- 1Department of Breast Surgery, Obstetrics and Gynecology Hospital, Fudan University School of Medicine, Shanghai, China
- 2Center for Immune-Related Diseases at Shanghai Institute of Immunology, Department of Respiratory and Critical Care Medicine of Ruijin Hospital, Shanghai Jiao Tong University School of Medicine, Shanghai, China
- 3Department of Gastrointestinal Surgery, Renji Hospital, Shanghai Jiao Tong University School of Medicine, Shanghai, China
- 4Department of Orthopedics, Zhongshan Hospital, Fudan University School of Medicine, Shanghai, China
- 5Institute of Arthritis Research, Guanghua Integrative Medicine Hospital, Shanghai University of Traditional Chinese Medicine, Shanghai, China
- 6Department of Integrated TCM & Western Medicine at Shanghai Skin Disease Hospital, School of Medicine, Tongji University, Shanghai, China
- 7Department of General Surgery, Huashan Hospital, Fudan University, Shanghai, China
FOXP3+ regulatory T (Treg) cells play critical roles in establishing the immunosuppressive tumour microenvironment, which is achieved and dynamically maintained with the contribution of various stromal and immune cell subsets. However, the dynamics of non-lymphoid FOXP3+ Treg cells and the mutual regulation of Treg cells and other cell types in solid tumour microenvironment remains largely unclear. In this review, we summarize the latest findings on the dynamic connections and reciprocal regulations of non-lymphoid Treg cell subsets in accordance with well-established and new emerging hallmarks of cancer, especially on the immune escape of tumour cells in solid tumours. Our comprehension of the interplay between FOXP3+ Treg cells and key hallmarks of cancer may provide new insights into the development of next-generation engineered T cell-based immune treatments for solid tumours.
Introduction
Tumour is a leading cause of death and a significant barrier to the increasing life expectancy worldwide (1, 2). It remains largely an incurable disease, urging us to explore the mystery of the tumour tissue microenvironment. Although the comprehensive mechanisms for tumour progression are still unclear, we have known for more than one decade that the insufficient anti-tumour immunity is caused by regulatory T (Treg) cell-mediated immunosuppression (3). Hanahan and Weinberg have previously published landmark reviews on The Hallmarks of Cancer to gather and categorize the knowledge of cancer into several hallmarks, leading to a systematic understanding of cancer occurrence and development as well as guiding the research direction in past decades (4, 5). In 2022, Hanahan has added four proposed emerging hallmarks and enabling characteristics, “unlocking phenotypic plasticity,” “nonmutational epigenetic reprogramming,” “polymorphic microbiomes,” and “senescent cells” in addition to the ten well-established ones, including “sustaining proliferative signaling,” “deregulating cellular metabolism,” “resisting cell death,” “genome instability and mutations,” “inducing or accessing vasculature,” “activating invasion and metastasis,” “tumour-promoting inflammation,” “enabling replicative immortality,” “avoiding immune destruction” and “evading growth suppressors” (6). In this review, we are going to discuss the potential connection between non-lymphoid FOXP3+ regulatory T cell dynamics and the new emerging and well-established hallmarks of cancer, especially on the immune escape of solid tumours.
Treg cells, also known as suppressor T cells, are a subpopulation of T cells that modulate the immune system (7). The lineage determining transcription factor, FOXP3 forms a large molecular complex with multiple transcription factors and enzymatic subunits to dynamically regulate the development and function of regulatory T cells (8–14). FOXP3+ Treg cells play essential roles in maintaining immune homeostasis in healthy people (15). However, tumour-infiltrating Treg cells have strong immunosuppressive function, which may promote the immune escape of cancer cells and the occurrence and development of tumours (16, 17). Meanwhile, the tumour-derived factors may also mutually modulate the induction, migration, and immunosuppressive function of FOXP3+ Treg cells (17).
Mechanisms of FOXP3+ Treg cell-mediated immune homeostasis and anti-tumour immunity in solid tumour microenvironment
Tumour progression is not only related to the anabolic metabolism of tumour cells themselves, but also to the extracellular matrix in the tumour microenvironment (TME). Within TME, stromal cells maintain tissue homeostasis which favours the growth of tumours, while Treg cells dominate the formation of immunosuppressive TME, resulting in the failure of launching effective anti-tumour responses (18). Although the ablation of Treg cells can eradicate tumours rapidly, severe autoimmune and inflammatory complications are developed due to the loss of Treg cell function (19). During the development of tumours, Treg cells proliferate and undergo functional maturation, which are promoted by metabolites produced by tumour cells. Therefore, a deep understanding of underlying mechanisms of FOXP3+ Treg cells mediated immune homeostasis and anti-tumour immunity is required for developing more effective anti-tumour immunotherapies.
The main function of Treg cells is to maintain the immune balance and promote tissue homeostasis. In the tumour microenvironment, Treg cells have multiple functions and could suppress the anti-tumour response through several mechanisms. Treg cells express immune inhibitory receptors and ligands such as CTLA-4, PD-1, and PD-L1 (20). In addition, Treg cells can express high-affinity IL-2 receptor subunit CD25, which may deplete the pro-inflammatory factor IL-2 in TME (21). Treg cells also express cell surface ectonucleotidases CD39 and CD73, which degrade extracellular ATP into adenosine, leading to the functional immunosuppression of target cells (22). FOXP3+ Treg cells may also secrete anti-inflammatory factors (TGF-β, IL-10, and IL-35), perforins, and granzymes to inhibit or kill T cells, NK cells, and antigen-presenting cells (23). Blimp1 in Treg cells affects the growth rate of tumours dependent on the expression of Eomesodermin (Eomes), and causes changes in CD45 cells’ type I interferon in TME, resulting in the changes of the downstream angiogenic related genes, MHC I and MHC II molecules, and antigens, thereby altering the activity of tumour immune cells and immunogenicity of the tumour (24).
An updated view of FOXP3+ Treg cells and solid tumour microenvironment
This review will focus on the functional regulation of tumour-infiltrating FOXP3+ Treg cell dynamics in accordance with well-established and new emerging cancer hallmarks in order to provide a more comprehensive understanding of the mutual regulation between FOXP3+ Treg cell dynamics and solid tumour progression (as shown in Figure 1).
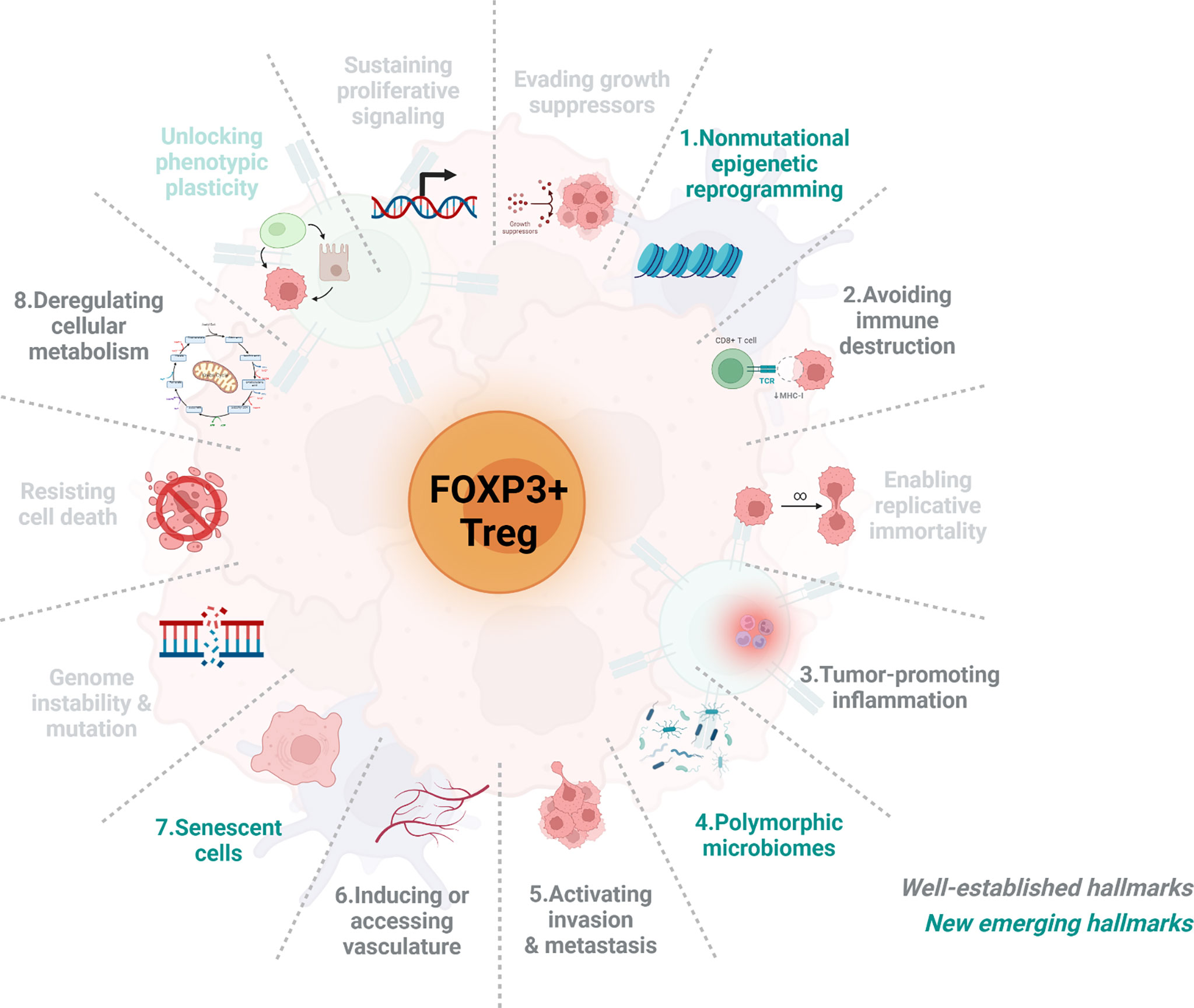
Figure 1 The schematic representation of the associations between FOXP3+ Treg cells and eight cancer hallmarks. Over the past decades, our understanding of cancer has evolved tremendously. Recently, Hanahan and Weinberg have categorized and summarized knowledge of cancers into 14 hallmarks, including 10 well-estlabished hallmarks (grey) and 4 new emerging hallmarks (green). Here we briefly introduce the connection between Treg cells dynamics and the feature of eight either well-established or new emerging cancer hallmarks including 1) nonmutational epigenetic reprogramming, 2) Avoiding immune destruction, 3) tumour-promoting inflammation, 4) polymorphic microbiomes, 5) activating invasion & metastasis, 6) inducing or accessing vasculature, 7) senescent cells, and 8) deregulating cellular metabolism. As very few papers have reported the association of Treg cells with the remaining hallmarks and thus will not be included.
FOXP3+ Treg cells and the new hallmark: Nonmutational epigenetic reprogramming
Douglas Hanahan has proposed “nonmutational epigenetic reprogramming” as one of the emerging hallmarks of cancer (6). It has been reported that epigenetic changes within TME, such as excessive alteration of DNA methylation, histone modification, chromatin accessibility, and posttranslational modification, significantly contribute to the development and progression of malignant tumours (6).
Malignant cells apply epigenetic modifications to dysregulate the expression of certain ligands and affect the immunosuppressive ability of Treg cells. One persuasive example is in lymphoma. Pharmacologic inhibition/blockade of Histone deacetylase (HDAC) 11 enhances the expression of OX40L in Hodgkin lymphoma (HL) cells, and the HDAC inhibitor-induced OX40L inhibits the immunosuppressive function of interleukin 10 (IL-10)-producing Treg cells and alters cytokine secretion of HL cells to favour a Th1- and Th17-type response (25). Moreover, studies have reported that OX40 triggers the inhibition of FOXP3 gene expression and the TGF-β–induced conversion of CD4+ naive T cells into CD4+ CD25+ FOXP3+ Treg cells (26, 27).
Cancer epigenetic reprogram also modulates Treg cell functions via PD-L1 expression. The interaction between PD-1 and PD-L1 negatively impacts the functions of effector and immunosuppressive T cells. Thus, blocking PD-1/PD-L1 may reactivate anti-tumour T cell immunity, thereby inhibiting tumour growth. Both HDAC inhibitors and DNA-methyltransferase-targeted inhibitors may increase PD-L1 expression in various tumours (28–31). Combination of epigenetic modulators with anti-PD-1/PD-L1 antibodies emerges as promising therapeutics for cancer treatment (29, 32–34). Our recent study has found that gallic acid, a small molecule compound found in traditional Chinese medicine, when combined with anti-PD-1 antibody, significantly dampen tumour- infiltrating FOXP3+ Treg cell function by impairing PD-1/PD-L1 signaling and Foxp3 stability in colorectal cancer (CRC) model (35). By inhibiting the inducible expression of PD-L1, the metabolic molecule L-5-hydroxytryptophan could also stimulate anti-tumour immunity (36). In Treg cells, the PD-1/PD-L1 axis inhibits the phosphorylation of ZAP70 and AKT through phosphorylation of SHP2, which are well established in CD8+ T cells (37–39). Interaction between malignant cells and Tregs is mediated in part through PDL1 and PD1 and epigenetic mechanisms modulated PD-L1 expression level (31, 40). The increase of PD-L1 by malignant cells enhances PD-L1 and PD-1 interactions, which might inhibit both effector T cells and Treg cells, suggesting the epigenetic inhibition might affect anti-tumour immune response. Therefore, the balance of PD-1 expressed by effector T cells and Treg cells in TME, might be considered in the combination of PD-1/PD-L1 blockage and epigenetic inhibition (41).
