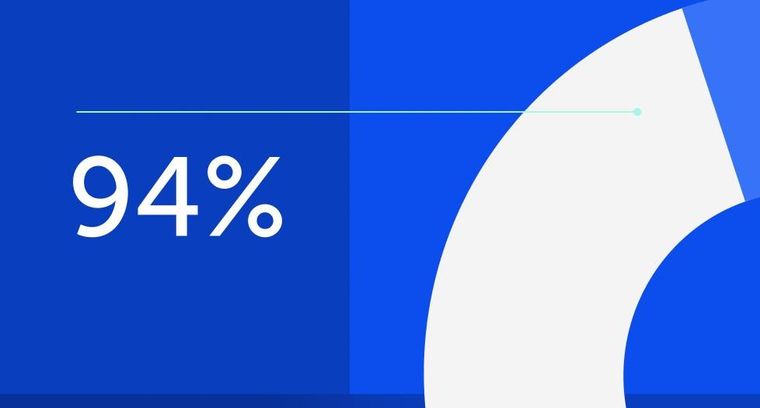
94% of researchers rate our articles as excellent or good
Learn more about the work of our research integrity team to safeguard the quality of each article we publish.
Find out more
REVIEW article
Front. Immunol., 20 September 2022
Sec. Autoimmune and Autoinflammatory Disorders : Autoimmune Disorders
Volume 13 - 2022 | https://doi.org/10.3389/fimmu.2022.952398
This article is part of the Research TopicOrthodox vs Paradox: The Roles of Glycomics, Genetics and Beyond in Immunity, Immune Disorders and GlycomedicineView all 12 articles
Carbohydrates serve as important energy sources and structural substances for human body as well as for gut microbes. As evidenced by the advances in immunometabolism, glucose metabolism and adenosine triphosphate (ATP) generation are deeply involved in immune cell activation, proliferation, and signaling transduction as well as trafficking and effector functions, thus contributing to immune response programming and assisting in host adaption to microenvironment changes. Increased glucose uptake, aberrant expression of glucose transporter 1 (e.g., GLU1), and abnormal glycosylation patterns have been identified in autoimmunity and are suggested as partially responsible for the dysregulated immune response and the modification of gut microbiome composition in the autoimmune pathogenesis. The interaction between gut microbiota and host carbohydrate metabolism is complex and bidirectional. Their impact on host immune homeostasis and the development of autoimmune diseases remains to be elucidated. This review summarized the current knowledge on the crosstalk of glucose metabolism and glycosylation in the host with intestinal microbiota and discussed their possible role in the development and progression of autoimmune diseases. Potential therapeutic strategies targeting glucose metabolism and glycosylation in modulating gut ecosystem and treating autoimmune diseases were discussed as well.
Accumulated evidence showed an increased glucose uptake in autoimmune diseases as revealed by 18F-FDG PET-CT (1) and implied the impact of distinct glucose metabolic pathways on the immune system. Glucose, serving as an important energy resource and the major substrate for adenosine triphosphate (ATP) generation, is deeply involved in multiple physiological and pathological bioactivities such as immune cell activation, proliferation, signaling transduction, leukocyte trafficking, and cytokine production. Based on the current evidence, immune cells can modulate their cytology to accommodate environmental glucose levels. T cells separated from mice with hyperglycemia showed a more active response to T cell receptor (TCR) stimulation, with accelerated proliferation of CD4+ T cells and a higher expression of Th1, Th2, and Th17 cytokines compared with those separated from healthy controls (2), indicating the proinflammatory effects of hyperglycemia circumstances. Glucose deprivation or restricted energy availability steers the metabolic preference from glycolysis towards fatty acid oxidation and consequently adjusts cell fate and polarization; thus, it can be taken as an immune-metabolic checkpoint (3).
During activation, T cells undergo increased glycolysis and oxidative phosphorylation (OXPHOS) to meet the energy demand for proliferation and effector functions (4). Not surprisingly, the upregulation of glycolysis leads to the restricted but rapid generation of ATP and nucleotides, amino acids, and fatty acids synthesis, which are important for immune cells to fulfill multifold tasks, e.g., cytokine production and proliferation, and switch to inflammatory phenotypes. Increased glucose uptake and accelerated glycolysis are indispensable for immune cells to adapt to microenvironment changes and to cope with external stimuli like infectious pathogens.
Glucose can chemically attach to proteins and nucleic acids without the aid of enzymes, and excessive nonenzymatic glycosylation is involved in multiple biological effects such as inhibiting regulatory molecule binding, decreasing proteolysis susceptibility, influencing nucleic acid function, altering macromolecular endocytosis, and increasing immunogenicity (5). What is more, glycoproteins count on carbohydrates to achieve a proper three-dimensional conformation which is critical for the function and bioactivity of glycoprotein.
Fucosylation is one of the major glycosylation patterns in the human body and is of utmost importance in immune cell maturation, inflammatory cytokine secretion, and gut bacteria adhesion (6). Increased levels of fucosylation had been shown in patients with systemic lupus erythematosus (SLE) and was positively correlated with disease severity (7). Meanwhile, studies have shown that the state of gut microbes is largely regulated by their own carbohydrate modification and host dietary intake as well as host glycosylation (8–16). Both glucose metabolism and microbiota composition as well as the interaction between them are suggested to participate in host immune regulation (17–23). Dysbiosis of intestinal microbiota, such as a lower Shannon diversity index, symbiont translocation, and pathobiont enrichment, has been documented in many types of autoimmune diseases like SLE, rheumatoid arthritis (RA), type 1 diabetes mellitus (T1DM), and multiple sclerosis (MS) with the potential of promoting aberrant immune activation and self-tolerance breakdown (24–29). As a beneficial paradigm, Akkermansia muciniphila, a species of human commensal bacterium with mucin-degrading capability that abundantly resides in the mucus layer, can counteract inflammation-associated insulin resistance, display favorable metabolic effects on host glucose and adipose metabolism, reinforce intestinal barrier function, and influence host immune responses. Indeed A. muciniphila has shown therapeutic potentials in several inflammation-related diseases, including inflammatory bowel disease (IBD) and diabetes (30–32). In autoimmune diseases characterized by the overactivation of immune cells and aberrant self-attack (33), how glycosylation links glucose metabolism to the multiple physiopathological changes in the immune system and gut ecosystem is of great interest. However, studies on the molecular mechanism linkage of autoimmune diseases with glucose metabolism and glycosylation and their interaction with the gut microbiota were limited.
By analyzing the contribution of distinct glucose metabolic pathways to cell fate determination (34, 35), glycomics provides novel insights into the molecular metabolic pathogenesis of autoimmune diseases. Further research dissecting the complex mutual influence of host glucose metabolism and gut microbiota, together with host immune system programming, might help to exploit new targets or novel intervention strategies to control these diseases. In this review, the interaction of carbohydrate metabolism pathways and glycosylation with the intestinal microbiota and host immune response was discussed based on current knowledge and research. We also discussed their role in the development of autoimmune diseases and the potentials of measures targeting glucose metabolism and microbiota in the treatment of autoimmune diseases.
Glucose metabolism is fine-tuned to accommodate the cell demand for energy and biosynthesis. Glycolysis is a fast way to acquire energy, though not as efficient as OXPHOS in the mitochondria, and it is of preference for cells in rapid growth and proliferation. Interestingly, pro-inflammatory cells (e.g., effector T cells, type 1 macrophage) seemed to more preferentially rely on glycolysis process to acquire ATP for their fate determination and cytokine production, whereas regulatory cells (e.g., regulatory T cell, type 2 macrophage) might rely more on mitochondrial OXPHOS for their function (36). During glycolysis, glucose is catabolized into pyruvate and lactate which can subsequently be converted into acetyl-CoA to fuel the tricarboxylic acid cycle.
There are three glucose catabolic pathways: aerobic oxidation, anaerobic glycolysis, and pentose phosphate pathway (37). Among all three pathways, hexokinase (HK) is in the first step and the vital enzyme crucial in modulating the intracellular glucose concentration.
As known, mammalian target of rapamycin (mTOR) is also a key regulator in immunometabolism that can regulate glucose uptake and glycolysis in immune cells and, coupled with phosphoinositide 3-kinase/Akt, can modulate diverse cell activities.
Gluconeogenesis typically occurs in the liver but is unveiled recently as a novel function in the gut metabolism. The human intestinal mucosa can express glucose 6-phosphatase and phosphoenolpyruvate carboxykinase gene which is responsible for gluconeogenesis. It is suggested that dietary fiber fermentation products—butyrate and propionate—can induce gluconeogenesis gene expression and benefit body energy homeostasis (38).
The existence of carbohydrates in the human body is presented in several forms: monosaccharides (e.g., fructose, galactose, sucrose, ribose), complex carbohydrates (e.g., oligosaccharides or polysaccharides), and glycoconjugates (e.g., glycoprotein, glycolipid) (39). Sugars from the daily diet are digested to provide energy source through catabolic pathways or produce structural substances (e.g., fatty acids, FAs) through anabolic pathways (39). Some intermediate products formed in carbohydrate catabolism become raw materials and carbon frames for synthesizing biological macromolecules such as lipids, proteins, and nucleic acids. Small non-carbohydrate substances can also be converted into carbohydrate molecules or covalently polymerized with carbohydrate molecules to form glycoconjugates in the form of glycoproteins, proteoglycans, glycolipids, and glycophosphatidylinositol-linked proteins (40) and take part in diverse human physiological and biochemical processes.
A layer of glycocalyx covers almost every cell surface in human organs, which is a highly hydrated gel-like layer composed of a meshwork of proteoglycans, glycoproteins, glycosaminoglycans, and glycolipids and assembled with proteins embedded in the plasma membrane to form the external cell surface (41). Mounting evidence has revealed the function of endothelial glycocalyx in maintaining vasculature homeostasis by acting as vascular permeability barrier, physical force transmitter, antagonist of adhesion, and oxidative stress shield, etc. (41, 42). Under vascular pathological circumstances, the endothelial glycocalyx can be damaged by hypervolemia or hyperglycemia (43). The glycocalyx is involved in gut epithelial mucosal barrier formation as well, which is mainly composed of sulfated mucin glycoproteins produced by goblet cells (44–46). The gut barrier prevents the invasion of enteric pathogens, and its disruption can cause a “leaky gut” and subject the host to microbial invasion and translocation, which has been reported in multiple autoimmune diseases. In addition, the mucin barrier of the gastrointestinal epithelium might be disrupted under certain a pathological state like IBD, which brings the collapse of its barrier function and promotes bacterial adherence (47, 48). The disruption was found related with both host genetic susceptibility and environmental etiologies (49, 50).
The surfaces of immune cells are also equipped with a mixture of glycoconjugates with complex glycosylation, which play a vital role in regulating immune cell maturation and differentiation (51, 52).
By appending monosaccharide residues to saccharides, peptides, and lipids with glycosidic bonds, catalyzed by glycosyltransferase, glycoconjugates are formed, and the glycosylation process can transform molecular conformation and even alter the antigenicity. Human glycans are basically composed of 10 monosaccharides, including L-fucose, D-galactose, GalNAc, N-acetylglucosamine (GlcNAc), etc. (53). Glycosylation of protein is an important way of post-translational modification and ubiquitously involved in human physical activities. Based on whether adding a monosaccharide residue to the free-NH2 group or to the oxygen atom of the hydroxyl group in a polypeptide chain, protein glycosylation can be categorized into N-glycosylation and O-glycosylation (47, 53, 54). Though not completely understood, the regulation of the glycosylation process and the glycosyltransferase expression are determined by cell types, respond actively to environmental changes, and participate in cell–cell interactions and distinct protein activity regulation (47, 53). Fucosylation is one of the major forms of glycosylation with in-depth studies and known to participate in the formation of ABO blood group antigen determinants, Lewis antigen determinants, and selectin ligands (6). Fucosylation is accomplished with fucosyltransferases, GDP-fucose synthetic enzymes, and GDP-fucose transporter. Studies found that certain genes in the fucosylation process can upregulate the expression of PD-1 on the cell surface, thus subsequently inactivating the T cells and decreasing the alert threshold towards tumor cells (55). Furthermore, fucosylation of some classifications has been found to have a positive correlation with TNF expression (6), contributing to the inflammation.
