- 1Zhejiang Provincial Engineering Technology Research Center of Marine Biomedical Products, School of Food and Pharmacy, Zhejiang Ocean University, Zhoushan, China
- 2Zhoushan Institute for Food and Drug Control, Zhoushan, China
- 3Department of Aquaculture, Zhejiang Ocean University, Zhoushan, China
The effects of Laminaria japonica fucoidan (LF) on immune regulation and intestinal microflora in cyclophosphamide (CTX)-treated mice were investigated in this work. Results indicated that LF significantly enhanced the spleen and thymus indices, promoted spleen lymphocyte and peritoneal macrophages proliferation, and increased the immune-related cytokines production in serum. Moreover, LF could regulate intestinal flora composition, increasing the abundance of Lactobacillaceae and Alistipes, and inhibiting Erysipelotrichia, Turicibacter, Romboutsia, Peptostreptococcaceae, and Faecalibaculum. These results were positively correlated with immune characteristics. Overall, LF could be useful as a new potential strategy to mitigate CTX immunosuppression and intestinal microbiota disorders.
Introduction
The immune system comprises immune organs, cells, and immune-reactive substances, which play vital roles in the host’s defense against foreign pathogens and microorganisms (1–3). The interaction between the natural and specific immune systems is necessary to maintain host health, and therefore, infections and various diseases will occur when the immune system is dysfunctional or impaired (4). Cyclophosphamide (CTX) is a potent immunosuppressant widely used in treating several immune diseases and malignant tumors (5, 6). However, numerous studies indicated that CTX could disrupt the DNA structure and immune cells and reduce normal lymphocytes and macrophages, thereby inhibiting cellular and humoral immune responses (7, 8). On the other hand, long-term or high doses usage of CTX (80 or 100 mg/kg body weight (BW)) can cause intestinal injury and intestinal microbiota disruption (9–11). Therefore, it is essential to discover effective immunomodulators to reduce the CTX side effects.
Recently, numerous natural polysaccharides have attracted more attention due to their various physiological activities without side effects (12–15). Polysaccharides have been developed as novel immunomodulators, enhancing host immunity and preserving intestinal health (16–18). It has been described that sulfated yam polysaccharide possesses stronger immunomodulatory activity to modulate gut microbiota structure in CTX-treated mice (11). Mulberry leaf polysaccharide has immunomodulatory activity by restoring the injured intestinal barrier and gut microbiota composition in CTX-treated mice (16). At the same time, one report indicated that Millettia Speciosa Champ polysaccharide modulates gut health and ameliorates immunosuppression and intestinal injury in CTX-treated mice (17). Considering all these studies, polysaccharides have the potential to be used as an effective immunomodulator to enhance immune function and regulate intestinal flora.
Due to the exploitation and utilization of marine resources, marine natural products have become a research hotspot (19, 20). Fucoidan, a sulfated polysaccharide, has numerous biological activities, including antitumor, antioxidant, anti-inflammatory, immunoregulatory, among others (21–24). Kjellmaniella crassifolia or Undaria pinnatifida fucoidan could stimulate RAW264.7 cell proliferation in vitro and induce a significant immune enhancement in vivo (22). Hwang et al. (23) indicated that low-molecular-weight fucoidan from Laminaria japonica could enhance the innate and adaptive immune responses and protects against Mycoplasma pneumoniae antigen stimulation. Sun et al. (24) indicated that low-molecular-weight fucoidan from Laminaria japonica could play an antiviral role by improving the quality of immune organs, improving immune cell phagocytosis and humoral immunity. Nevertheless, there is still a lack of systematic studies regarding the link between the immune regulation function of Laminaria japonica fucoidan and intestinal flora.
In our previous studies, we discovered that Laminaria japonica fucoidan (LF) could effectively ameliorate CTX-induced liver and kidney injury (25). Herein, the immunomodulatory function of LF was investigated using immunosuppressed mice. In addition, the link between immunomodulatory function and gut microbiota was also evaluated. This systematic research could provide insights into the link between immune regulation function and intestinal flora, and also provided a better understanding of the LF applicability.
