- 1Department of Pulmonary and Critical Care Medicine, Shanghai Fifth People’s Hospital, Fudan University, Shanghai, China
- 2Center of Community-Based Health Research, Fudan University, Shanghai, China
The immune system generates memory cells on infection with a virus for the first time. These memory cells play an essential role in protection against reinfection. Tissue-resident memory T (TRM) cells can be generated in situ once attacked by pathogens. TRM cells dominate the defense mechanism during early stages of reinfection and have gradually become one of the most popular focuses in recent years. Here, we mainly reviewed the development and regulation of various TRM cell signaling pathways in the respiratory tract. Moreover, we explored the protective roles of TRM cells in immune response against various respiratory viruses, such as Respiratory Syncytial Virus (RSV) and influenza. The complex roles of TRM cells against SARS-CoV-2 infection are also discussed. Current evidence supports the therapeutic strategies targeting TRM cells, providing more possibilities for treatment. Rational utilization of TRM cells for therapeutics is vital for defense against respiratory viruses.
Introduction
Being exposed to various stimuli, the host forms an immune defense to protect the body for the first viral encounter and preserve immunological memory to prepare for reinfection. Memory T cells are the backbone of this process and are classified as central memory T (TCM) cells and effector memory T (TEM) cells (1). Generally, TCM cells express chemokine receptor CCR7, homing molecule CD62L, and Krupple-Like Factor 2 (KLF2), circulating in the peripheral lymph system for surveillance of secondary lymphoid organs and recalling of proliferative responses. Distribution of TCM cells includes peripheral tissues, immune organs, and lymph nodes (1–3). TEM cells are mainly located in non-lymphocyte tissues and organs, with the expression of CCR7 and CD62L being hardly detected. TEM cells participate in the body circulation and migrate to the peripheral inflammatory tissues to induce rapid effects (1–3). Later, resident and self-sustaining memory T cells surviving in the non-lymphoid tissues, namely TRM cells, were identified by parabiosis and tissue transplantation experiments (4, 5). Despite the shared cell marker CD44+ of memory T cells, TRM cells highly express CD69 and/or CD103 instead of CCR7 and CD62L (6, 7). They are the most abundant subset of memory T cells and reside in the non-lymphoid tissues for a long time, contributing to rapid and critical protective immune responses (8). In addition to proliferation, TRM cells secrete cytokines and pass the signals to other cells like natural killer cells to dominate local memory responses (9, 10) (Table 1; Figure 1).
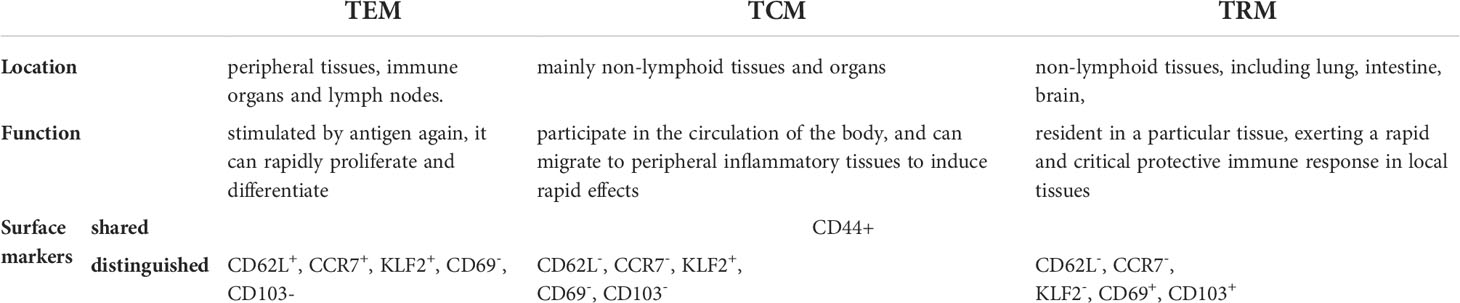
Table 1 basic traits of effector memory T cells, central memory T cells and resident memory T cells.
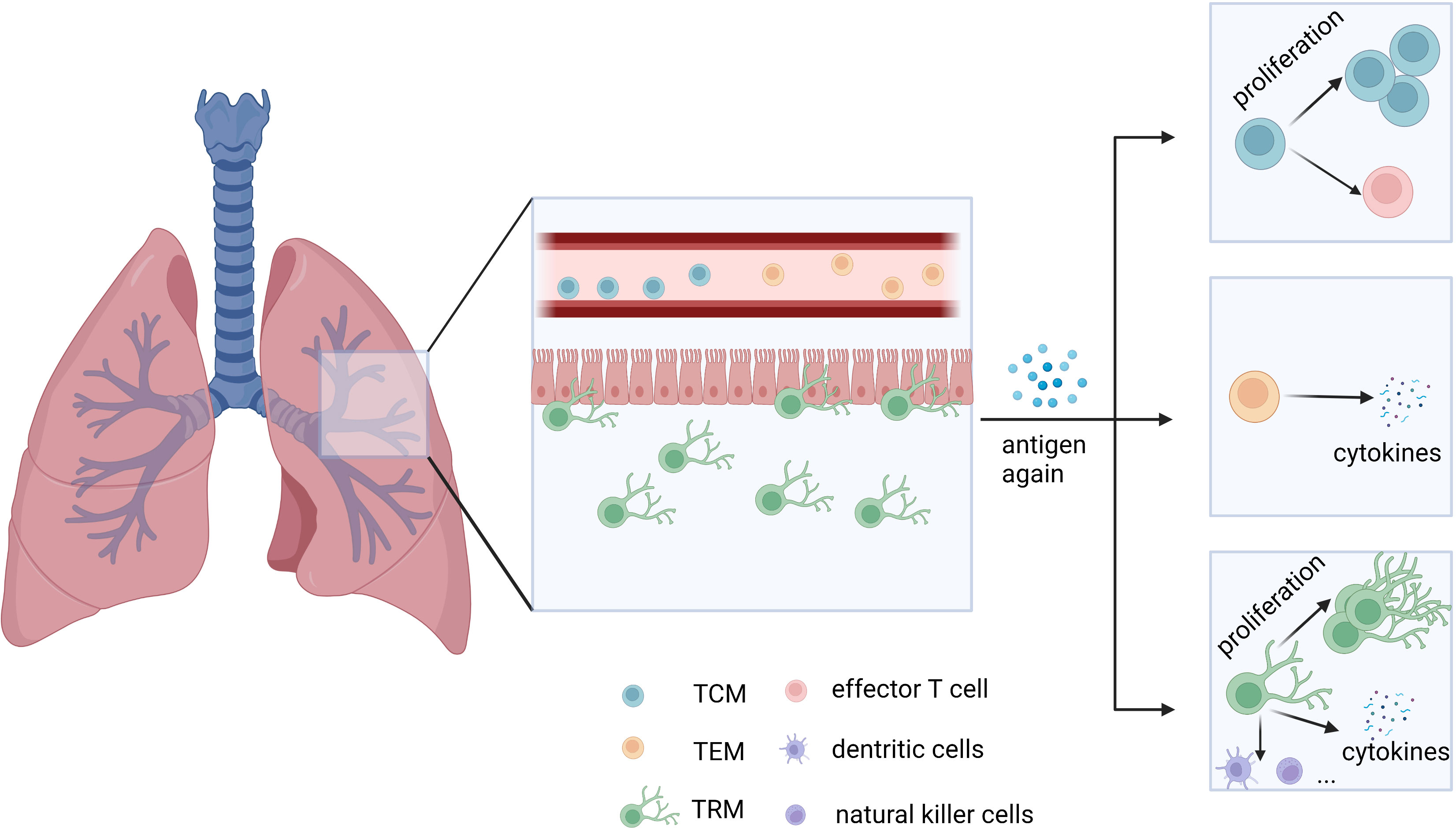
Figure 1 General distribution of TEM, TCM, and TRM cells in the lungs and their main mode of function. TEM and TCM cells migrate in circulation, while TRM cells reside in tissues. When stimulated by antigens again, TCM cells rapidly proliferate and differentiate, while TEM cells secrete effector molecules like granzyme B, similar to CD8+ T cells. TRM cells exert a rapid and critical immune response on local tissues. TCM: central memory T cells. TEM: effector memory T cells. TRM: tissue-resident memory T cells.
TRM cells found in both the upper and lower respiratory tract play an important role in the localized defense against respiratory infections. Due to rapid spread through air, respiratory viruses pose a considerable threat to humans. In recent years, respiratory viruses have stood on the stage of global pandemic. Having a deeper understanding of the role of immune cells in protecting the respiratory tract helps curb the spread of respiratory disease, casting light on new strategies. In this review, we summarize the characteristics of TRM cells and their complicated roles during RSV, Influenza, and SARS-CoV-2 infection. Several potential preventive and therapeutic strategies based on TRM cells are discussed.
Development of TRM cells in respiratory Tract
Once viruses attack the respiratory tract, DNGR1+ dendritic cells (DCs) preferentially migrate to the mediastinal lymph nodes (medLNs). These DCs activate naïve T cells to differentiate into precursor effector T cells (CD8+ T cells), some of which further migrate to the nasal cavity or lungs and convert into TRM cells (2, 11). Precursor CD4+ T cells can also transform into tissue-resident memory T cells. Generally, the phenotype of pulmonary CD4+ TRM cells is regarded as CD69+CD103-, while the phenotype of CD8+ TRM cells is CD69+CD103+ (2). Other phenotypes of TRM cells in respiratory viruses are summarized in Table 2. Multiple signaling pathways contribute to the initiation and maintenance of TRM cells.
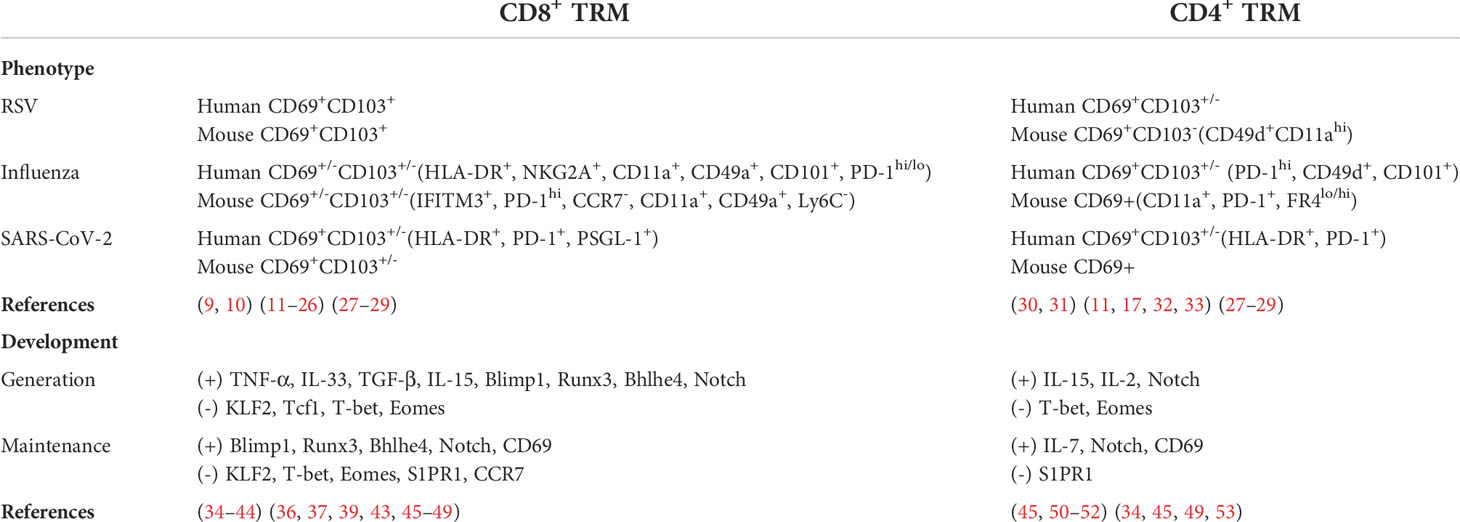
Table 2 phenotypes of CD8+ and CD4+ TRM cells in respiratory viruses, as well as cytokines and transcription factors in the regulation of pulmonary TRM cells.