Enhancer of zeste homologue 2 (EZH2) is a histone H3K27 methyltransferase of the polycomb repressor complex 2 (PRC2) (42). Blockade of this epigenetic regulator dramatically represses tumour via a T cell-dependent mechanism. EZH2 inhibition, either pharmacologically or genetically, destabilizes FOXP3 expression in Treg cells and specifically reprograms tumour- infiltrating Treg cells through driving the expression pro-inflammatory genes (e.g., IL-2) while inhibiting key immunosuppressive genes such as IL-10 and TGF-β (43). Treg cell reprogramming toward pro-inflammatory activities is critical for the efficacy of anti-tumour immune responses and enhancing immunotherapy.
FOXP3+ Treg cells and the immune escape of cancer
The induction and recruitment of immunosuppressive Treg cells is one of the critical processes involved in the acquisition of immune escape in cancer. First, cancer cells can establish immunosuppressive microenvironment by recruiting Treg cells into the tumour through multiple mechanisms. Specific combination of chemotactic molecules and their receptors are engaged in this process. CCR8, exhibiting chemotaxis to CCL1 (44), is a selectively upregulated molecule in intratumoural Treg cells (45, 46). In mouse and human tumour tissues, CCR8+ Treg cells account for 30% -80% of total tumour-infiltrating Treg cells, while that accounts for less than 10% in other tissues (47). Increased Helios+ CCR8+ Treg cell frequency in pancreatic ductal adenocarcinoma (PDAC) is associated with an invasive phenotype and poor survival (48). Anti-CCR8 monoclonal antibodies and anti-CCR8 antibody with Fc-dependent ADCC (antibody dependent cellular cytotoxicity) selectively depletes tumour-infiltrating Treg cells due to significantly increased CCR8 expression by the activated Treg cells in TME, resulting in a durable anti-tumour immune response without deleterious autoimmunity and the anti-tumour effects can be synergized with PD-1 blockers (47, 49, 50). CCR4, binds to CCL22 and CCL17, is another crucial chemokine receptor mediating Treg cells trafficking into the TME (51, 52). Increased CCR4 expression is observed in activated Treg cells. Inhibition of CCR4 has been shown to reduce Treg cells accumulation, potentiate anti-tumour immune activity, sensitize tumours to PD-1 blockade and improve survival (53–56). CCL5, activated by cancer FOXP3, is responsible for FOXP3 + Treg cells infiltration in pancreatic ductal adenocarcinoma (57). Moreover, CCR5-dependent Treg cell recruitment is reportedly in colon cancer and melanoma (58, 59). Beyond the traditional chemotactic mediators, recent studies have also demonstrated that the G protein-coupled receptor 15 (GPR15), an unconventional chemokine receptor, directs the infiltration of Treg cells into the colon and subsequently promotes immune evasion of colorectal cancer (60).
Second, Treg cells may also accumulate in tumour to mediate immunosuppression by conversion of conventional CD4 T (Tconv) cells to Treg cells. Specific cytokines and growth factors in TME are capable to initiate this process. Indoleamine 2,3-dioxygenase (IDO) expressed by cancer cells directly amplifies Treg cells by transforming CD4+CD25-T cells to CD4+CD25+ Treg cells (61). Tumour-derived TGF-β, IL-10, and vascular endothelial growth factor (VEGF) promote the expansion of natural Treg (nTreg) cells assisted by antigen-presenting cells (APCs) in a tolerogenic manner (62). Tumour-infiltrating Treg cells directly promote tumour immune evasion in multiple ways. One of the most important mechanisms is the expression of checkpoint suppressor molecules such as CTLA-4, PD-1, TIM-3, LAG-3, and TIGIT (17, 63). Treg cells function to bind and block corresponding ligands on APCs through these co-inhibitory receptor molecules, thereby inhibiting the maturation and function of APCs. CTLA-4 is constitutively expressed on Treg cells. Compared to CD28, CTLA4 has a higher affinity for CD80/CD86 (64). Once bound, Treg cells can reduce APCs’ expression of CD80/CD86 via CTLA-4–dependent trogocytosis (65–67). This CD80/CD86 reduction on APCs also upregulates free PD-L1 on APCs (67). Treatment with CTLA-4 blockers significantly enhances anti-tumour immunity (68). LAG3 expressed by Treg cells can inhibit the expression of MHC II in dendritic cells (DCs) (69). However, it has been demonstrated that the primary fuction of MHC II in LAG-3 immunosuppression is actually mediated by the fibrinogen-like protein 1 FGL1 (70).
Additionally, Treg cells express high levels of CD39 and CD73. These two ecto-nucleotidases contribute to the conversion of ATP released from apoptotic Treg cells into adenosine (71). This directly inhibits the growth of effector T cells and the function of dendritic cells through the adenosine A2A receptor (A2AR) (71). CD39 and CD73 expression in Treg cell are increased in human cancers (72). Blockade of adenosine A2A receptor has been shown to significantly reduce Treg cells and boosts the anti-tumour activity (73). Targeting CD39 by antisense oligonucleotide also represents a promising strategy (74).
FOXP3+ Treg cell-mediated immunosuppression is also executed by the release of multiple immunosuppressive cytokines. IL-10, IL-35, and TGF-β (75, 76) inhibit the function of APCs and Teff cells, while granzymes and perforin directly kill NK and CD8+ T cell (77, 78). Recent studies have also given special attention to T regulatory cells-derived extracellular vesicles and their ability in generating immune tolerance through effector T cells and DCs (79–81). Finally, Treg cells express a higher level of IL-2R α chain (CD25) and can compete with effector T cells for limited IL-2 in TME (82, 83), thereby robbing essential cytokine for the survival of effector T cells. All the above-mentioned studies further provide the mechanistic basis for FOXP3+ Treg cells promoted immune escape of cancer (as shown in Figure 2).
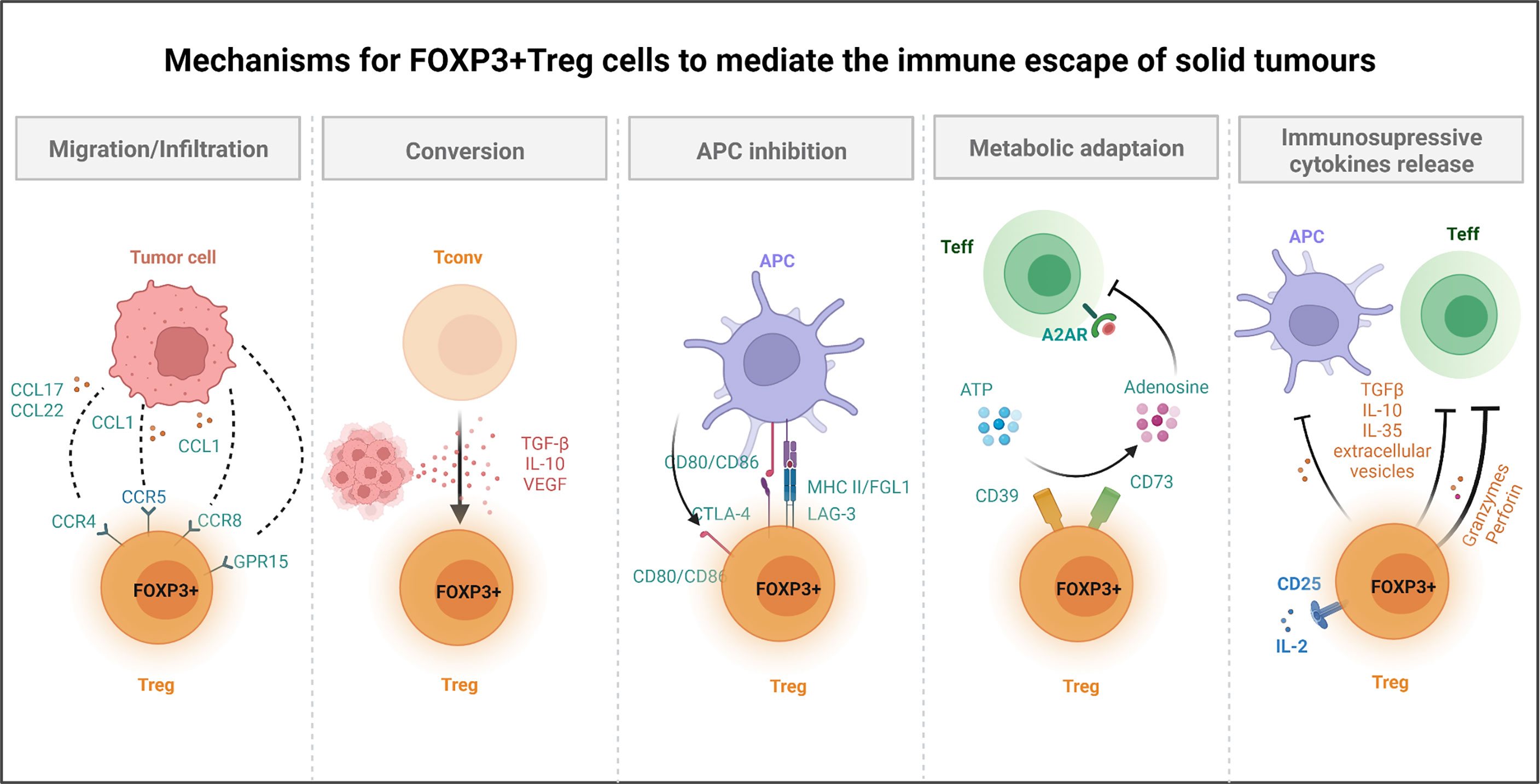
Figure 2 Mechanisms for FOXP3+ Treg cells to mediate the immune escape of solid tumours. Several mechanisms of Treg cells have been reported to help tumour to avoid immune destruction. For instance, Treg cells can promote the formation of immune suppressive microenvironment. Treg cells express chemokine receptors (e.g., CCR4, CCR8, CCR5, GPR15) and are recruited to the tumour site by chemokines produced by diverse cells within TME. Treg cells secreted immunosuppressive cytokines, TGF-β, and VEGF, which not only promote the conversion of Tconv cells to Treg cells, but also suppress Teff cells and APCs function. Treg cells constitutively express CTLA-4, while downregulate the expression of CD80/CD86 in APCs (through trans-endocytosis), thereby depriving co-stimulatory signals to responder T cells. Meanwhile, Treg cells inhibit the function of DCs through LAG-3 and MHC II interactions. For metabolic adaptation, Treg cells could converse ATP to adenosine by CD39 and CD73, which directly inhibits A2AR mediated Teff cells function. Cells within TME could also be killed by Treg cells secreted granzyme and perforin. CCL, C-C motif chemokine ligand; CCR, C-C motif chemokine receptor; GPR, G protein-coupled receptor; Tconv, conventional CD4 T cell; TGF, transforming growth factor; IL, interleukin; VEGF, vascular endothelial growth factor; CTLA-4, cytotoxic T-lymphocyte associated protein 4; MHC, major histocompatibility complex; FGL1, fibrinogen like 1; Teff, effector T cell; A2AR, adenosine A2A receptor; LAG-3, lymphocyte activating 3.
FOXP3+ Treg cells and tumour-promoting inflammation
The inflammatory response could help our body to remove the necrotic tissue and tumours, so it was once considered as a beneficial anti-tumour immune response. However, subsequent studies have demonstrated that the inflammatory process in TME can also lead to the emergence of tumour invasive metastasis, angiogenesis and other tumour-promoting features (84). FOXP3+ Treg cells may play an important role in regulating the balance of tumour inflammation. Traditionally, Treg cells are believed to be the main anti-inflammatory cells in humans, which suppress the function of immune cells and reduce the inflammatory response, resulting in a poorer prognosis in cancer patients. However, more recent studies have revealed the existence of different tissue resident FOXP3+ Treg cell subsets in CRC, in contrast to the classical Treg cell immunosuppressive function, can also exhibit a pro-inflammatory response profile and thus influence the development and progression of CRC (85). Saito and colleagues have grouped CRCs into two types, based on the proportion of FOXP3(lo) non-suppressive T cells (85). FOXP3(lo) Treg cells are distinguished from FOXP3 (+) T cells in the absence of the naïve T cell marker CD45RA, FOXP3 instability, and enhanced secretion of inflammatory cytokines (e.g., IFN-γ) by the FOXP3(lo) Treg subset (85). CRCs patients with abundant FOXP3(lo) Treg cell infiltration are predicted to have better survival. Mechanistically, Fusobacterium nucleatum, and possibly other intestinal bacteria mediate tumor tissues’ production of inflammatory cytokines (e.g., IL-12, TGF-β, and TNF-α) (85–90), thereby affecting the heterogeneity of tumour-infiltrating Treg cells in CRCs and facilitating the expansion of pro-inflammatory FOXP3(lo) non-Treg cells that, in turn, enhances anti-tumour immunity and inhibits tumour formation (85).