Materials and Methods
Materials and Reagents
LF, with an average molecular weight of 250 kDa, is composed of mannose, rhamnose, galactose, xylose, and fucose in a molar ratio of 2.04: 0.58: 1.04: 3.91: 12.43, respectively (25). CTX was supplied by Hengrui Medicine (Lianyungang, China). Concanavalin A (ConA) and lipopolysaccharide (LPS) were purchased from Sigma-Aldrich (Sigma-Aldrich, USA). Tumor necrosis factor (TNF)-α, interleukin (IL)-6, and IL-1β ELISA kits were purchased from Boster (Wuhan, China). Immunoglobulin G (IgG) ELISA kit was purchased from Solarbio (Beijing, China).
Animals and Design
Male ICR mice (18-22 g, 6-8 weeks) were purchased from the Zhejiang Academy of Medical Sciences. All mice were maintained in a breeding environment (23 ± 2°C, 60% ± 5 humidity) on a 12 h light/12 h dark cycle. After 7 days of adaptive feeding, mice were randomly divided into 4 groups (n = 8 per group) as follows: control (only treated with a saline solution), model (mice treated with 80 mg/kg BW CTX), 20 LF (mice treated with 20 mg/kg BW LF), and 40 LF (mice treated with 40 mg/kg BW LF). The control group received gavages with saline solution for 19 days (once daily), while the remaining three groups were firstly injected with CTX (80 mg/kg BW) for 5 days (once daily) and then saline solution or 20 and 40 mg/kg BW of LF for another 14 consecutive days (once daily) were received by gavage.
Spleen and Thymus Indices Determination
The spleen and thymus indices were calculated as follows:
Splenic Lymphocyte Proliferation Assay
Splenic lymphocyte was prepared according to Han and collaborators (26). Briefly, the mouse spleen was grinding, and splenic lymphocytes were collected. Next, erythrocyte lysis buffer was added to lyse the red blood cells, and the supernatant was collected by centrifugation (at 1500 × g, 5 min). After removing adherent cells, the cells were incubated in a 6-well plate for 6 h, and the suspended cells were collected as splenic lymphocytes. Then, the cells (1 × 106 cells/mL) were seeded in 96-well plates (final volume of 100 μL). Then, ten microliters of ConA (5 μg/mL) or LPS (1 μg/mL) were added and incubated for 72 h at 37°C with 5% CO2. Next, the MTT solution (10 µL, 5 mg/mL) was added, and the absorbance at 490 nm was detected on a microplate reader (Molecular Devices, CA, USA).
Macrophages Proliferation Assay
The mice were intraperitoneally injected with 5 mL sterile saline solution, and the abdomen was gently pressed for 2 min. After, the abdominal wall was cut open with sterilized scissors, and the abdominal fluid was sucked into a centrifuge tube with a sterile straw. The cell suspension was centrifuged (2000 rpm, 10 min) and resuspended with DMEM medium. The supernatant was discarded to obtain purified macrophages after incubation at 37°C with 5% CO2 for 4 h. After this, the cells (1 × 104 cells/mL) were seeded in a 96-well plate (final volume of 200 μL). After 24 h at 37°C with 5% CO2, an MTT solution (10 µL, 5 mg/mL) was added, and the absorbance at 490 nm was detected on a microplate reader.
Phagocytic Activity of Peritoneal Macrophages
The macrophages (1 × 104 cells/mL) were seeded in a 96-well plate (final volume of 200 μL). After 36 h, the medium was discarded, and a neutral red solution (0.1%) was added. After 3 h incubation, the excess neutral red was removed by washing twice with 0.1 M PBS, and lysis solutions (200 μL) were added and shaken for 10 min at 25°C. The absorbance at 540 nm was detected on a microplate reader.
