (233). The vaccines aim to enhance DCs immunogenicity and activate cytotoxic T cells (234). Some clinical trials of DCs vaccines have demonstrated that vaccines can significantly elevate the anti-tumor effectors in renal cell carcinoma (235), acute myeloid leukemia (236) and lung cancer (237). In addition, a combination of TAMs depletion and DCs vaccine has been reported to induce durable an-titumor immunity and improve survival than monotherapy in mesothelioma mouse models (238). As mentioned above, blockades of CD47/SIRPα signaling play an important role in regulating macrophages and T cells. It has also been found that anti-CD47 antibody can induce type I interferon expression in DCs and promote antigen presentation to CD8+ T cells (239). Though blockade of CD47/SIRPα signaling promoted macrophages phagocytosing tumor-originated mitochondrial DNA (mtDNA), it inhibited the phonological function of DCs which can reduce mtDNA degradation in DCs and activate DCs’ anti-tumor function by inducing type I interferon (240). Similarly, the CD40 agonist antibody not only reprograms M2 macrophages into M1 macrophages, but also activates DCs (147). Thus, TAMs-targeting therapy combined with DCs vaccines may be an effective strategy for regulating immune responses against tumors.
5.7 Targeting TAMs and NK cells in cancer cell therapy
Numerous studies have confirmed that CD47 is overexpressed in several tumor types, such as myeloma, breast cancer, leiomyosarcoma, and acute lymphocytic cancer (187, 241–243). Furthermore, CD47 is also an important marker for M2-type TAMs, and anti-CD47 therapy can reprogram TAMs to proinflammatory (M1-type) macrophages to kill tumor cells and prevent tumor metastases in human solid tumors (187). Zhang and colleagues first found differential phagocytosis effects of CD47-SIRPα inhibitors on human and mouse macrophage polarization isoforms in vitro (244). Although the polarization shift from the M2 to the M1 phenotype induced by anti-CD47 treatment was not verified in vitro, the in vivo results revealed that the macrophage population changed constantly and polarized towards the M1 subset with a proinflammatory immune response in the TME. Furthermore, the CD47/SIRPα signaling has also been shown to regulate NK cell functions. Overexpression of CD47/SIRPα inhibits NK cell activation and limits NK cell-mediated killing (245). Therefore, blockade of CD47/SIRPα may not only reprogram macrophages polarization, but also enhance the anti-tumor activity of NK cells.
IL-15 can potently enhance peripheral NK cells number and induce NK cells and macrophages activation (246). Furthermore, the interactions between NK cells and macrophages which can increase NK-cell activation are important for NK cells to express FcγRIV to exert cytotoxic effects under the stimulation of IL-15. Combination treatment of IL-15 and rituximab showed a better therapeutic effect which is mediated by both NK cells and macrophages to induce optimal antibody-dependent cellular cytotoxicity (246).
TAMs can be repolarized into the M1 phenotype via activating Toll-like receptor (TLR) and stimulate NK cell activity through expressing immunostimulatory cytokines IL-12 (247). Combination therapy used anti-tumor antibody, IL-12 and anti-PD-1 can induce macrophages repolarized into a M1 phenotype and promote NK cell proliferation, activation and cytotoxicity (248). Reprogrammed macrophages and NK cells trigger lymphocytes’ recruitment into tumors via secreting IFN-γ and facilitate tumor vascular normalization which greatly improved the anti-tumor efficacy. Thus, therapies targeting innate cell activation, such as macrophages and NK cells, may initiate T cell-mediated anti-tumor immune responses.
6 Perspectives and conclusions
Recently, increasing studies have revealed the role of TME in tumorigenesis, progression, and response to treatment (194, 249). Besides, substantial single-cell-related studies have also revealed that TAMs are one cell subgroup of the most abundant components in TME with important functions (7, 250). According to the current understanding, there are mainly two sources of TAMs, including tumor-resident macrophages and bone marrow-derived macrophages which are regularly further polarized into M1 and M2 phenotypes. Tumor-resident macrophages and M2-type TAMs normally play a pro-tumor role, while M1-type TAMs inhibit tumor development (40). Notably, interactions between TAMs and other immune cells in TME significantly influence tumor progression. Many current strategies for cancer treatment influence TME and the TME changes are associated with the therapeutic efficacy. Thus, novel strategies targeting TAMs and other immune cells and their crosstalk will be a promising approach to block or eradicate the tumor.
Currently, CAR-T cell therapies still occupy the major position, but cell therapy modalities that rely on other immune cells have solidified their growth in the past year (179). Given that the important role of TAMs in cancer progression and response to anti-cancer treatment, TAMs-based cell therapy may also be a promising direction. Furthermore, recent studies have revealed that regulating TAMs can significantly inhibit cancer progression and enhance the therapeutic effects of other treatments (186). Recently, CAR-M therapy and targeting strategies regulating TAMs or crosstalk between TAMs and other immune cells have been well studied and achieved encouraging results in cancer treatment.
Conclusively, the effects of TAMs on the initiation and progression of various cancers can be realized in multiple approaches. As the most abundant component in TME, TAMs had strong associations with other immune cells and these interactions exert important effects on cancer progression. Furthermore, targeting TAMs and the interactions with other immune cells can exert antitumor effects. Therefore, TAMs-related immunotherapy is a promising approach to improve therapeutic efficacy for cancer treatment.
Author contributions
CL provided the detection of the manuscript. ML and PJ wrote and edited the manuscript. ML and SW collected associated data. CL and ML drew the figures and tables. CL and JW guided the preparation of this manuscript. All authors read and approved the final manuscript. All authors contributed to the article and approved the submitted version.
Funding
This work was supported by the National Natural Science Foundation of China (82173174 and 81803051 to CL, 82073335 to JW), intramural funding by the Beijing University Third Hospital (BYSY2022044 to CL), Natural Science Foundation of Beijing Municipality (7192220 to CL), Special fund of the National Clinical Key Specialty Construction Program, P. R. China (2021), and Cancer Precision Radiotherapy Spark Program of China International Medical Foundation, Grant/Award Number: 2019-N-11-13.
Conflict of interest
The authors declare that the research was conducted in the absence of any commercial or financial relationships that could be construed as a potential conflict of interest.
Publisher’s note
All claims expressed in this article are solely those of the authors and do not necessarily represent those of their affiliated organizations, or those of the publisher, the editors and the reviewers. Any product that may be evaluated in this article, or claim that may be made by its manufacturer, is not guaranteed or endorsed by the publisher.
Glossary
References
1. Pitt JM, Marabelle A, Eggermont A, Soria JC, Kroemer G, Zitvogel L. Targeting the tumor microenvironment: removing obstruction to anticancer immune responses and immunotherapy. Ann Oncol (2016) 27(8):1482–92. doi: 10.1093/annonc/mdw168
2. Gomes-Santos IL, Amoozgar Z, Kumar AS, Ho WW, Roh K, Talele NP, et al. Exercise training improves tumor control by increasing CD8(+) T-cell infiltration via CXCR3 signaling and sensitizes breast cancer to immune checkpoint blockade. Cancer Immunol Res (2021) 9(7):765–78. doi: 10.1158/2326-6066.CIR-20-0499
3. Greten FR, Grivennikov SI. Inflammation and cancer: Triggers, mechanisms, and consequences. Immunity (2019) 51(1):27–41. doi: 10.1016/j.immuni.2019.06.025
4. Parihar R, Rivas C, Huynh M, Omer B, Lapteva N, Metelitsa LS, et al. NK cells expressing a chimeric activating receptor eliminate MDSCs and rescue impaired CAR-T cell activity against solid tumors. Cancer Immunol Res (2019) 7(3):363–75. doi: 10.1158/2326-6066.CIR-18-0572