In Colitis-Associated Colorectal Cancer (CAC), inflammation is a key driving factor in tumourigenesis and progression. Under extensive pro-inflammatory conditions, FOXP3+ Treg cells may be redirected to a Th17 response by inflammatory cytokine IL-6 together with TGF-β (91). In particular, FOXP3+IL-17A+ T cells accumulate in the colon of patients with ulcerative colitis and CACs. CAC patients with higher FOXP3+ Treg cell levels have a poor prognosis (92). Treg cells co-expressing the transcription factors FOXP3 and IL-17A-related transcription factor RORγt in the dysplastic areas of IBD patients (93). Tumour-infiltrating FOXP3+RORγt+ Treg cells suppress FoxO3 in DCs, leaving IL-6 expression uncontrolled. At the same time, high IL-6 level stimulates STAT3 activation and proliferation of dysplastic cells (93–96). RORγt inhibition in FOXP3+RORγt+ Treg cells suppresses IL-17A production and prevents inflammatory cytokine-induced destabilization of FOXP3 expression induced by pro-inflammatory cytokines (97). Also, inhibition of RORγt increases Th17-like Treg cells’ production of IL-10, thereby enhancing the inhibition of myeloid inflammatory factors (97). Our recent studies in a colitis-associated colorectal mouse model have shown that the inhibition of the MondoA-TXNIP regulatory axis attenuates the immunosuppressive function of Treg cell and induces Treg cells’ expression of Th17 signature genes in a glycolytic metabolic pattern, thus further promoting Th17-type inflammation in the colorectal TME (98). IL-17A expressing Treg cells may cause CD8+ T cell exhaustion by IL-17A, which could accelerate colorectal carcinogenesis and tumour progression. Notably, the use of IL-17A-blocking antibodies could slow the progression of AOM-DSS-induced colorectal cancer and reduce the susceptibility to colorectal cancer in MondoA-deficient mice. Combined treatment with anti-IL-17A and anti-PD-1 antibodies further reduces the size of colorectal tumours in animal model. Interestingly, it has been found that GPR15 expression on T cells also enhanced IL-17 secretion. Gene expression analysis shows that GPR15+ Treg cells have a Th17-like phenotype, leading to the production of IL-17 and TNF-α in AOM/DSS mouse model (60).
Interestingly, during tumour development, CD4+T cells may progressively transdifferentiate into IL-17A+ FOXP3+ and ex-Th17 IL-17A- FOXP3+ T cells (99). FOXP3-expressing subsets possess immunosuppressive function. IL-33, induced in transformed epithelial cells of CRC, is an important trans-differentiation regulator. IL-33/ST2 signaling suppresses IL-17A production and potentially promotes the conversion of IL-17-producing CD4+ T cell types to IL-17-negative (RORγt−) ST2+ FOXP3+ Treg cells, modifying the inflammatory signals within the tumour microenvironment to promote CRC (100).
Taken together, the pro-inflammatory tumour microenvironment, whether intrinsic or induced, may influence the phenotype and function of Treg cells, which consequently, exert anti- or pro-tumourigenic inflammatory responses.
FOXP3+ Treg cells and the new hallmark of cancer: Polymorphic microbiomes
The “Polymorphic microbiomes” is listed as a new hallmark of cancer (5). Although increasing evidence has shown microbiomes play critical roles in carcinogenesis, and the immune system is closely associated with microbiomes, the relationship among tumour, Treg cells, and microbiome is still largely unclear (101).
The association between Treg cells and microbiomes is mainly explored in colorectal cancer, for large proportion of microorganisms reside in the human gastrointestinal system (102). The immune-suppressive capacity of tumour-infiltrating Treg cells and the M2 subset of tumour-associated macrophages (TAM) are closely correlated with intestinal microbiota in colorectal cancer patients (103). FOXP3+ Treg cells could intervene in the protective process of fecal microbiota transplanted colitis-associated colon cancer mice model (104). GPR109a signaling could also induce the differentiation of IL-10-producing Treg cells (105). The combination of Lactobacillus acidophilus lysate and anti-CTLA-4 therapy could enhance anti-tumour immunity in a mouse model of colon cancer, accompanied with increased CD8 + T cells and effector memory T cells, but decreased Treg cells and M2 macrophages (106).
Apart from CRC, Treg cells and microbiomes also engage in other cancers. High blood butyrate and propionate levels affect anti-CTLA-4 therapy efficacy in mouse model and melanoma patients, along with increased Treg cell proportions, reduced DC and effector T cell activation, and lower responses to IL-2 (107). Probiotics modulate the gut microbiome composition to produce anti-inflammatory metabolites and promote the differentiation of anti-inflammatory IL-10-producing Treg cells, which may help to against hepatocarcinoma (108). Selected Bacteroides spp. (such as B. fragilis, B. thetaiotaomicron) can modulate colonic RORγt+ Treg cells through the bile acid receptor VDR (vitamin D3 receptor), which may be of great significance in treating gastrointestinal and hepatic cancers (109).
FOXP3+ Treg cells may also facilitate carcinogenesis induced by several microbiomes. In gastric, mycobacterial infection could aggravate Helicobacter pylori-induced gastric preneoplastic pathology via inducing Treg cells (110). Moreover, intratumour microbes are thought to create a tolerogenic program with lower proportions of tumour-infiltrating lymphocytes (TILs) but increased Treg cells in various types of cancers including colorectal, pancreatic, breast, and lung cancers (111–118).
FOXP3+ Treg cells and the classic hallmarks of cancer: metastasis and invasion
As invasion and metastasis are classical cancer markers, emerging evidence suggests Treg cells also play a role in promoting tumour metastasis via multiple manners (5, 119). The levels of FOXP3+ Treg cells are strongly associated with cancer metastasis in various human cancers (120). An Increasing ratio of Treg/Th2 can promote the metastasic progression of hepatocellular carcinoma (121). FOXP3+ Treg cell levels in the peripheral blood of NSCLC patients increase with tumour stage and peak in metastatic patients (117). Increased FOXP3+ Treg cells have also been linked to a higher risk of metastasis in other cancers, including breast, ovarian, prostate, thyroid, gastric, colorectal, and skin cancers (114, 115, 118, 119).
The underlying mechanisms that contribute to the increase of tumour-infiltrating FOXP3+ Treg cells could be categorised into three major pathways. Firstly, organs susceptible to be invaded and metastasized tend to contain more FOXP3+ Treg cells (120). For instance, lung tissue could induce more Treg cells through the upregulation of prolyl-hydroxylase (PHD) proteins and a local reduction of HIF1α, which are correlated with increased intrapulmonary metastasis (122). On the other hand, bone marrow has a relatively hypoxic environment, which contributes to higher Treg cell frequency during bone metastasis of tumours (123). FOXP3+ Treg cells also promote osteogenesis by suppressing osteoclast differentiation and function, a process that may favour the bone metastasis of prostate cancer (124). Secondly, the tumour locus can recruit Treg cells to build an immune-suppressive environment for tumour progression and metastasis. For instance, elevated levels of PGE2 in TME could lead to the recruitment of FOXP3+ Treg cells, which increase the risk of bone metastasis (125). Inhibition of the CXCL12/CXCR4 axis in combination with IDO1 blockage could reduce Treg cell and bone metastasis in breast cancer model (126). After the occurrence of tumour metastasis, breast cancer cells could stimulate lung tissue to secrete CCL17 and CCL22, which attract CCR4-positive Treg cells to accumulate in lung tissue, and thus facilitating lung metastasis of breast cancer (127). Thirdly, Treg cells can promote tumour invasion and metastasis directly. Tan et al. have found Treg cells to express a higher level of RANKL than Tconvs and stimulated pulmonary metastasis of human RANK (+)breast cancer cells, and blocking this pathway can reduce the frequency of pulmonary metastasis (128). Oh et al. have reported, in mouse model, increased invasive and metastatic potential of melanoma owing to the direct contact between melanoma cells and Treg cells. Elevated TGF-β produced by Treg cells induces the epithelial-to-mesenchymal transition (EMT), leading to increased lung metastasis (129).
FOXP3+ Treg cell function in tumour angiogenesis
Inducing angiogenesis is thought to be one of the mechanism to meet the demand of nutrients and oxygen of cancer and evacuate metabolic wastes and carbon dioxide from TME (5). Recent studies suggest that FOXP3+ Treg cells may also play a functional role in tumour angiogenesis directly or indirectly to promote carcinogenesis (130–133).
FOXP3+ Treg cells can intervene in cancer angiogenesis in two ways: through the VEGF pathways or the modulation of other immune cells with inflammatory cytokine release (134). VEGF family is closely related to blood vessel formation (135). Multiple studies reported the association between FOXP3+ Treg cells and VEGF in cancer patients and in vivo tumour animal models (136–142). Hypoxia-induced CCL28 may recruit intratumoural FOXP3+ Treg cells, which can upregulate VEGFA levels to promote angiogenesis directly in ovarian cancer (138) and breast cancer (142).
In addition to VEGF pathways, Treg cells can induce cancer angiogenesis via regulating other immune cell functions. For example, Casares et al. reported a reduction of Treg cells levels can induce IFN-γ produced by effector CD4 T cells to decrease tumour angiogenesis and enhance anti-tumour response (143). Beatty et al. also emphasized the critical role of IFN-gamma-dependent inhibition of tumour angiogenesis by tumour-infiltrating CD4+ T cells (144).
On the contrary, cancer angiogenesis could conversely exert an effect on tumour-infiltrating FOXP3+ Treg cells. Numerous clinical studies have demonstrated that antiangiogenic therapy, blocking VEGFR, used in human cancers is associated with a reduction of tumour-infiltrating FOXP3+ Treg cells (145–147). VEGF could promote FOXP3+ Treg cell migration and its immunosuppressive function, but the detailed mechanisms underlying VEGFR blocking therapy and tumour-infiltrating FOXP3+ Treg cells reduction are still unclear (148–150).
FOXP3+ Treg cells and the newly proposed hallmark of cancer: Cell senescence
Senescent cells are recently proposed as a new and emerging hallmark of cancer (6). Cell senescence is an irreversible cell cycle arrest caused by various factors including: telomere shortening, DNA damage, cellular stress, and oncogenes’ activation (151, 152). In solid tumour tissues, the senescence of immune cells (e.g.,macrophages and effector T cells) is associated with increased tumour malignancy, while the senescence of cancer cells is linked to the suppression of cancer progression (151, 152).
FOXP3+ Treg cells have recently been reported to induce effector T cell senescence by metabolic competition (153). The senescent T cells are characterized by the elevated expression of senescence-associated β-galactosidase (SA-β-gal), decreased expression of CD27 and CD28, and acquired immune suppressive capacities via the production of TGF-β and IL-10 (153–157). Tumour-infiltrating FOXP3+ Treg cells exhibit higher glycolysis, which hastens glucose consumption and reduces glucose availability within TME (158). Low concentrations of glucose alone can significantly induce the senescence of both CD4+ and CD8+ T cells (153). The induction of T cell senescence, by FOXP3+ Treg cells mediated glucose insufficient, is initiated via the activation of the AMP-activated protein kinase (AMPK) (159). The activated AMPK increases the phosphorylation of p53, facilitates the accumulation of p21WAF1, promotes the expression of p27, inhibits the activities of telomerase, and reduces the expression of key components in the Toll-like receptor signalosome (160, 161). In addition, the accumulation of cyclic adenosine monophosphate (cAMP), the metabolic product of Treg cells, is also an important inducer of T cell senescence (159). Treg cells are able to transfer cAMP into T cells via tight junctions,and the elevated intracellular level of cAMP in T cells triggers the nuclear kinase ataxia-telangiectasia mutated (ATM) protein associated DNA damage, which ultimately leads to T cell senescence (159, 162). Persistent DNA damage signaling provokes the secretion of senescence-associated inflammatory cytokines, IL-2, IL-6, IL-8, TNF-α, and IFN-γ, which induce more T cell senescence within the suppressive TME (as shown in Figure 3) (159). The accumulation of immune suppressive cells enables tumour cells to escape from anti-tumour immune responses (163). However, the effect of Treg cells in inducing the senescence of tumour cells is yet to be illustrated.