Immune cells undergo metabolic reprogramming upon antigen challenge. The development, proliferation, and cytokine production of cytotoxic and effector T cells (Teff) rely greatly on glucose metabolism with increased glucose transportation via glucose transporter 1 (56), accelerating glycolysis and the bioactive intermediates of the glycolytic pathway (57). The switch of oxidative phosphorylation to aerobic glycolysis is regarded as a hallmark of Teff cell activation (58, 59). Intriguingly, Treg shows unique energetic metabolism by relying on glycolysis to support its proliferation and migration whereas relying on OXPHOS (36) to maintain its suppressive capacity. Glycolysis suppression may reprogram T cell differentiation toward Treg and mTOR inhibitor by inhibiting glucose uptake and inducing hypoxia-inducible factor-1α, subsequently leading to the upregulation of FOXP3 expression, which may partially account for the immune tolerance restoring effects of rapamycin. Foxp3, the well-known transcription factor of Treg, functions to suppress glycolysis and enhance oxidative phosphorylation, thus reprogramming Treg cell metabolism to accommodate low glucose and high lactate conditions (57).
Immune cells also respond to the change of glucose level in the environment with metabolic adjustment, which, in turn, alters their own immune function. Long-term carbohydrate and caloric restriction (CR) lowers the inflammation risks but maintains the T cell repertoire (3, 60). Data from several studies suggested that CR led to the reduction of circulating inflammation markers, including lymphocytes, monocytes, and dendritic cells (DCs) (17–19), but increased the retention of pro-inflammatory monocytes and T cells in the bone marrow (20, 21). Jordan et al. (18) used HK inhibitors to block glycolysis in mice and found a similar shrink of a pool of circulating monocytes, which is mediated by the activation of 5-AMP-activated protein kinase, a key cellular energy sensor (18, 61).
Based on the current evidence, glucose deprivation or restricted energy availability steers the metabolic preference from glycolysis towards fatty acid oxidation and consequently transforms cell fate and polarization; thus, it can be taken as an immune-metabolic checkpoint (3).
Innate immune response is the first line to defend against microorganism invasion, which is initiated through the recognition of pathogen-associated molecular patterns (PAMPs) or damage-associated molecular patterns (DAMPs) from pathogens with pattern recognition receptors (PRRs) (e.g., Toll-like receptors, TLRs) either secreted by or anchored in the membrane of innate immune cells (Table 1). Most PAMPs have glycan components (47, 62), and bacteria also produce extracellular glycocalyx to mediate cell attachment, protect against antibacterial agents, retain humidity, etc. (64)—for example, lipopolysaccharide (LPS) from Gram-negative bacteria, known as endotoxin and the most studied PAMP, is a glycolipid that can induce an acute or chronic inflammatory reaction via TLR4 recognition and subsequent NF-κB activation and is associated with a poor prognosis of sepsis. LPS is mainly produced by gut microbes, and increased serum LPS may act as a clue of damaged gut mucus barrier which has been reported in some immune-mediated diseases such as SLE and IBD. Recently, it is suggested that LPS exposure in early life may induce endotoxin tolerance, help suppress Th-2 skewing effects, and modify allergic response, which may act as supportive evidence of the hygiene hypothesis (63). Polysaccharide A (PSA) from Bacteroides fragilis is another typical PAMPs, recognized by TLR2, and activates downstream MyD88- and TRIF-dependent pathways, allowing the translocation of transcription factors like NF-κB, AP-1, IRF5, and IRF7 into the nucleus, promoting the expression of inflammatory cytokine genes, and increasing the production of NO and secretion of TNFα in macrophages (22). PRR like TLRs are also heavily glycosylated themselves (62). The glycosylation sites of TLR determine its three-dimensional features and affect its function (47). Lectins are a group of carbohydrate-binding proteins with opsonization capability to mediate the attachment and binding of bacteria by aggregation (23). C-type lectins belonging to PRRs have a specific affinity to different glycosyls and can trigger a series of downstream immunoreactions including T cell activity regulation, cell adhesion, and cytokine secretion (72). Their function as information transmitter in regulating immune response and autoimmunity is coded by diverse glycosylation. According to their affinity to distinct monosaccharide, lectins can be classified into five groups: mannose, galactose/GalNAc, GlcNAc, fucose, and N-acetylneuraminic acid (73). Mannose-binding lectins (MBLs) have a high binding specificity with mannose and GlcNAc. The MBL pathway, as one of the three activation pathways in the complement system, is significant in innate, nonspecific immunity, especially in the initial immune response to sugar-decorated pathogen (74). MBLs and ficolins (another type of lectins in humans that can only bind with GlcNAc) can discriminate self and non-self and bind with carbohydrate groups on the surface of bacteria, fungi, or other pathogens through a COOH terminal carbohydrate recognition domain and activate MBL-associated serine protease (MASP) by a configuration change. MASP1cleaves C3 directly; MASP2 catalyzes the production of C4b2a (C3 convertase), thus triggering the complement cascade by the classical pathway (23). Once C3 is cleaved and C3b is generated, C3b (or C4b, iC3b) is fixed to the bacterial cell surface and binds with complement receptor 1 (CR1) (or CR3, CR4), which is expressed on the surface of phagocytic cells (macrophages, neutrophils, monocytes, etc.), and phagocytosis of these bacterial cells is initiated, which is also called opsonization (74–77). The galectins are a group of soluble members of lectin family, excreted by immune cells, endothelial cells, or epithelial cells, and can lead the microbes to the target cells (47, 78) (Figure 1).
Table 1 Summary about pathogen-associated molecular patterns (PAMPs), damage-associated molecular patterns (DAMPs), and pattern recognition receptors (PRRs) with their characteristics.
Figure 1 Roles of carbohydrates in immune cells. Carbohydrates are involved in immune response against bacteria at the cellular level. Glycans contained in the glycocalyx of bacteria serve as pathogen-associated molecular patterns that bind with pattern recognition receptors (e.g., Toll-like receptor and mannose-binding lectin) and activate downstream immune response pathways, either promoting the expression of inflammatory genes or activating the complement cascade and eliminating pathogens. Bacteria can also be presented by antigen-presenting cells via the MHCII pathway, which requires NO to help degrade bacterial polysaccharide A into small molecules and present them to CD4+ T cells.
DCs are an important component in innate immunity as the professional antigen-presenting cells. Brigitta et al. verified that the nonenzymatic glycosylation of human β2 glycoprotein I (β2GPI) was capable of activating immature DCs, priming of Th2 cell differentiation, and triggering the signaling pathway of ERK, p38 MAPK, and NF-κB. Glycosylation is known to be capable of influencing the immunogenicity of proteins, so it is rational to postulate that glycation of β2GPI may lead to the exposure of cryptic epitope or the formation of neo-determinants to trigger immunogenic DC (79).
Glycolysis induced by mTOR-HIF1α is also involved in neutrophil migration, neutrophil extracellular trap production, and killing activity (80).
Exploration of the role of carbohydrates and glycosylation on adaptive immune responses revealed that proper MHCII N-glycosylation is critical for the binding and presentation of PSA to CD4+ T cells for their subsequent activation and proliferation (81–83). Mice treated with mannosidase inhibitor to interfere N-glycan complex formation would lose the ability to expand PSA-specific T cells, while the transfer of normally glycosylated APCs to the mice helps restore the ability (84). Other studies found the exposure to PSA results in the upregulation of IFNγ, TNFα, IL-6, CXCL10, and surface and intracellular markers associated with M2 macrophages (85, 86) (Figure 1).
During inflammatory response, leukocyte adhesion and extravasation, homing to lymphoid organs, and recruitment to peripheral tissues are closely linked with selectins. Selectins are a group of C-type lectins that are found in the Weibel–Palade bodies of endothelial cells and platelet α-granules (P-selectins), on the surface of skin endothelial cells (E-selectins), and on the surface of most leukocytes (L-selectins) and are inducibly expressed during inflammatory responses (87–90). Most selectin ligands contain a glycan epitope named sialyl Lewis x (sLex) with fucose and sialic acid-decorated carbohydrate chains (90, 91). The main selectin ligand expressed by all calculating leukocytes, P-selectin glycoprotein ligand-1 (PSGL-1), supports the capture and rolling of a broad range of leukocytes (monocytes, DCs, mast cells, NK cells, neutrophils, basophils, eosinophils, activated T cells, and Treg cells) (88, 92). Therefore, malfunction of selectin due to the aberrant structure of selectins and their ligands mediated the impaired leukocyte trafficking which participated in the pathogenesis of autoimmune diseases, including MS, autoimmune skin inflammation, IBD, T1DM, RA, SLE, and systemic sclerosis (88) (Figure 2A).
Figure 2 Roles of carbohydrates in blood vessel endothelium and intestine epithelium. (A) Selectins and selectin ligands participate in leukocyte trafficking in immune response and autoimmune diseases. E-selectins and P-selectins expressed on the blood vessel wall bind with PSGL-1 and other selectin ligands expressed on the surface of circulating leukocytes, controlling their adhesion and recruitment to the endothelium of CNS venules (in multiple sclerosis), dermal vessels (in autoimmune skin inflammation), ileum and colon vessels (in inflammatory bowel disease), joint synovium (in rheumatoid arthritis), etc. (B) Carbohydrates and glycosylation mediate the interactions between the bacteria and the intestinal epithelial cells in a variety of approaches. The adhesion and the colonization of bacteria can be mediated by their cell surface glycans binding specifically with receptors on intestinal epithelial cells (IECs). The virulence of bacteria can be influenced as glycogroups on IEC function as bacterial toxin targets, and their breakdown releases products that regulate the expression of bacterial virulence genes. Certain species of bacteria can degrade IEC glycosyls and utilize them, thus influencing the condition of both the bacteria and the intestine epithelium, and several bacterial species can induce the fucosylation initiation of IECs.
Immune response mediated by antibodies, including phagocytosis, antibody-dependent cell-mediated cytotoxicity (ADCC), and complement activation involves the immunoregulatory role of glycosylation as well (93). IgG is the most abundant immunoglobulin in human serum. Each one of the two heavy chains of an IgG molecule contains an N-linked glycan at Asn-297 in its constant region (Fc), with the ingredients of the glycan including GlcNAc, fucose, mannose, galactose, and sialic acid (94). Distinct glycan modifications affect the shapes of the hinge or the CH2–CH3 interface of Fc domains and determine the specificity of receptor binding (94, 95). Fc gamma receptor I (FcγRI) is the only type of high-affinity IgG Fc receptors in promoting immune response against bacterial infection and may contribute to several autoimmune diseases. A significant structure to determine its higher affinity is the bond between the receptor D2 domain FG loop and the proximal carbohydrate units (GlcNAc) of Fc glycan from IgG, in the form of a hydrogen bond (95, 96). The FG loop of FcγRII and FcγRIII instead has a distance too far to form a hydrogen bond with the glycan of the IgG Fc domain, which leads to a lower affinity of these two receptors (95). Furthermore, the fucosylated Asn-162 of FcγRIIIA was found to bind with the nonfucosylated glycan of Fc more stably, and studies revealed that FcγRIIIA reached a significantly higher affinity to a fucosylated IgG (absence of core fucose at Asn-297) than fucosylated IgG, which means that the core fucosylation of the Fc domain inhibits the binding of IgG to FcγRIIIA, thus inhibiting ADCC (95, 97–99). On the contrary, the addition of GlcNAc and sialic acid might have the effect of promoting the affinity (100, 101).
Various kinds of carbohydrates and glycosyls exert bidirectional effects on the colonization of different bacteria species in the gut and contribute to the establishment of healthy infant gut microbiota (9, 102, 103). Human intestinal epithelial cells (IEC) mainly carry α (1, 2)-fucosylated glycans, which constitute an important part of the interface between host and microbiota (104). The protective intestinal (also respiratory and genitourinary) mucus layer is built up with large glycoproteins called mucins (MUC) in high density, which is characterized by high O-glycan ratio binding with water (47). MUC2, the major normal mucin in the intestinal tract, forms trimers once secreted and becomes an ideal net-like filter (105). The composition, function, and regulation of mucins are complex and only limitedly understood (45), but mucus glycosylation defection has been implied to be associated with gut flora diversity reduction and composition alteration (8) as exemplified by the finding that Fut2-deficient mice have lower intestinal flora diversity with a shrinking population of an unclassified genus of Clostridiales and expanded Parabacteroides, Eubacterium, Parasutterella, Bacteroides, and family Lachnospiraceae (9, 104), whereas Johansson et al. (106) found that germ-free (GF) mice had increased mucus penetrability and a decreased amount of MUC2 in colon mucus compared to conventional mice but can develop impenetrable mucus and normalized MUC2 after the colonization of conventional cecal flora, demonstrating the contribution of intestinal microbes to the development and glycosylation pattern of mucin.