Serum Cytokines and IgG Content Analysis
The blood was collected from eyeball extirpation, and serum was acquired by centrifugation (6000 × g, 3 min, 4°C) of the blood. The serum levels of TNF-α, IL-6, IL-1β, and IgG were determined using Boster’s ELISA kits.
Histological Analysis
The spleen and thymus cross-sections were fixed (4% paraformaldehyde) and embedded in paraffin (10%). The hematoxylin-eosin (HE) staining was performed as in previous studies (20, 27). Micrographs were taken using a light microscope CX31 (Olympus, Japan).
Oxidative Stress Index Analysis
The spleen was ground in saline, and the supernatant was collected by centrifugation. Malondialdehyde (MDA), glutathione peroxidase (GSH-Px), superoxide dismutase (SOD), and catalase (CAT) levels in spleen tissues were measured using Jiancheng’s commercial kits (Nanjing, China).
Fecal Microbiota Analysis
The genomic DNA of mice feces was extracted, and 338F and 806R primers were used to amplify the V3-V4 region of bacterial 16S rRNA as previously described (27, 28). The MiSeq library was constructed by adding the Illumina official connector sequences to the target area of the PCR products (Shanghai Origingene Bio-pharm Technology Co.Ltd., China). Then, the Illumina Novaseq6000 platform (Illumina, San Diego, USA) was applied for paired sequencing, and the paired-end reads were chosen according to the overlapping relationship. Operational taxonomic units (OTUs) clustering analysis and taxonomic analysis were performed to analyze the diversity indices and community structure at different taxonomic levels, respectively.
Correlation Between Key Microbes and Immunity-Related Parameters
The correlation heatmap analysis was used to calculate the Spearman rank correlation coefficient between the intestinal key microbial species and basic immunity-related parameters (29). In addition, the microorganisms expressing the highest correlation with LF to enhance the CTX-induced immunosuppression were screened out.