5. Nielsen SR, Strobech JE, Horton ER, Jackstadt R, Laitala A, Bravo MC, et al. Suppression of tumor-associated neutrophils by lorlatinib attenuates pancreatic cancer growth and improves treatment with immune checkpoint blockade. Nat Commun (2021) 12(1):3414. doi: 10.1038/s41467-021-23731-7
6. Sathe A, Grimes SM, Lau BT, Chen J, Suarez C, Huang RJ, et al. Single-cell genomic characterization reveals the cellular reprogramming of the gastric tumor microenvironment. Clin Cancer Res (2020) 26(11):2640–53. doi: 10.1158/1078-0432.CCR-19-3231
7. Maynard A, McCoach CE, Rotow JK, Harris L, Haderk F, Kerr DL, et al. Therapy-induced evolution of human lung cancer revealed by single-cell RNA sequencing. Cell (2020) 182(5):1232–51.e22. doi: 10.1016/j.cell.2020.07.017
8. Biffi G, Tuveson DA. Diversity and biology of cancer-associated fibroblasts. Physiol Rev (2021) 101(1):147–76. doi: 10.1152/physrev.00048.2019
9. Friebel E, Kapolou K, Unger S, Nunez NG, Utz S, Rushing EJ, et al. Single-cell mapping of human brain cancer reveals tumor-specific instruction of tissue-invading leukocytes. Cell (2020) 181(7):1626–42.e20. doi: 10.1016/j.cell.2020.04.055
10. Neijssen J, Herberts C, Drijfhout JW, Reits E, Janssen L, Neefjes J. Cross-presentation by intercellular peptide transfer through gap junctions. Nature (2005) 434(7029):83–8. doi: 10.1038/nature03290
11. Boulch M, Cazaux M, Loe-Mie Y, Thibaut R, Corre B, Lemaitre F, et al. A cross-talk between CAR T cell subsets and the tumor microenvironment is essential for sustained cytotoxic activity. Sci Immunol (2021) 6(57):eabd4344. doi: 10.1126/sciimmunol.abd4344
12. Li L, Yang L, Wang L, Wang F, Zhang Z, Li J, et al. Impaired T cell function in malignant pleural effusion is caused by TGF-beta derived predominantly from macrophages. Int J Cancer (2016) 139(10):2261–9. doi: 10.1002/ijc.30289
13. Watkins SC, Salter RD. Functional connectivity between immune cells mediated by tunneling nanotubules. Immunity (2005) 23(3):309–18. doi: 10.1016/j.immuni.2005.08.009
14. Wang S, Li F, Ye T, Wang J, Lyu C, Qing S, et al. Macrophage-tumor chimeric exosomes accumulate in lymph node and tumor to activate the immune response and the tumor microenvironment. Sci Transl Med (2021) 13(615):eabb6981. doi: 10.1126/scitranslmed.abb6981
15. Huse M. Mechanical forces in the immune system. Nat Rev Immunol (2017) 17(11):679–90. doi: 10.1038/nri.2017.74
16. Wilson CA, Tsuchida MA, Allen GM, Barnhart EL, Applegate KT, Yam PT, et al. Myosin II contributes to cell-scale actin network treadmilling through network disassembly. Nature (2010) 465(7296):373–7. doi: 10.1038/nature08994
17. Galon J, Bruni D. Approaches to treat immune hot, altered and cold tumours with combination immunotherapies. Nat Rev Drug Discov (2019) 18(3):197–218. doi: 10.1038/s41573-018-0007-y
18. Liotti F, Kumar N, Prevete N, Marotta M, Sorriento D, Ierano C, et al. PD-1 blockade delays tumor growth by inhibiting an intrinsic SHP2/Ras/MAPK signalling in thyroid cancer cells. J Exp Clin Cancer Res (2021) 40(1):22. doi: 10.1186/s13046-020-01818-1
19. Rotte A. Combination of CTLA-4 and PD-1 blockers for treatment of cancer. J Exp Clin Cancer Res (2019) 38(1):255. doi: 10.1186/s13046-019-1259-z
20. Janjigian YY, Shitara K, Moehler M, Garrido M, Salman P, Shen L, et al. First-line nivolumab plus chemotherapy versus chemotherapy alone for advanced gastric, gastro-oesophageal junction, and oesophageal adenocarcinoma (CheckMate 649): a randomised, open-label, phase 3 trial. Lancet (2021) 398(10294):27–40. doi: 10.1016/S0140-6736(21)00797-2
21. Sun L, Hsu M, Cohen RB, Langer CJ, Mamtani R, Aggarwal C. Association between KRAS variant status and outcomes with first-line immune checkpoint inhibitor-based therapy in patients with advanced non-Small-Cell lung cancer. JAMA Oncol (2021) 7(6):937–9. doi: 10.1001/jamaoncol.2021.0546
22. Williams CB, Yeh ES, Soloff AC. Tumor-associated macrophages: unwitting accomplices in breast cancer malignancy. NPJ Breast Cancer (2016) 2:15025. doi: 10.1038/npjbcancer.2015.25
23. Wolf NK, Kissiov DU, Raulet DH. Roles of natural killer cells in immunity to cancer, and applications to immunotherapy. Nat Rev Immunol (2022) 23(2)90–105. doi: 10.1038/s41577-022-00732-1
24. Duraiswamy J, Kaluza KM, Freeman GJ, Coukos G. Dual blockade of PD-1 and CTLA-4 combined with tumor vaccine effectively restores T-cell rejection function in tumors. Cancer Res (2013) 73(12):3591–603. doi: 10.1158/0008-5472.CAN-12-4100
25. Kumagai S, Togashi Y, Kamada T, Sugiyama E, Nishinakamura H, Takeuchi Y, et al. The PD-1 expression balance between effector and regulatory T cells predicts the clinical efficacy of PD-1 blockade therapies. Nat Immunol (2020) 21(11):1346–58. doi: 10.1038/s41590-020-0769-3
26. Dovedi SJ, Elder MJ, Yang C, Sitnikova SI, Irving L, Hansen A, et al. Design and efficacy of a monovalent bispecific PD-1/CTLA4 antibody that enhances CTLA4 blockade on PD-1(+) activated T cells. Cancer Discov (2021) 11(5):1100–17. doi: 10.1158/2159-8290.CD-20-1445
27. Qiu SQ, Waaijer SJH, Zwager MC, de Vries EGE, van der Vegt B, Schroder CP. Tumor-associated macrophages in breast cancer: Innocent bystander or important player? Cancer Treat Rev (2018) 70:178–89. doi: 10.1016/j.ctrv.2018.08.010
28. Etzerodt A, Moulin M, Doktor TK, Delfini M, Mossadegh-Keller N, Bajenoff M, et al. Tissue-resident macrophages in omentum promote metastatic spread of ovarian cancer. J Exp Med (2020) 217(4):e20191869. doi: 10.1084/jem.20191869
29. Singh S, Lee N, Pedroza DA, Bado IL, Hamor C, Zhang L, et al. Chemotherapy coupled to macrophage inhibition induces T-cell and b-cell infiltration and durable regression in triple-negative breast cancer. Cancer Res (2022) 82(12):2281–97. doi: 10.1158/0008-5472.CAN-21-3714
30. O'Hara MH, O'Reilly EM, Varadhachary G, Wolff RA, Wainberg ZA, Ko AH, et al. CD40 agonistic monoclonal antibody APX005M (sotigalimab) and chemotherapy, with or without nivolumab, for the treatment of metastatic pancreatic adenocarcinoma: an open-label, multicentre, phase 1b study. Lancet Oncol (2021) 22(1):118–31. doi: 10.1016/S1470-2045(20)30532-5
31. Chao MP, Alizadeh AA, Tang C, Myklebust JH, Varghese B, Gill S, et al. Anti-CD47 antibody synergizes with rituximab to promote phagocytosis and eradicate non-Hodgkin lymphoma. Cell (2010) 142(5):699–713. doi: 10.1016/j.cell.2010.07.044
32. Akkari L, Bowman RL, Tessier J, Klemm F, Handgraaf SM, de Groot M, et al. Dynamic changes in glioma macrophage populations after radiotherapy reveal CSF-1R inhibition as a strategy to overcome resistance. Sci Transl Med (2020) 12(552):eaaw7843. doi: 10.1126/scitranslmed.aaw7843
33. Babiker H, Brana I, Mahadevan D, Owonikoko T, Calvo E, Rischin D, et al. Phase I trial of cemiplimab, radiotherapy, cyclophosphamide, and granulocyte macrophage colony-stimulating factor in patients with recurrent or metastatic head and neck squamous cell carcinoma. Oncologist (2021) 26(9):e1508–e13. doi: 10.1002/onco.13810
34. Shi C, Pamer EG. Monocyte recruitment during infection and inflammation. Nat Rev Immunol (2011) 11(11):762–74. doi: 10.1038/nri3070
35. van Furth R, Cohn ZA. The origin and kinetics of mononuclear phagocytes. J Exp Med (1968) 128(3):415–35. doi: 10.1084/jem.128.3.415
36. Geissmann F, Manz MG, Jung S, Sieweke MH, Merad M, Ley K. Development of monocytes, macrophages, and dendritic cells. Science (2010) 327(5966):656–61. doi: 10.1126/science.1178331
37. De Palma M, Lewis CE. Macrophage regulation of tumor responses to anticancer therapies. Cancer Cell (2013) 23(3):277–86. doi: 10.1016/j.ccr.2013.02.013
38. Davies LC, Jenkins SJ, Allen JE, Taylor PR. Tissue-resident macrophages. Nat Immunol (2013) 14(10):986–95. doi: 10.1038/ni.2705
39. Epelman S, Lavine KJ, Randolph GJ. Origin and functions of tissue macrophages. Immunity (2014) 41(1):21–35. doi: 10.1016/j.immuni.2014.06.013
40. Casanova-Acebes M, Dalla E, Leader AM, LeBerichel J, Nikolic J, Morales BM, et al. Tissue-resident macrophages provide a pro-tumorigenic niche to early NSCLC cells. Nature (2021) 595(7868):578–84. doi: 10.1038/s41586-021-03651-8
41. Mantovani A, Sica A, Locati M. Macrophage polarization comes of age. Immunity (2005) 23(4):344–6. doi: 10.1016/j.immuni.2005.10.001