The carbohydrate modification of mucin domain determines the selection and adhesion of colonized bacteria partially via single carbohydrate epitope–protein interactions. As described earlier, bacteria express a layer of extracellular glycocalyx, consisting of adhesive structures named adhesins, whose interaction with host cells are mediated by either protein or glycan (45, 47, 107). The colonization of Escherichia coli (E. coli) is mediated by its P pili (Figure 2B) containing a terminal PapG unit that specifically binds with a digalactoside receptor determinant on gastrointestinal and urinary tract epithelial cells (10, 11, 47). Another study revealed that E. coli and Salmonella typhimurium bind to glycoprotein 2 on the epithelial M cells via bacterial FimH on their pilis (12, 104), and Vibrio parahaemolyticus targets the sulfated and fucosylated glycans on the surface of epithelial cells (13, 104).
The virulence of intestinal bacteria is also affected by the glycocalyx structure (Figure 2B). Vibrio cholera produces cholera toxin which binds to GM1 ganglioside, a type of glycolipid molecule expressed on epithelial cells and which conducts downstream signals to cause watery diarrhea. Galactose and sialic acid compose the sugar moieties of GM1 and might be responsible for the binding with cholera toxin (104, 108). Another study reported that L-fucose released from epithelial cells could inhibit the expression of virulence genes of enterohemorrhagic E. coli through a chemical sensing system of the bacteria called FusKR (14, 104).
Carbohydrate fermentation and absorption occurs in the gut, and the decomposition of dietary fibers rely on symbionts residing in the gut (Figure 2B). Apart from dietary starch, protein, and fiber (109) in the small intestine, some bacteria species, e.g., Bacteroides thetaiotaomicron, are able to express enzymes to sensor, transport, and degrade glycosyls from the host into short fatty acids (SCFAs) and acquire energy and structure material (45, 110). SCFAs serve as not only energy substrates but also immunomodulators via binding to G-protein-coupled receptors (GPCRs) that can induce DC tolerogenic phenotype, promote Treg differentiation, facilitate M2 macrophage conversion, and protect gut homeostasis. Upon stimulation of SCFAs, GPCRs would induce the production of peptide YY, modulating intestinal motility and nutrient absorption (111, 112). Different bacteria have different survival status on O-glycan dense mucus.
In addition, maternal milk contains a thousand types of fucosylated components and a large amount of oligosaccharides (human milk oligosaccharides) which can inhibit the binding of Campylobacter jejuni (C. jejuni) with fucosyl and prevent diseases associated with it. Both in vitro experiment of human epithelial cells and a mouse model infected in vivo with C. jejuni have proved that 2′-fucosyllactose (2′-FL), the dominant glycosyls in milk, inhibited the colonization, resisted the infection of C. jejuni, and mitigated the intestinal mucosa immune response towards C. jejuni (113, 114). 2′-FL can also be utilized by other species of bacteria like Bacteroides as substrates to produce fucose, lactose, and SCFAs (115).
In turn, some intestinal symbiotic bacteria can affect the glucose metabolism and glycosylation status in the host intestinal tract. A germ-free mouse showed defective epithelial α (1, 2)-fucosylation which recovered after commensal bacteria cultivation, implicating that the initiation of epithelial fucosylation partially relies on commensal bacteria (104, 116, 117) (Figure 2B). The frequency of fucosylated epithelial cells (F-Ecs) in different locations along the length of the small intestine was found to be positively correlated with the population of microorganisms, and the dependence of fucosylation on bacteria was further verified in both antibiotic-treated mice and GF mice such that a dramatic reduction in F-Ecs frequency and Fut2 expression was exhibited, which can be restored with antibiotic cessation or conventionalized cultivation (118). Additionally, researchers also found that commensal bacteria selectively induce the fucosylation of columnar epithelial cells and goblet cells instead of Paneth cells (118). Commensal bacteria may have unequilibrim potency in inducing fucosylation, such as Bacteroides thetaiotaomicron (B. thetaiotaomicron), Bacteroides fragilis (B. fragilis), Citrobacter rodentium, and segmented filamentous bacteria, while Lactobacillus murinus, Bifidobacterium, and Peptostreptococcus cannot initiate fucosylation (104, 117, 119, 120). However, once Bacteroides are deficient in fucose metabolic pathway, they lose the function of inducing epithelial fucosylation (104). Apart from commensal bacteria, some pathogenetic bacteria including S. typhimurium and Helicobacter bilis have also been reported to induce epithelial Fut2 expression and fucosylation (104, 121).
On the flip side, B. thetaiotaomicron and B. fragilis can also express fucosidase and cut off the terminal and subterminal fucosylation on epithelial cells for self-utilization. This may help to explain why B. fragilis has advantages in colonization compared with mutation strains with defects in fucosyl-utilizing enzymes (104, 122).
The human intestinal microbiota is made up of five dominant bacterial phyla: Firmicutes, Bacteroidetes, Actinobacteria, Proteobacteria, and Verrucomicrobia (111). Changes of the intestinal ecosystem have been reported in multiple autoimmune diseases, and the shifted gut microbiota architecture is suggested to be involved in skewing host immune responses through various mechanisms, which have been summarized elsewhere previously (26, 123). Table 2 shows a summary of the changes in gut micobiota composition in patients with different autoimmune diseases. Specifically, reduced microbial diversity and decreased Firmicutes/Bacteroides ratio were found in T1D patients (143), and the gut microbiota composition and the associated metabolites in adult patients with latent autoimmune diabetes (LADA) closely correlated with circulatory autoantibodies, glucose metabolism, islet function, and inflammatory factors (144). PSA from B. fragilis can reduce IL-17 expression and upregulate IL-10-producing inducible Tregs. B. fragilis colonization was proved to be negatively associated with LPS-induced inflammatory cytokines and chemokines, thus suppressing inflammatory risks (145, 146). An intraperitoneal injection of the bacterial cell wall from certain bacterial species including Bifidobacterium, Streptococcus, Lactobacillus, etc., induced arthritis in susceptible strains of rats, and researchers found that the peptidoglycan component in the bacteria determined their proinflammatory property (147).
Some pathogenetic bacteria in the intestine might stimulate host autoimmune responses via molecular mimicry, which may also associate with the glucosyl in bacterial peptide as glucosyl structure and density affect the configuration and even the antigenicity of glycoconjugates. GM1- and GD1a-like LPS on C. jejuni surface might be responsible for the generation of anti-GM1b antibodies in Guillain–Barré syndrome patients (148). Streptococcus agalactiae can produce sialic acid-capped structures mimicking host glycosylation patterns and bind with sialic acid recognizing Ig-superfamily lectins on DCs, macrophages, and NK cells (51, 149, 150). Group B Streptococci can present sialylated glycans on the cell wall as well and prevent attacks from the complement system (150). Studies also proved that the peptides homologous with human RNA binding autoantigen Ro60 and β2-glycoprotein I from B. thetaiotaomicron and Roseburia intestinalis could provoke autoimmunity and lupus-like manifestations in GF mice models (26, 151).
Goto et al. (118) pointed out that, besides the microbiota–epithelia crosstalk, the stimulation of CD90+RORγt+ILC3 cells is also essential in the initiation of EC fucosylation. By producing IL-22 and regulating epithelial Fut2 expression, CD90+ILC3 induces epithelial fucosylation and creates a protective barrier against infection in a commensal-bacteria-dependent and commensal-bacteria-independent manner, respectively (118). Fut2 or α (1, 2)-fucosedeficient mice were found to have an increased risk of Salmonella typhimurium infection and vaginal Candida albicans (104, 152).
In addition, the mucin barrier of the gastrointestinal epithelium might be disrupted under a certain pathological state like IBD, which brings the collapse of its barrier function and promotes bacterial adherence (35, 36). The disruption was found to be related with both host genetic susceptibility and environmental etiologies (37, 38).
There is a broad spectrum of autoimmune diseases but with common features of chronic excessive inflammation, necessitating extra energy and biomaterial supply. Typically, upregulation of GLU1 and increased glucose uptake have been recognized in RA, SLE, and psoriasis. The interference of glycolysis with GLU1 knockout or inhibitor displayed a therapeutic potential and improved the autoimmune phenotype in animal models (153).
In RA patients, CD4+ T cells transfer glucose to the pentose phosphate pathway to meet its energy and biosynthesis demand for rapid proliferation (154). In hypoxic synovial cavity, a metabolic shift to glycolysis instead of oxygen-consuming mitochondrial OXPHOS is required for synovium hyperplasia and invasive activity (153). Studies have also found that infiltrating lymphocytes in the synovium of RA mice model expressed increased HK2, the rate-limiting enzyme in glycolysis (155). Using an HK inhibitor, 2-deoxidation-D-glucose (2-DG), can obviously reduce the proportion of Tfh and Th17 cells (156). The galectin-1 expression of RA patients was found to be correlated with the regulation of cell apoptosis, and a galectin-1 injection can help relieve the clinical and histopathological manifestations (157). Another study also revealed the increased galectin-3 levels in the sera and synovial fluids of RA patients (72). From the fucosylation perspective, the expression of Futs catalyzing terminal and subterminal fucosylation was found to be significantly increased in synovial tissues, predominantly in M1 macrophages, which indicated that terminal fucosylation might be a novel hallmark of inflammatory macrophages (158). The antibody glycosylation condition was also altered in RA patients. Parekh et al. (159) found a lower rate of IgG containing galactose residues in RA and OA patients compared with healthy controls.
A similar aberrant glycosylation phenomenon was found in SLE, the prototype of autoimmune disease. Vučković et al. found decreased galactosylation and sialylation of IgG in SLE patients, along with a decreased core fucosylation level and an increased GlcNAc level (160). These alternations lead to a decreased immunosuppressive activity of immunoglobulins and are associated with disease severity (160).
Patients with SLE are prone to present insulin resistance, abnormal glucose tolerance, and diabetes (161), which might be attributed to the metabolic abnormalized effect of inflammatory factors and the functional changes of adipose tissue in SLE patients (162, 163). CD4+T cells in lupus patients and lupus-susceptible mice depend on enhanced glycolysis for rapid ATP production to meet their glucose requirements (154). The leukocyte recruitment pathway is mediated by selectin, the level of which is higher in SLE patients’ plasma, indicating that it may contribute to endothelial cell activation and the proinflammatory status in SLE patients. Higher levels of PSGL-1 with enhanced fucosylation on Treg cells in SLE downregulate the TGFβ pathway and inhibit the suppressive activity of Treg cells, which may facilitate self-tolerance breakdown (164).
MS patients, perceived to result from the autoimmune effect of T cell in damaging the myelin sheath, were found to have an elevated level of blood pyruvate in both fasting and postprandial times (165). By treating primary cultures of oligodendrocyte progenitor cells from rat cerebrum with MS-diseased cerebrospinal fluid, Mathur et al. revealed a decreased gene expression related with carbohydrate metabolism, glucose metabolism impairment, and reduced ATP availability for cellular damage repair (166, 167).
Several potential mechanisms have been raised to explain how the interaction between glucose metabolism and intestinal microbiota affects autoimmune diseases. According to the preceding discussion, prior to microbiota colonization, aberrant glucose metabolism and glucosylation condition might occur in patients with autoimmune diseases. On the one hand, this abnormality can cause potential damage to the intestinal mucosa, thus influencing the interaction between gut microbiota and host. On the other hand, aberrant glucose metabolism and glucosylation would also interfere with the function of the host immune system. Genetic factors that participate in the expression of glucose metabolism-related enzymes, like Fut, or intrinsic carbohydrate metabolism disturbance, like mucin disruption in IBD, would lead to the defective formation of the gut protective barrier, which, in turn, alters the gut ecosystem through influences on adhesion, virulence, and metabolism. An abnormal glycosylation level of antibodies either downregulates or upregulates immune responses to certain types of microbes and might damage organs and lead to disease development. Fut2 might be an important target gene in IBD. By constructing Fut2△IEC mice (Fut2 knockout mice), Tang et al. found that the intestinal epithelium-specific Fut2 deficiency increases the susceptibility to IBD by regulating the intestinal microbiota and the production of lysolecithin (168). After certain types of microbiota have interacted with the host–microbe interface and colonized, the PAMPs like PSA would be recognized by PRRs and mediate the immune responses. The process of glycosylation might be initiated, thus affecting the intestinal mucosa condition. The microbial metabolism in the gut might produce metabolites such as SCFAs, influencing the immune environment and either improving or exacerbating the disease manifestations (169).