Initiation and generation of TRM cells
The initiation and generation of TRM cells involves different signaling pathways and transcriptional factors that vary in different tissues. Local antigen stimulation triggers the initiation of TRM cells in various tissues, including the lungs, but not in the nasal cavity (36). Cytokines like TGF-β, interleukin (IL), and TNF-α are also involved in the regulation of TRM cell development. TGF-β has multiple effects in adaptive immunity with complex biological activities (37). Pulmonary CD103 expression by CD8+ TRM cells relies on TGF-β, which is regulated by CD1c+ DCs (38). TGF-β, IL-33, and TNF-α can also inhibit Krupple-Like Factor 2 (KLF2) expression to downregulate the expression of sphingosine-1-phosphate receptor (S1PR1), resulting in elevated CD69 expression in CD8+ TRM cells (39). Furthermore, TGF-β downregulates other transcription factors in the lungs to promote the establishment of pulmonary CD8+ TRM cells, such as T-bet, eomesodermin (Eomes), and transcription factor 1 (40, 41). In mouse experiments, high expression levels of T-bet, Eomes, and transcription factor 1 inhibit CD103 expression (41–43). Expression of CD103 can be stimulated either directly by Runx3 or Blimp1, which has been proven in the development of gut TRM cells (44, 50). Since TGF-β is upstream of Runx3 and Blimp1 in the gut, it might also regulate CD103 in the lungs by the same pathway. Additionally, transcription factor Bhlhe4 can also increase the expression of CD103 directly or indirectly via the recruitment of Runx3 (42, 51).
IL-15 reduces T-bet expression to mediate CD8+ TRM cell generation together with TGF-β (40). IL-33 and IL-12 increase Blimp-1 while inhibiting Eomes and TCF-1, promoting the generation of pulmonary CD8+ TRM cells (45). Additionally, IL-15 and IL-2 aid in the formation of CD4+ TRM cells in asthmatic mouse models (52, 54). In addition to ILs, other regulators shared by CD4+ and CD8+ T cells include low levels of transcription factors T-bet and Eomes and upregulated Notch signaling (40, 42, 55, 56). However, the detailed signaling mechanisms of CD4+ TRM cell generation are still unclear and more relevant studies are necessary.
Cell environment in situ can also influence TRM cell formation. Lung macrophages can regulate TRM cell formation, while their effects according to different experiments seem inconsistent. In influenza mouse models, macrophages exert negative effects (46), while human pulmonary macrophages provide costimulatory signals during CD8+ TRM cells generation (21). CD4+ T cells and T regulatory cells expressing T-bet assist in the formation of CD103+ pulmonary CD8+ TRM cells (29, 47).
Maintenance of TRM cells
The distinguishing characteristic of TRM cells is that they can sustain in non-lymphoid tissue for a long time, acting as the assault force in secondary infection. Repair-associated memory depots are the major niche where CD8+ TRM cells are stored in the lungs (48). Transcription factors play vital roles in the survival of TRM cells. Runx3 and Blimp1, which inhibit transcription factor 1, maintain the survival of CD8+ TRM cells by suppressing egress receptors CCR7 and S1PR1 (49, 57). S1PR1 induces the chemotaxis of sphingosinol-1-phosphate, mediating the expulsion of T cells from the tissues (53). Therefore, FTY720, an S1PR1 agonist, is often used in research focused on TRM cells to inhibit peripheral lymphatic circulation (58). KLF2, which promotes S1PR1 expression, is downregulated after CD8+ TRM cells enter non-lymphoid tissues (39). Furthermore, CD8+ TRM cells are sustained in a functional epigenetic state by Bhlhe4 (51). Reduction of T-bet and Eomes also enhances the maintenance of CD8+ TRM cells (40).
Additionally, some transcription factors regulating maintenance are common to both CD4+ and CD8+ TRM cells. Notch promotes the survival of CD4+ and CD8+ TRM cells (42, 55). CD69 antagonizes S1PR1 and inhibits TRM cells excretion (59), and research demonstrates that TRM cells cannot sustain in CD69-/- mice (27). With reference to CD4+ TRM cells, IL-7 is critical for the maintenance of CD4+ TRM cells in the lungs (60). Distinct from CD8+ TRM cells, inducible bronchus associated lymphoid tissue is the major niche for pulmonary CD4+ TRM cells (61).
Evidently, generation, maintenance, and regulation of TRM cells in the lungs is complicated (Figure 2; Figure 3; Table 2) and involves a variety of factors. Additional details are needed regarding the mechanism of TRM cell regulation to understand their traits. Thereafter, we can control the physiological process managed by them to regulate immunological homeostasis.
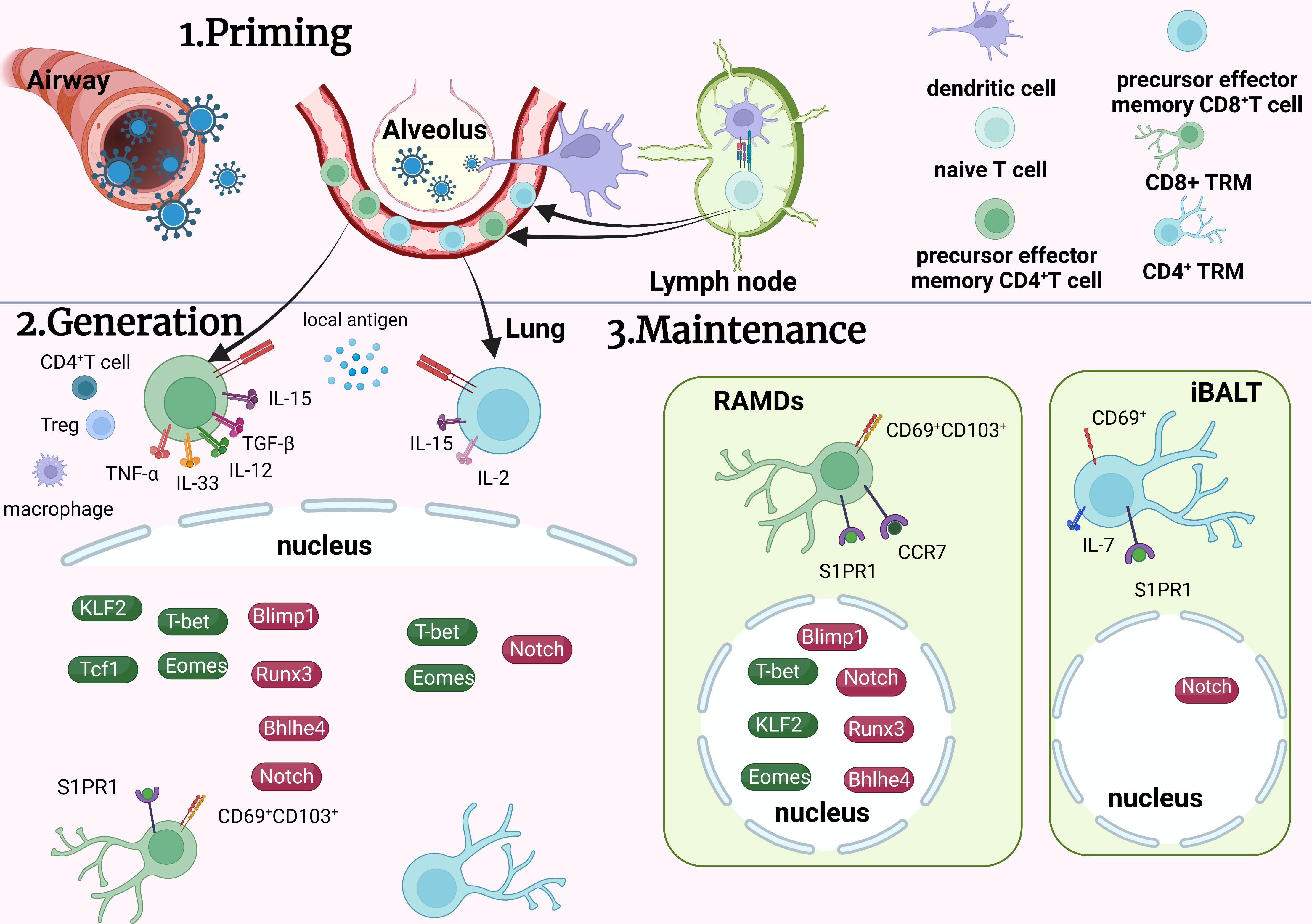
Figure 2 Development of TRM cells in the lung. On contact with the virus, specific dendritic cells migrate to lymph nodes to communicate with naïve T cells and activate the generation of effector T cells. Some effector T cells migrate to the lungs and transform into TRM cells by various signaling pathways. CD8+ TRM cells accumulate in RAMDs and CD4+ TRM cells accumulate in iBALs after formation. To maintain TRM cells in the lungs, many transcription factors coordinate with each other to achieve overall functionality. TRM, tissue resident memory T cells; RAMDs, repair associated memory depot; iBALs, inducible bronchus associated lymphoid tissue; APC, antigen presenting cells; Green icons, inhibitory roles; red icons, facilitating roles.