Statistical Analysis
Results are expressed as the mean ± standard deviation (SD). Data analysis was performed using SPSS 24.0 (SPSS Inc., Chicago, IL, USA). P < 0.05 was considered statistically significant.
Results
Effect of LF on Spleen and Thymus Indices
In the model group, the spleen and thymus indices were significantly decreased compared to the control group (Figure 1A). However, when treated with LF, the spleen and thymus indices showed a significant increase compared to the model (P < 0.05 or P < 0.01), suggesting that LF treatment could effectively alleviate the CTX-induced atrophy of the spleen and thymus.
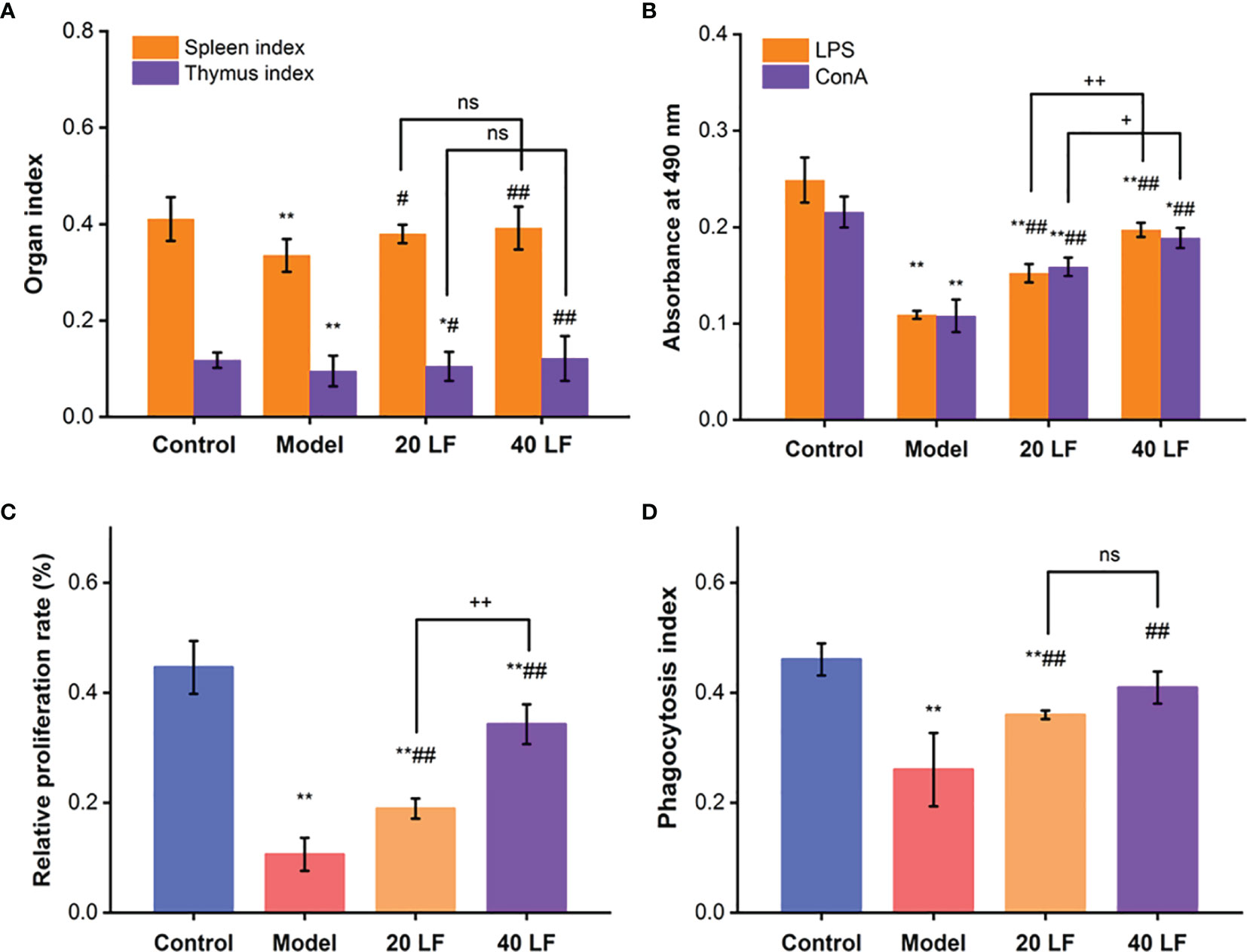
Figure 1 Immune organ indexes of spleen and thymus (A), splenic lymphocytes proliferation (B), peritoneal macrophage proliferation (C), and the phagocytic (D) index, (n = 8). *P < 0.05, **P < 0.01 vs control; #P < 0.05, ##P < 0.01 vs model, +P < 0.05, ++P < 0.01 compared between 20 LF and 40 LF, and ns means no significant difference.
Effect of LF on Splenic Lymphocyte Proliferation
When treated with ConA or LPS, spleen lymphocytes were induced into T and B lymphocytes, respectively (30, 31). Compared to the control, the proliferation of T and B lymphocytes was significantly reduced (P < 0.01) in the model, suggesting that CTX could modulate and reduce the cellular immune response (Figure 1B). On the other hand, when treated with both LF treatments, the proliferation indices were significantly enhanced compared with the model (P < 0.01), suggesting that LF could improve cellular immune response by promoting spleen lymphocytes proliferation. Compared to the model, the macrophages’ relative proliferation rate and the phagocytic index were significantly increased in the 20 LF and 40 LF groups (Figures 1C, D, P < 0.01), suggesting that LF could promote the macrophages’ proliferation and overcome the immunosuppressed activity induced by CTX.