A study revealed that the rapid fucosylation of intestinal epithelium sustained host–commensal symbiosis in sickness. Activation of the TLR signaling pathway leads to the rapid α (1, 2)-fucosylation of mouse IEC. The fucosylated proteins enter the intestinal lumen, and the fucose is then utilized by the intestinal microbiota, which affects bacterial metabolic pathways and decreases the expression of virulence genes (120).
Up to now, there are several studies about the treatment of autoimmune diseases targeting different underlying mechanisms, including glucose metabolism, glycosylation, microbiota, etc. They might have already shown potential therapeutic effects or indicated future research interests (Table 3).
Table 3 Potential glucose metabolism, glycosylation, and microbiota targeting therapeutic strategies.
As previously mentioned, the interaction between bacteria (C. jejuni) and fucosylation prompts a bacterial infection, and 2′-FL inhibits this adhesion. In a mouse infection model, feeding 2′-FL orally has been proven to lead to a strong protection of the intestinal mucosa against infections (113). This result might indicate the possibility of a novel kind of 2′-FL-derived drug which can be used orally and might be effective in IBD patients.
Studies have revealed the positive therapeutic effects of small molecules of fucosylation inhibitors. Fucosylation inhibitors can be categorized as competitive inhibitors and metabolic inhibitors; the former blocks the interaction between fucosyl and selectin, while the latter reduces the process of fucosylation (6). Focustatin II, designed as an analog of fucose-1-phosphonate, competitively reduces the fucosylation of therapeutic monoclonal antibodies and improves the efficacy without combination with antibodies (183). The treatment of 2-fluorofocus on a sickle cell disease mice model showed a significant decrease in the adhesion of leukocytes, neutrophils, and sickle red blood cells with the endothelium (170). Other competitive inhibitors include hyperacetylated 6-alkynyl-fucose, hyperacetylated 5-thiofucose (5T-Fuc), etc. 5T-Fuc can also play an important role in metabolic inhibition (6).
Glycoregulation therapy with 2-DG and metformin changes the T cell surface glycosylation patterns and enables immune tolerance induction as evidenced by the reduced anti-dsDNA antibody titers and the decreased renal deposition of immune complex as well as the restored tolerance to allografts in lupus-prone mice, probably via targeting glycolysis and oxidative phosphorylation (184).
Inspired by the mechanism that glycan modification of IgG Fc domains modulates divergent functions, scientists have come up with the idea of glycoengineering therapy. Pagan et al. increased the sialylation level of IgG in vivo in mice models through the administration of engineered glycosyltransferases and found that it successfully exerted an anti-inflammatory effect and was effective at relieving arthritis (171, 172). Furthermore, activated platelets were found to be significant ingredient donors of in vivo sialylation, and coordinately giving clopidogrel or other platelet inhibitor drugs would inhibit the anti-inflammatory effect of this glycoengineering therapy though the platelets themselves have pro-inflammatory effects (171, 185, 186). Glycoengineering has also attracted interest in the field of tumor as the mechanism of immune evasion of tumor cells also involves the sialylation of related proteins (150).
Probiotics are a group of bacteria that can be beneficial for human immune function, nutritional condition, intestinal microbiota constitution, etc. Prebiotics are indigestible food ingredients that improve the gut microbiota structure by providing carbon sources, making metabolic substrates available for the gut microbiota to utilize, and adjusting the gut microenvironment, such as in terms of pH value, gastrointestinal absorption, and enzyme hydrolysis (173, 174). Fructo-oligosaccharide (FOS) is a well-studied effective prebiotic consisting of one glucose and one fructose, and it has shown multiple beneficial effects in several animal models including the following: (a) resisting bacterial infection such as that of enterotoxigenic E. coli (176), (b) promoting the colonization of probiotics like Bifidobacterium and Lactobacillus (177–180), (c) increasing the production of SCFAs by the use of these probiotics (180), and (d) downregulating the pro-inflammatory cytokine expression and protecting the mucosal barrier (181). Besides FOS, inulin, galacto-oligosaccharides, and some foods containing indigestible β-glucans such as termites, a kind of Chinese traditional medicine, also have a similar effect (174).
As has been proved that chemically synthesized oligosaccharides with core fucosylation have the function of promoting Bifidobacterium and Lactobacillus growth, it is put forward that the core fucosylation in maternal milk is a promising prebiotic for infants (102). Gestational diabetes mellitus during maternity results in higher fucosylation and sialylation levels in milk that affects the immune function of infants, which indicates the importance of guidance in beneficial nutrition and healthcare of gestating women (187).
Furthermore, diet regulation has been raised as a helpful and safe intervention to guide the establishment of a healthy gut microbiome and restore beneficial metabolism. Resistant starch or rich-fiber diet has shown many benefits in lessening the inflammation status and improving metabolic aberrance by retarding glucose absorption, strengthening the gut barrier, flourishing beneficial commensals, and reducing cytokine release. Kearney et al. (188) reported an increase of relative abundance of Bacteroides plebeius by more than two orders of magnitude in mice fed with a diet containing 1% raw seaweed. Dietary resistant starch is proven to be beneficial to lupus-prone hosts by suppressing the abundance and translocation of Lactobacillus reuteri which drives autoimmunity (182). Studies also report that the Mediterranean-style diet, characterized by rich unsaturated fatty acids and fibers, has anti-inflammatory effects and produces a beneficial effect to relieve the symptoms of MS patients (189, 190).
Though carbohydrates play a vital role in physiological and pathological events, they can be easily eliminated by the digestive and urinary systems; therefore, the development of drugs directly derived from carbohydrates was limited. Some carbohydrate-derived drugs, like sodium hyaluronate (decreasing the risks of infection and pain in arthroscopic debridement) (191) and sulfated glycosaminoglycans (binding to antithrombin and promoting anticoagulation in thrombosis) (40, 192), have been indicated as beneficial for osteoarthritis. Researchers also attempted to deliver carbohydrates orally or by injection and observe their pharmacokinetics and effects. Pozharitskaya et al. intragastrically administrated fucoidan to rats and found that it accumulated firstly in the kidney, spleen, and liver and had a relatively long elimination time from circulation, thus revealing the possibility of fucoidan-derived drugs (193). Wu et al. (194) treated induced obese mice with fucose by intragastric administration and found decreased body weight gain, fat accumulation, and hepatic triglyceride elevation in mice.
In addition, several novel oral heptadecaglycoside antibiotics have been studied and developed. Saccharomicins A and B, isolated from Saccharothrix espanaensis, showed inhibition effects to pathogenetic bacteria growth both in vivo and in vitro (195). The microscopic observations showed that the treatment of these drugs could inhibit the synthesis of DNA, RNA, and proteins in bacteria (195, 196).
In conclusion, the mutual influence of glucose metabolism, glycosylation, and intestinal microbes is complicated, and their contributions to autoimmune diseases are largely unclarified. A well-defined integrated network can improve our substantial understanding about the dynamic change of immunity and metabolism under the era of microbiota. From the perspective of the synergistic effect of glucose metabolism, glycosylation, and intestinal microbiota, new inspirations and intervention strategy may be developed for autoimmune diseases.
All authors were involved in drafting this paper or revising it critically for key intellectual content. JZ and LZ had full access to all the data in the article. Conception: LZ and JZ; methodology: LZ, HX, and LW; interpretation and writing: LW, HX, HY, and LZ; and supervision: LZ and JZ. All authors contributed to the article and approved the submitted version.
This study was supported by the National Natural Science Foundation of China (82071840) and CAMS Innovation Fund for Medical Sciences (CIFMS) (2020-I2M-C&T-B-013).
The authors declare that the research was conducted in the absence of any commercial or financial relationships that could be construed as a potential conflict of interest.
All claims expressed in this article are solely those of the authors and do not necessarily represent those of their affiliated organizations, or those of the publisher, the editors and the reviewers. Any product that may be evaluated in this article, or claim that may be made by its manufacturer, is not guaranteed or endorsed by the publisher.
1. Ahn SS, Hwang SH, Jung SM, Lee SW, Park YB, Yun M, et al. Evaluation of spleen glucose metabolism using (18)F-fdg Pet/Ct in patients with febrile autoimmune disease. J Nucl Med (2017) 58(3):507–13. doi: 10.2967/jnumed.116.180729
2. Martinez N, Vallerskog T, West K, Nunes-Alves C, Lee J, Martens GW, et al. Chromatin decondensation and T cell hyperresponsiveness in diabetes-associated hyperglycemia. J Immunol (Baltimore Md: 1950) (2014) 193(9):4457–68. doi: 10.4049/jimmunol.1401125
3. Lee AH, Dixit VD. Dietary regulation of immunity. Immunity (2020) 53(3):510–23. doi: 10.1016/j.immuni.2020.08.013
4. Palmer CS, Ostrowski M, Balderson B, Christian N, Crowe SM. Glucose metabolism regulates T cell activation, differentiation, and functions. Front Immunol (2015) 6:1. doi: 10.3389/fimmu.2015.00001
5. Brownlee M, Vlassara H, Cerami A. Nonenzymatic glycosylation and the pathogenesis of diabetic complications. Ann Intern Med (1984) 101(4):527–37. doi: 10.7326/0003-4819-101-4-527
6. Li J, Hsu HC, Mountz JD, Allen JG. Unmasking fucosylation: From cell adhesion to immune system regulation and diseases. Cell Chem Biol (2018) 25(5):499–512. doi: 10.1016/j.chembiol.2018.02.005
7. Liang W, Mao S, Sun S, Li M, Li Z, Yu R, et al. Core fucosylation of the T cell receptor is required for T cell activation. Front Immunol (2018) 9:78. doi: 10.3389/fimmu.2018.00078
8. Sommer F, Adam N, Johansson ME, Xia L, Hansson GC, Bäckhed F. Altered mucus glycosylation in core 1 O-Glycan-Deficient mice affects microbiota composition and intestinal architecture. PloS One (2014) 9(1):e85254. doi: 10.1371/journal.pone.0085254
9. Kashyap PC, Marcobal A, Ursell LK, Smits SA, Sonnenburg ED, Costello EK, et al. Genetically dictated change in host mucus carbohydrate landscape exerts a diet-dependent effect on the gut microbiota. Proc Natl Acad Sci USA. (2013) 110(42):17059–64. doi: 10.1073/pnas.1306070110
10. Kuehn MJ, Heuser J, Normark S, Hultgren SJ. P pili in uropathogenic e. coli are composite fibres with distinct fibrillar adhesive tips. Nature (1992) 356(6366):252–5. doi: 10.1038/356252a0