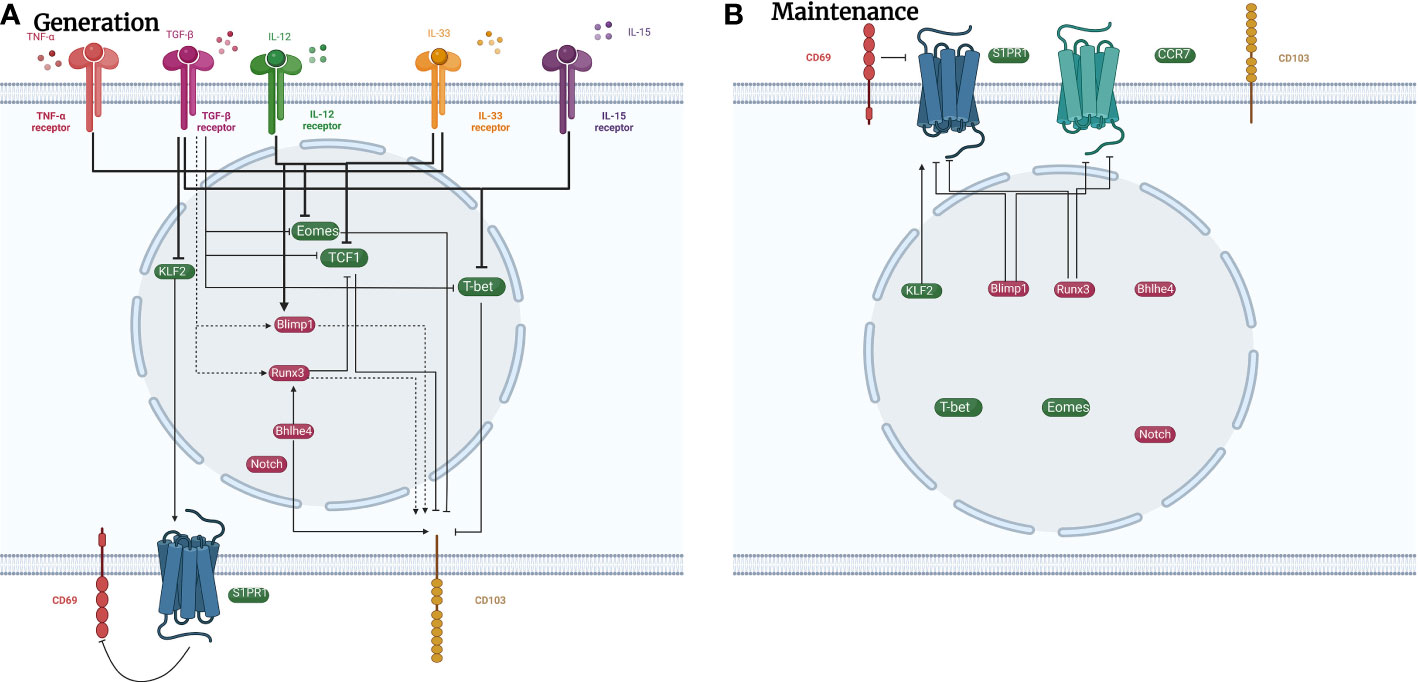
Figure 3 Signaling pathways in regulating the generation and maintenance of pulmonary CD8+ TRM cells. Plenty of signaling pathways regulate the development of CD8+ TRM cells. (A) TNF-α, IL-33, TGF-β, and IL-15 promote the development of CD8+ TRM cells by increasing the expression of transcription factors such as Runx3, Blimp1, and Bhlhe4, and decreasing the expression of transcription factors such as Eomes, T-bet, and KLF2. (B) Transcription factors such as Blimp1 and Runx3 inhibit the expression of cell surface receptors CCR7 and S1PR1 to maintain the presence of CD8+ TRM cells in the lungs. Cell surface marker CD69 can also decrease the expression of S1PR1 to sustain CD8+ TRM cells. green icons: inhibitory roles; red icons: facilitating roles. dotted line: possible impact.
Basic functions and ‘Workflow’ of TRM cells
As fundamental components of the immune network, TRM cells monitor local homeostasis (8). Furthermore, they participate in the response to external stimuli and internal abnormalities, maintaining homeostasis (13, 62). TRM cells patrol colonization site, recognize reinfection antigen or tumor-associated antigens, secrete pro-inflammatory cytokines, and release cytotoxic particles to eliminate these antigens (63). Contrastingly, signals induced by TRM cells break tissue homeostasis with hybrid populations of white blood cells. For instance, independent of circulating immune memory cells, CD4+ TRM cells are considered the primary reactive immune memory cells in the early period of asthma in house dust mite asthmatic mouse model (54). Clearly, functions of TRM cells are diverse under different circumstances.
TRM cells work mainly in two steps: the first step is scanning. Long resident memory T cells slowly and randomly migrate to previously infected tissues. In the inflammatory tissue, T cells increase their movement five-fold in the mucosa and bind to fibronectin with integrins CD103 and CD49a, which are important markers of TRM cells in the lungs (64, 65). Local immune surveillance against relapse or reinfection is enhanced by TRM cells scanning the environment (66). On contact with the antigen, TRM cells initiate the second step: clearance. In addition to the direct control of the pathogen, some tissue-specific CD8+ TRM cells sustain constitutive granzyme B expression, mediate extracellular toxicity (67, 68), and eliminate pathogens by non-cytolytic progress (69). Furthermore, they convey this information to neighboring cells, stimulating localized memory responses. TRM cell-dependent parenchymal immunity, including innate and adaptive immune activation, triggers the induction of locally protective antiviral status (9, 10).
Overall, TRM cells can settle in a particular niche for a long time, leading to a rapid and critical protective immune response in the local tissues. Through the two steps —scan and clearance — TRM cells are involved in infection protection, tumor control, and monitoring homeostasis to play various roles in different diseases.
Role of TRM cells in respiratory virus infection
Respiratory syncytial virus
Since it was first isolated in Chimpanzee with respiratory illness in 1957 (70), RSV has evolved as a vast threat to children, adults, and the elderly, especially infants. RSV-associated hospitalization rates are highest in infants younger than six months and almost all children get infected with RSV by the age of two (71). A large systematic study has shown that RSV was responsible for 2.0% and 3.6% of deaths in children aged 0–60 months and 28 days–6 months, respectively, in 2019 (72).
In an RSV mouse model, Kinnear et al. observed that TRM cells have a protective effect against RSV (13). Compared with mice infected with RSV for the second and third time, mice infected for the first time had significantly lower body weight, increased viral load, and significantly lower serum anti-RSV immunoglobulin after infection. Mice infected multiple times produced numerous antigen-specific CD8+ TRM cells in the respiratory tract. Researchers then transferred cells from the respiratory tract of previously infected mice to the respiratory tract of uninfected mice, which partially rescued the weight loss in uninfected mice. Airway CD8+ TRM cells decreased weight loss, viral load, and increased IFN-γ, suggesting their protective effect against RSV infection. In RSV infected healthy adult volunteers, pulmonary virus-specific CD8+ TRM cells were observed which accumulated extensively during the recovery period (12). During infection, the proportion of CD8+ T cells expressing CD103 was upregulated, peaking on day 10 and decreasing in the bronchoalveolar lavage (BAL) as the infection subsided. Enrichment of CD8+ TRM cells in the airway was associated with mitigated respiratory symptoms, virus control, and reduced disease severity. This indicates that CD8+ TRM cells in human lungs defend against severe respiratory viral disease. RSV-specific CD8+ T cells in the BAL of African green monkey were about ten times higher than that in the blood, showing effector memory (CD95+CD28-)/tissue-resident memory (CD69+CD103+) T cell phenotype (73). The dynamics of RSV-specific CD8+ T cells in blood and BAL were associated with a decrease in the viral titer. Different models have shown that TRM cells protect the host against RSV (Figure 4).
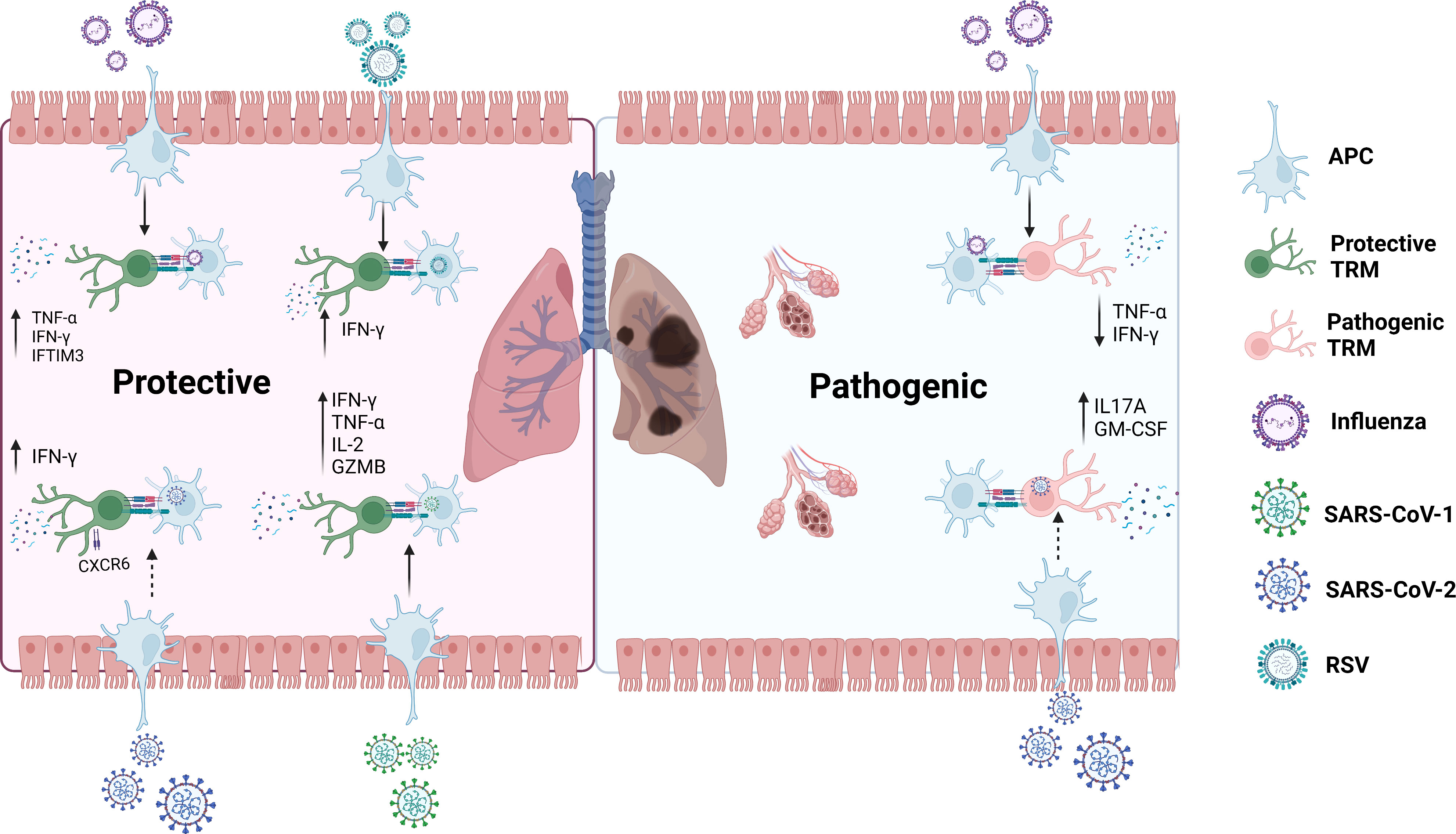
Figure 4 Roles of TRM cells in RSV, Influenza, SARS-CoV-1 and SARS-CoV-2.In the secondary infection, TRM cells rapidly react to protect the host from Influenza, RSV and SARS-CoV-1 through secreting important molecules such as IFN-γ, TNF-α, and IL-2. It may also have the same effect in SARS-CoV-2. On the other side, TRM cells could also arouse pathogenic effects when the host is infected with Influenza, which might also happen in SARS-CoV-2 infection.GZMB: granzyme B. GM-CSF: granulocyte-macrophage colony-stimulating factor. dotted line: possible impact.