Serum Cytokines and IgG Content Analysis
Compared to the control, the IL-6, IL-1β, TNF-α, and IgG levels in the model group were significantly decreased (Figure 2, P < 0.01), suggesting that CTX inhibited the immune regulation system of mice. However, after treatment with LF, these levels in the 20 LF and 40 LF groups were significantly restored compared to the model (P < 0.01), suggesting that LF could improve mice immunosuppression via enhancing the cytokines and IgG production.
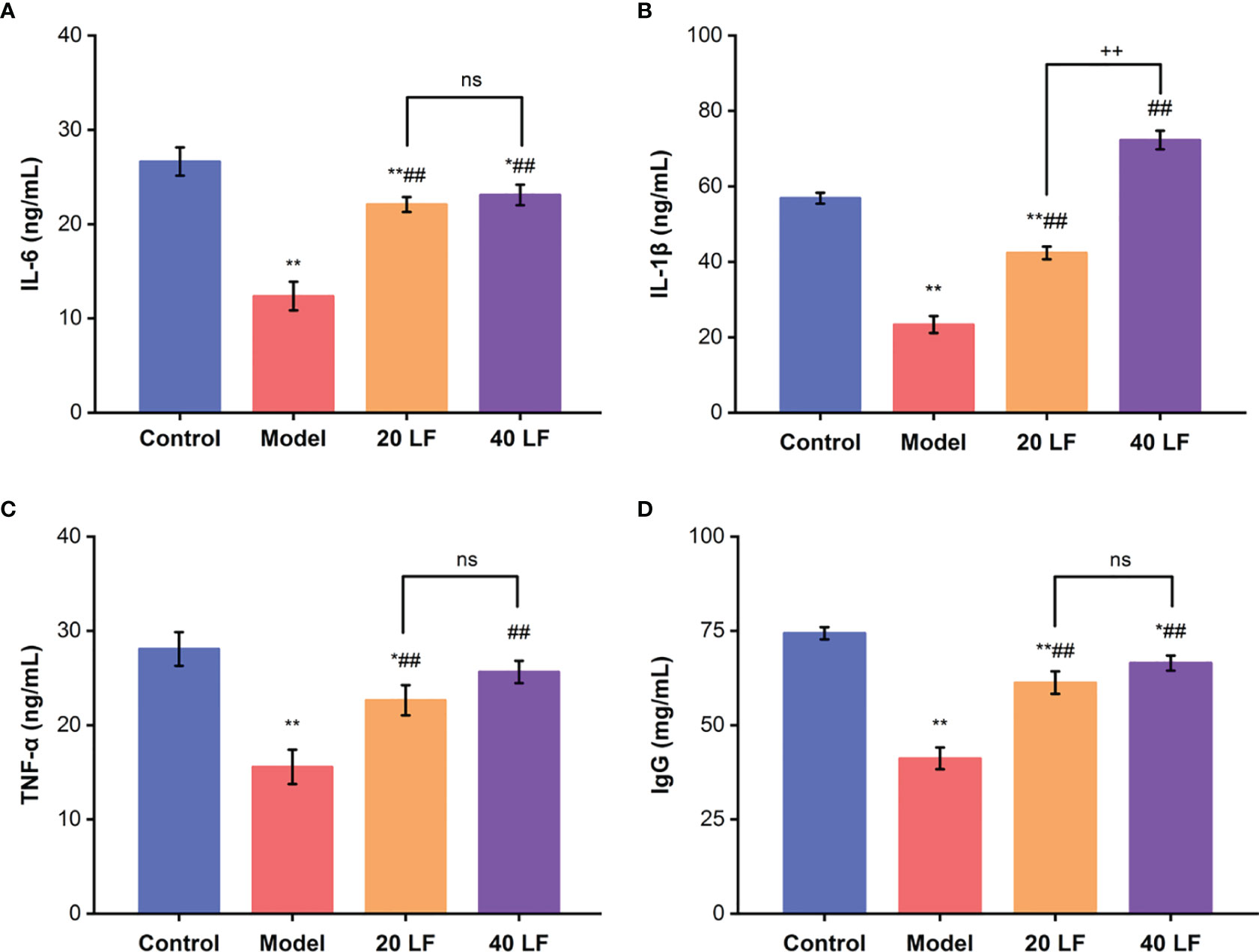
Figure 2 Effects of LF on the serum levels of IL-6 (A), IL-1β (B), TNF-α (C), and IgG (D), (n = 8). *P < 0.05, **P < 0.01 vs control; ##P < 0.01 vs model, ++P < 0.01 compared between 20 LF and 40 LF, and ns means no significant difference.