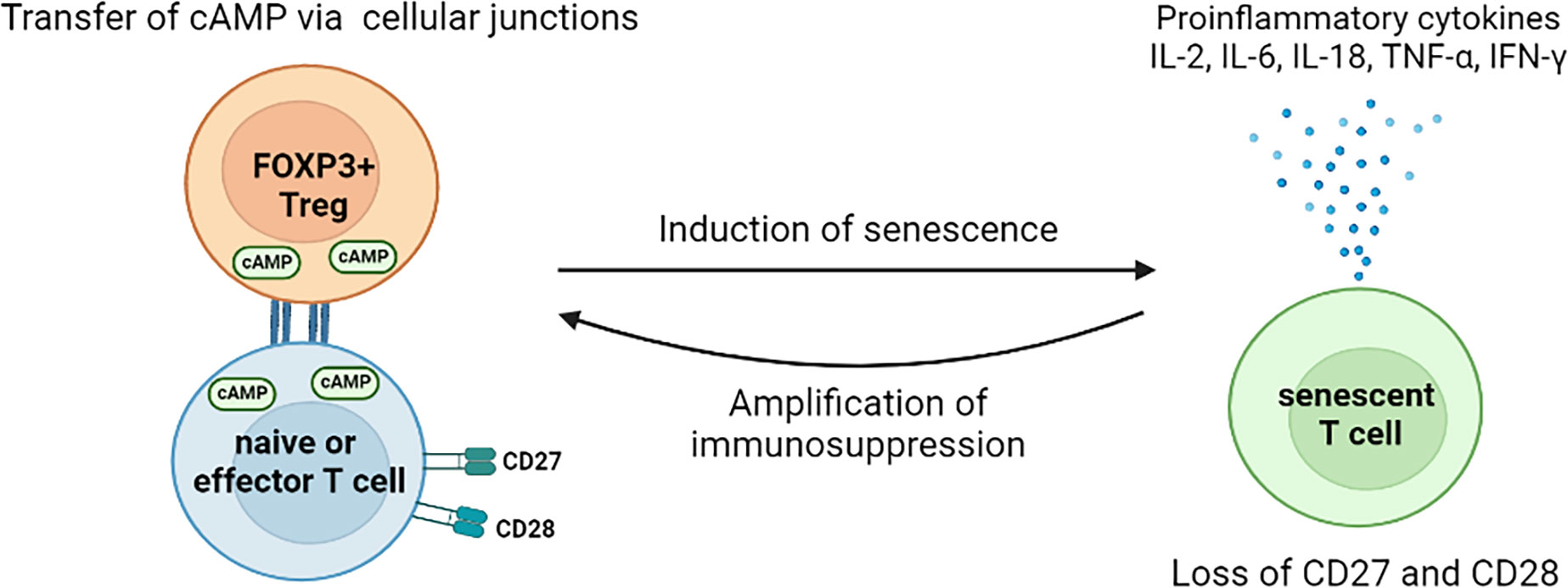
Figure 3 Mechanisms for FOXP3+ Treg cells to induce T cell senescence in the tumour microenvironment. The direct transfer of cAMPs, by Treg cells via cell junctions, induces the senescence of naïve and effector T cells. The induced senescent T cells cease the expression of CD27 and CD28 but increase the secretion of pro-inflammatory cytokines. Thus, those senescent T cells exhibit immunosuppressive features and argument the immunosuppression within TME. So far, no study of direct effect of tumour-associated Treg cells on tumour cell senescence has been found. However, Treg cells might mediate the senescence of tumour cells indirectly. For instance, the Treg cells induced senescent T cells exhibit unique SASP, which is characterized by augmented release of cytokines, chemokines, proteases, and metabolic wastes. The accumulation of these molecules as well as low glucose availability, caused by hyper-glycolysis of Treg cells, create a stress environment, thus may facilitate thesenescence of tumour cells. cAMP, cyclic adenosine monophosphate; IL, interleukin; TNF, tumour necrosis factor; IFN, interferon; TME, tumour microenvironment; SASP, senescence-associated secretory phenotype.
Metabolic connection between FOXP3+ Treg cells and cancer cells in the tumour microenvironment
Although immune receptors, signaling proteins, and transcription factors have participated in T cell responses, cellular metabolism has been recognized as one of the core determining factors for the survival, proliferation, and function of T cells. In general, immunosuppressive FOXP3+ Treg cells are more dependent on β-oxidation than glycolysis, compared with effector T cells (164, 165). However, lactic acid may provide metabolic support to tumour-infiltrating FOXP3+ Treg cells in highly glycolytic TME (166, 167). The ablation of lactate transporter MCT1 in B16 melanoma inoculated Slc16a1f/f Foxp3cre mice leads to decreased tumour growth, indicating the immunosuppressive function of Treg cells may be closely related to their ability of ingesting lactate acid (167). Tumour-infiltrating FOXP3+ Treg cells may convert lactic acid to pyruvate, which is then converted into malic acid and citric acid that ultimately participates in the tricarboxylic acid cycle (167). Further study has shown that a high lactic acid environment allows effector Treg (eTreg) cells to use MCT1 to uptake lactic acid, which upgrades the level of PD-1, leading to the functional and phenotypic changes of eTreg cells (166). In trials comparing the effects of high glycolysis tumours with low glycolysis tumours on CTLA-4 immunotherapy, the therapeutic effect of low glycolysis tumours is found to be more pronounced (168). Our recent studies have also found that the deletion of the MondoA-TXNIP transcriptional regulatory axis allows Treg cells to increase the expression and cell membrane localization of glucose transporter Glut1 for stronger glucose uptake and glycolytic metabolic capacity (98). Inhibiting mitochondria is liable to weaken Treg cells function (169–172). In Treg cells, the loss of mitochondrial transcription factor A (TFAM) is important for mitochondrial respiratory chain activity, impairs the accumulation and cell lineage stability of the tumour-infiltrating Treg cells, and thus, inhibits tumour growth (169, 170). Eliminating Treg cell-specific mitochondrial complex III increases DNA methylation, as well as enhances the accumulation of metabolites 2-hydroxyglutaric acid (2-HG) and succinic acid, thereby inhibiting Treg cells function (172). FOXP3+ Treg cells lacking transcription factor c-Myc have disrupted mitochondrial oxidative metabolic process, which decreases the accumulation and functional activation of Treg cells (171). In addition, there are other pathways related to the metabolic regulation of tissue-resident FOXP3+ Treg cells. For instance, glucose metabolism and glycolysis are selectively inhibited by TLR8 activation in tumour-infiltrating Treg cells (158). Moreover, CD36 expression on tumour-infiltrating Treg cells may mediate the uptake of long-chain fatty acids. Although the knockout of CD36 reduces FOXP3+ Treg cells within tumours, the preservation of peroxisome proliferation activation receptor-β (PPAR-β) signal-dependent mitochondrial adaptability leads to the inhibition of tumour growth (173). Inhibition of fatty acid binding protein 5 (FABP5) leads to changes in mitochondria that enhance the inhibitory capacity of FOXP3+ Treg cells (174). Besides, redox homeostasis is thought to modulate development and function of Treg cells (175, 176). Previous studies have demonstrated that increased Treg cells in tumour sites may be attributed to their increased antioxidative capacity (177, 178). Furthermore, scientists have also paid more attention to the association between Treg cells and redox homeostasis in TME. Thomas-Schoemann et al. have shown arsenic trioxide could increase anti-tumor immune response by decreasing Treg cell numbers. This Treg cell reduction is mediated by oxidative and nitrosative stress (179). Wang et al. have demonstrated that antioxidant protein thioredoxin (TRX) enhances Treg cell infiltration in melanoma, which in turn decreased anti-tumor immune reactions. Recently, Xu et al. have reported that glutathione peroxidase 4 (Gpx4) could prevent Treg cells from lipid peroxidation and ferroptosis in regulating immune homeostasis and anti-tumor immunity (180).
Conclusion and prospective
FOXP3+ Treg cells in the tumour microenvironment are regulated at multiple levels, which include Treg cell instability (181–183), Treg cell plasticity (184, 185), and tissue Treg cell specificity (186–188). Tissue-resident Treg cells maintain tissue homeostasis and improve tissue repair to prevent inflammation-induced cancer generation. While, within TME, Treg cells repress the anti-tumour immune responses. Treg cells also influence other hallmarks of tumour through cytokines or or other ligands to activate multiple signal pathways, for example, TGF-β is shown to promote tumour metastasis. Tumour cells recruit Treg cells through chemokines, cytokines, and metabolic regulation. Single-cell sequencing and FACS data indicate that in the tumour site there are different Treg cell subsets showing different functions, cytokine expression, and relationships with patient prognosis.
The efficacy of immunotherapy with immune checkpoint antibodies or engineered T cells, especially CAR-T cells, is also regulated by the tumour-infiltrating Treg cells. Several new strategies may be developed in the future to treat tumour by targeting Treg cells. First, develop dual-antibodies to suppress the function of tumour-infiltrating Treg cells; second, generate CAR-T cells resistant to the suppression of Treg cells; and last but not least, convert the suppressive Treg cells into Th1 or Th17-like Treg cells, which may improve their anti-tumour activity. Our understanding of the mutual regulation between tumour-infiltrating FOXP3+ Treg cells and the key hallmarks in solid tumours will provide new clues for generating engineered T cells to cure cancer patients.
Author contributions
YQ and SK wrote the article. JC, ZQ, WZ and YY designed and drew the figures. DM, GZ, KW, DL and BL proofread this article. All authors contributed to this article and approved the submitted version.
Funding
Our research is supported by National Key R&D Program of China 2019YFA09006100; National Natural Science Foundation of China grants 32130041, 81830051 and 31961133011; Innovative research team of high-level local universities in Shanghai SHSMU-ZDCX20210601; Key Laboratory of Cell Differentiation and Apoptosis of Chinese Ministry of Education, Shanghai Frontiers Science Center of Cellular Homeostasis and Human Diseases. Shenzhen Science and Technology Program KQTD20210811090115019.
Conflict of interest
Author BL is a co-founder of Biotheus Inc and chairman of its scientific advisory board.
The remaining authors declare that the research was conducted in the absence of any commercial or financial relationships that could be construed as a potential conflict of interest.
Publisher’s note
All claims expressed in this article are solely those of the authors and do not necessarily represent those of their affiliated organizations, or those of the publisher, the editors and the reviewers. Any product that may be evaluated in this article, or claim that may be made by its manufacturer, is not guaranteed or endorsed by the publisher.