Influenza
Influenza has caused several tragic events in the first half of the twentieth century, killing an estimated 50 million people in the 18 months after the end of World War I (74). It can present diverse conditions, ranging from asymptomatic infections and various respiratory syndromes to fulminant primary viral pneumonia and secondary bacterial pneumonia, damaging all organs. Pulmonary CD8+ TRM cells reveal unique traits during influenza infection. CD8+ TRM cells are recruited through chemokine (C-X-C motif) receptor 6/chemokine (C-X-C motif) ligand 16 CXCR6/CXCL16 from the lungs to the airway to realize ectopic denfence (17). Interestingly, pulmonary virus-induced CD8+ TRM cells can retrogradly migrate from the lungs to medLNs, providing long-term regional memory and recording previous antigen experience to provide protection during secondary virus attack (75).
In the medLNs, accumulation of conventional dendritic cells strengthens T cell initiation during the primary infection and the protective heterosubtypic immunity responses of the CD8+ TRM cells (76). Pulmonary TRM cells deposit bronchial-associated lymphoid tissue in the lung parenchyma and provide cross-immune protection against different influenza virus strains (25). Paik et al. (76) demonstrated that mice infected with H3N2 for 3 subsequent weeks could defend against H1N1. These mice displayed decreased virus load and relieved weight loss compared with previously uninfected heterologous mice, suggesting the protective effect of CD8+ TRM cells on influenza. Moreover, the use of FTY720 did not impair the heterologous protection, indicating that the protection is independent of the peripheral circulation and may be mediated by secreting TNF-α and IFN-γ (77). CD4+ TRM cells also mediate remarkable protection. After influenza infection, CD4+ TRM cells isolated from the lungs of mice have been shown to mediate enhanced viral clearance and survival during fatal influenza infections (34, 78).
However, the viability of TRM cells in human requires further investigation. Wu et al. (76) found that heterotypic immunity to influenza declined within six to seven months after the initial infection. Nguyende et al. discovered that CD8+ TRM cells were the most susceptible to age among all subsets of T cells and age was inversely correlated with CD8+ TRM cells in adults (14). This may also explain why older adults are more likely to develop severe clinical symptoms after contracting influenza. In essense, the unstability and waning of CD8+ TRM cells is primarly due to apoptosis (79). Nevertheless, the decay may be rescued by repeated antigen exposure. Van et al. clarified that repeated exposure to influenza enhanced the persistence of lung CD8+ TRM cells and prolonged the durability of heterologous (80).
Despite these factors, some research has introduced new regulatory mechanisms for CD8+ TRM cell formation in influenza. In mouse models, TRM cells selectively maintain the interferon-induced transmembrane protein IFITM3, a protein with extensive resistance to viral infection. Expression of IFITM3 in CD8+ TRM cells enhances resistance to influenza reinfection and increases CD8+ TRM cell maintenance (24). Furthermore, secretion of IFN-γ and restriction of T-bet expression are required for CD8+ TRM cell expression, which is accomplished by CD4+ T cell (29).
In conclusion, TRM cells can migrate to localized areas and provide long-term regional memory to defend against influenza (Figure 4). However, their durability is limited and related to age. Their decline is mainly due to self-apoptosis. Despite IFN-γ secretion by CD4+ T cells to upregulate CD8+ TRM cells, CD4+ TRM cells can reversely contribute to vigorous protection of CD8+ T cells (35). Notably, Goplen et al. (81) disclosed their pathogenic role in aged mice, contradicting the previous recognition of CD8+ TRM cells. Aged mice infected with H1N1 displayed more influenza-specific TRM cells than young mice, accompanied by extremely weakened heterologous immunity, enhanced pulmonary inflammation, and lung fibrosis. These CD8+ TRM cells were unable to secrete IFN-γ and TNF-α. After exhausting CD8+ TRM cells in the lungs, the inflammation and fibrosis were relieved. This unexpected discovery hints to more possibile roles of TRM cells in pulmonary Influenza infection.
SARS-CoV-2
Since the outbreak of COVID-19 in 2019, SARS-CoV-2 specific and vaccine-induced T-cell immune protection has become a popular topic of discussion. A previous study showed that SARS-COV-1-specific CD8+ TRM cells can last six years after infection, while memory B cells and viral antibodies cannot be detected in patients recovering from SARS. In addition to reducing viral load, CD8+ TRM cells effectively produce a variety of effector cytokines, including IFN-γ, TNF-α, and IL-2, and cytolysate molecules like granzyme B to provide protection (82).
However, we cannot simply regard TRM cells as defence against SARS-CoV-2. SARS-CoV-2-specific CD8+ TRM cells were found in the oropharyngeal lymphoid tissue of children and adults unexposed to COVID-19, which were functionally weaker than EBV-specific CD8+ TRM cells, possibly spearheading an early immune response. Niessl et al. pointed that preexisting CD8+ TRM cells may induce heterologous immune responses against COVID-19 (83). The protective role of lung TRM cells against SARS-CoV-2 has also been observed by a three-dimensional perfusion model of human lung tissue (84, 85). Concerning the paired airway and blood samples, Szabo et al. observed that in patients previously infected with COVID-19, CD4+ and CD8+ TRM cells have superiority in the lungs, dominating the airways. Airway T cells expressed upregulation of TRM cell-related gene markers such as CXCR6. More importantly, it upregulated the expression of key cytokines and chemokines, such as IFN-γ, suggesting that TRM cells may protect patients with severe COVID-19 (86). These TRM cells produce IFN-γ in response to in vitro stimulation that persist for at least 10 months in the lungs of patients in the covalescent period, highlighting the persistence of TRM cells-related immunity (87). Dai et al. exhibited that CD8+ TRM cells decreased by 2.4 times in patients with severe infection compared to moderate infection with lower expression of CXCR6, which may protect the lungs in previous experiments (88). Moreover, CD8+ TRM cells undergo active expansion in patiens with mild infection, while they perform more naïve funtions in severely infected patients (89). In patients with moderate infection, CD8+ TRM cells alleviate inflammation through CXCR6-mediated virus clearance, while the expression of CD8+ TRM cells is unstable and reduced in patients with severe infection, leading to viral replication (88) (Figure 4). Furthermore, in contrast to influenza, interferon response against SARS-CoV-2 induced by CD8+ TRM cells does not decline with age (14) and nasal CD8+ TRM cells can last at least two months after virus clearance (31), indicating the persistence of TRM cells against SARS-CoV-2.
However, other studies have challenged the protective effect of CD8+ TRM cells during SARS-CoV-2 infection. Roberts et al. (32) elucidated that though lung-resident T cells can be induced by SARS-CoV-2, they cannot provide adequate protection against secondary viral infection. In mice model, transferring T cells from SARS-CoV-2 infected mice to uninfected mice did not improve survival after reinfection. This prompts the unknown efficiency of TRM cells in protecting patients against SARS-CoV-2 during secondary infection. Additionally, many patients in the convalescent stage of COVID-19 infection still experienced respiratory symptoms for months. Vijayakumar et al. found that compared to healthy individuals, T cell frequencies in these patients were increased, particularly CD8+ TRM cells by immuno-proteomic profiling. The heightened number of CD8+ TRM cells was correlated with increased cell death and indicated persistent airway symptoms, namely decreased forced vital capacity (90).
This is evidence that TRM cells predominate during COVID-19 infection. Although some observations of human samples emphasized the possible protective correlation between TRM cells and SARS-CoV-2, studies in mice show that the protection is insufficient. Moreover, exploration of the TRM cells of patients with severe infection and convalescence with respiratory symptoms showed association with ongoing lung injury. Roles of TRM cells in SARS-CoV-2 are complicated. TRM cells at different disease stages and distinct populations may display different effects, being potential pathogenic or protective orchestrators in COVID-19. Furthermore, CD8+ TRM cells regulate CD4+ T cells to influence immune responses during SARS-CoV-2 infection. Kaneko et al. showed that decline of Bcl-6+ T follicular helper cells was responsible for the loss of germinal centers and accumulation of activated B cells from non-germinal sources, triggering low efficiency and unsustainable humor immune responses during acute and severe SARS-CoV-2 infection (91). Transcription factor Bcl-6 is indispensable for the development of T follicular helper cells and mutually antagonizes Blimp1 in the process (92, 93). As mentioned above, formation and maintenance of CD8+ TRM cells in the lungs requires Blimp1. We assume that by upregulation of Blimp1, CD8+ TRM cells inhibit Bcl-6 to impede the generation of T follicular helper cells, affecting humoral immunity in severe COVID-19. More studies are needed to verify this hypothesis and patients with different SARS-CoV-2 infection conditions should also be considered. An update on recognition of long SARS-CoV-2 T cell immunity is needed for more research on the absolute roles of TRM cells in SARS-CoV-2.
Application in preventive and therapeutic strategies
Till date, there are no drugs that efficiently cure the abovementioned respiratory viruses. Thousands of researchers work on the research and development of treatment. Although B cells eliminate the virus faster in the form of neutralizing antibodies, T cell immunity-related theraputic strategies can maintain long-term protection, and TRM cells largely contribute to immunity in situ.
The study and exploration of TRM cells highlights that the site of vaccine injection is critical, and nasal mucosal vaccination may become the trend to curb the replication of respiratory viruses. TRM cells are required for vaccine-induced influenza associated T cell immunity (94). Morabito et al.(95) investigated a murine cytomegalovirus vector vaccine expressing RSV M-protein. Compared with intraperitoneal injection, intranasal inoculation triggered a large amount of CD8+ TRM cells, mediating early viral clearance over time. A similar result was observed during influenza vaccine research (96). Mucosal immunity in the respiratory tract also encourages the application of inhaled vaccine. Aerosol inhalation of two doses of Ad5-nCoV, an aerosolised adenovirus type-5 vector-based COVID-19 vaccine, induced production of benign neutralization antibodies with well tolerance and consumed less vaccine dosage than intramuscular injection (97). Inhalation vaccine can both improve compliance to the medication and reduce the cost of vaccination. Enhancement of local mucosal immunity prevents infection and blocks tramsmission at the point of virus invasion, making it safer and more convenient for promotion in population.