Histological Analysis
To observe the effect of LF on the immune organs, HE staining was applied to detect alterations in the spleen and thymus (Figure 3A). The splenic vesicles in the control group remained structurally intact, and a clear demarcation line between the red and white marrow was observed. The splenic cords in the red marrow were connected as a whole, and the blood cells around the exterior of the splenic vesicles were surrounded orderly (Figure 3A). However, there were no clearly formed splenic vesicles in the spleen in the model group, and the white and red medullae were partially mixed. The thinning of the lymphatic sheath around the small central artery implies that CTX could damage the spleen immune cells, resulting in spleen atrophy. When treated with 20 mg/kg LF, the general structure of the splenic vesicles could be observed, but the boundary among the red and white medulla remained unclear. Moreover, after treatment with 40 LF, the red and white medulla could be observed, and the marginal area of the white medulla was widened. Overall, the morphology of the spleen in the LF group gradually returned to a similar shape found in the control, suggesting that LF could restore the spleen injury caused by CTX.
Moreover, the cortical and medullary structures of the control were clear and distinct, and evident thymus vesicles could be observed in the medulla (Figure 3B). However, when treated with CTX, the structure of the cortex and medulla in the model group was diffused, and few and not evident thymus vesicles were observed in the visual field. On the other hand, there was a clear distinction between the cortex and medulla when treated with LF, mainly in the 40 LF group. Furthermore, the morphology was similar to the control, suggesting that LF could restore the thymus injury caused by CTX.
Oxidative Stress Index Analysis