References
1. Bray F, Laversanne M, Weiderpass E, Soerjomataram I. The ever-increasing importance of cancer as a leading cause of premature death worldwide. Cancer (2021) 127:3029–30. doi: 10.1002/cncr.33587
2. Sung H, Ferlay J, Siegel RL, Laversanne M, Soerjomataram I, Jemal A, et al. Global cancer statistics 2020: GLOBOCAN estimates of incidence and mortality worldwide for 36 cancers in 185 countries. CA: Cancer J Clin (2021) 71:209–49. doi: 10.3322/caac.21660
3. Dees S, Ganesan R, Singh S, Grewal IS. Regulatory T cell targeting in cancer: Emerging strategies in immunotherapy. Eur J Immunol (2021) 51:280–91. doi: 10.1002/eji.202048992
4. Hanahan D, Weinberg RA. The hallmarks of cancer. Cell (2000) 100:57–70. doi: 10.1016/s0092-8674(00)81683-9
5. Hanahan D, Weinberg RA. Hallmarks of cancer: the next generation. Cell (2011) 144:646–74. doi: 10.1016/j.cell.2011.02.013
6. Hanahan D. Hallmarks of cancer: New dimensions. Cancer Discov (2022) 12:31–46. doi: 10.1158/2159-8290.CD-21-1059
7. Kondĕlková K, Vokurková D, Krejsek J, Borská L, Fiala Z, Ctirad A. Regulatory T cells (TREG) and their roles in immune system with respect to immunopathological disorders. Acta Med (Hradec Kralove) (2010) 53:73–7. doi: 10.14712/18059694.2016.63
8. Bin Dhuban K, d’Hennezel E, Nagai Y, Xiao Y, Shao S, Istomine R, et al. Suppression by human FOXP3+ regulatory T cells requires FOXP3-TIP60 interactions. Sci Immunol (2017) 2(12):eaai9297. doi: 10.1126/sciimmunol.aai9297
9. Kwon H-K, Chen H-M, Mathis D, Benoist C. Different molecular complexes that mediate transcriptional induction and repression by FoxP3. Nat Immunol (2017) 18:1238–48. doi: 10.1038/ni.3835
10. Li B, Samanta A, Song X, Furuuchi K, Iacono KT, Kennedy S, et al. FOXP3 ensembles in T-cell regulation. Immunol Rev (2006) 212:99–113. doi: 10.1111/j.0105-2896.2006.00405.x
11. Li B, Samanta A, Song X, Iacono KT, Brennan P, Chatila TA, et al. FOXP3 is a homo-oligomer and a component of a supramolecular regulatory complex disabled in the human XLAAD/IPEX autoimmune disease. Int Immunol (2007) 19:825–35. doi: 10.1093/intimm/dxm043
12. Ono M, Yaguchi H, Ohkura N, Kitabayashi I, Nagamura Y, Nomura T, et al. Foxp3 controls regulatory T-cell function by interacting with AML1/Runx1. Nature (2007) 446:685–9. doi: 10.1038/nature05673
13. Rudra D, deRoos P, Chaudhry A, Niec RE, Arvey A, Samstein RM, et al. Transcription factor Foxp3 and its protein partners form a complex regulatory network. Nat Immunol (2012) 13:1010–9. doi: 10.1038/ni.2402
14. Wu Y, Borde M, Heissmeyer V, Feuerer M, Lapan AD, Stroud JC, et al. FOXP3 controls regulatory T cell function through cooperation with NFAT. Cell (2006) 126:375–87. doi: 10.1016/j.cell.2006.05.042
15. Li Z, Li D, Tsun A, Li B. FOXP3+ regulatory T cells and their functional regulation. Cell Mol Immunol (2015) 12:558–65. doi: 10.1038/cmi.2015.10
16. Chen B-J, Zhao J-W, Zhang D-H, Zheng A-H, Wu G-Q. Immunotherapy of cancer by targeting regulatory T cells. Int Immunopharmacol (2022) 104:108469. doi: 10.1016/j.intimp.2021.108469
17. Li C, Jiang P, Wei S, Xu X, Wang J. Regulatory T cells in tumor microenvironment: New mechanisms, potential therapeutic strategies and future prospects. Mol Cancer (2020) 19:116. doi: 10.1186/s12943-020-01234-1
18. Orimo A, Weinberg RA. Stromal fibroblasts in cancer: a novel tumor-promoting cell type. Cell Cycle (2006) 5:1597–601. doi: 10.4161/cc.5.15.3112
19. Sharma P. Primary, adaptive, and acquired resistance to cancer immunotherapy. Cell (2017) 168(4):707–23. doi: 10.1016/j.cell.2017.01.017
20. Park HJ, Park JS, Jeong YH, Son J, Ban YH, Lee B-H, et al. PD-1 upregulated on regulatory T cells during chronic virus infection enhances the suppression of CD8+ T cell immune response via the interaction with PD-L1 expressed on CD8+ T cells. J Immunol (2015) 194:5801–11. doi: 10.4049/jimmunol.1401936
21. Kim J-H, Kim BS, Lee S-K. Regulatory T cells in tumor microenvironment and approach for anticancer immunotherapy. Immune Netw (2020) 20:e4. doi: 10.4110/in.2020.20.e4
22. Rueda CM, Jackson CM, Chougnet CA. Regulatory T-Cell-Mediated suppression of conventional T-cells and dendritic cells by different cAMP intracellular pathways. Front Immunol (2016) 7:216. doi: 10.3389/fimmu.2016.00216
23. Grover P, Goel PN, Greene MI. Regulatory T cells: Regulation of identity and function. Front Immunol (2021) 12:750542. doi: 10.3389/fimmu.2021.750542
24. Dixon ML, Luo L, Ghosh S, Grimes JM, Leavenworth JD, Leavenworth JW. Remodeling of the tumor microenvironment via disrupting Blimp1+ effector treg activity augments response to anti-PD-1 blockade. Mol Cancer (2021) 20:150. doi: 10.1186/s12943-021-01450-3
25. Buglio D, Khaskhely NM, Voo KS, Martinez-Valdez H, Liu Y-J, Younes A. HDAC11 plays an essential role in regulating OX40 ligand expression in Hodgkin lymphoma. Blood (2011) 117:2910–7. doi: 10.1182/blood-2010-08-303701
26. So T, Croft M. Cutting edge: OX40 inhibits TGF-beta- and antigen-driven conversion of naive CD4 T cells into CD25+Foxp3+ T cells. J Immunol (2007) 179:1427–30. doi: 10.4049/jimmunol.179.3.1427
27. Vu MD, Xiao X, Gao W, Degauque N, Chen M, Kroemer A, et al. OX40 costimulation turns off Foxp3+ tregs. Blood (2007) 110:2501–10. doi: 10.1182/blood-2007-01-070748
28. Deng S, Hu Q, Zhang H, Yang F, Peng C, Huang C. HDAC3 inhibition upregulates PD-L1 expression in b-cell lymphomas and augments the efficacy of anti-PD-L1 therapy. Mol Cancer Ther (2019) 18:900–8. doi: 10.1158/1535-7163.MCT-18-1068
29. Hicks KC, Fantini M, Donahue RN, Schwab A, Knudson KM, Tritsch SR, et al. Epigenetic priming of both tumor and NK cells augments antibody-dependent cellular cytotoxicity elicited by the anti-PD-L1 antibody avelumab against multiple carcinoma cell types. Oncoimmunology (2018) 7:e1466018. doi: 10.1080/2162402X.2018.1466018
30. Hua S, Gu M, Wang Y, Ban D, Ji H. Oxymatrine reduces expression of programmed death-ligand 1 by promoting DNA demethylation in colorectal cancer cells. Clin Transl Oncol (2021) 23:750–6. doi: 10.1007/s12094-020-02464-x
31. Que Y, Zhang X-L, Liu Z-X, Zhao J-J, Pan Q-Z, Wen X-Z, et al. Frequent amplification of HDAC genes and efficacy of HDAC inhibitor chidamide and PD-1 blockade combination in soft tissue sarcoma. J Immunother Cancer (2021) 9(2):e001696. doi: 10.1136/jitc-2020-001696
32. Llopiz D, Ruiz M, Villanueva L, Iglesias T, Silva L, Egea J, et al. Enhanced anti-tumor efficacy of checkpoint inhibitors in combination with the histone deacetylase inhibitor belinostat in a murine hepatocellular carcinoma model. Cancer Immunol Immunother (2019) 68:379–93. doi: 10.1007/s00262-018-2283-0
33. Ny L, Jespersen H, Karlsson J, Alsén S, Filges S, All-Eriksson C, et al. The PEMDAC phase 2 study of pembrolizumab and entinostat in patients with metastatic uveal melanoma. Nat Commun (2021) 12:5155. doi: 10.1038/s41467-021-25332-w
34. Tu K, Yu Y, Wang Y, Yang T, Hu Q, Qin X, et al. Combination of chidamide-mediated epigenetic modulation with immunotherapy: Boosting tumor immunogenicity and response to PD-1/PD-L1 blockade. ACS Appl Mater Interfaces (2021) 13:39003–17. doi: 10.1021/acsami.1c08290
35. Deng B, Yang B, Chen J, Wang S, Zhang W, Guo Y, et al. Gallic Acid induces T-helper-1- like treg cells and strengthens immune checkpoint blockade efficacy. J Immunother Cancer (2022) 10(7):e004037. doi: 10.1136/jitc-2021-004037
36. Huang J, Wang X, Li B, Shen S, Wang R, Tao H, et al. L-5-hydroxytryptophan promotes antitumor immunity by inhibiting PD-L1 inducible expression. J Immunother Cancer (2022) 10(6):e003957. doi: 10.1136/jitc-2021-003957
37. Hui E, Cheung J, Zhu J, Su X, Taylor MJ, Wallweber HA, et al. T Cell costimulatory receptor CD28 is a primary target for PD-1-mediated inhibition. Science (2017) 355:1428–33. doi: 10.1126/science.aaf1292
38. Kamphorst AO, Wieland A, Nasti T, Yang S, Zhang R, Barber DL, et al. Rescue of exhausted CD8 T cells by PD-1-targeted therapies is CD28-dependent. Science (2017) 355:1423–7. doi: 10.1126/science.aaf0683
39. Yokosuka T, Takamatsu M, Kobayashi-Imanishi W, Hashimoto-Tane A, Azuma M, Saito T. Programmed cell death 1 forms negative costimulatory microclusters that directly inhibit T cell receptor signaling by recruiting phosphatase SHP2. J Exp Med (2012) 209:1201–17. doi: 10.1084/jem.20112741
40. Kumar S, Sharawat SK. Epigenetic regulators of programmed death-ligand 1 expression in human cancers. Transl Res (2018) 202:129–45. doi: 10.1016/j.trsl.2018.05.011
41. Kumagai S, Togashi Y, Kamada T, Sugiyama E, Nishinakamura H, Takeuchi Y, et al. The PD-1 expression balance between effector and regulatory T cells predicts the clinical efficacy of PD-1 blockade therapies. Nat Immunol (2020) 21:1346–58. doi: 10.1038/s41590-020-0769-3
42. DuPage M, Chopra G, Quiros J, Rosenthal WL, Morar MM, Holohan D, et al. The chromatin-modifying enzyme Ezh2 is critical for the maintenance of regulatory T cell identity after activation. Immunity (2015) 42:227–38. doi: 10.1016/j.immuni.2015.01.007
43. Wang D, Quiros J, Mahuron K, Pai C-C, Ranzani V, Young A, et al. Targeting EZH2 reprograms intratumoral regulatory T cells to enhance cancer immunity. Cell Rep (2018) 23:3262–74. doi: 10.1016/j.celrep.2018.05.050
44. Barsheshet Y, Wildbaum G, Levy E, Vitenshtein A, Akinseye C, Griggs J, et al. CCR8+FOXp3+ treg cells as master drivers of immune regulation. Proc Natl Acad Sci U.S.A. (2017) 114:6086–91. doi: 10.1073/pnas.1621280114
45. Simone M, Arrigoni A, Rossetti G, Gruarin P, Ranzani V, Politano C, et al. Transcriptional landscape of human tissue lymphocytes unveils uniqueness of tumor-infiltrating T regulatory cells. Immunity (2016) 45:1135–47. doi: 10.1016/j.immuni.2016.10.021
46. Plitas G, Konopacki C, Wu K, Bos PD, Morrow M, Putintseva EV, et al. Regulatory T cells exhibit distinct features in human breast cancer. Immunity (2016) 45:1122–34. doi: 10.1016/j.immuni.2016.10.032
47. Kidani Y, Nogami W, Yasumizu Y, Kawashima A, Tanaka A, Sonoda Y, et al. CCR8-targeted specific depletion of clonally expanded treg cells in tumor tissues evokes potent tumor immunity with long-lasting memory. Proc Natl Acad Sci USA (2022) 119(7):e2114282119. doi: 10.1073/pnas.2114282119
48. Yi G, Guo S, Liu W, Wang H, Liu R, Tsun A, et al. Identification and functional analysis of heterogeneous FOXP3+ treg cell subpopulations in human pancreatic ductal adenocarcinoma. Sci Bull (2018) 63:972–81. doi: 10.1016/j.scib.2018.05.028
49. Campbell JR, McDonald BR, Mesko PB, Siemers NO, Singh PB, Selby M, et al. Fc-optimized anti-CCR8 antibody depletes regulatory T cells in human tumor models. Cancer Res (2021) 81:2983–94. doi: 10.1158/0008-5472.CAN-20-3585
50. van Damme H, Dombrecht B, Kiss M, Roose H, Allen E, van Overmeire E, et al. Therapeutic depletion of CCR8+ tumor-infiltrating regulatory T cells elicits antitumor immunity and synergizes with anti-PD-1 therapy. J Immunother Cancer (2021) 9(2):e001749. doi: 10.1136/jitc-2020-001749
51. Bromley SK, Mempel TR, Luster AD. Orchestrating the orchestrators: chemokines in control of T cell traffic. Nat Immunol (2008) 9:970–80. doi: 10.1038/ni.f.213