TRM cells mediate superior protection in heterotypic infection, highlighting their potential as a universal vaccine target. For production of large amounts of TRM cells, Bosnjak et al. utilized a prime-boost protocol on a novel modified vaccinia virus Ankara (MVA)-SARS-2-spike vaccine candidate to induce immune responses, which alleviated weight loss, increased clinical score, and decreased viral titers in rodents (98). Furthermore, Lei et al. developed a recombinant RBD vaccine against variants of concern for intranasal administration, which not only induced and maintained high IgG levels, but also enhanced mucosal immunity, including lung TRM cells (99). In particular, humoral immunity and TRM cells attained by intranasally delivered SARS-CoV−2 DNA vaccine efficiently suppresses the wild type and Beta variant, providing persistent protection (100). Remarkably, in the absence or with low expression of virus-neutralizing antibody, systemic or pulmonary CD4+ TRM cells and protective CD8 T cells defended effectively against the Beta variant, without lung immunopathology (101). Vaccines targeted at TRM cells are promising for successful prevention of reinfection.
Various combination adjuvants may also improve efficiency by stimulating or maintaining TRM cells. As mentioned above, conventional dendritic cells enhance the protection induced by TRM cells. Wakim et al. developed an antibody targeted inoculation method to present the antigen only to respiratory dendritic cells, facilitating the generation of protective pulmonary TRM cells against influenza (102).
Several TRM cells stimulators, such as IL-1β and zymosan, were used in conjugation with with vaccine, which proved to be more effective in influenza mice models. IL-1β was used as an adjuvant in recombinant adenovirus vector encoding hemagglutinin and nuclear protein in mice. Abundant TRM cells were accumulated and weight loss and virus copies in mice were mitigated. IL-1β and local antigen resulted in activation of key checkpoints in TRM cell formation, including epithelial cell activation, expression of chemokines and adhesion molecules, and recruitment of lung-derived CD103+ DCs (103). Without the antigen, only the adjuvant usually cannot drive the generation of TRM cells. Notably, zymosan promoted the differentiation from effector T cells to TRM cells passing by antigen. When used in combination with injectable influenza vaccine, intranasal zymosan significantly increased influenza-specific pulmonary TRM cells (104).
However, there are some complications with treatments based on TRM cells. Firstly, the existence of TRM cells is not stable enough to maintain permanent protection. Using an adenovirus expressing influenza nucleoprotein, Uddback et al. demonstrated that CD8+ TRM cells in the lungs could sustain for at least a year post vaccination (105). Other than apoptosis, retrocedent migration into medLNs may also explain why TRM cells decrease overtime as described previously, which hinders protection in the lungs. Apparently, the existence of TRM cells is dynamic. Maintenance of protective TRM cells in situ is worth exploring. Secondly, the balance between protective and pathogenic roles of TRM cells is unknown. The switching point at which TRM cells change from protective to pathogenic is unknown, especially in the elderly.
TRM cells have supreme value in the treatment of respiratory viruses. In addition to vaccines, new drugs targeted at TRM cells have also been released. Pretreated FC-fused IL-7 protects mice from fatal influenza infection depending on tissue-resident memory-like T cells in the lungs, and lasts for several weeks (106). Utilizing the unique advantages of TRM cells, such as persistence in tissues and remarkable ability to undertake heterotypic immunity, a portable, and highly protective vaccine inducing TRM cells against multiple pathogens can be developed in the future. Moreover, devotion to clinical administration is a big step for vaccines and drugs based on TRM cells.
Concluding remarks
TRM cells are persistent in non-lymphoid tissues and act as a sentinel in reinfection immunity, responding to infection more quickly. A variety of signaling pathways regulate the generation of TRM cells. However, little is known about the regulation of CD4+ TRM cells. Regarding the traits, TRM cells are usually limited in situ and migrate localizedly in traditional opinion. As stated above, CD8+ TRM cells move to the airway and medLNs to induce effective protection, breaking the stereotype. Nevertheless, TRM cells perform effector-like functions in many tissues and maintain their state without antigen to provide powerful protection (107, 108). Therefore, they are often regarded as terminal differentiation of effector cells, which is actually a semblance. TRM cells expand in the skin and mucosa on contact with the antigen (109, 110), which is quite different from terminally differentiated effector memory (TEMRA) cells. TEMRA cells also reside in situ, providing steady protection during SARS-CoV-2 infection (111), while TRM cells display more plasticity and instability. A recent study showed that reactivational TRM cells in intestine rejoin the lymphoid circulation and have the potential to differentiate into TCM and TEM cells (112, 113). While the phenomenon has not been observed in the lungs, experiments aimed to reconfirm the unstability of TRM cells are still ongoing.
Specifically, TRM cells have different populations. For instance, CD4+ TRM cells have distinct subsets, including Th1, Th2, and Th17 TRM cells (114). Influenza-specific CD4+ TRM cells characterize as Th1-like TRM cells secreting IFN-γ and IL-2 and contribute to enhanced protection against influenza (15, 34, 115). Th1 TRM cells provide protection against influenza reinfection and influenza matrix protein ectodomain (M2e)-specific Th17 TRM cells are stimulated through intranasal immunization with M2e adjuvanted with CTA1-DD, generating strong protection against influenza (15, 116). Conversely, in the BAL collected from patients with severe COVID-19, Th17 TRM cells persisted even after virus clearance. These Th17 TRM cells expressing IL-17A and granulocyte-macrophage colony-stimulating factor are potentially pathogenic cytokines and can interact with lung macrophages and cytotoxic CD8+ T cells, influencing lung injury (117). Little is known about Th2 TRM cells in respiratory virus infection. Th2 CD4+ TRM cells in the peritoneum mediated protective immunity against helminths in mouse intestinal Heligmosomoides polygyrus infection (118). A key research question that needs to be addressed is whether distinct TRM subsets play different roles in reinfection of the same pathogen.
TRM cells exhibit consolidated protection against RSV and influenza, and probable effects on SARS-CoV-2. Consistently, therapeutic strategies targeting TRM cells are good and promising choices to cope with respiratory viruses. This illustrates the bright future of vaccines based on TRM cells in response to respiratory virus. Considering their diverse roles, we may adopt antagonism when TRM cells turn pathogenic. For instance, employing CpG, an agonist of Toll-like receptor, and Il685458, a Notch pathway inhibitor, can ease the airway inflammation caused by formalin-inactivated vaccine, which once failed in prevention of exacerbation of lung disease (119, 120). We believe that insightful application of TRM cells is key to protective mucosal immunity generated by future universal vaccine candidates.
Author contributions
MZ and ZJ contributed to the central idea and coordinated the writing of the manuscript. NL, YH, TS, and ZJ read, discussed, and revised the manuscript. All authors contributed to the article and approved the submitted version.
Funding
This study was supported by National Natural Science Foundation of China (NSFC) programs (No. 82070024), Natural Science Foundation of Shanghai (No. 20ZR1443700), Specialized Department Foundation of Minhang District (No: 2020MWTZB02), Plan for leading talent of Minghang District (201807) and Fund from Shanghai Fifth People’s Hospital (2019WYZD03).
Acknowledgments
The figures in this review were created with Biorender.com.
Conflict of interest
The authors declare that the research was conducted in the absence of any commercial or financial relationships that could be construed as a potential conflict of interest.
Publisher’s note
All claims expressed in this article are solely those of the authors and do not necessarily represent those of their affiliated organizations, or those of the publisher, the editors and the reviewers. Any product that may be evaluated in this article, or claim that may be made by its manufacturer, is not guaranteed or endorsed by the publisher.
Abbreviations
Tissue-resident memory T (TRM) cells; central memory T (TCM) cells; effector memory T (TEM) cells; dendritic cells (DCs); mediastinal lymph nodes (medLNs); interleukin (IL); Krupple-Like Factor 2 (KLF2); sphingosine-1-phosphate receptor (S1PR1); eomesodermin (Eomes); transcription factor 1(TCF1); Respiratory syncytial virus (RSV); bronchoalveolar lavage (BAL).
References
1. Sallusto F, Lenig D, Förster R, Lipp M, Lanzavecchia A. Two subsets of memory T lymphocytes with distinct homing potentials and effector functions. Nature (1999) 14:708–12. doi: 10.1038/44385.