42. Orecchioni M, Ghosheh Y, Pramod AB, Ley K. Macrophage polarization: Different gene signatures in M1(LPS+) vs. classically and M2(LPS-) vs. alternatively activated macrophages. Front Immunol (2019) 10:1084. doi: 10.3389/fimmu.2019.01084
43. Tong Y, Guo YJ, Zhang Q, Bi HX, Kai K, Zhou RY. Combined treatment with dihydrotestosterone and lipopolysaccharide modulates prostate homeostasis by upregulating TNF-alpha from M1 macrophages and promotes proliferation of prostate stromal cells. Asian J Androl (2022) 24(5):513–20. doi: 10.4103/aja2021114
44. Hu W, Lin J, Lian X, Yu F, Liu W, Wu Y, et al. M2a and M2b macrophages predominate in kidney tissues and M2 subpopulations were associated with the severity of disease of IgAN patients. Clin Immunol (2019) 205:8–15. doi: 10.1016/j.clim.2019.05.005
45. Wang Y, Harris DC. Macrophages in renal disease. J Am Soc Nephrol (2011) 22(1):21–7. doi: 10.1681/ASN.2010030269
46. Loegl J, Hiden U, Nussbaumer E, Schliefsteiner C, Cvitic S, Lang I, et al. Hofbauer cells of M2a, M2b and M2c polarization may regulate feto-placental angiogenesis. Reproduction (2016) 152(5):447–55. doi: 10.1530/REP-16-0159
47. Lurier EB, Dalton D, Dampier W, Raman P, Nassiri S, Ferraro NM, et al. Transcriptome analysis of IL-10-stimulated (M2c) macrophages by next-generation sequencing. Immunobiology (2017) 222(7):847–56. doi: 10.1016/j.imbio.2017.02.006
48. Wang Q, Ni H, Lan L, Wei X, Xiang R, Wang Y. Fra-1 protooncogene regulates IL-6 expression in macrophages and promotes the generation of M2d macrophages. Cell Res (2010) 20(6):701–12. doi: 10.1038/cr.2010.52
49. Ferrante CJ, Pinhal-Enfield G, Elson G, Cronstein BN, Hasko G, Outram S, et al. The adenosine-dependent angiogenic switch of macrophages to an M2-like phenotype is independent of interleukin-4 receptor alpha (IL-4Ralpha) signaling. Inflammation (2013) 36(4):921–31. doi: 10.1007/s10753-013-9621-3
50. Cassetta L, Pollard JW. Targeting macrophages: therapeutic approaches in cancer. Nat Rev Drug Discov (2018) 17(12):887–904. doi: 10.1038/nrd.2018.169
51. DeNardo DG, Barreto JB, Andreu P, Vasquez L, Tawfik D, Kolhatkar N, et al. CD4(+) T cells regulate pulmonary metastasis of mammary carcinomas by enhancing protumor properties of macrophages. Cancer Cell (2009) 16(2):91–102. doi: 10.1016/j.ccr.2009.06.018
52. Tang X, Li M, Wu X, Guo T, Zhang L, Tang L, et al. Neoadjuvant PD-1 blockade plus chemotherapy induces a high pathological complete response rate and anti-tumor immune subsets in clinical stage III gastric cancer. Oncoimmunology (2022) 11(1):2135819. doi: 10.1080/2162402X.2022.2135819
53. Lin EY, Nguyen AV, Russell RG, Pollard JW. Colony-stimulating factor 1 promotes progression of mammary tumors to malignancy. J Exp Med (2001) 193(6):727–40. doi: 10.1084/jem.193.6.727
54. Wyckoff J, Wang W, Lin EY, Wang Y, Pixley F, Stanley ER, et al. A paracrine loop between tumor cells and macrophages is required for tumor cell migration in mammary tumors. Cancer Res (2004) 64(19):7022–9. doi: 10.1158/0008-5472.CAN-04-1449
55. Esser S, Lampugnani MG, Corada M, Dejana E, Risau W. Vascular endothelial growth factor induces VE-cadherin tyrosine phosphorylation in endothelial cells. J Cell Sci (1998) 111(Pt 13):1853–65. doi: 10.1242/jcs.111.13.1853
56. Xiao P, Long X, Zhang L, Ye Y, Guo J, Liu P, et al. Neurotensin/IL-8 pathway orchestrates local inflammatory response and tumor invasion by inducing M2 polarization of tumor-associated macrophages and epithelial-mesenchymal transition of hepatocellular carcinoma cells. Oncoimmunology (2018) 7(7):e1440166. doi: 10.1080/2162402X.2018.1440166
57. Chen J, Yao Y, Gong C, Yu F, Su S, Chen J, et al. CCL18 from tumor-associated macrophages promotes breast cancer metastasis via PITPNM3. Cancer Cell (2011) 19(4):541–55. doi: 10.1016/j.ccr.2011.02.006
58. Su S, Liu Q, Chen J, Chen J, Chen F, He C, et al. A positive feedback loop between mesenchymal-like cancer cells and macrophages is essential to breast cancer metastasis. Cancer Cell (2014) 25(5):605–20. doi: 10.1016/j.ccr.2014.03.021
59. Wei C, Yang C, Wang S, Shi D, Zhang C, Lin X, et al. Crosstalk between cancer cells and tumor associated macrophages is required for mesenchymal circulating tumor cell-mediated colorectal cancer metastasis. Mol Cancer (2019) 18(1):64. doi: 10.1186/s12943-019-0976-4
60. Kessenbrock K, Plaks V, Werb Z. Matrix metalloproteinases: regulators of the tumor microenvironment. Cell (2010) 141(1):52–67. doi: 10.1016/j.cell.2010.03.015
61. Noy R, Pollard JW. Tumor-associated macrophages: from mechanisms to therapy. Immunity (2014) 41(1):49–61. doi: 10.1016/j.immuni.2014.06.010
62. Konstantinov AS, Kovaleva OV, Samoilova DV, Shelekhova KV. Role of macrophages in progression of colorectal cancer: a contrast with the traditional paradigm. Int J Clin Exp Pathol (2022) 15(10):403–11.
63. Podlesnaya PA, Kovaleva OV, Petrenko AA, Gratchev AN. Cytotoxic activity of macrophages as a tumor malignancy factor. Bull Exp Biol Med (2022) 174(1):147–51. doi: 10.1007/s10517-022-05664-3
64. Lv C, Li S, Zhao J, Yang P, Yang C. M1 macrophages enhance survival and invasion of oral squamous cell carcinoma by inducing GDF15-mediated ErbB2 phosphorylation. ACS Omega (2022) 7(13):11405–14. doi: 10.1021/acsomega.2c00571
65. Butte MJ, Keir ME, Phamduy TB, Sharpe AH, Freeman GJ. Programmed death-1 ligand 1 interacts specifically with the B7-1 costimulatory molecule to inhibit T cell responses. Immunity (2007) 27(1):111–22. doi: 10.1016/j.immuni.2007.05.016
66. Underhill DM, Bassetti M, Rudensky A, Aderem A. Dynamic interactions of macrophages with T cells during antigen presentation. J Exp Med (1999) 190(12):1909–14. doi: 10.1084/jem.190.12.1909
67. Orlikowsky T, Dannecker GE, Wang Z, Horowitz H, Niethammer D, Hoffmann MK. Activation or destruction of T cells via macrophages. Pathobiology (1999) 67(5-6):298–301. doi: 10.1159/000028084
68. Nalio Ramos R, Missolo-Koussou Y, Gerber-Ferder Y, Bromley CP, Bugatti M, Nunez NG, et al. Tissue-resident FOLR2(+) macrophages associate with CD8(+) T cell infiltration in human breast cancer. Cell (2022) 185(7):1189–207.e25. doi: 10.1016/j.cell.2022.02.021
69. Munn DH, Shafizadeh E, Attwood JT, Bondarev I, Pashine A, Mellor AL. Inhibition of T cell proliferation by macrophage tryptophan catabolism. J Exp Med (1999) 189(9):1363–72. doi: 10.1084/jem.189.9.1363
70. Chow A, Schad S, Green MD, Hellmann MD, Allaj V, Ceglia N, et al. Tim-4(+) cavity-resident macrophages impair anti-tumor CD8(+) T cell immunity. Cancer Cell (2021) 39(7):973–88.e9. doi: 10.1016/j.ccell.2021.05.006
71. Cianciaruso C, Beltraminelli T, Duval F, Nassiri S, Hamelin R, Mozes A, et al. Molecular profiling and functional analysis of macrophage-derived tumor extracellular vesicles. Cell Rep (2019) 27(10):3062–80.e11. doi: 10.1016/j.celrep.2019.05.008
72. Pu J, Xu Z, Nian J, Fang Q, Yang M, Huang Y, et al. M2 macrophage-derived extracellular vesicles facilitate CD8+T cell exhaustion in hepatocellular carcinoma via the miR-21-5p/YOD1/YAP/beta-catenin pathway. Cell Death Discov (2021) 7(1):182. doi: 10.1038/s41420-021-00556-3
73. Kersten K, Hu KH, Combes AJ, Samad B, Harwin T, Ray A, et al. Spatiotemporal co-dependency between macrophages and exhausted CD8(+) T cells in cancer. Cancer Cell (2022) 40(6):624–38.e9. doi: 10.1016/j.ccell.2022.05.004
74. Plitas G, Rudensky AY. Regulatory T cells: Differentiation and function. Cancer Immunol Res (2016) 4(9):721–5. doi: 10.1158/2326-6066.CIR-16-0193
75. Proto JD, Doran AC, Gusarova G, Yurdagul A Jr., Sozen E, Subramanian M, et al. Regulatory T cells promote macrophage efferocytosis during inflammation resolution. Immunity (2018) 49(4):666–77.e6. doi: 10.1016/j.immuni.2018.07.015
76. Liu G, Ma H, Qiu L, Li L, Cao Y, Ma J, et al. Phenotypic and functional switch of macrophages induced by regulatory CD4+CD25+ T cells in mice. Immunol Cell Biol (2011) 89(1):130–42. doi: 10.1038/icb.2010.70
77. Hu X, Liu G, Hou Y, Shi J, Zhu L, Jin D, et al. Induction of M2-like macrophages in recipient NOD-scid mice by allogeneic donor CD4(+)CD25(+) regulatory T cells. Cell Mol Immunol (2012) 9(6):464–72. doi: 10.1038/cmi.2012.47
78. Li J, Kim SY, Lainez NM, Coss D, Nair MG. Macrophage-regulatory T cell interactions promote type 2 immune homeostasis through resistin-like molecule alpha. Front Immunol (2021) 12:710406. doi: 10.3389/fimmu.2021.710406