52. Jorapur A, Marshall LA, Jacobson S, Xu M, Marubayashi S, Zibinsky M, et al. EBV+ tumors exploit tumor cell-intrinsic and -extrinsic mechanisms to produce regulatory T cell-recruiting chemokines CCL17 and CCL22. PloS Pathog (2022) 18:e1010200. doi: 10.1371/journal.ppat.1010200
53. Gao Y, You M, Fu J, Tian M, Zhong X, Du C, et al. Intratumoral stem-like CCR4+ regulatory T cells orchestrate the immunosuppressive microenvironment in HCC associated with hepatitis b. J Hepatol (2022) 76:148–59. doi: 10.1016/j.jhep.2021.08.029
54. Kurose K, Ohue Y, Sato E, Yamauchi A, Eikawa S, Isobe M, et al. Increase in activated treg in TIL in lung cancer and in vitro depletion of treg by ADCC using an antihuman CCR4 mAb (KM2760). J Thorac Oncol (2015) 10:74–83. doi: 10.1097/JTO.0000000000000364
55. Maeda S, Motegi T, Iio A, Kaji K, Goto-Koshino Y, Eto S, et al. Anti-CCR4 treatment depletes regulatory T cells and leads to clinical activity in a canine model of advanced prostate cancer. J Immunother Cancer (2022) 10(2):e003731. doi: 10.1136/jitc-2021-003731
56. Marshall LA, Marubayashi S, Jorapur A, Jacobson S, Zibinsky M, Robles O, et al. Tumors establish resistance to immunotherapy by regulating treg recruitment via CCR4. J Immunother Cancer (2020) 8(2):e000764. doi: 10.1136/jitc-2020-000764
57. Wang X, Lang M, Zhao T, Feng X, Zheng C, Huang C, et al. Cancer-FOXP3 directly activated CCL5 to recruit FOXP3+ Treg cells in pancreatic ductal adenocarcinoma. Oncogene (2017) 36:3048–58. doi: 10.1038/onc.2016.458
58. Chang L-Y, Lin Y-C, Mahalingam J, Huang C-T, Chen T-W, Kang C-W, et al. Tumor-derived chemokine CCL5 enhances TGF-β-mediated killing of CD8(+) T cells in colon cancer by T-regulatory cells. Cancer Res (2012) 72:1092–102. doi: 10.1158/0008-5472.CAN-11-2493
59. Schlecker E, Stojanovic A, Eisen C, Quack C, Falk CS, Umansky V, et al. Tumor-infiltrating monocytic myeloid-derived suppressor cells mediate CCR5-dependent recruitment of regulatory T cells favoring tumor growth. J Immunol (2012) 189:5602–11. doi: 10.4049/jimmunol.1201018
60. Adamczyk A, Pastille E, Kehrmann J, Vu VP, Geffers R, Wasmer M-H, et al. GPR15 facilitates recruitment of regulatory T cells to promote colorectal cancer. Cancer Res (2021) 81:2970–82. doi: 10.1158/0008-5472.CAN-20-2133
61. Curti A, Pandolfi S, Valzasina B, Aluigi M, Isidori A, Ferri E, et al. Modulation of tryptophan catabolism by human leukemic cells results in the conversion of CD25- into CD25+ T regulatory cells. Blood (2007) 109:2871–7. doi: 10.1182/blood-2006-07-036863
62. Zhou G, Levitsky HI. Natural regulatory T cells and de novo-induced regulatory T cells contribute independently to tumor-specific tolerance. J Immunol (2007) 178:2155–62. doi: 10.4049/jimmunol.178.4.2155
63. Yu X, Harden K, Gonzalez LC, Francesco M, Chiang E, Irving B, et al. The surface protein TIGIT suppresses T cell activation by promoting the generation of mature immunoregulatory dendritic cells. Nat Immunol (2009) 10:48–57. doi: 10.1038/ni.1674
64. Tanaka A, Sakaguchi S. Regulatory T cells in cancer immunotherapy. Cell Res (2017) 27:109–18. doi: 10.1038/cr.2016.151
65. Gu P, Gao JF, D’Souza CA, Kowalczyk A, Chou K-Y, Zhang L. Trogocytosis of CD80 and CD86 by induced regulatory T cells. Cell Mol Immunol (2012) 9:136–46. doi: 10.1038/cmi.2011.62
66. Qureshi OS, Zheng Y, Nakamura K, Attridge K, Manzotti C, Schmidt EM, et al. Trans-endocytosis of CD80 and CD86: A molecular basis for the cell-extrinsic function of CTLA-4. Science (2011) 332:600–3. doi: 10.1126/science.1202947
67. Tekguc M, Wing JB, Osaki M, Long J, Sakaguchi S. Treg-expressed CTLA-4 depletes CD80/CD86 by trogocytosis, releasing free PD-L1 on antigen-presenting cells. Proc Natl Acad Sci USA (2021) 118(30):e2023739118. doi: 10.1073/pnas.2023739118
68. Pai C-CS, Simons DM, Lu X, Evans M, Wei J, Wang Y-H, et al. Tumor-conditional anti-CTLA4 uncouples antitumor efficacy from immunotherapy-related toxicity. J Clin Invest (2019) 129:349–63. doi: 10.1172/JCI123391
69. Liang B, Workman C, Lee J, Chew C, Dale BM, Colonna L, et al. Regulatory T cells inhibit dendritic cells by lymphocyte activation gene-3 engagement of MHC class II. J Immunol (2008) 180:5916–26. doi: 10.4049/jimmunol.180.9.5916
70. Wang J, Sanmamed MF, Datar I, Su TT, Ji L, Sun J, et al. Fibrinogen-like protein 1 is a major immune inhibitory ligand of LAG-3. Cell (2019) 176:334–347.e12. doi: 10.1016/j.cell.2018.11.010
71. Maj T, Wang W, Crespo J, Zhang H, Wang W, Wei S, et al. Oxidative stress controls regulatory T cell apoptosis and suppressor activity and PD-L1-blockade resistance in tumor. Nat Immunol (2017) 18:1332–41. doi: 10.1038/ni.3868
72. Mandapathil M, Szczepanski MJ, Szajnik M, Ren J, Lenzner DE, Jackson EK, et al. Increased ectonucleotidase expression and activity in regulatory T cells of patients with head and neck cancer. Clin Cancer Res (2009) 15:6348–57. doi: 10.1158/1078-0432.CCR-09-1143
73. Ma S-R, Deng W-W, Liu J-F, Mao L, Yu G-T, Bu L-L, et al. Blockade of adenosine A2A receptor enhances CD8+ T cells response and decreases regulatory T cells in head and neck squamous cell carcinoma. Mol Cancer (2017) 16:99. doi: 10.1186/s12943-017-0665-0
74. Kashyap AS, Thelemann T, Klar R, Kallert SM, Festag J, Buchi M, et al. Antisense oligonucleotide targeting CD39 improves anti-tumor T cell immunity. J Immunother Cancer (2019) 7:67. doi: 10.1186/s40425-019-0545-9
75. Turnis ME, Sawant DV, Szymczak-Workman AL, Andrews LP, Delgoffe GM, Yano H, et al. Interleukin-35 limits anti-tumor immunity. Immunity (2016) 44:316–29. doi: 10.1016/j.immuni.2016.01.013
76. Togashi Y, Shitara K, Nishikawa H. Regulatory T cells in cancer immunosuppression - implications for anticancer therapy. Nat Rev Clin Oncol (2019) 16:356–71. doi: 10.1038/s41571-019-0175-7
77. Cao X, Cai SF, Fehniger TA, Song J, Collins LI, Piwnica-Worms DR, et al. Granzyme b and perforin are important for regulatory T cell-mediated suppression of tumor clearance. Immunity (2007) 27:635–46. doi: 10.1016/j.immuni.2007.08.014
78. Grossman WJ, Verbsky JW, Barchet W, Colonna M, Atkinson JP, Ley TJ. Human T regulatory cells can use the perforin pathway to cause autologous target cell death. Immunity (2004) 21:589–601. doi: 10.1016/j.immuni.2004.09.002
79. Okoye IS, Coomes SM, Pelly VS, Czieso S, Papayannopoulos V, Tolmachova T, et al. MicroRNA-containing T-Regulatory-Cell-Derived exosomes suppress pathogenic T helper 1 cells. Immunity (2014) 41:503. doi: 10.1016/j.immuni.2014.08.008
80. Smyth LA, Ratnasothy K, Tsang JY, Boardman D, Warley A, Lechler R, et al. CD73 expression on extracellular vesicles derived from CD4+ CD25+ Foxp3+ T cells contributes to their regulatory function. Eur J Immunol (2013) 43:2430–40. doi: 10.1002/eji.201242909
81. Tung SL, Boardman DA, Sen M, Letizia M, Peng Q, Cianci N, et al. Regulatory T cell-derived extracellular vesicles modify dendritic cell function. Sci Rep (2018) 8:6065. doi: 10.1038/s41598-018-24531-8
82. Pandiyan P, Zheng L, Ishihara S, Reed J, Lenardo MJ. CD4+CD25+Foxp3+ regulatory T cells induce cytokine deprivation-mediated apoptosis of effector CD4+ T cells. Nat Immunol (2007) 8:1353–62. doi: 10.1038/ni1536
83. Fontenot JD, Rasmussen JP, Gavin MA, Rudensky AY. A function for interleukin 2 in Foxp3-expressing regulatory T cells. Nat Immunol (2005) 6:1142–51. doi: 10.1038/ni1263
84. Mair F, Erickson JR, Frutoso M, Konecny AJ, Greene E, Voillet V, et al. Extricating human tumour immune alterations from tissue inflammation. Nature (2022) 605:728–35. doi: 10.1038/s41586-022-04718-w
85. Saito T, Nishikawa H, Wada H, Nagano Y, Sugiyama D, Atarashi K, et al. Two FOXP3(+)CD4(+) T cell subpopulations distinctly control the prognosis of colorectal cancers. Nat Med (2016) 22:679–84. doi: 10.1038/nm.4086
86. Wu P, Wu D, Ni C, Ye J, Chen W, Hu G, et al. γδT17 cells promote the accumulation and expansion of myeloid-derived suppressor cells in human colorectal cancer. Immunity (2014) 40:785–800. doi: 10.1016/j.immuni.2014.03.013
87. Chung L, Orberg ET, Geis AL, Chan JL, Fu K, DeStefano Shields CE, et al. Bacteroides fragilis toxin coordinates a pro-carcinogenic inflammatory cascade via targeting of colonic epithelial cells. Cell Host Microbe (2018) 23:421. doi: 10.1016/j.chom.2018.02.004
88. Grivennikov SI, Wang K, Mucida D, Stewart CA, Schnabl B, Jauch D, et al. Adenoma-linked barrier defects and microbial products drive IL-23/IL-17-mediated tumour growth. Nature (2012) 491:254–8. doi: 10.1038/nature11465
89. Ling Z, Shao L, Liu X, Cheng Y, Yan C, Mei Y, et al. Regulatory T cells and plasmacytoid dendritic cells within the tumor microenvironment in gastric cancer are correlated with gastric microbiota dysbiosis: A preliminary study. Front Immunol (2019) 10:533. doi: 10.3389/fimmu.2019.00533
90. Lopès A, Billard E, Casse AH, Villéger R, Veziant J, Roche G, et al. Colibactin-positive escherichia coli induce a procarcinogenic immune environment leading to immunotherapy resistance in colorectal cancer. Int J Cancer (2020) 146:3147–59. doi: 10.1002/ijc.32920
91. Bettelli E, Carrier Y, Gao W, Korn T, Strom TB, Oukka M, et al. Reciprocal developmental pathways for the generation of pathogenic effector TH17 and regulatory T cells. Nature (2006) 441:235–8. doi: 10.1038/nature04753
92. Soh JS, Jo S, Lee H, Do E-J, Hwang SW, Park SH, et al. Immunoprofiling of colitis-associated and sporadic colorectal cancer and its clinical significance. Sci Rep (2019) 9:6833. doi: 10.1038/s41598-019-42986-1
93. Rizzo A, Di Giovangiulio M, Stolfi C, Franzè E, Fehling H-J, Carsetti R, et al. RORγt-expressing tregs drive the growth of colitis-associated colorectal cancer by controlling IL6 in dendritic cells. Cancer Immunol Res (2018) 6:1082–92. doi: 10.1158/2326-6066.CIR-17-0698
94. Becker C, Fantini MC, Schramm C, Lehr HA, Wirtz S, Nikolaev A, et al. TGF-beta suppresses tumor progression in colon cancer by inhibition of IL-6 trans-signaling. Immunity (2004) 21:491–501. doi: 10.1016/j.immuni.2004.07.020
95. Cross-Knorr S, Lu S, Perez K, Guevara S, Brilliant K, Pisano C, et al. RKIP phosphorylation and STAT3 activation is inhibited by oxaliplatin and camptothecin and are associated with poor prognosis in stage II colon cancer patients. BMC Cancer (2013) 13:463. doi: 10.1186/1471-2407-13-463
96. Shi W, Men L, Pi X, Jiang T, Peng D, Huo S, et al. Shikonin suppresses colon cancer cell growth and exerts synergistic effects by regulating ADAM17 and the IL−6/STAT3 signaling pathway. Int J Oncol (2021) 59(6):99. doi: 10.3892/ijo.2021.5279
97. Boardman DA, Garcia RV, Ivison SM, Bressler B, Dhar TM, Zhao Q, et al. Pharmacological inhibition of RORC2 enhances human Th17-treg stability and function. Eur J Immunol (2020) 50:1400–11. doi: 10.1002/eji.201948435
98. Lu Y, Li Y, Liu Q, Tian N, Du P, Zhu F, et al. MondoA-Thioredoxin-Interacting protein axis maintains regulatory T-cell identity and function in colorectal cancer microenvironment. Gastroenterology (2021) 161:575–591.e16. doi: 10.1053/j.gastro.2021.04.041