11. Sung MA, Fleming K, Chen HA, Matthews S. The solution structure of papgii from uropathogenic escherichia coli and its recognition of glycolipid receptors. EMBO Rep (2001) 2(7):621–7. doi: 10.1093/embo-reports/kve133
12. Hase K, Kawano K, Nochi T, Pontes GS, Fukuda S, Ebisawa M, et al. Uptake through glycoprotein 2 of fimh(+) bacteria by m cells initiates mucosal immune response. Nature (2009) 462(7270):226–30. doi: 10.1038/nature08529
13. Blondel CJ, Park JS, Hubbard TP, Pacheco AR, Kuehl CJ, Walsh MJ, et al. Crispr/Cas9 screens reveal requirements for host cell sulfation and fucosylation in bacterial type iii secretion system-mediated cytotoxicity. Cell Host Microbe (2016) 20(2):226–37. doi: 10.1016/j.chom.2016.06.010
14. Pacheco AR, Curtis MM, Ritchie JM, Munera D, Waldor MK, Moreira CG, et al. Fucose sensing regulates bacterial intestinal colonization. Nature (2012) 492(7427):113–7. doi: 10.1038/nature11623
15. Cao M, Peng Y, Lu Y, Zou Z, Chen J, Bottino R, et al. Controls of hyperglycemia improves dysregulated microbiota in diabetic mice. Transplantation (2021) 105(9):1980–8. doi: 10.1097/tp.0000000000003603
16. Simon MC, Reinbeck AL, Wessel C, Heindirk J, Jelenik T, Kaul K, et al. Distinct alterations of gut morphology and microbiota characterize accelerated diabetes onset in nonobese diabetic mice. J Biol Chem (2020) 295(4):969–80. doi: 10.1074/jbc.RA119.010816
17. Meydani SN, Das SK, Pieper CF, Lewis MR, Klein S, Dixit VD, et al. Long-term moderate calorie restriction inhibits inflammation without impairing cell-mediated immunity: A randomized controlled trial in non-obese humans. Aging (Albany NY) (2016) 8(7):1416–31. doi: 10.18632/aging.100994
18. Jordan S, Tung N, Casanova-Acebes M, Chang C, Cantoni C, Zhang D, et al. Dietary intake regulates the circulating inflammatory monocyte pool. Cell (2019) 178(5):1102–14.e17. doi: 10.1016/j.cell.2019.07.050
19. Ho TP, Zhao X, Courville AB, Linderman JD, Smith S, Sebring N, et al. Effects of a 12-month moderate weight loss intervention on insulin sensitivity and inflammation status in nondiabetic overweight and obese subjects. Horm Metab Res (2015) 47(4):289–96. doi: 10.1055/s-0034-1382011
20. De Rosa V, Galgani M, Santopaolo M, Colamatteo A, Laccetti R, Matarese G. Nutritional control of immunity: Balancing the metabolic requirements with an appropriate immune function. Semin Immunol (2015) 27(5):300–9. doi: 10.1016/j.smim.2015.10.001
21. Collins N, Han SJ, Enamorado M, Link VM, Huang B, Moseman EA, et al. The bone marrow protects and optimizes immunological memory during dietary restriction. Cell (2019) 178(5):1088–101.e15. doi: 10.1016/j.cell.2019.07.049
22. Kawai T, Akira S. Tlr signaling. Semin Immunol (2007) 19(1):24–32. doi: 10.1016/j.smim.2006.12.004
23. Fujita T, Matsushita M, Endo Y. The lectin-complement pathway–its role in innate immunity and evolution. Immunol Rev (2004) 198:185–202. doi: 10.1111/j.0105-2896.2004.0123.x
24. Chen BD, Jia XM, Xu JY, Zhao LD, Ji JY, Wu BX, et al. An autoimmunogenic and proinflammatory profile defined by the gut microbiota of patients with untreated systemic lupus erythematosus. Arthritis Rheumatol (2021) 73(2):232–43. doi: 10.1002/art.41511
25. Manfredo Vieira S, Hiltensperger M, Kumar V, Zegarra-Ruiz D, Dehner C, Khan N, et al. Translocation of a gut pathobiont drives autoimmunity in mice and humans. Sci (New York NY) (2018) 359(6380):1156–61. doi: 10.1126/science.aar7201
26. Zhang X, Chen BD, Zhao LD, Li H. The gut microbiota: Emerging evidence in autoimmune diseases. Trends Mol Med (2020) 26(9):862–73. doi: 10.1016/j.molmed.2020.04.001
27. Zhang X, Zhang D, Jia H, Feng Q, Wang D, Liang D, et al. The oral and gut microbiomes are perturbed in rheumatoid arthritis and partly normalized after treatment. Nat Med (2015) 21(8):895–905. doi: 10.1038/nm.3914
28. Knip M, Siljander H. The role of the intestinal microbiota in type 1 diabetes mellitus. Nat Rev Endocrinol (2016) 12(3):154–67. doi: 10.1038/nrendo.2015.218
29. Calvo-Barreiro L, Eixarch H, Montalban X, Espejo C. Combined therapies to treat complex diseases: The role of the gut microbiota in multiple sclerosis. Autoimmun Rev (2018) 17(2):165–74. doi: 10.1016/j.autrev.2017.11.019
30. Macchione IG, Lopetuso LR, Ianiro G, Napoli M, Gibiino G, Rizzatti G, et al. Akkermansia muciniphila: Key player in metabolic and gastrointestinal disorders. Eur Rev Med Pharmacol Sci (2019) 23(18):8075–83. doi: 10.26355/eurrev_201909_19024
31. Hänninen A, Toivonen R, Pöysti S, Belzer C, Plovier H, Ouwerkerk JP, et al. Akkermansia muciniphila induces gut microbiota remodelling and controls islet autoimmunity in nod mice. Gut (2018) 67(8):1445–53. doi: 10.1136/gutjnl-2017-314508
32. Rodrigues VF, Elias-Oliveira J, Pereira ÍS, Pereira JA, Barbosa SC, Machado MSG, et al. Akkermansia muciniphila and gut immune system: A good friendship that attenuates inflammatory bowel disease, obesity, and diabetes. Front Immunol (2022) 13:934695. doi: 10.3389/fimmu.2022.934695
33. Rose NR. Prediction and prevention of autoimmune disease in the 21st century: A review and preview. Am J Epidemiol (2016) 183(5):403–6. doi: 10.1093/aje/kwv292
34. Cao WQ, Liu MQ, Kong SY, Wu MX, Huang ZZ, Yang PY. Novel methods in glycomics: A 2019 update. Expert Rev Proteomics (2020) 17(1):11–25. doi: 10.1080/14789450.2020.1708199
35. Kellman BP, Lewis NE. Big-data glycomics: Tools to connect glycan biosynthesis to extracellular communication. Trends Biochem Sci (2021) 46(4):284–300. doi: 10.1016/j.tibs.2020.10.004
36. Kempkes RWM, Joosten I, Koenen H, He X. Metabolic pathways involved in regulatory T cell functionality. Front Immunol (2019) 10:2839. doi: 10.3389/fimmu.2019.02839
37. Patra KC, Wang Q, Bhaskar PT, Miller L, Wang Z, Wheaton W, et al. Hexokinase 2 is required for tumor initiation and maintenance and its systemic deletion is therapeutic in mouse models of cancer. Cancer Cell (2013) 24(2):213–28. doi: 10.1016/j.ccr.2013.06.014
38. Mithieux G, Gautier-Stein A. Intestinal glucose metabolism revisited. Diabetes Res Clin Pract (2014) 105(3):295–301. doi: 10.1016/j.diabres.2014.04.008
39. Chandel NS. Carbohydrate metabolism. Cold Spring Harbor Perspect Biol (2021) 13(1):a037788. doi: 10.1101/cshperspect.a040568
40. Ernst B, Magnani JL. From carbohydrate leads to glycomimetic drugs. Nat Rev Drug Discovery (2009) 8(8):661–77. doi: 10.1038/nrd2852
41. Uchimido R, Schmidt EP, Shapiro NI. The glycocalyx: A novel diagnostic and therapeutic target in sepsis. Crit Care (London England) (2019) 23(1):16. doi: 10.1186/s13054-018-2292-6
42. Tarbell JM, Cancel LM. The glycocalyx and its significance in human medicine. J Internal Med (2016) 280(1):97–113. doi: 10.1111/joim.12465
43. Alphonsus CS, Rodseth RN. The endothelial glycocalyx: A review of the vascular barrier. Anaesthesia (2014) 69(7):777–84. doi: 10.1111/anae.12661
44. McGuckin MA, Lindén SK, Sutton P, Florin TH. Mucin dynamics and enteric pathogens. Nat Rev Microbiol (2011) 9(4):265–78. doi: 10.1038/nrmicro2538
45. Hansson GC. Mucins and the microbiome. Annu Rev Biochem (2020) 89:769–93. doi: 10.1146/annurev-biochem-011520-105053
46. Patel G, Misra A. 10 - oral delivery of proteins and peptides: Concepts and applications. In: Misra A, editor. Challenges in delivery of therapeutic genomics and proteomics, vol. . p . London: Elsevier (2011). p. 481–529.
47. Baum LG, Cobb BA. The direct and indirect effects of glycans on immune function. Glycobiology (2017) 27(7):619–24. doi: 10.1093/glycob/cwx036
48. Kudelka MR, Stowell SR, Cummings RD, Neish AS. Intestinal epithelial glycosylation in homeostasis and gut microbiota interactions in ibd. Nat Rev Gastroenterol Hepatol (2020) 17(10):597–617. doi: 10.1038/s41575-020-0331-7
49. Wu Y, Murray GK, Byrne EM, Sidorenko J, Visscher PM, Wray NR. Gwas of peptic ulcer disease implicates helicobacter pylori infection, other gastrointestinal disorders and depression. Nat Commun (2021) 12(1):1146. doi: 10.1038/s41467-021-21280-7
50. Boltin D, Perets TT, Vilkin A, Niv Y. Mucin function in inflammatory bowel disease: An update. J Clin Gastroenterol (2013) 47(2):106–11. doi: 10.1097/MCG.0b013e3182688e73
51. Crocker PR, Paulson JC, Varki A. Siglecs and their roles in the immune system. Nat Rev Immunol (2007) 7(4):255–66. doi: 10.1038/nri2056
52. Gabius HJ, André S, Jiménez-Barbero J, Romero A, Solís D. From lectin structure to functional glycomics: Principles of the sugar code. Trends Biochem Sci (2011) 36(6):298–313. doi: 10.1016/j.tibs.2011.01.005
53. De Bousser E, Meuris L, Callewaert N, Festjens N. Human T cell glycosylation and implications on immune therapy for cancer. Hum Vaccines immunother (2020) 16(10):2374–88. doi: 10.1080/21645515.2020.1730658
54. Moremen KW, Tiemeyer M, Nairn AV. Vertebrate protein glycosylation: Diversity, synthesis and function. Nat Rev Mol Cell Biol (2012) 13(7):448–62. doi: 10.1038/nrm3383
55. Okada M, Chikuma S, Kondo T, Hibino S, Machiyama H, Yokosuka T, et al. Blockage of core fucosylation reduces cell-surface expression of pd-1 and promotes anti-tumor immune responses of T cells. Cell Rep (2017) 20(5):1017–28. doi: 10.1016/j.celrep.2017.07.027
56. Macintyre AN, Gerriets VA, Nichols AG, Michalek RD, Rudolph MC, Deoliveira D, et al. The glucose transporter Glut1 is selectively essential for Cd4 T cell activation and effector function. Cell Metab (2014) 20(1):61–72. doi: 10.1016/j.cmet.2014.05.004
57. Angelin A, Gil-de-Gómez L, Dahiya S, Jiao J, Guo L, Levine MH, et al. Foxp3 reprograms T cell metabolism to function in low-glucose, high-lactate environments. Cell Metab (2017) 25(6):1282–93.e7. doi: 10.1016/j.cmet.2016.12.018
58. Chang CH, Curtis JD, Maggi LB Jr., Faubert B, Villarino AV, O’Sullivan D, et al. Posttranscriptional control of T cell effector function by aerobic glycolysis. Cell (2013) 153(6):1239–51. doi: 10.1016/j.cell.2013.05.016
59. Chang CH, Qiu J, O’Sullivan D, Buck MD, Noguchi T, Curtis JD, et al. Metabolic competition in the tumor microenvironment is a driver of cancer progression. Cell (2015) 162(6):1229–41. doi: 10.1016/j.cell.2015.08.016
60. Yang H, Youm YH, Dixit VD. Inhibition of thymic adipogenesis by caloric restriction is coupled with reduction in age-related thymic involution. J Immunol (Baltimore Md: 1950) (2009) 183(5):3040–52. doi: 10.4049/jimmunol.0900562
61. Hardie DG, Ross FA, Hawley SA. Ampk: A nutrient and energy sensor that maintains energy homeostasis. Nat Rev Mol Cell Biol (2012) 13(4):251–62. doi: 10.1038/nrm3311
62. Matsuura E, Tan XW, Shen LH, Azmi NU, Lopez LR. Chapter 6 - inflammasomes and inflammatory cytokines in early atherosclerosis. In: Atzeni F, Doria A, Nurmohamed M, Pauletto P, editors. Handbook of systemic autoimmune diseases, vol. 14. Netherlands: Elsevier (2017). p. 155–67.
63. Lin TH, Su HH, Kang HY, Chang TH. The interactive roles of lipopolysaccharides and Dsrna/Viruses on respiratory epithelial cells and dendritic cells in allergic respiratory disorders: The hygiene hypothesis. Int J Mol Sci (2017) 18(10):2219. doi: 10.3390/ijms18102219
64. Kapellos GE, Alexiou TS. Chapter 1 - modeling momentum and mass transport in cellular biological media: From the molecular to the tissue scale. In: Becker SM, Kuznetsov AV, editors. Transport in biological media. Boston: Elsevier (2013). p. 1–40.