79. Kraaij MD, Savage ND, van der Kooij SW, Koekkoek K, Wang J, van den Berg JM, et al. Induction of regulatory T cells by macrophages is dependent on production of reactive oxygen species. Proc Natl Acad Sci U.S.A. (2010) 107(41):17686–91. doi: 10.1073/pnas.1012016107
80. Gao Q, Qiu SJ, Fan J, Zhou J, Wang XY, Xiao YS, et al. Intratumoral balance of regulatory and cytotoxic T cells is associated with prognosis of hepatocellular carcinoma after resection. J Clin Oncol (2007) 25(18):2586–93. doi: 10.1200/JCO.2006.09.4565
81. Clark NM, Martinez LM, Murdock S, deLigio JT, Olex AL, Effi C, et al. Regulatory T cells support breast cancer progression by opposing IFN-gamma-Dependent functional reprogramming of myeloid cells. Cell Rep (2020) 33(10):108482. doi: 10.1016/j.celrep.2020.108482
82. Zheng Y, Chen Z, Han Y, Han L, Zou X, Zhou B, et al. Immune suppressive landscape in the human esophageal squamous cell carcinoma microenvironment. Nat Commun (2020) 11(1):6268. doi: 10.1038/s41467-020-20019-0
83. Erlandsson A, Carlsson J, Lundholm M, Falt A, Andersson SO, Andren O, et al. M2 macrophages and regulatory T cells in lethal prostate cancer. Prostate (2019) 79(4):363–9. doi: 10.1002/pros.23742
84. Kos K, Salvagno C, Wellenstein MD, Aslam MA, Meijer DA, Hau CS, et al. Tumor-associated macrophages promote intratumoral conversion of conventional CD4(+) T cells into regulatory T cells via PD-1 signalling. Oncoimmunology (2022) 11(1):2063225. doi: 10.1080/2162402X.2022.2063225
85. Ma X, Gao Y, Chen Y, Liu J, Yang C, Bao C, et al. M2-type macrophages induce tregs generation by activating the TGF-beta/Smad signalling pathway to promote colorectal cancer development. Onco Targets Ther (2021) 14:5391–402. doi: 10.2147/OTT.S336548
86. Zhou J, Li X, Wu X, Zhang T, Zhu Q, Wang X, et al. Exosomes released from tumor-associated macrophages transfer miRNAs that induce a Treg/Th17 cell imbalance in epithelial ovarian cancer. Cancer Immunol Res (2018) 6(12):1578–92. doi: 10.1158/2326-6066.CIR-17-0479
87. Liu C, Chikina M, Deshpande R, Menk AV, Wang T, Tabib T, et al. Treg cells promote the SREBP1-dependent metabolic fitness of tumor-promoting macrophages via repression of CD8(+) T cell-derived interferon-gamma. Immunity (2019) 51(2):381–97.e6. doi: 10.1016/j.immuni.2019.06.017
88. Weichselbaum RR, Liang H, Deng L, Fu YX. Radiotherapy and immunotherapy: a beneficial liaison? Nat Rev Clin Oncol (2017) 14(6):365–79. doi: 10.1038/nrclinonc.2016.211
89. Mondini M, Loyher PL, Hamon P, Gerbe de Thore M, Laviron M, Berthelot K, et al. CCR2-dependent recruitment of tregs and monocytes following radiotherapy is associated with TNFalpha-mediated resistance. Cancer Immunol Res (2019) 7(3):376–87. doi: 10.1158/2326-6066.CIR-18-0633
90. Goyal L, Shi L, Liu LY, Fece de la Cruz F, Lennerz JK, Raghavan S, et al. TAS-120 overcomes resistance to ATP-competitive FGFR inhibitors in patients with FGFR2 fusion-positive intrahepatic cholangiocarcinoma. Cancer Discov (2019) 9(8):1064–79. doi: 10.1158/2159-8290.CD-19-0182
91. Andersen LB, Norgaard M, Rasmussen M, Fredsoe J, Borre M, Ulhoi BP, et al. Immune cell analyses of the tumor microenvironment in prostate cancer highlight infiltrating regulatory T cells and macrophages as adverse prognostic factors. J Pathol (2021) 255(2):155–65. doi: 10.1002/path.5757
92. Ye L, Zhang T, Kang Z, Guo G, Sun Y, Lin K, et al. Tumor-infiltrating immune cells act as a marker for prognosis in colorectal cancer. Front Immunol (2019) 10:2368. doi: 10.3389/fimmu.2019.02368
93. Zhou J, Ding T, Pan W, Zhu LY, Li L, Zheng L. Increased intratumoral regulatory T cells are related to intratumoral macrophages and poor prognosis in hepatocellular carcinoma patients. Int J Cancer (2009) 125(7):1640–8. doi: 10.1002/ijc.24556
94. Chen Y, McAndrews KM, Kalluri R. Clinical and therapeutic relevance of cancer-associated fibroblasts. Nat Rev Clin Oncol (2021) 18(12):792–804. doi: 10.1038/s41571-021-00546-5
95. Raz Y, Cohen N, Shani O, Bell RE, Novitskiy SV, Abramovitz L, et al. Bone marrow-derived fibroblasts are a functionally distinct stromal cell population in breast cancer. J Exp Med (2018) 215(12):3075–93. doi: 10.1084/jem.20180818
96. Yin C, Evason KJ, Asahina K, Stainier DY. Hepatic stellate cells in liver development, regeneration, and cancer. J Clin Invest (2013) 123(5):1902–10. doi: 10.1172/JCI66369
97. Bachem MG, Schneider E, Gross H, Weidenbach H, Schmid RM, Menke A, et al. Identification, culture, and characterization of pancreatic stellate cells in rats and humans. Gastroenterology (1998) 115(2):421–32. doi: 10.1016/s0016-5085(98)70209-4
98. Bochet L, Lehuede C, Dauvillier S, Wang YY, Dirat B, Laurent V, et al. Adipocyte-derived fibroblasts promote tumor progression and contribute to the desmoplastic reaction in breast cancer. Cancer Res (2013) 73(18):5657–68. doi: 10.1158/0008-5472.CAN-13-0530
99. Zeisberg EM, Potenta S, Xie L, Zeisberg M, Kalluri R. Discovery of endothelial to mesenchymal transition as a source for carcinoma-associated fibroblasts. Cancer Res (2007) 67(21):10123–8. doi: 10.1158/0008-5472.CAN-07-3127
100. Kalluri R. The biology and function of fibroblasts in cancer. Nat Rev Cancer (2016) 16(9):582–98. doi: 10.1038/nrc.2016.73
101. Sahai E, Astsaturov I, Cukierman E, DeNardo DG, Egeblad M, Evans RM, et al. A framework for advancing our understanding of cancer-associated fibroblasts. Nat Rev Cancer (2020) 20(3):174–86. doi: 10.1038/s41568-019-0238-1
102. Pelon F, Bourachot B, Kieffer Y, Magagna I, Mermet-Meillon F, Bonnet I, et al. Cancer-associated fibroblast heterogeneity in axillary lymph nodes drives metastases in breast cancer through complementary mechanisms. Nat Commun (2020) 11(1):404. doi: 10.1038/s41467-019-14134-w
103. Su S, Chen J, Yao H, Liu J, Yu S, Lao L, et al. CD10(+)GPR77(+) cancer-associated fibroblasts promote cancer formation and chemoresistance by sustaining cancer stemness. Cell (2018) 172(4):841–56.e16. doi: 10.1016/j.cell.2018.01.009
104. Kobayashi H, Gieniec KA, Lannagan TRM, Wang T, Asai N, Mizutani Y, et al. The origin and contribution of cancer-associated fibroblasts in colorectal carcinogenesis. Gastroenterology (2022) 162(3):890–906. doi: 10.1053/j.gastro.2021.11.037
105. Ozdemir BC, Pentcheva-Hoang T, Carstens JL, Zheng X, Wu CC, Simpson TR, et al. Depletion of carcinoma-associated fibroblasts and fibrosis induces immunosuppression and accelerates pancreas cancer with reduced survival. Cancer Cell (2014) 25(6):719–34. doi: 10.1016/j.ccr.2014.04.005
106. Herrera M, Herrera A, Dominguez G, Silva J, Garcia V, Garcia JM, et al. Cancer-associated fibroblast and M2 macrophage markers together predict outcome in colorectal cancer patients. Cancer Sci (2013) 104(4):437–44. doi: 10.1111/cas.12096
107. Mao X, Xu J, Wang W, Liang C, Hua J, Liu J, et al. Crosstalk between cancer-associated fibroblasts and immune cells in the tumor microenvironment: new findings and future perspectives. Mol Cancer (2021) 20(1):131. doi: 10.1186/s12943-021-01428-1
108. Zhang R, Qi F, Zhao F, Li G, Shao S, Zhang X, et al. Cancer-associated fibroblasts enhance tumor-associated macrophages enrichment and suppress NK cells function in colorectal cancer. Cell Death Dis (2019) 10(4):273. doi: 10.1038/s41419-019-1435-2
109. Chen S, Morine Y, Tokuda K, Yamada S, Saito Y, Nishi M, et al. Cancerassociated fibroblastinduced M2polarized macrophages promote hepatocellular carcinoma progression via the plasminogen activator inhibitor1 pathway. Int J Oncol (2021) 59(2):59. doi: 10.3892/ijo.2021.5239
110. Cho H, Seo Y, Loke KM, Kim SW, Oh SM, Kim JH, et al. Cancer-stimulated CAFs enhance monocyte differentiation and protumoral TAM activation via IL6 and GM-CSF secretion. Clin Cancer Res (2018) 24(21):5407–21. doi: 10.1158/1078-0432.CCR-18-0125
111. Ksiazkiewicz M, Gottfried E, Kreutz M, Mack M, Hofstaedter F, Kunz-Schughart LA. Importance of CCL2-CCR2A/2B signaling for monocyte migration into spheroids of breast cancer-derived fibroblasts. Immunobiology (2010) 215(9-10):737–47. doi: 10.1016/j.imbio.2010.05.019
112. Garcia Garcia CJ, Huang Y, Fuentes NR, Turner MC, Monberg ME, Lin D, et al. Stromal HIF2 regulates immune suppression in the pancreatic cancer microenvironment. Gastroenterology (2022) 162(7)2018–31. doi: 10.1053/j.gastro.2022.02.024
113. Gok Yavuz B, Gunaydin G, Gedik ME, Kosemehmetoglu K, Karakoc D, Ozgur F, et al. Cancer associated fibroblasts sculpt tumour microenvironment by recruiting monocytes and inducing immunosuppressive PD-1(+) TAMs. Sci Rep (2019) 9(1):3172. doi: 10.1038/s41598-019-39553-z