2. Mueller SN, Gebhardt T, Carbone FR, Heath WR. Memory T cell subsets, migration patterns, and tissue residence. Annu Rev Immunol (2013) 31(1):137–61. doi: 10.1146/annurev-immunol-032712-095954
3. Raphael I, Joern RR, Forsthuber TG. Memory Cd4(+) T cells in immunity and autoimmune diseases. Cells (2020) 9(3):531. doi: 10.3390/cells9030531
4. Jiang X, Clark RA, Liu L, Wagers AJ, Fuhlbrigge RC, Kupper TS. Skin infection generates non-migratory memory Cd8+ T(Rm) cells providing global skin immunity. Nature (2012) 483(7388):227–31. doi: 10.1038/nature10851
5. Gebhardt T, Wakim LM, Eidsmo L, Reading PC, Heath WR, Carbone FR. Memory T cells in nonlymphoid tissue that provide enhanced local immunity during infection with herpes simplex virus. Nat Immunol (2009) 10(5):524–30. doi: 10.1038/ni.1718
6. Gebhardt T, Mueller SN, Heath WR, Carbone FR. Peripheral tissue surveillance and residency by memory T cells. Trends Immunol (2013) 34(1):27–32. doi: 10.1016/j.it.2012.08.008
7. Forster R, Schubel A, Breitfeld D, Kremmer E, Renner-Muller I, Wolf E, et al. Ccr7 coordinates the primary immune response by establishing functional microenvironments in secondary lymphoid organs. Cell (1999) 99:23–33. doi: 10.1016/s0092-8674(00)80059-8
8. Masopust D, Soerens AG. Tissue-resident T cells and other resident leukocytes. Annu Rev Immunol (2019) 37:521–46. doi: 10.1146/annurev-immunol-042617-053214
9. Ariotti S, Hogenbirk MA, Dijkgraaf FE, Visser LL, Hoekstra ME, Song J-Y, et al. Skin-resident memory Cd8+ T cells trigger a state of tissue-wide pathogen alert. Science (2014) 346:101–5. doi: 10.1126/science.1254803
10. Schenkel JM, Pauken E, Vezys V, Fraser KA, Masopust D, Beura LK. Resident memory Cd8 T cells trigger protective innate and adaptive immune responses. Science (2014) 346:98–101. doi: 10.1126/science.1254536
11. Iborra S, Martinez-Lopez M, Khouili SC, Enamorado M, Cueto FJ, Conde-Garrosa R, et al. Optimal generation of tissue-resident but not circulating memory T cells during viral infection requires crosspriming by dngr-1(+) dendritic cells. Immunity (2016) 45(4):847–60. doi: 10.1016/j.immuni.2016.08.019
12. Jozwik A, Habibi MS, Paras A, Zhu J, Guvenel A, Dhariwal J, et al. Rsv-specific airway resident memory Cd8+ T cells and differential disease severity after experimental human infection. Nat Commun (2015) 6:10224. doi: 10.1038/ncomms10224
13. Kinnear E, Lambert L, McDonald JU, Cheeseman HM, Caproni LJ, Tregoning JS. Airway T cells protect against rsv infection in the absence of antibody. Mucosal Immunol (2018) 11(1):249–56. doi: 10.1038/mi.2017.46
14. Nguyen TH, McAuley JL, Kim Y, Zheng MZ, Gherardin NA, Godfrey DI, et al. Influenza, but not sars-Cov-2, infection induces a rapid interferon response that wanes with age and diminished tissue-resident memory Cd8(+) T cells. Clin Transl Immunol (2021) 10(1):e1242. doi: 10.1002/cti2.1242
15. Turner DL, Bickham KL, Thome JJ, Kim CY, D'Ovidio F, Wherry EJ, et al. Lung niches for the generation and maintenance of tissue-resident memory T cells. Mucosal Immunol (2014) 7(3):501–10. doi: 10.1038/mi.2013.67
16. Wang Z, Wang S, Goplen NP, Li C, Cheon IS, Dai Q, et al. Pd-1 Hi Cd8+ resident memory T cells balance immunity and fibrotic sequelae. Sci Immunol (2019) 4(36):eaaw1217. doi: 10.1126/sciimmunol.aaw1217
17. Wein AN, McMaster SR, Takamura S, Dunbar PR, Cartwright EK, Hayward SL, et al. Cxcr6 regulates localization of tissue-resident memory Cd8 T cells to the airways. J Exp Med (2019) 216(12):2748–62. doi: 10.1084/jem.20181308
18. de Bree GJ, van Leeuwen EM, Out TA, Jansen HM, Jonkers RE, van Lier RA. Selective accumulation of differentiated Cd8+ T cells specific for respiratory viruses in the human lung. J Exp Med (2005) 202(10):1433–42. doi: 10.1084/jem.20051365
19. Piet B, de Bree GJ, Smids-Dierdorp BS, van der Loos CM, Remmerswaal EB, von der Thusen JH, et al. Cd8(+) T cells with an intraepithelial phenotype upregulate cytotoxic function upon influenza infection in human lung. J Clin Invest (2011) 121(6):2254–63. doi: 10.1172/JCI44675
20. Snyder ME, Finlayson MO, Connors TJ, Dogra P, Senda T, Bush E, et al. Generation and persistence of human tissue-resident memory T cells in lung transplantation. Sci Immunol (2019) 4(33):eaav5581. doi: 10.1126/sciimmunol.aav5581
21. Snyder ME, Sembrat J, Noda K, Myerburg MM, Craig A, Mitash N, et al. Human lung-resident macrophages colocalize with and provide costimulation to Pd1 Hi tissue-resident memory T cells. Am J Respir Crit Care Med (2021) 203:1230–44. doi: 10.1164/rccm.202006-2403OC
22. Pizzolla A, Nguyen THO, Smith JM, Brooks AG, Kedzieska K, Heath WR, et al. Resident memory Cd8 T cells in the upper respiratory tract prevent pulmonary influenza virus infection. Sci Immunol (2017) 2(12):eaam6970. doi: 10.1126/sciimmunol.aam6970
23. Hayward SL, Scharer CD, Cartwright EK, Takamura S, Li ZT, Boss JM, et al. Environmental cues regulate epigenetic reprogramming of airway-resident memory Cd8(+) T cells. Nat Immunol (2020) 21(3):309–20. doi: 10.1038/s41590-019-0584-x
24. Wakim LM, Gupta N, Mintern JD, Villadangos JA. Enhanced survival of lung tissue-resident memory Cd8(+) T cells during infection with influenza virus due to selective expression of Ifitm3. Nat Immunol (2013) 14(3):238–45. doi: 10.1038/ni.2525
25. Wu T, Hu Y, Lee YT, Bouchard KR, Benechet A, Khanna K, et al. Lung-resident memory Cd8 T cells (Trm) are indispensable for optimal cross-protection against pulmonary virus infection. J Leukocyte Biol (2014) 95(2):215–24. doi: 10.1189/jlb.0313180
26. Guvenel A, Jozwik A, Ascough S, Ung SK, Paterson S, Kalyan M, et al. Epitope-specific airway-resident Cd4+ T cell dynamics during experimental human rsv infection. J Clin Invest (2020) 130(1):523–38. doi: 10.1172/JCI131696
27. Lee YT, Suarez-Ramirez JE, Wu T, Redman JM, Bouchard K, Hadley GA, et al. Environmental and antigen receptor-derived signals support sustained surveillance of the lungs by pathogen-specific cytotoxic T lymphocytes. J Virol (2011) 85(9):4085–94. doi: 10.1128/JVI.02493-10
28. McMaster SR, Wein AN, Dunbar PR, Hayward SL, Cartwright EK, Denning TL, et al. Pulmonary antigen encounter regulates the establishment of tissue-resident Cd8 memory T cells in the lung airways and parenchyma. Mucosal Immunol (2018) 11(4):1071–8. doi: 10.1038/s41385-018-0003-x
29. Laidlaw BJ, Zhang N, Marshall HD, Staron MM, Guan T, Hu Y, et al. Cd4+ T cell help guides formation of Cd103+ lung-resident memory Cd8+ T cells during influenza viral infection. Immunity (2014) 41(4):633–45. doi: 10.1016/j.immuni.2014.09.007
30. Goplen NP, Cheon IS, Sun J. Age-related dynamics of lung-resident memory Cd8(+) T cells in the age of covid-19. Front Immunol (2021) 12:636118. doi: 10.3389/fimmu.2021.636118
31. Roukens AHE, Pothast CR, Konig M, Huisman W, Dalebout T, Tak T, et al. Prolonged activation of nasal immune cell populations and development of tissue-resident sars-Cov-2-Specific Cd8(+) T cell responses following covid-19. Nat Immunol (2022) 23(1):23–32. doi: 10.1038/s41590-021-01095-w
32. Roberts LM, Jessop F, Wehrly TD, Bosio CM. Cutting edge: Lung-resident T cells elicited by sars-Cov-2 do not mediate protection against secondary infection. J Immunol (2021) 207(10):2399–404. doi: 10.4049/jimmunol.2100608
33. Luangrath MA, Schmidt ME, Hartwig SM, Varga SM. Tissue-resident memory T cells in the lungs protect against acute respiratory syncytial virus infection. Immunohorizons (2021) 5(2):59–69. doi: 10.4049/immunohorizons.2000067
34. Teijaro JR, Turner D, Pham Q, Wherry EJ, Lefrancois L, Farber DL. Cutting edge: Tissue-retentive lung memory Cd4 T cells mediate optimal protection to respiratory virus infection. J Immunol (2011) 187(11):5510–4. doi: 10.4049/jimmunol.1102243
35. Son YM, Cheon IS, Wu Y, Li C, Wang Z, Gao X, et al. Tissue-resident Cd4 T helper cells assist the development of protective respiratory b and Cd8 T cell memory responses. Sci Immunol (2021) 6(55):eabb6852. doi: 10.1126/sciimmunol.abb6852
36. Pizzolla A, Nguyen THO, Smith JM, Brooks AG, Kedzieska K, Heath WR, et al. Resident memory Cd8 + T cells in the upper respiratory tract prevent pulmonary influenza virus infection. Sci Immunol (2017) 2(12):eaam6970. doi: 10.1126/sciimmunol.aam6970
37. Travis MA, Sheppard D. Tgf-beta activation and function in immunity. Annu Rev Immunol (2014) 32:51–82. doi: 10.1146/annurev-immunol-032713-120257
38. Yu CI, Becker C, Wang Y, Marches F, Helft J, Leboeuf M, et al. Human Cd1c+ dendritic cells drive the differentiation of Cd103+ Cd8+ mucosal effector T cells Via the cytokine tgf-beta. Immunity (2013) 38(4):818–30. doi: 10.1016/j.immuni.2013.03.004
39. Skon CN, Lee JY, Anderson KG, Masopust D, Hogquist KA, Jameson SC. Transcriptional downregulation of S1pr1 is required for the establishment of resident memory Cd8+ T cells. Nat Immunol (2013) 14(12):1285–93. doi: 10.1038/ni.2745
40. Mackay LK, Wynne-Jones E, Freestone D, Pellicci DG, Mielke LA, Newman DM, et al. T-Box transcription factors combine with the cytokines tgf-beta and il-15 to control tissue-resident memory T cell fate. Immunity (2015) 43(6):1101–11. doi: 10.1016/j.immuni.2015.11.008
41. Wu J, Madi A, Mieg A, Hotz-Wagenblatt A, Weisshaar N, Ma S, et al. T Cell factor 1 suppresses Cd103+ lung tissue-resident memory T cell development. Cell Rep (2020) 31(1):107484. doi: 10.1016/j.celrep.2020.03.048
42. Hombrink P, Helbig C, Backer RA, Piet B, Oja AE, Stark R, et al. Programs for the persistence, vigilance and control of human Cd8(+) lung-resident memory T cells. Nat Immunol (2016) 17(12):1467–78. doi: 10.1038/ni.3589
43. Zens KD, Chen JK, Guyer RS, Wu FL, Cvetkovski F, Miron M, et al. Reduced generation of lung tissue-resident memory T cells during infancy. J Exp Med (2017) 214(10):2915–32. doi: 10.1084/jem.20170521