65. Zindel J, Kubes P. Damps, pamps, and lamps in immunity and sterile inflammation. Annu Rev Pathol (2020) 15:493–518. doi: 10.1146/annurev-pathmechdis-012419-032847
66. Gong T, Liu L, Jiang W, Zhou R. Damp-sensing receptors in sterile inflammation and inflammatory diseases. Nat Rev Immunol (2020) 20(2):95–112. doi: 10.1038/s41577-019-0215-7
67. Jacquelot N, Duong CPM, Belz GT, Zitvogel L. Targeting chemokines and chemokine receptors in melanoma and other cancers. Front Immunol (2018) 9:2480. doi: 10.3389/fimmu.2018.02480
68. Medzhitov R. Origin and physiological roles of inflammation. Nature (2008) 454(7203):428–35. doi: 10.1038/nature07201
69. Kubes P, Mehal WZ. Sterile inflammation in the liver. Gastroenterology (2012) 143(5):1158–72. doi: 10.1053/j.gastro.2012.09.008
70. Chen GY, Nuñez G. Sterile inflammation: Sensing and reacting to damage. Nat Rev Immunol (2010) 10(12):826–37. doi: 10.1038/nri2873
71. Li D, Wu M. Pattern recognition receptors in health and diseases. Signal Transduct Target Ther (2021) 6(1):291. doi: 10.1038/s41392-021-00687-0
72. Bianco GA, Toscano MA, Ilarregui JM, Rabinovich GA. Impact of protein-glycan interactions in the regulation of autoimmunity and chronic inflammation. Autoimmun Rev (2006) 5(5):349–56. doi: 10.1016/j.autrev.2006.02.003
73. Sharon N, Lis H. Lectins. In: Lennarz WJ, Lane MD, editors. Encyclopedia of biological chemistry (Second edition). Waltham: Academic Press (2013). p. 701–5.
74. Araten DJ, Mandle RJ, Isenman DE, Carroll MC. Chapter 24 - complement and immunoglobulin biology leading to clinical translation. In: Hoffman R, Benz EJ, Silberstein LE, Heslop HE, Weitz JI, Anastasi J, et al, editors. Hematology (Seventh edition). (Netherlands: Elsevier) (2018). p. 261–84.e6.
75. Matsushita M, Fujita T. Ficolins and the lectin complement pathway. Immunol Rev (2001) 180:78–85. doi: 10.1034/j.1600-065x.2001.1800107.x
76. Endo Y, Matsushita M, Fujita T. New insights into the role of ficolins in the lectin pathway of innate immunity. Int Rev Cell Mol Biol (2015) 316:49–110. doi: 10.1016/bs.ircmb.2015.01.003
77. Endo Y, Matsushita M, Fujita T. The role of ficolins in the lectin pathway of innate immunity. Int J Biochem Cell Biol (2011) 43(5):705–12. doi: 10.1016/j.biocel.2011.02.003
78. Thiemann S, Baum LG. Galectins and immune responses-just how do they do those things they do? Annu Rev Immunol (2016) 34:243–64. doi: 10.1146/annurev-immunol-041015-055402
79. Buttari B, Profumo E, Capozzi A, Facchiano F, Saso L, Sorice M, et al. Advanced glycation end products of human β2 glycoprotein I modulate the maturation and function of dcs. Blood (2011) 117(23):6152–61. doi: 10.1182/blood-2010-12-325514
80. Amini P, Stojkov D, Felser A, Jackson CB, Courage C, Schaller A, et al. Neutrophil extracellular trap formation requires Opa1-dependent glycolytic atp production. Nat Commun (2018) 9(1):2958. doi: 10.1038/s41467-018-05387-y
81. Duan J, Avci FY, Kasper DL. Microbial carbohydrate depolymerization by antigen-presenting cells: Deamination prior to presentation by the mhcii pathway. Proc Natl Acad Sci USA (2008) 105(13):5183–8. doi: 10.1073/pnas.0800974105
82. Avci FY, Li X, Tsuji M, Kasper DL. Carbohydrates and T cells: A sweet twosome. Semin Immunol (2013) 25(2):146–51. doi: 10.1016/j.smim.2013.05.005
83. Johnson JL, Jones MB, Cobb BA. Polysaccharide a from the capsule of bacteroides fragilis induces clonal Cd4+ T cell expansion. J Biol Chem (2015) 290(8):5007–14. doi: 10.1074/jbc.M114.621771
84. Ryan SO, Bonomo JA, Zhao F, Cobb BA. Mhcii glycosylation modulates bacteroides fragilis carbohydrate antigen presentation. J Exp Med (2011) 208(5):1041–53. doi: 10.1084/jem.20100508
85. Alvarez CA, Jones MB, Hambor J, Cobb BA. Characterization of polysaccharide a response reveals interferon responsive gene signature and immunomodulatory marker expression. Front Immunol (2020) 11:556813. doi: 10.3389/fimmu.2020.556813
86. Zhou JY, Zhou D, Telfer K, Reynero K, Jones MB, Hambor J, et al. Antigen presenting cell response to polysaccharide a is characterized by the generation of anti-inflammatory macrophages. Glycobiology (2022) 32(2):136–47. doi: 10.1093/glycob/cwab111
87. Wang N. Cell adhesion molecules. In: Aminoff MJ, Daroff RB, editors. Encyclopedia of the neurological sciences (Second edition). Oxford: Academic Press (2014). p. 628–9.
88. Angiari S. Selectin-mediated leukocyte trafficking during the development of autoimmune disease. Autoimmun Rev (2015) 14(11):984–95. doi: 10.1016/j.autrev.2015.06.006
89. Silva M, Martin KC, Mondal N, Sackstein R. Slex expression delineates distinct functional subsets of human blood central and effector memory T cells. J Immunol (Baltimore Md: 1950) (2020) 205(7):1920–32. doi: 10.4049/jimmunol.1900679
90. Borsig L. Selectins in cancer immunity. Glycobiology (2018) 28(9):648–55. doi: 10.1093/glycob/cwx105
91. Shan M, Yang D, Dou H, Zhang L. Fucosylation in cancer biology and its clinical applications. Prog Mol Biol Trans Sci (2019) 162:93–119. doi: 10.1016/bs.pmbts.2019.01.002
92. Tu L, Murphy PG, Li X, Tedder TF. L-selectin ligands expressed by human leukocytes are heca-452 antibody-defined carbohydrate epitopes preferentially displayed by p-selectin glycoprotein ligand-1. J Immunol (Baltimore Md: 1950) (1999) 163(9):5070–8.
93. Vidarsson G, Dekkers G, Rispens T. Igg subclasses and allotypes: From structure to effector functions. Front Immunol (2014) 5:520. doi: 10.3389/fimmu.2014.00520
94. Jennewein MF, Alter G. The immunoregulatory roles of antibody glycosylation. Trends Immunol (2017) 38(5):358–72. doi: 10.1016/j.it.2017.02.004
95. Lu J, Chu J, Zou Z, Hamacher NB, Rixon MW, Sun PD. Structure of fcγri in complex with fc reveals the importance of glycan recognition for high-affinity igg binding. Proc Natl Acad Sci USA (2015) 112(3):833–8. doi: 10.1073/pnas.1418812112
96. Lux A, Yu X, Scanlan CN, Nimmerjahn F. Impact of immune complex size and glycosylation on igg binding to human fcγrs. J Immunol (Baltimore Md: 1950) (2013) 190(8):4315–23. doi: 10.4049/jimmunol.1200501
97. Ferrara C, Grau S, Jäger C, Sondermann P, Brünker P, Waldhauer I, et al. Unique carbohydrate-carbohydrate interactions are required for high affinity binding between fcgammariii and antibodies lacking core fucose. Proc Natl Acad Sci United States America (2011) 108(31):12669–74. doi: 10.1073/pnas.1108455108
98. Mizushima T, Yagi H, Takemoto E, Shibata-Koyama M, Isoda Y, Iida S, et al. Structural basis for improved efficacy of therapeutic antibodies on defucosylation of their fc glycans. Genes to cells: devoted to Mol Cell Mech (2011) 16(11):1071–80. doi: 10.1111/j.1365-2443.2011.01552.x
99. Imai-Nishiya H, Mori K, Inoue M, Wakitani M, Iida S, Shitara K, et al. Double knockdown of α1,6-fucosyltransferase (Fut8) and gdp-mannose 4,6-dehydratase (Gmd) in antibody-producing cells: A new strategy for generating fully non-fucosylated therapeutic antibodies with enhanced adcc. BMC Biotechnol (2007) 7(1):84. doi: 10.1186/1472-6750-7-84
100. Davies J, Jiang L, Pan LZ, LaBarre MJ, Anderson D, Reff M. Expression of gntiii in a recombinant anti-Cd20 cho production cell line: Expression of antibodies with altered glycoforms leads to an increase in adcc through higher affinity for fc gamma riii. Biotechnol bioeng (2001) 74(4):288–94.
101. Wang TT, Ravetch JV. Functional diversification of iggs through fc glycosylation. J Clin Invest (2019) 129(9):3492–8. doi: 10.1172/jci130029
102. Li M, Bai Y, Zhou J, Huang W, Yan J, Tao J, et al. Core fucosylation of maternal milk n-glycan evokes b cell activation by selectively promoting the l-fucose metabolism of gut bifidobacterium spp. and lactobacillus spp. mBio (2019) 10(2):e00128-19. doi: 10.1128/mBio.00128-19
103. Pham TA, Clare S, Goulding D, Arasteh JM, Stares MD, Browne HP, et al. Epithelial il-22ra1-Mediated fucosylation promotes intestinal colonization resistance to an opportunistic pathogen. Cell Host Microbe (2014) 16(4):504–16. doi: 10.1016/j.chom.2014.08.017
104. Goto Y, Uematsu S, Kiyono H. Epithelial glycosylation in gut homeostasis and inflammation. Nat Immunol (2016) 17(11):1244–51. doi: 10.1038/ni.3587
105. Tang MLK. 10 - the physiological induction of tolerance to allergens. In: Wahn U, Sampson HA, editors. Allergy, immunity and tolerance in early childhood. Amsterdam: Academic Press (2016). p. 153–70.