114. Gordon SR, Maute RL, Dulken BW, Hutter G, George BM, McCracken MN, et al. PD-1 expression by tumour-associated macrophages inhibits phagocytosis and tumour immunity. Nature (2017) 545(7655):495–9. doi: 10.1038/nature22396
115. Comito G, Giannoni E, Segura CP, Barcellos-de-Souza P, Raspollini MR, Baroni G, et al. Cancer-associated fibroblasts and M2-polarized macrophages synergize during prostate carcinoma progression. Oncogene (2014) 33(19):2423–31. doi: 10.1038/onc.2013.191
116. Ueshima E, Fujimori M, Kodama H, Felsen D, Chen J, Durack JC, et al. Macrophage-secreted TGF-beta1 contributes to fibroblast activation and ureteral stricture after ablation injury. Am J Physiol Renal Physiol (2019) 317(7):F52–64. doi: 10.1152/ajprenal.00260.2018
117. Patil NS, Nabet BY, Muller S, Koeppen H, Zou W, Giltnane J, et al. Intratumoral plasma cells predict outcomes to PD-L1 blockade in non-small cell lung cancer. Cancer Cell (2022) 40(3):289–300.e4. doi: 10.1016/j.ccell.2022.02.002
118. Batista FD, Harwood NE. The who, how and where of antigen presentation to b cells. Nat Rev Immunol (2009) 9(1):15–27. doi: 10.1038/nri2454
119. Szakal AK, Holmes KL, Tew JG. Transport of immune complexes from the subcapsular sinus to lymph node follicles on the surface of nonphagocytic cells, including cells with dendritic morphology. J Immunol (1983) 131(4):1714–27. doi: 10.4049/jimmunol.131.4.1714
120. Farr AG, Cho Y, De Bruyn PP. The structure of the sinus wall of the lymph node relative to its endocytic properties and transmural cell passage. Am J Anat (1980) 157(3):265–84. doi: 10.1002/aja.1001570304
121. Karlsson MC, Guinamard R, Bolland S, Sankala M, Steinman RM, Ravetch JV. Macrophages control the retention and trafficking of b lymphocytes in the splenic marginal zone. J Exp Med (2003) 198(2):333–40. doi: 10.1084/jem.20030684
122. Audzevich T, Bashford-Rogers R, Mabbott NA, Frampton D, Freeman TC, Potocnik A, et al. Pre/pro-b cells generate macrophage populations during homeostasis and inflammation. Proc Natl Acad Sci U.S.A. (2017) 114(20):E3954–E63. doi: 10.1073/pnas.1616417114
123. Almeida SR, Aroeira LS, Frymuller E, Dias MA, Bogsan CS, Lopes JD, et al. Mouse b-1 cell-derived mononuclear phagocyte, a novel cellular component of acute non-specific inflammatory exudate. Int Immunol (2001) 13(9):1193–201. doi: 10.1093/intimm/13.9.1193
124. Craxton A, Magaletti D, Ryan EJ, Clark EA. Macrophage- and dendritic cell–dependent regulation of human b-cell proliferation requires the TNF family ligand BAFF. Blood (2003) 101(11):4464–71. doi: 10.1182/blood-2002-10-3123
125. Thies FG, Laurindo MF, Perez EC, Novaes e Brito RR, Mariano M, Popi AF. Cross talk between peritoneal macrophages and b-1 cells in vitro. PloS One (2013) 8(5):e62805. doi: 10.1371/journal.pone.0062805
126. Wong SC, Puaux AL, Chittezhath M, Shalova I, Kajiji TS, Wang X, et al. Macrophage polarization to a unique phenotype driven by b cells. Eur J Immunol (2010) 40(8):2296–307. doi: 10.1002/eji.200940288
127. Andreu P, Johansson M, Affara NI, Pucci F, Tan T, Junankar S, et al. FcRgamma activation regulates inflammation-associated squamous carcinogenesis. Cancer Cell (2010) 17(2):121–34. doi: 10.1016/j.ccr.2009.12.019
128. Sallusto F, Baggiolini M. Chemokines and leukocyte traffic. Nat Immunol (2008) 9(9):949–52. doi: 10.1038/ni.f.214
129. Liu RX, Wei Y, Zeng QH, Chan KW, Xiao X, Zhao XY, et al. Chemokine (C-X-C motif) receptor 3-positive b cells link interleukin-17 inflammation to protumorigenic macrophage polarization in human hepatocellular carcinoma. Hepatology (2015) 62(6):1779–90. doi: 10.1002/hep.28020
130. Mantovani A, Cassatella MA, Costantini C, Jaillon S. Neutrophils in the activation and regulation of innate and adaptive immunity. Nat Rev Immunol (2011) 11(8):519–31. doi: 10.1038/nri3024
131. Fridlender ZG, Sun J, Kim S, Kapoor V, Cheng G, Ling L, et al. Polarization of tumor-associated neutrophil phenotype by TGF-beta: "N1" versus "N2" TAN. Cancer Cell (2009) 16(3):183–94. doi: 10.1016/j.ccr.2009.06.017
132. Kumar V, Sharma A. Neutrophils: Cinderella of innate immune system. Int Immunopharmacol (2010) 10(11):1325–34. doi: 10.1016/j.intimp.2010.08.012
133. Kersten K, Coffelt SB, Hoogstraat M, Verstegen NJM, Vrijland K, Ciampricotti M, et al. Mammary tumor-derived CCL2 enhances pro-metastatic systemic inflammation through upregulation of IL1beta in tumor-associated macrophages. Oncoimmunology (2017) 6(8):e1334744. doi: 10.1080/2162402X.2017.1334744
134. Coffelt SB, Kersten K, Doornebal CW, Weiden J, Vrijland K, Hau CS, et al. IL-17-producing gammadelta T cells and neutrophils conspire to promote breast cancer metastasis. Nature (2015) 522(7556):345–8. doi: 10.1038/nature14282
135. Zhou SL, Zhou ZJ, Hu ZQ, Huang XW, Wang Z, Chen EB, et al. Tumor-associated neutrophils recruit macrophages and T-regulatory cells to promote progression of hepatocellular carcinoma and resistance to sorafenib. Gastroenterology (2016) 150(7):1646–58.e17. doi: 10.1053/j.gastro.2016.02.040
136. Deryugina EI, Zajac E, Juncker-Jensen A, Kupriyanova TA, Welter L, Quigley JP. Tissue-infiltrating neutrophils constitute the major in vivo source of angiogenesis-inducing MMP-9 in the tumor microenvironment. Neoplasia (2014) 16(10):771–88. doi: 10.1016/j.neo.2014.08.013
137. Harimoto N, Hoshino K, Muranushi R, Hagiwara K, Yamanaka T, Ishii N, et al. Prognostic significance of neutrophil-lymphocyte ratio in resectable pancreatic neuroendocrine tumors with special reference to tumor-associated macrophages. Pancreatology (2019) 19(6):897–902. doi: 10.1016/j.pan.2019.08.003
138. Yang Z, Shi J, Xie J, Wang Y, Sun J, Liu T, et al. Large-Scale generation of functional mRNA-encapsulating exosomes via cellular nanoporation. Nat BioMed Eng (2020) 4(1):69–83. doi: 10.1038/s41551-019-0485-1
139. Zhou D, Liang J, Xu LI, He F, Zhou Z, Zhang Y, et al. Derived neutrophil to lymphocyte ratio predicts prognosis for patients with HBV-associated hepatocellular carcinoma following transarterial chemoembolization. Oncol Lett (2016) 11(5):2987–94. doi: 10.3892/ol.2016.4359
140. Huang X, Pan Y, Ma J, Kang Z, Xu X, Zhu Y, et al. Prognostic significance of the infiltration of CD163(+) macrophages combined with CD66b(+) neutrophils in gastric cancer. Cancer Med (2018) 7(5):1731–41. doi: 10.1002/cam4.1420
141. Kelly B, O'Neill LA. Metabolic reprogramming in macrophages and dendritic cells in innate immunity. Cell Res (2015) 25(7):771–84. doi: 10.1038/cr.2015.68
142. Grabowska J, Lopez-Venegas MA, Affandi AJ, den Haan JMM. CD169(+) macrophages capture and dendritic cells instruct: The interplay of the gatekeeper and the general of the immune system. Front Immunol (2018) 9:2472. doi: 10.3389/fimmu.2018.02472
143. Savina A, Amigorena S. Phagocytosis and antigen presentation in dendritic cells. Immunol Rev (2007) 219:143–56. doi: 10.1111/j.1600-065X.2007.00552.x
144. Janeway CA Jr., Medzhitov R. Innate immune recognition. Annu Rev Immunol (2002) 20:197–216. doi: 10.1146/annurev.immunol.20.083001.084359
145. Hoebe K, Janssen E, Beutler B. The interface between innate and adaptive immunity. Nat Immunol (2004) 5(10):971–4. doi: 10.1038/ni1004-971
146. Guilliams M, Bruhns P, Saeys Y, Hammad H, Lambrecht BN. The function of fcgamma receptors in dendritic cells and macrophages. Nat Rev Immunol (2014) 14(2):94–108. doi: 10.1038/nri3582
147. Zhang L, Li Z, Skrzypczynska KM, Fang Q, Zhang W, O'Brien SA, et al. Single-cell analyses inform mechanisms of myeloid-targeted therapies in colon cancer. Cell (2020) 181(2):442–59.e29. doi: 10.1016/j.cell.2020.03.048
148. Sabado RL, Balan S, Bhardwaj N. Dendritic cell-based immunotherapy. Cell Res (2017) 27(1):74–95. doi: 10.1038/cr.2016.157
149. Gabrilovich DI, Chen HL, Girgis KR, Cunningham HT, Meny GM, Nadaf S, et al. Production of vascular endothelial growth factor by human tumors inhibits the functional maturation of dendritic cells. Nat Med (1996) 2(10):1096–103. doi: 10.1038/nm1096-1096
150. Menetrier-Caux C, Montmain G, Dieu MC, Bain C, Favrot MC, Caux C, et al. Inhibition of the differentiation of dendritic cells from CD34(+) progenitors by tumor cells: role of interleukin-6 and macrophage colony-stimulating factor. Blood (1998) 92(12):4778–91. doi: 10.1182/blood.V92.12.4778.424k14_4778_4791