44. Ito Y, Miyazono K. Runx transcription factors as key targets of tgf-b superfamily signaling. Curr Opin Genet Dev (2003) 13(1):43–7. doi: 10.1016/s0959-437x(03)00007-8
45. Yang Q, Li G, Zhu Y, Liu L, Chen E, Turnquist H, et al. Il-33 synergizes with tcr and il-12 signaling to promote the effector function of Cd8+ T cells. Eur J Immunol (2011) 41(11):3351–60. doi: 10.1002/eji.201141629
46. Goplen NP, Huang S, Zhu B, Cheon IS, Son YM, Wang Z, et al. Tissue-resident macrophages limit pulmonary Cd8 resident memory T cell establishment. Front Immunol (2019) 10:2332. doi: 10.3389/fimmu.2019.02332
47. Ferreira C, Barros L, Baptista M, Blankenhaus B, Barros A, Figueiredo-Campos P, et al. Type 1 treg cells promote the generation of Cd8(+) tissue-resident memory T cells. Nat Immunol (2020) 21(7):766–76. doi: 10.1038/s41590-020-0674-9
48. Takamura S, Kohlmeier JE. Establishment and maintenance of conventional and circulation-driven lung-resident memory Cd8(+) T cells following respiratory virus infections. Front Immunol (2019) 10:733. doi: 10.3389/fimmu.2019.00733
49. Behr FM, Kragten NAM, Wesselink TH, Nota B, van Lier RAW, Amsen D, et al. Blimp-1 rather than hobit drives the formation of tissue-resident memory Cd8(+) T cells in the lungs. Front Immunol (2019) 10:400. doi: 10.3389/fimmu.2019.00400
50. Salehi S, Bankoti R, Benevides L, Willen J, Couse M, Silva JS, et al. B lymphocyte-induced maturation protein-1 contributes to intestinal mucosa homeostasis by limiting the number of il-17-Producing Cd4+ T cells. J Immunol (2012) 189(12):5682–93. doi: 10.4049/jimmunol.1201966
51. Li C, Zhu B, Son YM, Wang Z, Jiang L, Xiang M, et al. The transcription factor Bhlhe40 programs mitochondrial regulation of resident Cd8(+) T cell fitness and functionality. Immunity (2019) 51(3):491–507. doi: 10.1016/j.immuni.2019.08.013
52. Strutt TM, Dhume K, Finn CM, Hwang JH, Castonguay C, Swain SL, et al. Il-15 supports the generation of protective lung-resident memory Cd4 T cells. Mucosal Immunol (2018) 11(3):668–80. doi: 10.1038/mi.2017.101
53. Cyster JG, Schwab SR. Sphingosine-1-Phosphate and lymphocyte egress from lymphoid organs. Annu Rev Immunol (2012) 30:69–94. doi: 10.1146/annurev-immunol-020711-075011
54. Hondowicz BD, An D, Schenkel JM, Kim KS, Steach HR, Krishnamurty AT, et al. Interleukin-2-Dependent allergen-specific tissue-resident memory cells drive asthma. Immunity (2016) 44(1):155–66. doi: 10.1016/j.immuni.2015.11.004
55. Dhume K, Finn CM, Strutt TM, Sell S, McKinstry KK. T-Bet optimizes Cd4 T-cell responses against influenza through Cxcr3-dependent lung trafficking but not functional programming. Mucosal Immunol (2019) 12(5):1220–30. doi: 10.1038/s41385-019-0183-z
56. Oja AE, Piet B, Helbig C, Stark R, van der Zwan D, Blaauwgeers H, et al. Trigger-happy resident memory Cd4(+) T cells inhabit the human lungs. Mucosal Immunol (2018) 11(3):654–67. doi: 10.1038/mi.2017.94
57. Milner JJ, Toma C, Yu B, Zhang K, Omilusik K, Phan AT, et al. Runx3 programs Cd8(+) T cell residency in non-lymphoid tissues and tumours. Nature (2017) 552(7684):253–7. doi: 10.1038/nature24993
58. Mandala S, Hajdu R, Bergstrom J, Quackenbush E, Xie J, Milligan J, et al. Alteration of lymphocyte trafficking by sphingosine-1-Phosphate receptor agonists. Sci (New York NY) (2002) 296(5566):346–9. doi: 10.1126/science.1070238
59. Shiow LR, Rosen DB, Brdickova N, Xu Y, An J, Lanier LL, et al. Cd69 acts downstream of interferon-Alpha/Beta to inhibit S1p1 and lymphocyte egress from lymphoid organs. Nature (2006) 440(7083):540–4. doi: 10.1038/nature04606
60. Yeon SM, Halim L, Chandele A, Perry CJ, Kim SH, Kim SU, et al. Il-7 plays a critical role for the homeostasis of allergen-specific memory Cd4 T cells in the lung and airways. Sci Rep (2017) 7(1):11155. doi: 10.1038/s41598-017-11492-7
61. Takamura S. Niches for the long-term maintenance of tissue-resident memory T cells. Front Immunol (2018) 9:1214. doi: 10.3389/fimmu.2018.01214
62. Stary G, Olive A, Radovic-Moreno AF, Gondek D, Alvarez D, Basto PA, et al. Vaccines. a mucosal vaccine against chlamydia trachomatis generates two waves of protective memory T cells. Science (2015) 348(6241):aaa8205. doi: 10.1126/science.aaa8205
63. Byrne A, Savas P, Sant S, Li R, Virassamy B, Luen SJ, et al. Tissue-resident memory T cells in breast cancer control and immunotherapy responses. Nat Rev Clin Oncol (2020) 17(6):341–8. doi: 10.1038/s41571-020-0333-y
64. Overstreet MG, Gaylo A, Angermann BR, Hughson A, Hyun YM, Lambert K, et al. Inflammation-induced interstitial migration of effector Cd4(+) T cells is dependent on integrin alphav. Nat Immunol (2013) 14(9):949–58. doi: 10.1038/ni.2682
65. Reilly EC, Lambert Emo K, Buckley PM, Reilly NS, Smith I, Chaves FA, et al. Trm integrins Cd103 and Cd49a differentially support adherence and motility after resolution of influenza virus infection. Proc Natl Acad Sci U S A (2020) 117(22):12306–14. doi: 10.1073/pnas.1915681117
66. Mueller SN, Mackay LK. Tissue-resident memory T cells: Local specialists in immune defence. Nat Rev Immunol (2016) 16(2):79–89. doi: 10.1038/nri.2015.3
67. Kim S-K, Reed DS, Olson S, Schnell MJ, Rose JK, Morton PA, et al. Generation of mucosal cytotoxic T cells against soluble protein by tissue-specific environmental and costimulatory signals. Proc Natl Acad Sci U S A (1998) 95:10814–9. doi: 10.1073/pnas.95.18.10814
68. Masopust D, Vezys V, Wherry EJ, Barber DL, Ahmed R. Cutting edge: Gut microenvironment promotes differentiation of a unique memory Cd8 T cell population. J Immunol (2006) 176(4):2079–83. doi: 10.4049/jimmunol.176.4.2079
69. Muller AJ, Filipe-Santos O, Eberl G, Aebischer T, Spath GF, Bousso P. Cd4+ T cells rely on a cytokine gradient to control intracellular pathogens beyond sites of antigen presentation. Immunity (2012) 37(1):147–57. doi: 10.1016/j.immuni.2012.05.015
70. Morris JA, Blount RE Jr., Savage RE. Recovery of cytopathogenic agent from chimpanzees with coryza. Proc Soc Exp Biol Med (1956) 92:544–9. doi: 10.3181/00379727-92-22538
71. Nair H, Nokes DJ, Gessner BD, Dherani M, Madhi SA, Singleton RJ, et al. Global burden of acute lower respiratory infections due to respiratory syncytial virus in young children: A systematic review and meta-analysis. Lancet (2010) 375(9725):1545–55. doi: 10.1016/s0140-6736(10)60206-1
72. Wang X, Li Y, Deloria-Knoll M, Madhi SA, Cohen C, Ali A, et al. Global burden of acute lower respiratory infection associated with human metapneumovirus in children under 5 years in 2018: A systematic review and modelling study. Lancet Global Health (2021) 9(1):e33–43. doi: 10.1016/S2214-109X(20)30393-4
73. Li H, Callahan C, Citron M, Wen Z, Touch S, Monslow MA, et al. Respiratory syncytial virus elicits enriched Cd8+ T lymphocyte responses in lung compared with blood in African green monkeys. PLoS One (2017) 12(11):e0187642. doi: 10.1371/journal.pone.0187642
75. Stolley JM, Johnston TS, Soerens AG, Beura LK, Rosato PC, Joag V, et al. Retrograde migration supplies resident memory T cells to lung-draining ln after influenza infection. J Exp Med (2020) 217(8):e20192197. doi: 10.1084/jem.20192197
76. Paik DH, Farber DL. Influenza infection fortifies local lymph nodes to promote lung-resident heterosubtypic immunity. J Exp Med (2021) 218(1):e20200218. doi: 10.1084/jem.20200218
77. Pizzolla A, Nguyen TH, Sant S, Jaffar J, Loudovaris T, Mannering SI, et al. Influenza-specific lung-resident memory T cells are proliferative and polyfunctional and maintain diverse tcr profiles. J Clin Invest (2018) 128(2):721–33. doi: 10.1172/JCI96957
78. Wilkinson TM, Li CKF, Chui CSC, Huang AKY, Perkins M, Liebner JC, et al. Preexisting influenza-specific CD4+ T cells correlate with disease protection against influenza challenge in humans. Nat Med (2012) 18(2):274–80. doi: 10.1038/nm.2612
79. Slütter B, Van Braeckel-Budimir N, Abboud G, Varga SM, Salek-Ardakani S, Harty JT. Dynamics of influenza-induced lung-resident memory T cells underlie waning heterosubtypic immunity. Sci Immunol (2017) 2(7):eaag2031. doi: 10.1126/sciimmunol.aag2031
80. Van Braeckel-Budimir N, Varga SM, Badovinac VP, Harty JT. Repeated antigen exposure extends the durability of influenza-specific lung-resident memory Cd8(+) T cells and heterosubtypic immunity. Cell Rep (2018) 24(13):3374–82. doi: 10.1016/j.celrep.2018.08.073
81. Goplen NP, Wu Y, Son YM, Li C, Wang Z, Cheon IS, et al. Tissue-resident Cd8(+) T cells drive age-associated chronic lung sequelae after viral pneumonia. Sci Immunol (2020) 5(53):eabc4557. doi: 10.1126/sciimmunol.abc4557
82. Channappanavar R, Fett C, Zhao J, Meyerholz DK, Perlman S. Virus-specific memory Cd8 T cells provide substantial protection from lethal severe acute respiratory syndrome coronavirus infection. J Virol (2014) 88(19):11034–44. doi: 10.1128/JVI.01505-14
83. Niessl J, Sekine T, Lange J, Konya V, Forkel M, Maric J, et al. Identification of resident memory Cd8 T cells with functional specificity for sars-Cov-2 in unexposed oropharyngeal lymphoid tissue. Sci Immunol (2021) 6(64):eabk0894. doi: 10.1126/sciimmunol.abk0894
84. Qian Y, Zhu Y, Li Y, Li B. Legend of the sentinels: Development of lung resident memory T cells and their roles in diseases. Front Immunol (2020) 11:624411. doi: 10.3389/fimmu.2020.624411
85. Goliwas KF, Wood AM, Simmons CS, Khan R, Khan SA, Wang Y, et al. Local sars-Cov-2 peptide-specific immune responses in lungs of convalescent and uninfected human subjects. medRxiv (2022) 2021.09.02.21263042. doi: 10.1101/2021.09.02.21263042
86. Szabo PA, Dogra P, Gray JI, Wells SB, Connors TJ, Weisberg SP, et al. Longitudinal profiling of respiratory and systemic immune responses reveals myeloid cell-driven lung inflammation in severe covid-19. Immunity (2021) 54(4):797–814. doi: 10.1016/j.immuni.2021.03.005