106. Johansson ME, Jakobsson HE, Holmén-Larsson J, Schütte A, Ermund A, Rodríguez-Piñeiro AM, et al. Normalization of host intestinal mucus layers requires long-term microbial colonization. Cell Host Microbe (2015) 18(5):582–92. doi: 10.1016/j.chom.2015.10.007
107. Solanki V, Tiwari M, Tiwari V. Host-bacteria interaction and adhesin study for development of therapeutics. Int J Biol macromol (2018) 112:54–64. doi: 10.1016/j.ijbiomac.2018.01.151
108. Aigal S, Claudinon J, Römer W. Plasma membrane reorganization: A glycolipid gateway for microbes. Biochim Biophys Acta (2015) 1853(4):858–71. doi: 10.1016/j.bbamcr.2014.11.014
109. Wang Z, Zhao Y. Gut microbiota derived metabolites in cardiovascular health and disease. Protein Cell (2018) 9(5):416–31. doi: 10.1007/s13238-018-0549-0
110. Koropatkin NM, Cameron EA, Martens EC. How glycan metabolism shapes the human gut microbiota. Nat Rev Microbiol (2012) 10(5):323–35. doi: 10.1038/nrmicro2746
111. Moffa S, Mezza T, Cefalo CMA, Cinti F, Impronta F, Sorice GP, et al. The interplay between immune system and microbiota in diabetes. Mediators Inflamm (2019) 2019:9367404. doi: 10.1155/2019/9367404
112. Melhem H, Kaya B, Ayata CK, Hruz P, Niess JH. Metabolite-sensing G protein-coupled receptors connect the diet-Microbiota-Metabolites axis to inflammatory bowel disease. Cells (2019) 8(5):450. doi: 10.3390/cells8050450
113. Yu ZT, Nanthakumar NN, Newburg DS. The human milk oligosaccharide 2’-fucosyllactose quenches campylobacter jejuni-induced inflammation in human epithelial cells hep-2 and ht-29 and in mouse intestinal mucosa. J Nutr (2016) 146(10):1980–90. doi: 10.3945/jn.116.230706
114. Morrow AL, Ruiz-Palacios GM, Altaye M, Jiang X, Guerrero ML, Meinzen-Derr JK, et al. Human milk oligosaccharides are associated with protection against diarrhea in breast-fed infants. J Pediatr (2004) 145(3):297–303. doi: 10.1016/j.jpeds.2004.04.054
115. Kuntz S, Kunz C, Borsch C, Vazquez E, Buck R, Reutzel M, et al. Metabolic fate and distribution of 2´-fucosyllactose: Direct influence on gut microbial activity but not on brain. Mol Nutr Food Res (2019) 63(13):e1900035. doi: 10.1002/mnfr.201900035
116. Nanthakumar NN, Dai D, Newburg DS, Walker WA. The role of indigenous microflora in the development of murine intestinal fucosyl- and sialyltransferases. FASEB J (2003) 17(1):44–6. doi: 10.1096/fj.02-0031fje
117. Bry L, Falk PG, Midtvedt T, Gordon JI. A model of host-microbial interactions in an open mammalian ecosystem. Sci (New York NY) (1996) 273(5280):1380–3. doi: 10.1126/science.273.5280.1380
118. Goto Y, Obata T, Kunisawa J, Sato S, Ivanov II, Lamichhane A, et al. Innate lymphoid cells regulate intestinal epithelial cell glycosylation. Sci (New York NY) (2014) 345(6202):1254009. doi: 10.1126/science.1254009
119. Gaboriau-Routhiau V, Rakotobe S, Lécuyer E, Mulder I, Lan A, Bridonneau C, et al. The key role of segmented filamentous bacteria in the coordinated maturation of gut helper T cell responses. Immunity (2009) 31(4):677–89. doi: 10.1016/j.immuni.2009.08.020
120. Pickard JM, Maurice CF, Kinnebrew MA, Abt MC, Schenten D, Golovkina TV, et al. Rapid fucosylation of intestinal epithelium sustains host-commensal symbiosis in sickness. Nature (2014) 514(7524):638–41. doi: 10.1038/nature13823
121. Liu Z, Henderson AL, Nettleton D, Wilson-Welder JH, Hostetter JM, Ramer-Tait A, et al. Mucosal gene expression profiles following the colonization of immunocompetent defined-flora C3h mice with helicobacter bilis: A prelude to typhlocolitis. Microbes infect (2009) 11(3):374–83. doi: 10.1016/j.micinf.2008.12.013
122. Comstock LE, Kasper DL. Bacterial glycans: Key mediators of diverse host immune responses. Cell (2006) 126(5):847–50. doi: 10.1016/j.cell.2006.08.021
123. Shahi SK, Freedman SN, Mangalam AK. Gut microbiome in multiple sclerosis: The players involved and the roles they play. Gut Microbes (2017) 8(6):607–15. doi: 10.1080/19490976.2017.1349041
124. Chen J, Wright K, Davis JM, Jeraldo P, Marietta EV, Murray J, et al. An expansion of rare lineage intestinal microbes characterizes rheumatoid arthritis. Genome Med (2016) 8(1):43. doi: 10.1186/s13073-016-0299-7
125. Rogier R, Evans-Marin H, Manasson J, van der Kraan PM, Walgreen B, Helsen MM, et al. Alteration of the intestinal microbiome characterizes preclinical inflammatory arthritis in mice and its modulation attenuates established arthritis. Sci Rep (2017) 7(1):15613. doi: 10.1038/s41598-017-15802-x
126. Lange L, Thiele GM, McCracken C, Wang G, Ponder LA, Angeles-Han ST, et al. Symptoms of periodontitis and antibody responses to porphyromonas gingivalis in juvenile idiopathic arthritis. Pediatr Rheumatol Online J (2016) 14(1):8. doi: 10.1186/s12969-016-0068-6
127. Hevia A, Milani C, López P, Cuervo A, Arboleya S, Duranti S, et al. Intestinal dysbiosis associated with systemic lupus erythematosus. mBio (2014) 5(5):e01548-14. doi: 10.1128/mBio.01548-14
128. He Z, Shao T, Li H, Xie Z, Wen C. Alterations of the gut microbiome in Chinese patients with systemic lupus erythematosus. Gut Pathog (2016) 8:64. doi: 10.1186/s13099-016-0146-9
129. Neuman H, Koren O. The gut microbiota: A possible factor influencing systemic lupus erythematosus. Curr Opin Rheumatol (2017) 29(4):374–7. doi: 10.1097/bor.0000000000000395
130. Andréasson K, Alrawi Z, Persson A, Jönsson G, Marsal J. Intestinal dysbiosis is common in systemic sclerosis and associated with gastrointestinal and extraintestinal features of disease. Arthritis Res Ther (2016) 18(1):278. doi: 10.1186/s13075-016-1182-z
131. Szymula A, Rosenthal J, Szczerba BM, Bagavant H, Fu SM. Deshmukh US. T cell epitope mimicry between sjögren’s syndrome antigen a (Ssa)/Ro60 and oral, gut, skin and vaginal bacteria. Clin Immunol (2014) 152(1-2):1–9. doi: 10.1016/j.clim.2014.02.004
132. Siddiqui H, Chen T, Aliko A, Mydel PM, Jonsson R, Olsen I. Microbiological and bioinformatics analysis of primary sjogren’s syndrome patients with normal salivation. J Oral Microbiol (2016) 8:31119. doi: 10.3402/jom.v8.31119
133. Mandl T, Marsal J, Olsson P, Ohlsson B, Andréasson K. Severe intestinal dysbiosis is prevalent in primary sjögren’s syndrome and is associated with systemic disease activity. Arthritis Res Ther (2017) 19(1):237. doi: 10.1186/s13075-017-1446-2
134. de Paiva CS, Jones DB, Stern ME, Bian F, Moore QL, Corbiere S, et al. Altered mucosal microbiome diversity and disease severity in sjögren syndrome. Sci Rep (2016) 6:23561. doi: 10.1038/srep23561
135. Zhang L, Han R, Zhang X, Fang G, Chen J, Li J, et al. Fecal microbiota in patients with ankylosing spondylitis: Correlation with dietary factors and disease activity. Clin Chim Acta (2019) 497:189–96. doi: 10.1016/j.cca.2019.07.038
136. Yin J, Sternes PR, Wang M, Song J, Morrison M, Li T, et al. Shotgun metagenomics reveals an enrichment of potentially cross-reactive bacterial epitopes in ankylosing spondylitis patients, as well as the effects of tnfi therapy upon microbiome composition. Ann Rheum Dis (2020) 79(1):132–40. doi: 10.1136/annrheumdis-2019-215763
137. Cosorich I, Dalla-Costa G, Sorini C, Ferrarese R, Messina MJ, Dolpady J, et al. High frequency of intestinal T(H)17 cells correlates with microbiota alterations and disease activity in multiple sclerosis. Sci Adv (2017) 3(7):e1700492. doi: 10.1126/sciadv.1700492
138. Ventura RE, Iizumi T, Battaglia T, Liu M, Perez-Perez GI, Herbert J, et al. Gut microbiome of treatment-naïve Ms patients of different ethnicities early in disease course. Sci Rep (2019) 9(1):16396. doi: 10.1038/s41598-019-52894-z
139. Duscha A, Gisevius B, Hirschberg S, Yissachar N, Stangl GI, Eilers E, et al. Propionic acid shapes the multiple sclerosis disease course by an immunomodulatory mechanism. Cell (2020) 180(6):1067–80.e16. doi: 10.1016/j.cell.2020.02.035
140. Murri M, Leiva I, Gomez-Zumaquero JM, Tinahones FJ, Cardona F, Soriguer F, et al. Gut microbiota in children with type 1 diabetes differs from that in healthy children: A case-control study. BMC Med (2013) 11:46. doi: 10.1186/1741-7015-11-46
141. Giongo A, Gano KA, Crabb DB, Mukherjee N, Novelo LL, Casella G, et al. Toward defining the autoimmune microbiome for type 1 diabetes. Isme J (2011) 5(1):82–91. doi: 10.1038/ismej.2010.92
142. Brown CT, Davis-Richardson AG, Giongo A, Gano KA, Crabb DB, Mukherjee N, et al. Gut microbiome metagenomics analysis suggests a functional model for the development of autoimmunity for type 1 diabetes. PloS One (2011) 6(10):e25792. doi: 10.1371/journal.pone.0025792
143. Zhou H, Sun L, Zhang S, Zhao X, Gang X, Wang G. Evaluating the causal role of gut microbiota in type 1 diabetes and its possible pathogenic mechanisms. Front Endocrinol (2020) 11:125. doi: 10.3389/fendo.2020.00125
144. Fang Y, Zhang C, Shi H, Wei W, Shang J, Zheng R, et al. Characteristics of the gut microbiota and metabolism in patients with latent autoimmune diabetes in adults: A case-control study. Diabetes Care (2021) 44(12):2738–46. doi: 10.2337/dc20-2975
145. Johnson CC, Ownby DR. Allergies and asthma: Do atopic disorders result from inadequate immune homeostasis arising from infant gut dysbiosis? Expert Rev Clin Immunol (2016) 12(4):379–88. doi: 10.1586/1744666x.2016.1139452
146. Sjögren YM, Tomicic S, Lundberg A, Böttcher MF, Björkstén B, Sverremark-Ekström E, et al. Influence of early gut microbiota on the maturation of childhood mucosal and systemic immune responses. Clin Exp allergy: J Br Soc Allergy Clin Immunol (2009) 39(12):1842–51. doi: 10.1111/j.1365-2222.2009.03326.x
147. Simelyte E, Rimpiläinen M, Zhang X, Toivanen P. Role of peptidoglycan subtypes in the pathogenesis of bacterial cell wall arthritis. Ann rheum Dis (2003) 62(10):976–82. doi: 10.1136/ard.62.10.976
148. Koga M, Gilbert M, Li J, Yuki N. Complex of Gm1- and Gd1a-like lipo-oligosaccharide mimics Gm1b, inducing anti-Gm1b antibodies. PloS One (2015) 10(4):e0124004. doi: 10.1371/journal.pone.0124004
149. Varki A, Lennarz WJ, Lane MD. Siglecs. In: Encyclopedia of biological chemistry. New York: Elsevier (2004). p. 38–40.