151. Song S, Wang Y, Wang J, Lian W, Liu S, Zhang Z, et al. Tumour-derived IL-10 within tumour microenvironment represses the antitumour immunity of Socs1-silenced and sustained antigen expressing DCs. Eur J Cancer (2012) 48(14):2252–9. doi: 10.1016/j.ejca.2011.12.009
152. Ruffell B, Chang-Strachan D, Chan V, Rosenbusch A, Ho CM, Pryer N, et al. Macrophage IL-10 blocks CD8+ T cell-dependent responses to chemotherapy by suppressing IL-12 expression in intratumoral dendritic cells. Cancer Cell (2014) 26(5):623–37. doi: 10.1016/j.ccell.2014.09.006
153. Nam EH, Park SR, Kim PH. TGF-beta1 induces mouse dendritic cells to express VEGF and its receptor (Flt-1) under hypoxic conditions. Exp Mol Med (2010) 42(9):606–13. doi: 10.3858/emm.2010.42.9.059
154. Hawiger D, Inaba K, Dorsett Y, Guo M, Mahnke K, Rivera M, et al. Dendritic cells induce peripheral T cell unresponsiveness under steady state conditions in vivo. J Exp Med (2001) 194(6):769–79. doi: 10.1084/jem.194.6.769
155. Di Virgilio F, Sarti AC, Falzoni S, De Marchi E, Adinolfi E. Extracellular ATP and P2 purinergic signalling in the tumour microenvironment. Nat Rev Cancer (2018) 18(10):601–18. doi: 10.1038/s41568-018-0037-0
156. Hayashi K, Nikolos F, Lee YC, Jain A, Tsouko E, Gao H, et al. Tipping the immunostimulatory and inhibitory DAMP balance to harness immunogenic cell death. Nat Commun (2020) 11(1):6299. doi: 10.1038/s41467-020-19970-9
157. Vultaggio-Poma V, Sarti AC, Di Virgilio F. Extracellular ATP: A feasible target for cancer therapy. Cells (2020) 9(11):2496. doi: 10.3390/cells9112496
158. Ghiringhelli F, Apetoh L, Tesniere A, Aymeric L, Ma Y, Ortiz C, et al. Activation of the NLRP3 inflammasome in dendritic cells induces IL-1beta-dependent adaptive immunity against tumors. Nat Med (2009) 15(10):1170–8. doi: 10.1038/nm.2028
159. Korbelik M, Hamblin MR. The impact of macrophage-cancer cell interaction on the efficacy of photodynamic therapy. Photochem Photobiol Sci (2015) 14(8):1403–9. doi: 10.1039/c4pp00451e
160. Saez PJ, Vargas P, Shoji KF, Harcha PA, Lennon-Dumenil AM, Saez JC. ATP promotes the fast migration of dendritic cells through the activity of pannexin 1 channels and P2X7 receptors. Sci Signal (2017) 10(506):eaah7107. doi: 10.1126/scisignal.aah7107
161. Locovei S, Wang J, Dahl G. Activation of pannexin 1 channels by ATP through P2Y receptors and by cytoplasmic calcium. FEBS Lett (2006) 580(1):239–44. doi: 10.1016/j.febslet.2005.12.004
162. Sang Q, Zhang Z, Shi J, Sun X, Li B, Yan Z, et al. A pannexin 1 channelopathy causes human oocyte death. Sci Transl Med (2019) 11(485):eaav8731. doi: 10.1126/scitranslmed.aav8731
163. Pelegrin P, Barroso-Gutierrez C, Surprenant A. P2X7 receptor differentially couples to distinct release pathways for IL-1beta in mouse macrophage. J Immunol (2008) 180(11):7147–57. doi: 10.4049/jimmunol.180.11.7147
164. Zhou Y, Yang D, Yang Q, Lv X, Huang W, Zhou Z, et al. Single-cell RNA landscape of intratumoral heterogeneity and immunosuppressive microenvironment in advanced osteosarcoma. Nat Commun (2020) 11(1):6322. doi: 10.1038/s41467-020-20059-6
165. Smyth MJ, Hayakawa Y, Takeda K, Yagita H. New aspects of natural-killer-cell surveillance and therapy of cancer. Nat Rev Cancer (2002) 2(11):850–61. doi: 10.1038/nrc928
166. Deng G, Sun Z, Li S, Peng X, Li W, Zhou L, et al. Cell-membrane immunotherapy based on natural killer cell membrane coated nanoparticles for the effective inhibition of primary and abscopal tumor growth. ACS Nano (2018) 12(12):12096–108. doi: 10.1021/acsnano.8b05292
167. Mattiola I, Pesant M, Tentorio PF, Molgora M, Marcenaro E, Lugli E, et al. Priming of human resting NK cells by autologous M1 macrophages via the engagement of IL-1beta, IFN-beta, and IL-15 pathways. J Immunol (2015) 195(6):2818–28. doi: 10.4049/jimmunol.1500325
168. Michel T, Hentges F, Zimmer J. Consequences of the crosstalk between monocytes/macrophages and natural killer cells. Front Immunol (2012) 3:403. doi: 10.3389/fimmu.2012.00403
169. Takayama T, Kamada N, Chinen H, Okamoto S, Kitazume MT, Chang J, et al. Imbalance of NKp44(+)NKp46(-) and NKp44(-)NKp46(+) natural killer cells in the intestinal mucosa of patients with crohn's disease. Gastroenterology (2010) 139(3):882–92, 892.e1-3. doi: 10.1053/j.gastro.2010.05.040
170. Ma PF, Gao CC, Yi J, Zhao JL, Liang SQ, Zhao Y, et al. Cytotherapy with M1-polarized macrophages ameliorates liver fibrosis by modulating immune microenvironment in mice. J Hepatol (2017) 67(4):770–9. doi: 10.1016/j.jhep.2017.05.022
171. Peng LS, Zhang JY, Teng YS, Zhao YL, Wang TT, Mao FY, et al. Tumor-associated Monocytes/Macrophages impair NK-cell function via TGFbeta1 in human gastric cancer. Cancer Immunol Res (2017) 5(3):248–56. doi: 10.1158/2326-6066.CIR-16-0152
172. Bellora F, Castriconi R, Dondero A, Reggiardo G, Moretta L, Mantovani A, et al. The interaction of human natural killer cells with either unpolarized or polarized macrophages results in different functional outcomes. Proc Natl Acad Sci U.S.A. (2010) 107(50):21659–64. doi: 10.1073/pnas.1007654108
173. Paul S, Chhatar S, Mishra A, Lal G. Natural killer T cell activation increases iNOS(+)CD206(-) M1 macrophage and controls the growth of solid tumor. J Immunother Cancer (2019) 7(1):208. doi: 10.1186/s40425-019-0697-7
174. Coquet JM, Chakravarti S, Kyparissoudis K, McNab FW, Pitt LA, McKenzie BS, et al. Diverse cytokine production by NKT cell subsets and identification of an IL-17-producing CD4-NK1.1- NKT cell population. Proc Natl Acad Sci U.S.A. (2008) 105(32):11287–92. doi: 10.1073/pnas.0801631105
175. Michel ML, Keller AC, Paget C, Fujio M, Trottein F, Savage PB, et al. Identification of an IL-17-producing NK1.1(neg) iNKT cell population involved in airway neutrophilia. J Exp Med (2007) 204(5):995–1001. doi: 10.1084/jem.20061551
176. Kim JH, Chung DH. CD1d-restricted IFN-gamma-secreting NKT cells promote immune complex-induced acute lung injury by regulating macrophage-inflammatory protein-1alpha production and activation of macrophages and dendritic cells. J Immunol (2011) 186(3):1432–41. doi: 10.4049/jimmunol.1003140
177. Hodge G, Hodge S, Liu H, Nguyen P, Holmes-Liew CL, Holmes M. Bronchiolitis obliterans syndrome is associated with increased senescent lymphocytes in the small airways. J Heart Lung Transplant (2021) 40(2):108–19. doi: 10.1016/j.healun.2019.12.005
178. Song L, Asgharzadeh S, Salo J, Engell K, Wu HW, Sposto R, et al. Valpha24-invariant NKT cells mediate antitumor activity via killing of tumor-associated macrophages. J Clin Invest (2009) 119(6):1524–36. doi: 10.1172/JCI37869
179. Saez-Ibanez AR, Upadhaya S, Partridge T, Shah M, Correa D, Campbell J. Landscape of cancer cell therapies: trends and real-world data. Nat Rev Drug Discov (2022) 21(9):631–2. doi: 10.1038/d41573-022-00095-1
180. Lin JK, Muffly LS, Spinner MA, Barnes JI, Owens DK, Goldhaber-Fiebert JD. Cost effectiveness of chimeric antigen receptor T-cell therapy in multiply relapsed or refractory adult Large b-cell lymphoma. J Clin Oncol (2019) 37(24):2105–19. doi: 10.1200/JCO.18.02079
181. Luo H, Su J, Sun R, Sun Y, Wang Y, Dong Y, et al. Coexpression of IL7 and CCL21 increases efficacy of CAR-T cells in solid tumors without requiring preconditioned lymphodepletion. Clin Cancer Res (2020) 26(20):5494–505. doi: 10.1158/1078-0432.CCR-20-0777
182. Klichinsky M, Ruella M, Shestova O, Lu XM, Best A, Zeeman M, et al. Human chimeric antigen receptor macrophages for cancer immunotherapy. Nat Biotechnol (2020) 38(8):947–53. doi: 10.1038/s41587-020-0462-y
183. Zhang L, Tian L, Dai X, Yu H, Wang J, Lei A, et al. Pluripotent stem cell-derived CAR-macrophage cells with antigen-dependent anti-cancer cell functions. J Hematol Oncol (2020) 13(1):153. doi: 10.1186/s13045-020-00983-2
184. Pan K, Farrukh H, Chittepu V, Xu H, Pan CX, Zhu Z. CAR race to cancer immunotherapy: from CAR T, CAR NK to CAR macrophage therapy. J Exp Clin Cancer Res (2022) 41(1):119. doi: 10.1186/s13046-022-02327-z
185. Jia X, Li X, Shen Y, Miao J, Liu H, Li G, et al. MiR-16 regulates mouse peritoneal macrophage polarization and affects T-cell activation. J Cell Mol Med (2016) 20(10):1898–907. doi: 10.1111/jcmm.12882
186. Xiong H, Mittman S, Rodriguez R, Moskalenko M, Pacheco-Sanchez P, Yang Y, et al. Anti-PD-L1 treatment results in functional remodeling of the macrophage compartment. Cancer Res (2019) 79(7):1493–506. doi: 10.1158/0008-5472.CAN-18-3208