99. Downs-Canner S, Berkey S, Delgoffe GM, Edwards RP, Curiel T, Odunsi K, et al. Suppressive IL-17A+Foxp3+ and ex-Th17 IL-17AnegFoxp3+ treg cells are a source of tumour-associated treg cells. Nat Commun (2017) 8:14649. doi: 10.1038/ncomms14649
100. Pastille E, Wasmer M-H, Adamczyk A, Vu VP, Mager LF, Phuong NN, et al. The IL-33/ST2 pathway shapes the regulatory T cell phenotype to promote intestinal cancer. Mucosal Immunol (2019) 12:990–1003. doi: 10.1038/s41385-019-0176-y
101. Derosa L, Routy B, Desilets A, Daillère R, Terrisse S, Kroemer G, et al. Microbiota-centered interventions: The next breakthrough in immuno-oncology? Cancer Discovery (2021) 11:2396–412. doi: 10.1158/2159-8290.CD-21-0236
102. Park EM, Chelvanambi M, Bhutiani N, Kroemer G, Zitvogel L, Wargo JA. Targeting the gut and tumor microbiota in cancer. Nat Med (2022) 28:690–703. doi: 10.1038/s41591-022-01779-2
103. Kikuchi T, Mimura K, Ashizawa M, Okayama H, Endo E, Saito K, et al. Characterization of tumor-infiltrating immune cells in relation to microbiota in colorectal cancers. Cancer Immunol Immunother (2020) 69:23–32. doi: 10.1007/s00262-019-02433-6
104. Wang Z, Hua W, Li C, Chang H, Liu R, Ni Y, et al. Protective role of fecal microbiota transplantation on colitis and colitis-associated colon cancer in mice is associated with treg cells. Front Microbiol (2019) 10:2498. doi: 10.3389/fmicb.2019.02498
105. Singh N, Gurav A, Sivaprakasam S, Brady E, Padia R, Shi H, et al. Activation of Gpr109a, receptor for niacin and the commensal metabolite butyrate, suppresses colonic inflammation and carcinogenesis. Immunity (2014) 40:128–39. doi: 10.1016/j.immuni.2013.12.007
106. Zhuo Q, Yu B, Zhou J, Zhang J, Zhang R, Xie J, et al. Lysates of lactobacillus acidophilus combined with CTLA-4-blocking antibodies enhance antitumor immunity in a mouse colon cancer model. Sci Rep (2019) 9:20128. doi: 10.1038/s41598-019-56661-y
107. Coutzac C, Jouniaux J-M, Paci A, Schmidt J, Mallardo D, Seck A, et al. Systemic short chain fatty acids limit antitumor effect of CTLA-4 blockade in hosts with cancer. Nat Commun (2020) 11:2168. doi: 10.1038/s41467-020-16079-x
108. Li J, Sung CY, Lee N, Ni Y, Pihlajamäki J, Panagiotou G, et al. Probiotics modulated gut microbiota suppresses hepatocellular carcinoma growth in mice. Proc Natl Acad Sci U.S.A. (2016) 113:E1306–15. doi: 10.1073/pnas.1518189113
109. Song X, Sun X, Oh SF, Wu M, Zhang Y, Zheng W, et al. Microbial bile acid metabolites modulate gut RORγ+ regulatory T cell homeostasis. Nature (2020) 577:410–5. doi: 10.1038/s41586-019-1865-0
110. Artola-Borán M, Fallegger A, Priola M, Jeske R, Waterboer T, Dohlman AB, et al. Mycobacterial infection aggravates helicobacter pylori-induced gastric preneoplastic pathology by redirection of de novo induced treg cells. Cell Rep (2022) 38:110359. doi: 10.1016/j.celrep.2022.110359
111. Hamada T, Zhang X, Mima K, Bullman S, Sukawa Y, Nowak JA, et al. Fusobacterium nucleatum in colorectal cancer relates to immune response differentially by tumor microsatellite instability status. Cancer Immunol Res (2018) 6:1327–36. doi: 10.1158/2326-6066.CIR-18-0174
112. Jin C, Lagoudas GK, Zhao C, Bullman S, Bhutkar A, Hu B, et al. Commensal microbiota promote lung cancer development via γδ T cells. Cell (2019) 176:998–1013.e16. doi: 10.1016/j.cell.2018.12.040
113. Le Noci V, Guglielmetti S, Arioli S, Camisaschi C, Bianchi F, Sommariva M, et al. Modulation of pulmonary microbiota by antibiotic or probiotic aerosol therapy: A strategy to promote immunosurveillance against lung metastases. Cell Rep (2018) 24:3528–38. doi: 10.1016/j.celrep.2018.08.090
114. Mima K, Sukawa Y, Nishihara R, Qian ZR, Yamauchi M, Inamura K, et al. Fusobacterium nucleatum and T cells in colorectal carcinoma. JAMA Oncol (2015) 1:653–61. doi: 10.1001/jamaoncol.2015.1377
115. Parhi L, Alon-Maimon T, Sol A, Nejman D, Shhadeh A, Fainsod-Levi T, et al. Breast cancer colonization by fusobacterium nucleatum accelerates tumor growth and metastatic progression. Nat Commun (2020) 11:3259. doi: 10.1038/s41467-020-16967-2
116. Pushalkar S, Hundeyin M, Daley D, Zambirinis CP, Kurz E, Mishra A, et al. The pancreatic cancer microbiome promotes oncogenesis by induction of innate and adaptive immune suppression. Cancer Discov (2018) 8:403–16. doi: 10.1158/2159-8290.CD-17-1134
117. Roberti MP, Yonekura S, Duong CP, Picard M, Ferrere G, Tidjani Alou M, et al. Chemotherapy-induced ileal crypt apoptosis and the ileal microbiome shape immunosurveillance and prognosis of proximal colon cancer. Nat Med (2020) 26:919–31. doi: 10.1038/s41591-020-0882-8
118. Sepich-Poore GD, Zitvogel L, Straussman R, Hasty J, Wargo JA, Knight R. The microbiome and human cancer. Science (2021) 371(6536):eabc4552. doi: 10.1126/science.abc4552
119. Halvorsen EC, Mahmoud SM, Bennewith KL. Emerging roles of regulatory T cells in tumour progression and metastasis. Cancer Metastasis Rev (2014) 33:1025–41. doi: 10.1007/s10555-014-9529-x
120. Huppert LA, Green MD, Kim L, Chow C, Leyfman Y, Daud AI, et al. Tissue-specific tregs in cancer metastasis: opportunities for precision immunotherapy. Cell Mol Immunol (2022) 19:33–45. doi: 10.1038/s41423-021-00742-4
121. Li C, Li S, Zhang J, Sun F. El Aumento de la proporción de Treg/Th2 en la promoción de la metástasis del carcinoma hepatocelular. Cir Cir (2022) 90:187–92. doi: 10.24875/CIRU.21000361
122. Clever D, Roychoudhuri R, Constantinides MG, Askenase MH, Sukumar M, Klebanoff CA, et al. Oxygen sensing by T cells establishes an immunologically tolerant metastatic niche. Cell (2016) 166:1117–1131.e14. doi: 10.1016/j.cell.2016.07.032
123. Clambey ET, McNamee EN, Westrich JA, Glover LE, Campbell EL, Jedlicka P, et al. Hypoxia-inducible factor-1 alpha-dependent induction of FoxP3 drives regulatory T-cell abundance and function during inflammatory hypoxia of the mucosa. Proc Natl Acad Sci USA (2012) 109:E2784–93. doi: 10.1073/pnas.1202366109
124. Zhao E, Wang L, Dai J, Kryczek I, Wei S, Vatan L, et al. Regulatory T cells in the bone marrow microenvironment in patients with prostate cancer. Oncoimmunology (2012) 1:152–61. doi: 10.4161/onci.1.2.18480
125. Karavitis J, Hix LM, Shi YH, Schultz RF, Khazaie K, Zhang M. Regulation of COX2 expression in mouse mammary tumor cells controls bone metastasis and PGE2-induction of regulatory T cell migration. PloS One (2012) 7:e46342. doi: 10.1371/journal.pone.0046342
126. Zhang J, Pang Y, Xie T, Zhu L. CXCR4 antagonism in combination with IDO1 inhibition weakens immune suppression and inhibits tumor growth in mouse breast cancer bone metastases. Onco Targets Ther (2019) 12:4985–92. doi: 10.2147/OTT.S200643
127. Núñez NG, Tosello Boari J, Ramos RN, Richer W, Cagnard N, Anderfuhren CD, et al. Tumor invasion in draining lymph nodes is associated with treg accumulation in breast cancer patients. Nat Commun (2020) 11:3272. doi: 10.1038/s41467-020-17046-2
128. Tan W, Zhang W, Strasner A, Grivennikov S, Cheng JQ, Hoffman RM, et al. Tumour-infiltrating regulatory T cells stimulate mammary cancer metastasis through RANKL-RANK signalling. Nature (2011) 470:548–53. doi: 10.1038/nature09707
129. Oh E, Hong J, Yun C-O. Regulatory T cells induce metastasis by increasing tgf-β and enhancing the epithelial–mesenchymal transition. Cells (2019) 8(11):1387. doi: 10.3390/cells8111387
130. Gasparri ML, Bellati F, Napoletano C, Panici PB, Nuti M. Interaction between treg cells and angiogenesis: a dark double track. Int J Cancer (2013) 132:2469. doi: 10.1002/ijc.27920
131. Giatromanolaki A, Bates GJ, Koukourakis MI, Sivridis E, Gatter KC, Harris AL, et al. The presence of tumor-infiltrating FOXP3+ lymphocytes correlates with intratumoral angiogenesis in endometrial cancer. Gynecol Oncol (2008) 110:216–21. doi: 10.1016/j.ygyno.2008.04.021
132. Yu X, Li H, Ren X. Interaction between regulatory T cells and cancer stem cells. Int J Cancer (2012) 131:1491–8. doi: 10.1002/ijc.27634
133. Zhan H-L, Gao X, Zhou X-F, Pu X-Y, Wang D-J. Presence of tumour-infiltrating FOXP3+ lymphocytes correlates with immature tumour angiogenesis in renal cell carcinomas. Asian Pac J Cancer Prev (2012) 13:867–72. doi: 10.7314/apjcp.2012.13.3.867
134. Lužnik Z, Anchouche S, Dana R, Yin J. Regulatory T cells in angiogenesis. J Immunol (2020) 205:2557–65. doi: 10.4049/jimmunol.2000574
135. Ferrara N, Gerber H-P, LeCouter J. The biology of VEGF and its receptors. Nat Med (2003) 9:669–76. doi: 10.1038/nm0603-669
136. Bencsikova B, Budinska E, Selingerova I, Pilatova K, Fedorova L, Greplova K, et al. Circulating T cell subsets are associated with clinical outcome of anti-VEGF-based 1st-line treatment of metastatic colorectal cancer patients: a prospective study with focus on primary tumor sidedness. BMC Cancer (2019) 19:687. doi: 10.1186/s12885-019-5909-5
137. Carvalho MI, Pires I, Prada J, Gregório H, Lobo L, Queiroga FL. Intratumoral FoxP3 expression is associated with angiogenesis and prognosis in malignant canine mammary tumors. Vet Immunol Immunopathol (2016) 178:1–9. doi: 10.1016/j.vetimm.2016.06.006
138. Facciabene A, Peng X, Hagemann IS, Balint K, Barchetti A, Wang L-P, et al. Tumour hypoxia promotes tolerance and angiogenesis via CCL28 and t(reg) cells. Nature (2011) 475:226–30. doi: 10.1038/nature10169
139. Morimoto Y, Tamura R, Ohara K, Kosugi K, Oishi Y, Kuranari Y, et al. Prognostic significance of VEGF receptors expression on the tumor cells in skull base chordoma. J Neurooncol (2019) 144:65–77. doi: 10.1007/s11060-019-03221-z
140. Ning H, Shao Q-Q, Ding K-J, Gao D-X, Lu Q, Cao Q-W, et al. Tumor-infiltrating regulatory T cells are positively correlated with angiogenic status in renal cell carcinoma. Chin (Engl) (2012) 125:2120–5. doi: 10.3760/cma.j.issn.0366-6999.2012.12.008
141. Gupta S, Joshi K, Wig JD, Arora SK. Intratumoral FOXP3 expression in infiltrating breast carcinoma: Its association with clinicopathologic parameters and angiogenesis. Acta Oncol (2007) 46:792–7. doi: 10.1080/02841860701233443
142. Kajal K, Bose S, Panda AK, Chakraborty D, Chakraborty S, Pati S, et al. Transcriptional regulation of VEGFA expression in T-regulatory cells from breast cancer patients. Cancer Immunol Immunother (2021) 70:1877–91. doi: 10.1007/s00262-020-02808-0
143. Casares N, Arribillaga L, Sarobe P, Dotor J, Lopez-Diaz de Cerio A, Melero I, et al. CD4+/CD25+ regulatory cells inhibit activation of tumor-primed CD4+ T cells with IFN-gamma-dependent antiangiogenic activity, as well as long-lasting tumor immunity elicited by peptide vaccination. J Immunol (2003) 171:5931–9. doi: 10.4049/jimmunol.171.11.5931
144. Beatty G, Paterson Y. IFN-gamma-dependent inhibition of tumor angiogenesis by tumor-infiltrating CD4+ T cells requires tumor responsiveness to IFN-gamma. J Immunol (2001) 166:2276–82. doi: 10.4049/jimmunol.166.4.2276
145. Adotevi O, Pere H, Ravel P, Haicheur N, Badoual C, Merillon N, et al. A decrease of regulatory T cells correlates with overall survival after sunitinib-based antiangiogenic therapy in metastatic renal cancer patients. J Immunother (2010) 33:991–8. doi: 10.1097/CJI.0b013e3181f4c208