150. Anderluh M, Berti F, Bzducha-Wróbel A, Chiodo F, Colombo C, Compostella F, et al. Emerging glyco-based strategies to steer immune responses. FEBS J (2021) 288(16):4746–72. doi: 10.1111/febs.15830
151. Greiling TM, Dehner C, Chen X, Hughes K, Iñiguez AJ, Boccitto M, et al. Commensal orthologs of the human autoantigen Ro60 as triggers of autoimmunity in lupus. Sci Trans Med (2018) 10(434):eaan2306. doi: 10.1126/scitranslmed.aan2306
152. Hurd EA, Domino SE. Increased susceptibility of secretor factor gene Fut2-null mice to experimental vaginal candidiasis. Infect Immun (2004) 72(7):4279–81. doi: 10.1128/iai.72.7.4279-4281.2004
153. Zezina E, Sercan-Alp O, Herrmann M, Biesemann N. Glucose transporter 1 in rheumatoid arthritis and autoimmunity. Wiley Interdiscip Rev Syst Biol Med (2020) 12(4):e1483. doi: 10.1002/wsbm.1483
154. Weyand CM, Zeisbrich M, Goronzy JJ. Metabolic signatures of T-cells and macrophages in rheumatoid arthritis. Curr Opin Immunol (2017) 46:112–20. doi: 10.1016/j.coi.2017.04.010
155. Okano T, Saegusa J, Nishimura K, Takahashi S, Sendo S, Ueda Y, et al. 3-bromopyruvate ameliorate autoimmune arthritis by modulating Th17/Treg cell differentiation and suppressing dendritic cell activation. Sci Rep (2017) 7:42412. doi: 10.1038/srep42412
156. Abboud G, Choi SC, Kanda N, Zeumer-Spataro L, Roopenian DC, Morel L. Inhibition of glycolysis reduces disease severity in an autoimmune model of rheumatoid arthritis. Front Immunol (2018) 9:1973. doi: 10.3389/fimmu.2018.01973
157. Mendez-Huergo SP, Hockl PF, Stupirski JC, Maller SM, Morosi LG, Pinto NA, et al. Clinical relevance of galectin-1 and galectin-3 in rheumatoid arthritis patients: Differential regulation and correlation with disease activity. Front Immunol (2018) 9:3057. doi: 10.3389/fimmu.2018.03057
158. Li J, Hsu HC, Ding Y, Li H, Wu Q, Yang P, et al. Inhibition of fucosylation reshapes inflammatory macrophages and suppresses type ii collagen-induced arthritis. Arthritis Rheumatol (Hoboken NJ) (2014) 66(9):2368–79. doi: 10.1002/art.38711
159. Parekh RB, Dwek RA, Sutton BJ, Fernandes DL, Leung A, Stanworth D, et al. Association of rheumatoid arthritis and primary osteoarthritis with changes in the glycosylation pattern of total serum igg. Nature (1985) 316(6027):452–7. doi: 10.1038/316452a0
160. Vučković F, Krištić J, Gudelj I, Teruel M, Keser T, Pezer M, et al. Association of systemic lupus erythematosus with decreased immunosuppressive potential of the igg glycome. Arthritis Rheumatol (Hoboken NJ) (2015) 67(11):2978–89. doi: 10.1002/art.39273
161. Gheita TA, Raafat HA, Sayed S, El-Fishawy H, Nasrallah MM, Abdel-Rasheed E. Metabolic syndrome and insulin resistance comorbidity in systemic lupus erythematosus. effect on carotid intima-media thickness. Z fur Rheumatol (2013) 72(2):172–7. doi: 10.1007/s00393-012-1058-9
162. Parra S, Cabré A, Marimon F, Ferré R, Ribalta J, Gonzàlez M, et al. Circulating Fabp4 is a marker of metabolic and cardiovascular risk in sle patients. Lupus (2014) 23(3):245–54. doi: 10.1177/0961203313517405
163. Chung CP, Long AG, Solus JF, Rho YH, Oeser A, Raggi P, et al. Adipocytokines in systemic lupus erythematosus: Relationship to inflammation, insulin resistance and coronary atherosclerosis. Lupus (2009) 18(9):799–806. doi: 10.1177/0961203309103582
164. Otto G. Selectins block T cells in sle. Nat Rev Rheumatol (2021) 17(9):510. doi: 10.1038/s41584-021-00671-6
165. Mathur D, López-Rodas G, Casanova B, Marti MB. Perturbed glucose metabolism: Insights into multiple sclerosis pathogenesis. Front Neurol (2014) 5:250. doi: 10.3389/fneur.2014.00250
166. Mathur D, Riffo-Campos AL, Castillo J, Haines JD, Vidaurre OG, Zhang F, et al. Bioenergetic failure in rat oligodendrocyte progenitor cells treated with cerebrospinal fluid derived from multiple sclerosis patients. Front Cell Neurosci (2017) 11:209. doi: 10.3389/fncel.2017.00209
167. Mathur D, María-Lafuente E, Ureña-Peralta JR, Sorribes L, Hernández A, Casanova B, et al. Disturbed glucose metabolism in rat neurons exposed to cerebrospinal fluid obtained from multiple sclerosis subjects. Brain Sci (2017) 8(1):1. doi: 10.3390/brainsci8010001
168. Tang X, Wang W, Hong G, Duan C, Zhu S, Tian Y, et al. Gut microbiota-mediated lysophosphatidylcholine generation promotes colitis in intestinal epithelium-specific Fut2 deficiency. J Biomed Sci (2021) 28(1):20. doi: 10.1186/s12929-021-00711-z
169. Parada Venegas D, de la Fuente MK, Landskron G, González MJ, Quera R, Dijkstra G, et al. Short chain fatty acids (Scfas)-mediated gut epithelial and immune regulation and its relevance for inflammatory bowel diseases. Front Immunol (2019) 10:277. doi: 10.3389/fimmu.2019.00277
170. Belcher JD, Chen C, Nguyen J, Abdulla F, Nguyen P, Nguyen M, et al. The fucosylation inhibitor, 2-fluorofucose, inhibits vaso-occlusion, leukocyte-endothelium interactions and nf-ĸb activation in transgenic sickle mice. PloS One (2015) 10(2):e0117772. doi: 10.1371/journal.pone.0117772
171. Pagan JD, Kitaoka M, Anthony RM. Engineered sialylation of pathogenic antibodies in vivo attenuates autoimmune disease. Cell (2018) 172(3):564–77.e13. doi: 10.1016/j.cell.2017.11.041
172. Li T, DiLillo DJ, Bournazos S, Giddens JP, Ravetch JV, Wang LX. Modulating igg effector function by fc glycan engineering. Proc Natl Acad Sci United States America (2017) 114(13):3485–90. doi: 10.1073/pnas.1702173114
173. Gibson GR, Probert HM, Loo JV, Rastall RA, Roberfroid MB. Dietary modulation of the human colonic microbiota: Updating the concept of prebiotics. Nutr Res Rev (2004) 17(2):259–75. doi: 10.1079/nrr200479
174. Yu ZT, Liu B, Mukherjee P, Newburg DS. Trametes versicolor extract modifies human fecal microbiota composition in vitro. Plant Foods Hum Nutr (2013) 68(2):107–12. doi: 10.1007/s11130-013-0342-4
175. Zamani B, Golkar HR, Farshbaf S, Emadi-Baygi M, Tajabadi-Ebrahimi M, Jafari P, et al. Clinical and metabolic response to probiotic supplementation in patients with rheumatoid arthritis: A randomized, double-blind, placebo-controlled trial. Int J Rheum Dis (2016) 19(9):869–79. doi: 10.1111/1756-185x.12888
176. Luo Y, Liu L, Chen D, Yu B, Zheng P, Mao X, et al. Dietary supplementation of fructooligosaccharides alleviates enterotoxigenic e. coli-induced disruption of intestinal epithelium in a weaned piglet model. Br J Nutr (2021) 75(2):1–27. doi: 10.1017/s0007114521004451
177. Fuhren J, Schwalbe M, Boekhorst J, Rösch C, Schols HA, Kleerebezem M. Dietary calcium phosphate strongly impacts gut microbiome changes elicited by inulin and galacto-oligosaccharides consumption. Microbiome (2021) 9(1):218. doi: 10.1186/s40168-021-01148-0
178. Renye JA Jr., White AK, Hotchkiss AT Jr. Identification of lactobacillus strains capable of fermenting fructo-oligosaccharides and inulin Microorganisms (2021) 9(10):2020 doi: 10.3390/microorganisms9102020
179. Gu J, Mao B, Cui S, Tang X, Liu Z, Zhao J, et al. Bifidobacteria exhibited stronger ability to utilize fructooligosaccharides, compared with other bacteria in the mouse intestine. J Sci Food Agric (2021) 102(6):2413–23. doi: 10.1002/jsfa.11580
180. Hajar-Azhari S, Hafiz Abd Rahim M, Razid Sarbini S, Muhialdin BJ, Olusegun L, Saari N. Enzymatically synthesised fructooligosaccharides from sugarcane syrup modulate the composition and short-chain fatty acid production of the human intestinal microbiota. Food Res Int (2021) 149:110677. doi: 10.1016/j.foodres.2021.110677
181. Liao M, Zhang Y, Qiu Y, Wu Z, Zhong Z, Zeng X, et al. Fructooligosaccharide supplementation alleviated the pathological immune response and prevented the impairment of intestinal barrier in dss-induced acute colitis mice. Food Funct (2021) 12(20):9844–54. doi: 10.1039/d1fo01147b
182. Zegarra-Ruiz DF, El Beidaq A, Iñiguez AJ, Lubrano Di Ricco M, Manfredo Vieira S, Ruff WE, et al. A diet-sensitive commensal lactobacillus strain mediates Tlr7-dependent systemic autoimmunity. Cell Host Microbe (2019) 25(1):113–27.e6. doi: 10.1016/j.chom.2018.11.009
183. Allen JG, Mujacic M, Frohn MJ, Pickrell AJ, Kodama P, Bagal D, et al. Facile modulation of antibody fucosylation with small molecule fucostatin inhibitors and cocrystal structure with gdp-mannose 4,6-dehydratase. ACS Chem Biol (2016) 11(10):2734–43. doi: 10.1021/acschembio.6b00460
184. Wilson CS, Stocks BT, Hoopes EM, Rhoads JP, McNew KL, Major AS, et al. Metabolic preconditioning in Cd4+ T cells restores inducible immune tolerance in lupus-prone mice. JCI Insight (2021) 6(19):e143245. doi: 10.1172/jci.insight.143245
185. McHugh J. Autoimmunity: Glycoengineering has therapeutic potential. Nat Rev Rheumatol (2018) 14(3):121. doi: 10.1038/nrrheum.2018.8
186. An X, Jiang G, Cheng C, Lv Z, Liu Y, Wang F. Inhibition of platelets by clopidogrel suppressed ang ii-induced vascular inflammation, oxidative stress, and remodeling. J Am Heart Assoc (2018) 7(21):e009600. doi: 10.1161/jaha.118.009600
187. Zhou J, Wang Y, Fan Q, Liu Y, Liu H, Yan J, et al. High levels of fucosylation and sialylation of milk n-glycans from mothers with gestational diabetes mellitus alter the offspring gut microbiome and immune balance in mice. FASEB J (2020) 34(3):3715–31. doi: 10.1096/fj.201901674R
188. Kearney SM, Gibbons SM, Erdman SE, Alm EJ. Orthogonal dietary niche enables reversible engraftment of a gut bacterial commensal. Cell Rep (2018) 24(7):1842–51. doi: 10.1016/j.celrep.2018.07.032
189. Ertaş Öztürk Y, Helvaci EM, Sökülmez Kaya P, Terzi M. Is Mediterranean diet associated with multiple sclerosis related symptoms and fatigue severity? Nutr Neurosci (2022) 25(2):1–7. doi: 10.1080/1028415x.2022.2034241
190. Widmer RJ, Flammer AJ, Lerman LO, Lerman A. The Mediterranean diet, its components, and cardiovascular disease. Am J Med (2015) 128(3):229–38. doi: 10.1016/j.amjmed.2014.10.014
191. Li X, Shah A, Franklin P, Merolli R, Bradley J, Busconi B. Arthroscopic debridement of the osteoarthritic knee combined with hyaluronic acid (Orthovisc) treatment: A case series and review of the literature. J orthop Surg Res (2008) 3:43. doi: 10.1186/1749-799x-3-43
192. Yu C, Li L, Liang D, Wu A, Dong Q, Jia S, et al. Glycosaminoglycan-based injectable hydrogels with multi-functions in the alleviation of osteoarthritis. Carbohydr polymers (2022) 290:119492. doi: 10.1016/j.carbpol.2022.119492
193. Pozharitskaya ON, Shikov AN, Faustova NM, Obluchinskaya ED, Kosman VM, Vuorela H, et al. Pharmacokinetic and tissue distribution of fucoidan from fucus vesiculosus after oral administration to rats. Mar Drugs (2018) 16(4):132. doi: 10.3390/md16040132
194. Wu G, Niu M, Tang W, Hu J, Wei G, He Z, et al. L-fucose ameliorates high-fat diet-induced obesity and hepatic steatosis in mice. J Trans Med (2018) 16(1):344. doi: 10.1186/s12967-018-1718-x
195. Singh MP, Petersen PJ, Weiss WJ, Kong F, Greenstein M. Saccharomicins, novel heptadecaglycoside antibiotics produced by saccharothrix espanaensis: Antibacterial and mechanistic activities. Antimicrob Agents chemother (2000) 44(8):2154–9. doi: 10.1128/aac.44.8.2154-2159.2000
Keywords: autoimmune diseases, glucose metabolism, glycosylation, gut microbiota, systemic lupus erythematosus
Citation: Wang L, Xu H, Yang H, Zhou J, Zhao L and Zhang F (2022) Glucose metabolism and glycosylation link the gut microbiota to autoimmune diseases. Front. Immunol. 13:952398. doi: 10.3389/fimmu.2022.952398
Received: 25 May 2022; Accepted: 19 August 2022;
Published: 20 September 2022.
Edited by:
Tianfu Wu, University of Houston, United StatesReviewed by:
Laurence Morel, The University of Texas Health Science Center at San Antonio, United StatesCopyright © 2022 Wang, Xu, Yang, Zhou, Zhao and Zhang. This is an open-access article distributed under the terms of the Creative Commons Attribution License (CC BY). The use, distribution or reproduction in other forums is permitted, provided the original author(s) and the copyright owner(s) are credited and that the original publication in this journal is cited, in accordance with accepted academic practice. No use, distribution or reproduction is permitted which does not comply with these terms.
*Correspondence: Jiaxin Zhou, cHVtY3pob3VAc2luYS5jb20=; Lidan Zhao, emhhb2xpZGFuQGhvdG1haWwuY29t
†These authors have contributed equally to this work
Disclaimer: All claims expressed in this article are solely those of the authors and do not necessarily represent those of their affiliated organizations, or those of the publisher, the editors and the reviewers. Any product that may be evaluated in this article or claim that may be made by its manufacturer is not guaranteed or endorsed by the publisher.
Research integrity at Frontiers
Learn more about the work of our research integrity team to safeguard the quality of each article we publish.