187. Willingham SB, Volkmer JP, Gentles AJ, Sahoo D, Dalerba P, Mitra SS, et al. The CD47-signal regulatory protein alpha (SIRPa) interaction is a therapeutic target for human solid tumors. Proc Natl Acad Sci U.S.A. (2012) 109(17):6662–7. doi: 10.1073/pnas.1121623109
188. Bian Z, Shi L, Kidder K, Zen K, Garnett-Benson C, Liu Y. Intratumoral SIRPalpha-deficient macrophages activate tumor antigen-specific cytotoxic T cells under radiotherapy. Nat Commun (2021) 12(1):3229. doi: 10.1038/s41467-021-23442-z
189. Tao H, Qian P, Wang F, Yu H, Guo Y. Targeting CD47 enhances the efficacy of anti-PD-1 and CTLA-4 in an esophageal squamous cell cancer preclinical model. Oncol Res (2017) 25(9):1579–87. doi: 10.3727/096504017X14900505020895
190. Sockolosky JT, Dougan M, Ingram JR, Ho CC, Kauke MJ, Almo SC, et al. Durable antitumor responses to CD47 blockade require adaptive immune stimulation. Proc Natl Acad Sci U.S.A. (2016) 113(19):E2646–54. doi: 10.1073/pnas.1604268113
191. Beatty GL, Chiorean EG, Fishman MP, Saboury B, Teitelbaum UR, Sun W, et al. CD40 agonists alter tumor stroma and show efficacy against pancreatic carcinoma in mice and humans. Science (2011) 331(6024):1612–6. doi: 10.1126/science.1198443
192. Diggs LP, Ruf B, Ma C, Heinrich B, Cui L, Zhang Q, et al. CD40-mediated immune cell activation enhances response to anti-PD-1 in murine intrahepatic cholangiocarcinoma. J Hepatol (2021) 74(5):1145–54. doi: 10.1016/j.jhep.2020.11.037
193. Chen L, Ma X, Dang M, Dong H, Hu H, Su X, et al. Simultaneous T cell activation and macrophage polarization to promote potent tumor suppression by iron oxide-embedded Large-pore mesoporous organosilica core-shell nanospheres. Adv Healthc Mater (2019) 8(9):e1900039. doi: 10.1002/adhm.201900039
194. Yang Y, Wang Y. Role of epigenetic regulation in plasticity of tumor immune microenvironment. Front Immunol (2021) 12:640369. doi: 10.3389/fimmu.2021.640369
195. Pennock ND, Martinson HA, Guo Q, Betts CB, Jindal S, Tsujikawa T, et al. Ibuprofen supports macrophage differentiation, T cell recruitment, and tumor suppression in a model of postpartum breast cancer. J Immunother Cancer (2018) 6(1):98. doi: 10.1186/s40425-018-0406-y
196. Zhuang H, Dai X, Zhang X, Mao Z, Huang H. Sophoridine suppresses macrophage-mediated immunosuppression through TLR4/IRF3 pathway and subsequently upregulates CD8(+) T cytotoxic function against gastric cancer. BioMed Pharmacother (2020) 121:109636. doi: 10.1016/j.biopha.2019.109636
197. Rao E, Hou Y, Huang X, Wang L, Wang J, Zheng W, et al. All-trans retinoic acid overcomes solid tumor radioresistance by inducing inflammatory macrophages. Sci Immunol (2021) 6(60):eaba8426. doi: 10.1126/sciimmunol.aba8426
198. Park JH, Riviere I, Gonen M, Wang X, Senechal B, Curran KJ, et al. Long-term follow-up of CD19 CAR therapy in acute lymphoblastic leukemia. N Engl J Med (2018) 378(5):449–59. doi: 10.1056/NEJMoa1709919
199. Rodriguez-Garcia A, Lynn RC, Poussin M, Eiva MA, Shaw LC, O'Connor RS, et al. CAR-T cell-mediated depletion of immunosuppressive tumor-associated macrophages promotes endogenous antitumor immunity and augments adoptive immunotherapy. Nat Commun (2021) 12(1):877. doi: 10.1038/s41467-021-20893-2
200. Liu D, Xu X, Dai Y, Zhao X, Bao S, Ma W, et al. Blockade of AIM2 inflammasome or alpha1-AR ameliorates IL-1beta release and macrophage-mediated immunosuppression induced by CAR-T treatment. J Immunother Cancer (2021) 9(1):e001466. doi: 10.1136/jitc-2020-001466
201. Norelli M, Camisa B, Barbiera G, Falcone L, Purevdorj A, Genua M, et al. Monocyte-derived IL-1 and IL-6 are differentially required for cytokine-release syndrome and neurotoxicity due to CAR T cells. Nat Med (2018) 24(6):739–48. doi: 10.1038/s41591-018-0036-4
202. Maude SL, Frey N, Shaw PA, Aplenc R, Barrett DM, Bunin NJ, et al. Chimeric antigen receptor T cells for sustained remissions in leukemia. N Engl J Med (2014) 371(16):1507–17. doi: 10.1056/NEJMoa1407222
203. Lee DW, Kochenderfer JN, Stetler-Stevenson M, Cui YK, Delbrook C, Feldman SA, et al. T Cells expressing CD19 chimeric antigen receptors for acute lymphoblastic leukaemia in children and young adults: a phase 1 dose-escalation trial. Lancet (2015) 385(9967):517–28. doi: 10.1016/S0140-6736(14)61403-3
204. Turtle CJ, Hanafi LA, Berger C, Gooley TA, Cherian S, Hudecek M, et al. CD19 CAR-T cells of defined CD4+:CD8+ composition in adult b cell ALL patients. J Clin Invest (2016) 126(6):2123–38. doi: 10.1172/JCI85309
205. Brudno JN, Maric I, Hartman SD, Rose JJ, Wang M, Lam N, et al. T Cells genetically modified to express an anti-B-Cell maturation antigen chimeric antigen receptor cause remissions of poor-prognosis relapsed multiple myeloma. J Clin Oncol (2018) 36(22):2267–80. doi: 10.1200/JCO.2018.77.8084
206. Lee DW, Gardner R, Porter DL, Louis CU, Ahmed N, Jensen M, et al. Current concepts in the diagnosis and management of cytokine release syndrome. Blood (2014) 124(2):188–95. doi: 10.1182/blood-2014-05-552729
207. Curiel TJ, Coukos G, Zou L, Alvarez X, Cheng P, Mottram P, et al. Specific recruitment of regulatory T cells in ovarian carcinoma fosters immune privilege and predicts reduced survival. Nat Med (2004) 10(9):942–9. doi: 10.1038/nm1093
208. Telang S, Rasku MA, Clem AL, Carter K, Klarer AC, Badger WR, et al. Phase II trial of the regulatory T cell-depleting agent, denileukin diftitox, in patients with unresectable stage IV melanoma. BMC Cancer (2011) 11:515. doi: 10.1186/1471-2407-11-515
209. Barnett B, Kryczek I, Cheng P, Zou W, Curiel TJ. Regulatory T cells in ovarian cancer: biology and therapeutic potential. Am J Reprod Immunol (2005) 54(6):369–77. doi: 10.1111/j.1600-0897.2005.00330.x
210. Matalonga J, Glaria E, Bresque M, Escande C, Carbo JM, Kiefer K, et al. The nuclear receptor LXR limits bacterial infection of host macrophages through a mechanism that impacts cellular NAD metabolism. Cell Rep (2017) 18(5):1241–55. doi: 10.1016/j.celrep.2017.01.007
211. Pencheva N, Buss CG, Posada J, Merghoub T, Tavazoie SF. Broad-spectrum therapeutic suppression of metastatic melanoma through nuclear hormone receptor activation. Cell (2014) 156(5):986–1001. doi: 10.1016/j.cell.2014.01.038
212. Nelson ER, Wardell SE, Jasper JS, Park S, Suchindran S, Howe MK, et al. 27-hydroxycholesterol links hypercholesterolemia and breast cancer pathophysiology. Science (2013) 342(6162):1094–8. doi: 10.1126/science.1241908
213. Tavazoie MF, Pollack I, Tanqueco R, Ostendorf BN, Reis BS, Gonsalves FC, et al. LXR/ApoE activation restricts innate immune suppression in cancer. Cell (2018) 172(4):825–40.e18. doi: 10.1016/j.cell.2017.12.026
214. Carbo JM, Leon TE, Font-Diaz J, de la Rosa JV, Castrillo A, Picard FR, et al. Pharmacologic activation of LXR alters the expression profile of tumor-associated macrophages and the abundance of regulatory T cells in the tumor microenvironment. Cancer Res (2021) 81(4):968–85. doi: 10.1158/0008-5472.CAN-19-3360
215. Granucci F, Petralia F, Urbano M, Citterio S, Di Tota F, Santambrogio L, et al. The scavenger receptor MARCO mediates cytoskeleton rearrangements in dendritic cells and microglia. Blood (2003) 102(8):2940–7. doi: 10.1182/blood-2002-12-3651
216. La Fleur L, Botling J, He F, Pelicano C, Zhou C, He C, et al. Targeting MARCO and IL37R on immunosuppressive macrophages in lung cancer blocks regulatory T cells and supports cytotoxic lymphocyte function. Cancer Res (2021) 81(4):956–67. doi: 10.1158/0008-5472.CAN-20-1885
217. Goulet CR, Champagne A, Bernard G, Vandal D, Chabaud S, Pouliot F, et al. Cancer-associated fibroblasts induce epithelial-mesenchymal transition of bladder cancer cells through paracrine IL-6 signalling. BMC Cancer (2019) 19(1):137. doi: 10.1186/s12885-019-5353-6
218. Pyonteck SM, Akkari L, Schuhmacher AJ, Bowman RL, Sevenich L, Quail DF, et al. CSF-1R inhibition alters macrophage polarization and blocks glioma progression. Nat Med (2013) 19(10):1264–72. doi: 10.1038/nm.3337
219. Rao R, Han R, Ogurek S, Xue C, Wu LM, Zhang L, et al. Glioblastoma genetic drivers dictate the function of tumor-associated macrophages/microglia and responses to CSF1R inhibition. Neuro Oncol (2022) 24(4):584–97. doi: 10.1093/neuonc/noab228