87. Grau-Exposito J, Sanchez-Gaona N, Massana N, Suppi M, Astorga-Gamaza A, Perea D, et al. Peripheral and lung resident memory T cell responses against sars-Cov-2. Nat Commun (2021) 12(1):3010. doi: 10.1038/s41467-021-23333-3
88. Dai Y, Wang J, Jeong HH, Chen W, Jia P, Zhao Z. Association of Cxcr6 with covid-19 severity: Delineating the host genetic factors in transcriptomic regulation. Hum Genet (2021) 140(9):1313–28. doi: 10.1007/s00439-021-02305-z
89. Wauters E, Van Mol P, Garg AD, Jansen S, Van Herck Y, Vanderbeke L, et al. Discriminating mild from critical covid-19 by innate and adaptive immune single-cell profiling of bronchoalveolar lavages. Cell Res (2021) 31(3):272–90. doi: 10.1038/s41422-020-00455-9
90. Vijayakumar B, Boustani K, Ogger PP, Papadaki A, Tonkin J, Orton CM, et al. Immuno-proteomic profiling reveals aberrant immune cell regulation in the airways of individuals with ongoing post-Covid-19 respiratory disease. Immunity (2022) 55(3):542–56.e5. doi: 10.1016/j.immuni.2022.01.017
91. Kaneko N, Kuo HH, Boucau J, Farmer JR, Allard-Chamard H, Mahajan VS, et al. Loss of bcl-6-Expressing T follicular helper cells and germinal centers in covid-19. Cell (2020) 183(1):143–57.e13. doi: 10.1016/j.cell.2020.08.025
92. Nurieva RI, Chung Y, Martinez GJ, Yang XO, Tanaka S, Matskevitch TD, et al. Bcl6 mediates the development of T follicular helper cells. Science (2009) 325(5943):1001–5. doi: 10.1126/science.1176676
93. Johnston RJ, Poholek AC, DiToro D, Yusuf I, Eto D, Barnett B, et al. Bcl6 and blimp-1 are reciprocal and antagonistic regulators of T follicular helper cell differentiation. Science (2009) 325(5943):1006–10. doi: 10.1126/science.1175870
94. Marinaik CB, Kingstad-Bakke B, Lee W, Hatta M, Sonsalla M, Larsen A, et al. Programming multifaceted pulmonary T cell immunity by combination adjuvants. Cell Rep Med (2020) 1(6):100095. doi: 10.1016/j.xcrm.2020.100095
95. Morabito KM, Ruckwardt TJ, Bar-Haim E, Nair D, Moin SM, Redwood AJ, et al. Memory inflation drives tissue-resident memory Cd8(+) T cell maintenance in the lung after intranasal vaccination with murine cytomegalovirus. Front Immunol (2018) 9:1861. doi: 10.3389/fimmu.2018.01861
96. Knight FC, Gilchuk P, Kumar A, Becker KW, Sevimli S, Jacobson ME, et al. Mucosal immunization with a ph-responsive nanoparticle vaccine induces protective Cd8(+) lung-resident memory T cells. ACS Nano (2019) 13(10):10939–60. doi: 10.1021/acsnano.9b00326
97. Wu S, Huang J, Zhang Z, Wu J, Zhang J, Hu H, et al. Safety, tolerability, and immunogenicity of an aerosolised adenovirus type-5 vector-based covid-19 vaccine (Ad5-ncov) in adults: Preliminary report of an open-label and randomised phase 1 clinical trial. Lancet Infect Dis (2021) 21(12):1654–64. doi: 10.1016/s1473-3099(21)00396-0
98. Bosnjak B, Odak I, Barros-Martins J, Sandrock I, Hammerschmidt SI, Permanyer M, et al. Intranasal delivery of mva vector vaccine induces effective pulmonary immunity against sars-Cov-2 in rodents. Front Immunol (2021) 12:772240. doi: 10.3389/fimmu.2021.772240
99. Lei H, Alu A, Yang J, Ren W, He C, Lan T, et al. Intranasal administration of a recombinant rbd vaccine induces long-term immunity against omicron-included sars-Cov-2 variants. Signal Transduct Target Ther (2022) 7(1):159. doi: 10.1038/s41392-022-01002-1
100. Kumar US, Afjei R, Ferrara K, Massoud TF, Paulmurugan R. Gold-Nanostar-Chitosan-Mediated delivery of sars-Cov-2 DNA vaccine for respiratory mucosal immunization: Development and proof-of-Principle. ACS Nano (2021) 15(11):7582–601. doi: 10.1021/acsnano.1c05002
101. Kingstad-Bakke B, Lee W, Chandrasekar SS, Gasper DJ, Salas-Quinchucua C, Cleven T, et al. Vaccine-induced systemic and mucosal T cell immunity to sars-Cov-2 viral variants. Proc Natl Acad Sci USA (2022) 119(20):e2118312119. doi: 10.1073/pnas.2118312119
102. Wakim LM, Smith J, Caminschi I, Lahoud MH, Villadangos JA. Antibody-targeted vaccination to lung dendritic cells generates tissue-resident memory Cd8 T cells that are highly protective against influenza virus infection. Mucosal Immunol (2015) 8(5):1060–71. doi: 10.1038/mi.2014.133
103. Lapuente D, Storcksdieck Genannt Bonsmann M, Maaske A, Stab V, Heinecke V, Watzstedt K, et al. Il-1β as mucosal vaccine adjuvant: The specific induction of tissue-resident memory T cells improves the heterosubtypic immunity against influenza a viruses. Mucosal Immunol (2018) 11(4):1265–78. doi: 10.1038/s41385-018-0017-4
104. Caminschi I, Lahoud MH, Pizzolla A, Wakim LM. Zymosan by-passes the requirement for pulmonary antigen encounter in lung tissue-resident memory Cd8(+) T cell development. Mucosal Immunol (2019) 12(2):403–12. doi: 10.1038/s41385-018-0124-2
105. Uddback I, Cartwright EK, Scholler AS, Wein AN, Hayward SL, Lobby J, et al. Long-term maintenance of lung resident memory T cells is mediated by persistent antigen. Mucosal Immunol (2021) 14(1):92–9. doi: 10.1038/s41385-020-0309-3
106. Kang MC, Choi DH, Choi YW, Park SJ, Namkoong H, Park KS, et al. Intranasal introduction of fc-fused interleukin-7 provides long-lasting prophylaxis against lethal influenza virus infection. J Virol (2015) 90(5):2273–84. doi: 10.1128/JVI.02768-15
107. Casey KA, Fraser KA, Schenkel JM, Moran A, Abt MC, Beura LK, et al. Antigen-independent differentiation and maintenance of effector-like resident memory T cells in tissues. J Immunol (2012) 188(10):4866–75. doi: 10.4049/jimmunol.1200402
108. Mackay LK, Stock AT, Ma JZ, Jones CM, Kent SJ, Mueller SN, et al. Long-lived epithelial immunity by tissue-resident memory T (Trm) cells in the absence of persisting local antigen presentation. Proc Natl Acad Sci U S A (2012) 109(18):7037–42. doi: 10.1073/pnas.1202288109
109. Park SL, Zaid A, Hor JL, Christo SN, Prier JE, Davies B, et al. Local proliferation maintains a stable pool of tissue-resident memory T cells after antiviral recall responses. Nat Immunol (2018) 19(2):183–91. doi: 10.1038/s41590-017-0027-5
110. Beura LK, Mitchell JS, Thompson EA, Schenkel JM, Mohammed J, Wijeyesinghe S, et al. Intravital mucosal imaging of Cd8(+) resident memory T cells shows tissue-autonomous recall responses that amplify secondary memory. Nat Immunol (2018) 19(2):173–82. doi: 10.1038/s41590-017-0029-3
111. Dan JM, Mateus J, Kato Y, Hastie KM, Yu ED, Faliti CE, et al. Immunological memory to sars-Cov-2 assessed for up to 8 months after infection. Science (2021) 371(6529):eabf4063. doi: 10.1126/science.abf4063
112. Fonseca R, Beura LK, Quarnstrom CF, Ghoneim HE, Fan Y, Zebley CC, et al. Developmental plasticity allows outside-in immune responses by resident memory T cells. Nat Immunol (2020) 21(4):412–21. doi: 10.1038/s41590-020-0607-7
113. Behr FM, Parga-Vidal L, Kragten NAM, van Dam TJP, Wesselink TH, Sheridan BS, et al. Tissue-resident memory Cd8(+) T cells shape local and systemic secondary T cell responses. Nat Immunol (2020) 21(9):1070–81. doi: 10.1038/s41590-020-0723-4
114. Wilk MM, Mills KHG. Cd4 trm cells following infection and immunization: Implications for more effective vaccine design. Front Immunol (2018) 9:1860. doi: 10.3389/fimmu.2018.01860
115. Deng N, Weaver JM, Mosmann TR. Cytokine diversity in the Th1-dominated human anti-influenza response caused by variable cytokine expression by Th1 cells, and a minor population of uncommitted il-2+ ifngamma- thpp cells. PLoS One (2014) 9(5):e95986. doi: 10.1371/journal.pone.0095986
116. Omokanye A, Ong LC, Lebrero-Fernandez C, Bernasconi V, Schon K, Stromberg A, et al. Clonotypic analysis of protective influenza M2e-specific lung resident Th17 memory cells reveals extensive functional diversity. Mucosal Immunol (2022) 15(4):717–29. doi: 10.1038/s41385-022-00497-9
117. Zhao Y, Kilian C, Turner J-E, Bosurgi L, Roedl K, Bartsch P, et al. Clonal expansion and activation of tissue-resident memory-like Th17 cells expressing gm-csf in the lungs of severe covid-19 patients. Sci Immunol (2021) 6(56):eabf6692. doi: 10.1126/sciimmunol.abf6692
118. Steinfelder S, Rausch S, Michael D, Kuhl AA, Hartmann S. Intestinal helminth infection induces highly functional resident memory Cd4(+) T cells in mice. Eur J Immunol (2017) 47(2):353–63. doi: 10.1002/eji.201646575
119. Zhang L, Li H, Hai Y, Yin W, Li W, Zheng B, et al. Cpg in combination with an inhibitor of notch signaling suppresses formalin-inactivated respiratory syncytial virus-enhanced airway hyperresponsiveness and inflammation by inhibiting Th17 memory responses and promoting tissue-resident memory cells in lungs. J Virol (2017) 91(10):e02111–16. doi: 10.1128/JVI.02111-16
Keywords: immune memory, tissue-resident memory T cells, respiratory syncytial virus, influenza, SARS-CoV-2, therapeutic strategies
Citation: Zhang M, Li N, He Y, Shi T and Jie Z (2022) Pulmonary resident memory T cells in respiratory virus infection and their inspiration on therapeutic strategies. Front. Immunol. 13:943331. doi: 10.3389/fimmu.2022.943331
Received: 13 May 2022; Accepted: 26 July 2022;
Published: 12 August 2022.
Edited by:
Julio Villena, CONICET Centro de Referencia para Lactobacilos (CERELA), ArgentinaReviewed by:
Adil Bhat, University of California, United StatesMaría Fernanda Raya Tonetti, Consejo Nacional de Investigaciones Científicas y Técnicas (CONICET), Argentina
Copyright © 2022 Zhang, Li, He, Shi and Jie. This is an open-access article distributed under the terms of the Creative Commons Attribution License (CC BY). The use, distribution or reproduction in other forums is permitted, provided the original author(s) and the copyright owner(s) are credited and that the original publication in this journal is cited, in accordance with accepted academic practice. No use, distribution or reproduction is permitted which does not comply with these terms.
*Correspondence: Zhijun Jie, amllempseGhAMTYzLmNvbQ==