146. Chen M-L, Yan B-S, Lu W-C, Chen M-H, Yu S-L, Yang P-C, et al. Sorafenib relieves cell-intrinsic and cell-extrinsic inhibitions of effector T cells in tumor microenvironment to augment antitumor immunity. Int J Cancer (2014) 134:319–31. doi: 10.1002/ijc.28362
147. Finke JH, Rini B, Ireland J, Rayman P, Richmond A, Golshayan A, et al. Sunitinib reverses type-1 immune suppression and decreases T-regulatory cells in renal cell carcinoma patients. Clin Cancer Res (2008) 14:6674–82. doi: 10.1158/1078-0432.CCR-07-5212
148. Suzuki H, Onishi H, Wada J, Yamasaki A, Tanaka H, Nakano K, et al. VEGFR2 is selectively expressed by FOXP3high CD4+ treg. Eur J Immunol (2010) 40:197–203. doi: 10.1002/eji.200939887
149. Terme M, Pernot S, Marcheteau E, Sandoval F, Benhamouda N, Colussi O, et al. VEGFA-VEGFR pathway blockade inhibits tumor-induced regulatory T-cell proliferation in colorectal cancer. Cancer Res (2013) 73:539–49. doi: 10.1158/0008-5472.CAN-12-2325
150. Zhu P, Hu C, Hui K, Jiang X. The role and significance of VEGFR2+ regulatory T cells in tumor immunity. Onco Targets Ther (2017) 10:4315–9. doi: 10.2147/OTT.S142085
151. Park SS, Choi YW, Kim J-H, Kim HS, Park TJ. Senescent tumor cells: an overlooked adversary in the battle against cancer. Exp Mol Med (2021) 53:1834–41. doi: 10.1038/s12276-021-00717-5
152. Wyld L, Bellantuono I, Tchkonia T, Morgan J, Turner O, Foss F, et al. Senescence and cancer: A review of clinical implications of senescence and senotherapies. Cancers (Basel) (2020) 12(8):2134. doi: 10.3390/cancers12082134
153. Liu X, Mo W, Ye J, Li L, Zhang Y, Hsueh EC, et al. Regulatory T cells trigger effector T cell DNA damage and senescence caused by metabolic competition. Nat Commun (2018) 9:249. doi: 10.1038/s41467-017-02689-5
154. Dimri GP, Lee X, Basile G, Acosta M, Scott G, Roskelley C, et al. A biomarker that identifies senescent human cells in culture and in aging skin in vivo. Proc Natl Acad Sci U.S.A. (1995) 92:9363–7. doi: 10.1073/pnas.92.20.9363
155. Vallejo AN. CD28 extinction in human T cells: altered functions and the program of T-cell senescence. Immunol Rev (2005) 205:158–69. doi: 10.1111/j.0105-2896.2005.00256.x
156. Ye J, Huang X, Hsueh EC, Zhang Q, Ma C, Zhang Y, et al. Human regulatory T cells induce T-lymphocyte senescence. Blood (2012) 120:2021–31. doi: 10.1182/blood-2012-03-416040
157. Ye J, Ma C, Hsueh EC, Eickhoff CS, Zhang Y, Varvares MA, et al. Tumor-derived γδ regulatory T cells suppress innate and adaptive immunity through the induction of immunosenescence. J Immunol (2013) 190:2403–14. doi: 10.4049/jimmunol.1202369
158. Li L, Liu X, Sanders KL, Edwards JL, Ye J, Si F, et al. TLR8-mediated metabolic control of human treg function: A mechanistic target for cancer immunotherapy. Cell Metab (2019) 29:103–123.e5. doi: 10.1016/j.cmet.2018.09.020
159. Liu X, Hoft DF, Peng G. Senescent T cells within suppressive tumor microenvironments: emerging target for tumor immunotherapy. J Clin Invest (2020) 130:1073–83. doi: 10.1172/JCI133679
160. Imamura K, Ogura T, Kishimoto A, Kaminishi M, Esumi H. Cell cycle regulation via p53 phosphorylation by a 5’-AMP activated protein kinase activator, 5-aminoimidazole- 4-carboxamide-1-beta-D-ribofuranoside, in a human hepatocellular carcinoma cell line. Biochem Biophys Res Commun (2001) 287:562–7. doi: 10.1006/bbrc.2001.5627
161. Liang J, Shao SH, Xu Z-X, Hennessy B, Ding Z, Larrea M, et al. The energy sensing LKB1-AMPK pathway regulates p27(kip1) phosphorylation mediating the decision to enter autophagy or apoptosis. Nat Cell Biol (2007) 9:218–24. doi: 10.1038/ncb1537
162. van Nguyen T, Puebla-Osorio N, Pang H, Dujka ME, Zhu C. DNA Damage-induced cellular senescence is sufficient to suppress tumorigenesis: a mouse model. J Exp Med (2007) 204:1453–61. doi: 10.1084/jem.20062453
163. Domblides C, Lartigue L, Faustin B. Control of the antitumor immune response by cancer metabolism. Cells (2019) 8(2):104. doi: 10.3390/cells8020104
164. Michalek RD, Gerriets VA, Jacobs SR, Macintyre AN, MacIver NJ, Mason EF, et al. Cutting edge: Distinct glycolytic and lipid oxidative metabolic programs are essential for effector and regulatory CD4+ T cell subsets. J Immunol (2011) 186:3299–303. doi: 10.4049/jimmunol.1003613
165. Shi LZ, Wang R, Huang G, Vogel P, Neale G, Green DR, et al. HIF1alpha-dependent glycolytic pathway orchestrates a metabolic checkpoint for the differentiation of TH17 and treg cells. J Exp Med (2011) 208:1367–76. doi: 10.1084/jem.20110278
166. Kumagai S, Koyama S, Itahashi K, Tanegashima T, Lin Y-T, Togashi Y, et al. Lactic acid promotes PD-1 expression in regulatory T cells in highly glycolytic tumor microenvironments. Cancer Cell (2022) 40:201–218.e9. doi: 10.1016/j.ccell.2022.01.001
167. Watson MJ, Vignali PD, Mullett SJ, Overacre-Delgoffe AE, Peralta RM, Grebinoski S, et al. Metabolic support of tumour-infiltrating regulatory T cells by lactic acid. Nature (2021) 591:645–51. doi: 10.1038/s41586-020-03045-2
168. Zappasodi R, Serganova I, Cohen IJ, Maeda M, Shindo M, Senbabaoglu Y, et al. CTLA-4 blockade drives loss of treg stability in glycolysis-low tumours. Nature (2021) 591:652–8. doi: 10.1038/s41586-021-03326-4
169. Chapman NM, Zeng H, Nguyen T-LM, Wang Y, Vogel P, Dhungana Y, et al. mTOR coordinates transcriptional programs and mitochondrial metabolism of activated treg subsets to protect tissue homeostasis. Nat Commun (2018) 9:2095. doi: 10.1038/s41467-018-04392-5
170. Fu Z, Ye J, Dean JW, Bostick JW, Weinberg SE, Xiong L, et al. Requirement of mitochondrial transcription factor a in tissue-resident regulatory T cell maintenance and function. Cell Rep (2019) 28:159–171.e4. doi: 10.1016/j.celrep.2019.06.024
171. Saravia J, Zeng H, Dhungana Y, Bastardo Blanco D, Nguyen T-LM, Chapman NM, et al. Homeostasis and transitional activation of regulatory T cells require c-myc. Sci Adv (2020) 6:eaaw6443. doi: 10.1126/sciadv.aaw6443
172. Weinberg SE, Singer BD, Steinert EM, Martinez CA, Mehta MM, Martínez-Reyes I, et al. Mitochondrial complex III is essential for suppressive function of regulatory T cells. Nature (2019) 565:495–9. doi: 10.1038/s41586-018-0846-z
173. Wang H, Franco F, Tsui Y-C, Xie X, Trefny MP, Zappasodi R, et al. CD36-mediated metabolic adaptation supports regulatory T cell survival and function in tumors. Nat Immunol (2020) 21:298–308. doi: 10.1038/s41590-019-0589-5
174. Field CS, Baixauli F, Kyle RL, Puleston DJ, Cameron AM, Sanin DE, et al. Mitochondrial integrity regulated by lipid metabolism is a cell-intrinsic checkpoint for treg suppressive function. Cell Metab (2020) 31:422–437.e5. doi: 10.1016/j.cmet.2019.11.021
175. Patwardhan RS, Singh B, Pal D, Checker R, Bandekar M, Sharma D, et al. Redox regulation of regulatory T-cell differentiation and functions. Free Radic Res (2020) 54:947–60. doi: 10.1080/10715762.2020.1745202
176. Yin M, O’Neill LA. The role of the electron transport chain in immunity. FASEB J (2021) 35:e21974. doi: 10.1096/fj.202101161R
177. Mougiakakos D, Johansson CC, Jitschin R, Böttcher M, Kiessling R. Increased thioredoxin-1 production in human naturally occurring regulatory T cells confers enhanced tolerance to oxidative stress. Blood (2011) 117:857–61. doi: 10.1182/blood-2010-09-307041
178. Mougiakakos D, Johansson CC, Kiessling R. Naturally occurring regulatory T cells show reduced sensitivity toward oxidative stress-induced cell death. Blood (2009) 113:3542–5. doi: 10.1182/blood-2008-09-181040
179. Thomas-Schoemann A, Batteux F, Mongaret C, Nicco C, Chéreau C, Annereau M, et al. Arsenic trioxide exerts antitumor activity through regulatory T cell depletion mediated by oxidative stress in a murine model of colon cancer. J Immunol (2012) 189:5171–7. doi: 10.4049/jimmunol.1103094
180. Xu C, Sun S, Johnson T, Qi R, Zhang S, Zhang J, et al. The glutathione peroxidase Gpx4 prevents lipid peroxidation and ferroptosis to sustain treg cell activation and suppression of antitumor immunity. Cell Rep (2021) 35:109235. doi: 10.1016/j.celrep.2021.109235
181. Chen Z, Barbi J, Bu S, Yang H-Y, Li Z, Gao Y, et al. The ubiquitin ligase Stub1 negatively modulates regulatory T cell suppressive activity by promoting degradation of the transcription factor Foxp3. Immunity (2013) 39:272–85. doi: 10.1016/j.immuni.2013.08.006
182. van Loosdregt J, Fleskens V, Tiemessen MM, Mokry M, van Boxtel R, Meerding J, et al. Canonical wnt signaling negatively modulates regulatory T cell function. Immunity (2013) 39:298–310. doi: 10.1016/j.immuni.2013.07.019
183. Yang J, Wei P, Barbi J, Huang Q, Yang E, Bai Y, et al. The deubiquitinase USP44 promotes treg function during inflammation by preventing FOXP3 degradation. EMBO Rep (2020) 21:e50308. doi: 10.15252/embr.202050308
184. Zhou X, Bailey-Bucktrout SL, Jeker LT, Penaranda C, Martínez-Llordella M, Ashby M, et al. Instability of the transcription factor Foxp3 leads to the generation of pathogenic memory T cells in vivo. Nat Immunol (2009) 10:1000–7. doi: 10.1038/ni.1774
185. Wan YY, Flavell RA. Regulatory T-cell functions are subverted and converted owing to attenuated Foxp3 expression. Nature (2007) 445:766–70. doi: 10.1038/nature05479
186. Burzyn D, Benoist C, Mathis D. Regulatory T cells in nonlymphoid tissues. Nat Immunol (2013) 14:1007–13. doi: 10.1038/ni.2683
187. Delacher M, Imbusch CD, Hotz-Wagenblatt A, Mallm J-P, Bauer K, Simon M, et al. Precursors for nonlymphoid-tissue treg cells reside in secondary lymphoid organs and are programmed by the transcription factor BATF. Immunity (2020) 52:295–312.e11. doi: 10.1016/j.immuni.2019.12.002
Keywords: regulatory T Cells, FOXP3+, tumour microenvironment, immune escape, immune metabolism
Citation: Qiu Y, Ke S, Chen J, Qin Z, Zhang W, Yuan Y, Meng D, Zhao G, Wu K, Li B and Li D (2022) FOXP3+ regulatory T cells and the immune escape in solid tumours. Front. Immunol. 13:982986. doi: 10.3389/fimmu.2022.982986
Received: 30 June 2022; Accepted: 01 September 2022;
Published: 13 October 2022.
Edited by:
Paula D. Bos, Virginia Commonwealth University, United StatesReviewed by:
Kai Yang, Indiana University School of Medicine-Lafayette, United StatesMaria Bettini, The University of Utah, United States
Copyright © 2022 Qiu, Ke, Chen, Qin, Zhang, Yuan, Meng, Zhao, Wu, Li and Li. This is an open-access article distributed under the terms of the Creative Commons Attribution License (CC BY). The use, distribution or reproduction in other forums is permitted, provided the original author(s) and the copyright owner(s) are credited and that the original publication in this journal is cited, in accordance with accepted academic practice. No use, distribution or reproduction is permitted which does not comply with these terms.
*Correspondence: Kejin Wu, d3VrZWppbjE3NjJAZmNreXkub3JnLmNu; Dan Li, ZGFubGlAc2hzbXUuZWR1LmNu; Bin Li, YmlubGlAc2hzbXUuZWR1LmNu
†These authors have contributed equally to this work