220. Kumar V, Donthireddy L, Marvel D, Condamine T, Wang F, Lavilla-Alonso S, et al. Cancer-associated fibroblasts neutralize the anti-tumor effect of CSF1 receptor blockade by inducing PMN-MDSC infiltration of tumors. Cancer Cell (2017) 32(5):654–68.e5. doi: 10.1016/j.ccell.2017.10.005
221. Inoue S, Leitner WW, Golding B, Scott D. Inhibitory effects of b cells on antitumor immunity. Cancer Res (2006) 66(15):7741–7. doi: 10.1158/0008-5472.CAN-05-3766
222. Steidl C, Lee T, Shah SP, Farinha P, Han G, Nayar T, et al. Tumor-associated macrophages and survival in classic hodgkin's lymphoma. N Engl J Med (2010) 362(10):875–85. doi: 10.1056/NEJMoa0905680
223. Polk A, Lu Y, Wang T, Seymour E, Bailey NG, Singer JW, et al. Colony-stimulating factor-1 receptor is required for nurse-like cell survival in chronic lymphocytic leukemia. Clin Cancer Res (2016) 22(24):6118–28. doi: 10.1158/1078-0432.CCR-15-3099
224. Galletti G, Scielzo C, Barbaglio F, Rodriguez TV, Riba M, Lazarevic D, et al. Targeting macrophages sensitizes chronic lymphocytic leukemia to apoptosis and inhibits disease progression. Cell Rep (2016) 14(7):1748–60. doi: 10.1016/j.celrep.2016.01.042
225. Salvagno C, Ciampricotti M, Tuit S, Hau CS, van Weverwijk A, Coffelt SB, et al. Therapeutic targeting of macrophages enhances chemotherapy efficacy by unleashing type I interferon response. Nat Cell Biol (2019) 21(4):511–21. doi: 10.1038/s41556-019-0298-1
226. Voets E, Parade M, Lutje Hulsik D, Spijkers S, Janssen W, Rens J, et al. Functional characterization of the selective pan-allele anti-SIRPalpha antibody ADU-1805 that blocks the SIRPalpha-CD47 innate immune checkpoint. J Immunother Cancer (2019) 7(1):340. doi: 10.1186/s40425-019-0772-0
227. Ring NG, Herndler-Brandstetter D, Weiskopf K, Shan L, Volkmer JP, George BM, et al. Anti-SIRPalpha antibody immunotherapy enhances neutrophil and macrophage antitumor activity. Proc Natl Acad Sci U.S.A. (2017) 114(49):E10578–E85. doi: 10.1073/pnas.1710877114
228. Nie W, Yu T, Sang Y, Gao X. Tumor-promoting effect of IL-23 in mammary cancer mediated by infiltration of M2 macrophages and neutrophils in tumor microenvironment. Biochem Biophys Res Commun (2017) 482(4):1400–6. doi: 10.1016/j.bbrc.2016.12.048
229. Hayes TS, Billington CJ, Robinson KA, Sampt ER, Fernandez GA, Gomez-Cambronero J. Binding of GM-CSF to adherent neutrophils activates phospholipase d. Cell Signal (1999) 11(3):195–204. doi: 10.1016/s0898-6568(98)00066-7
230. Meats JE, Steele L, Bowen JG. Identification of phospholipase d (PLD) activity in mouse peritoneal macrophages. Agents Actions (1993) 39 Spec No:C14–6. doi: 10.1007/BF01972706
231. Henkels KM, Muppani NR, Gomez-Cambronero J. PLD-specific small-molecule inhibitors decrease tumor-associated macrophages and neutrophils infiltration in breast tumors and lung and liver metastases. PloS One (2016) 11(11):e0166553. doi: 10.1371/journal.pone.0166553
232. Zhuang X, Zhang H, Li X, Li X, Cong M, Peng F, et al. Differential effects on lung and bone metastasis of breast cancer by wnt signalling inhibitor DKK1. Nat Cell Biol (2017) 19(10):1274–85. doi: 10.1038/ncb3613
233. Palucka K, Banchereau J. Dendritic-cell-based therapeutic cancer vaccines. Immunity (2013) 39(1):38–48. doi: 10.1016/j.immuni.2013.07.004
234. Butterfield LH. Dendritic cells in cancer immunotherapy clinical trials: are we making progress? Front Immunol (2013) 4:454. doi: 10.3389/fimmu.2013.00454
235. Kim JH, Lee Y, Bae YS, Kim WS, Kim K, Im HY, et al. Phase I/II study of immunotherapy using autologous tumor lysate-pulsed dendritic cells in patients with metastatic renal cell carcinoma. Clin Immunol (2007) 125(3):257–67. doi: 10.1016/j.clim.2007.07.014
236. Chevallier P, Saiagh S, Dehame V, Guillaume T, Peterlin P, Bercegeay S, et al. A phase I/II feasibility vaccine study by autologous leukemic apoptotic corpse-pulsed dendritic cells for elderly AML patients. Hum Vaccin Immunother (2021) 17(10):3511–4. doi: 10.1080/21645515.2021.1943991
237. Ding Z, Li Q, Zhang R, Xie L, Shu Y, Gao S, et al. Personalized neoantigen pulsed dendritic cell vaccine for advanced lung cancer. Signal Transduct Target Ther (2021) 6(1):26. doi: 10.1038/s41392-020-00448-5
238. Dammeijer F, Lievense LA, Kaijen-Lambers ME, van Nimwegen M, Bezemer K, Hegmans JP, et al. Depletion of tumor-associated macrophages with a CSF-1R kinase inhibitor enhances antitumor immunity and survival induced by DC immunotherapy. Cancer Immunol Res (2017) 5(7):535–46. doi: 10.1158/2326-6066.CIR-16-0309
239. Tseng D, Volkmer JP, Willingham SB, Contreras-Trujillo H, Fathman JW, Fernhoff NB, et al. Anti-CD47 antibody-mediated phagocytosis of cancer by macrophages primes an effective antitumor T-cell response. Proc Natl Acad Sci U.S.A. (2013) 110(27):11103–8. doi: 10.1073/pnas.1305569110
240. Xu MM, Pu Y, Han D, Shi Y, Cao X, Liang H, et al. Dendritic cells but not macrophages sense tumor mitochondrial DNA for cross-priming through signal regulatory protein alpha signaling. Immunity (2017) 47(2):363–73.e5. doi: 10.1016/j.immuni.2017.07.016
241. Kim D, Wang J, Willingham SB, Martin R, Wernig G, Weissman IL. Anti-CD47 antibodies promote phagocytosis and inhibit the growth of human myeloma cells. Leukemia (2012) 26(12):2538–45. doi: 10.1038/leu.2012.141
242. Edris B, Weiskopf K, Volkmer AK, Volkmer JP, Willingham SB, Contreras-Trujillo H, et al. Antibody therapy targeting the CD47 protein is effective in a model of aggressive metastatic leiomyosarcoma. Proc Natl Acad Sci U.S.A. (2012) 109(17):6656–61. doi: 10.1073/pnas.1121629109
243. Chao MP, Alizadeh AA, Tang C, Jan M, Weissman-Tsukamoto R, Zhao F, et al. Therapeutic antibody targeting of CD47 eliminates human acute lymphoblastic leukemia. Cancer Res (2011) 71(4):1374–84. doi: 10.1158/0008-5472.CAN-10-2238
244. Zhang M, Hutter G, Kahn SA, Azad TD, Gholamin S, Xu CY, et al. Anti-CD47 treatment stimulates phagocytosis of glioblastoma by M1 and M2 polarized macrophages and promotes M1 polarized macrophages In vivo. PloS One (2016) 11(4):e0153550. doi: 10.1371/journal.pone.0153550
245. Deuse T, Hu X, Agbor-Enoh S, Jang MK, Alawi M, Saygi C, et al. The SIRPalpha-CD47 immune checkpoint in NK cells. J Exp Med (2021) 218(3):e20200839. doi: 10.1084/jem.20200839
246. Zhang M, Wen B, Anton OM, Yao Z, Dubois S, Ju W, et al. IL-15 enhanced antibody-dependent cellular cytotoxicity mediated by NK cells and macrophages. Proc Natl Acad Sci U.S.A. (2018) 115(46):E10915–E24. doi: 10.1073/pnas.1811615115
247. Bellora F, Castriconi R, Dondero A, Pessino A, Nencioni A, Liggieri G, et al. TLR activation of tumor-associated macrophages from ovarian cancer patients triggers cytolytic activity of NK cells. Eur J Immunol (2014) 44(6):1814–22. doi: 10.1002/eji.201344130
248. Wang C, Cui A, Bukenya M, Aung A, Pradhan D, Whittaker CA, et al. Reprogramming NK cells and macrophages via combined antibody and cytokine therapy primes tumors for elimination by checkpoint blockade. Cell Rep (2021) 37(8):110021. doi: 10.1016/j.celrep.2021.110021
249. Kim R, An M, Lee H, Mehta A, Heo YJ, Kim KM, et al. Early tumor-immune microenvironmental remodeling and response to frontline fluoropyrimidine and platinum chemotherapy in advanced gastric cancer. Cancer Discov (2021). doi: 10.1158/2159-8290.CD-21-0888
Keywords: macrophages, tumor-associated macrophages, tumor microenvironment, crosstalk, cancer immunotherapy
Citation: Li M, Jiang P, Wei S, Wang J and Li C (2023) The role of macrophages-mediated communications among cell compositions of tumor microenvironment in cancer progression. Front. Immunol. 14:1113312. doi: 10.3389/fimmu.2023.1113312
Received: 01 December 2022; Accepted: 30 January 2023;
Published: 09 February 2023.
Edited by:
Tahseen H. Nasti, Emory University, United StatesReviewed by:
Sakshi M., Emory University, United StatesOlga Kovaleva, Russian Cancer Research Center NN Blokhin, Russia
Copyright © 2023 Li, Jiang, Wei, Wang and Li. This is an open-access article distributed under the terms of the Creative Commons Attribution License (CC BY). The use, distribution or reproduction in other forums is permitted, provided the original author(s) and the copyright owner(s) are credited and that the original publication in this journal is cited, in accordance with accepted academic practice. No use, distribution or reproduction is permitted which does not comply with these terms.
*Correspondence: Chunxiao Li, Y2h1bnhpYW9saUBwa3UuZWR1LmNu; Junjie Wang, anVuamlld2FuZ0Bwa3UuZWR1LmNu
†These authors share first authorship