The MDA levels and the activities of GSH-Px, SOD, and CAT were also assessed in the spleen. Compared to the control, the MDA contents were significantly increased, and the GSH-Px, SOD, and CAT activities were significantly decreased in the model (Figure 4) (P < 0.01). After being treated with LF, the MDA contents were significantly reduced (P < 0.01), and the GSH-Px, SOD, and CAT activities were significantly increased compared to the model group (P < 0.01). These results suggest that LF could improve CTX-induced oxidative stress in the spleen tissues.
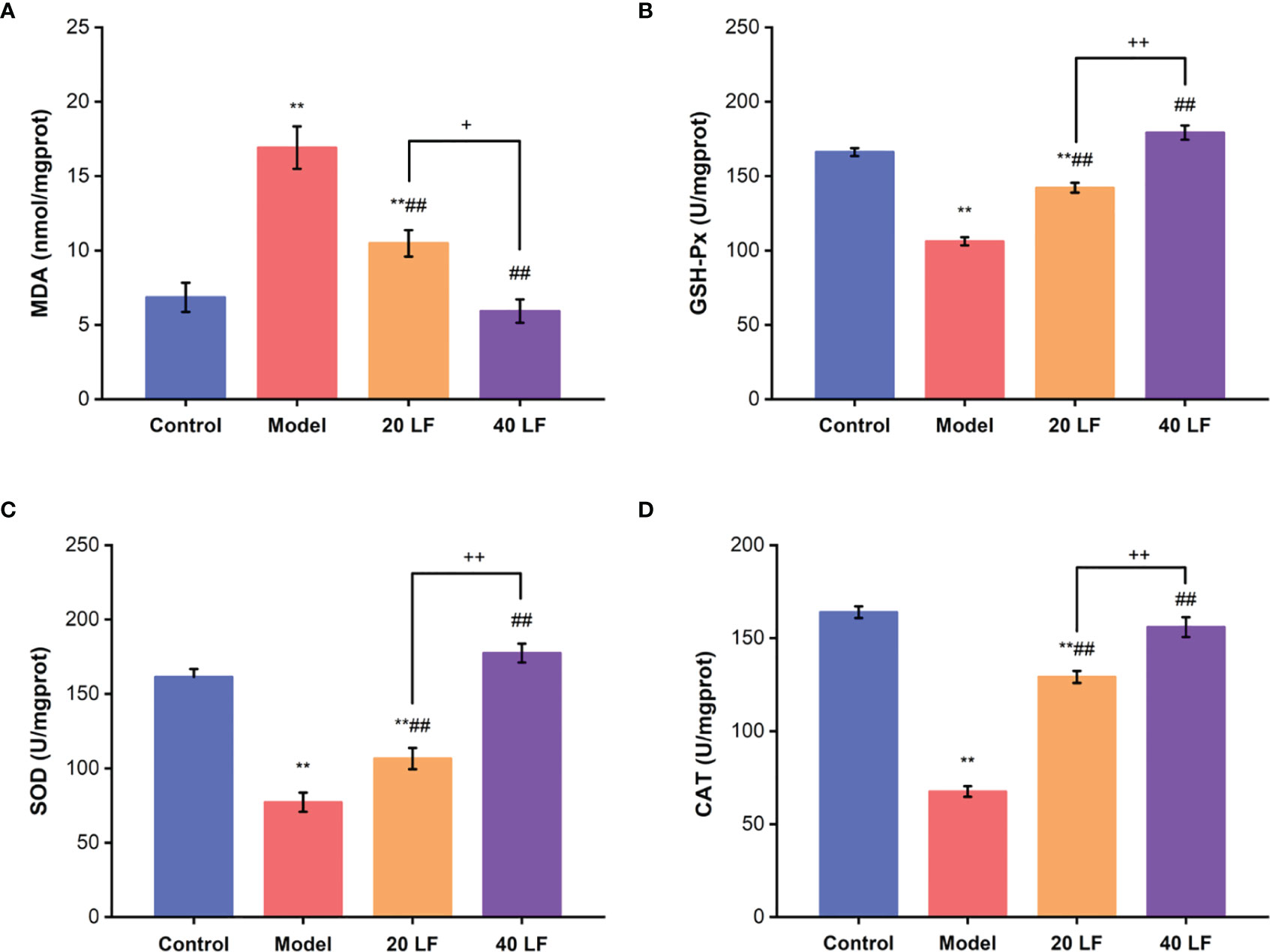
Figure 4 Effects of LF on the MDA levels (A), GSH-Px, SOD, and CAT activities GSH-Px (B), SOD (C), and CAT (D) of the spleen (n = 8). **P < 0.01 vs control; ##P < 0.01 vs model, *P < 0.05, ++P < 0.01 compared between 20 LF and 40 LF.
Diversity Analysis of the Intestinal Flora
To investigate the LF effect on intestinal microflora composition in mice, a 16S rRNA sequencing was performed. The Venn diagram showed common and unique OTUs between different samples (Figure 5A), and 803 OTUs in all samples were identified. Additionally, 640 shared OTUs in the four groups, accounting for 79.70% of the total OTUs, indicating that the bacterial compositions of all groups were similar. Non-metric multi-dimensional scaling (NMDS) analysis can reflect the relationship between microbial communities of different samples. As shown in Figure 5B, there are significant differences in the microbial community structure between the control and the model group, while the microorganisms of the 20 LF and 40 LF groups are relatively similar to those of the control group. In addition, the alpha diversity change (Shannon and Chao indices) was shown in Figures 5C, D. Except for the Chao index of the 40 LF group, the results of alpha diversity were significantly different from the CTX group after different doses of LF intervention (P < 0.05), indicating that LF could reverse the changes in the abundance and diversity of fecal bacteria caused by CTX.
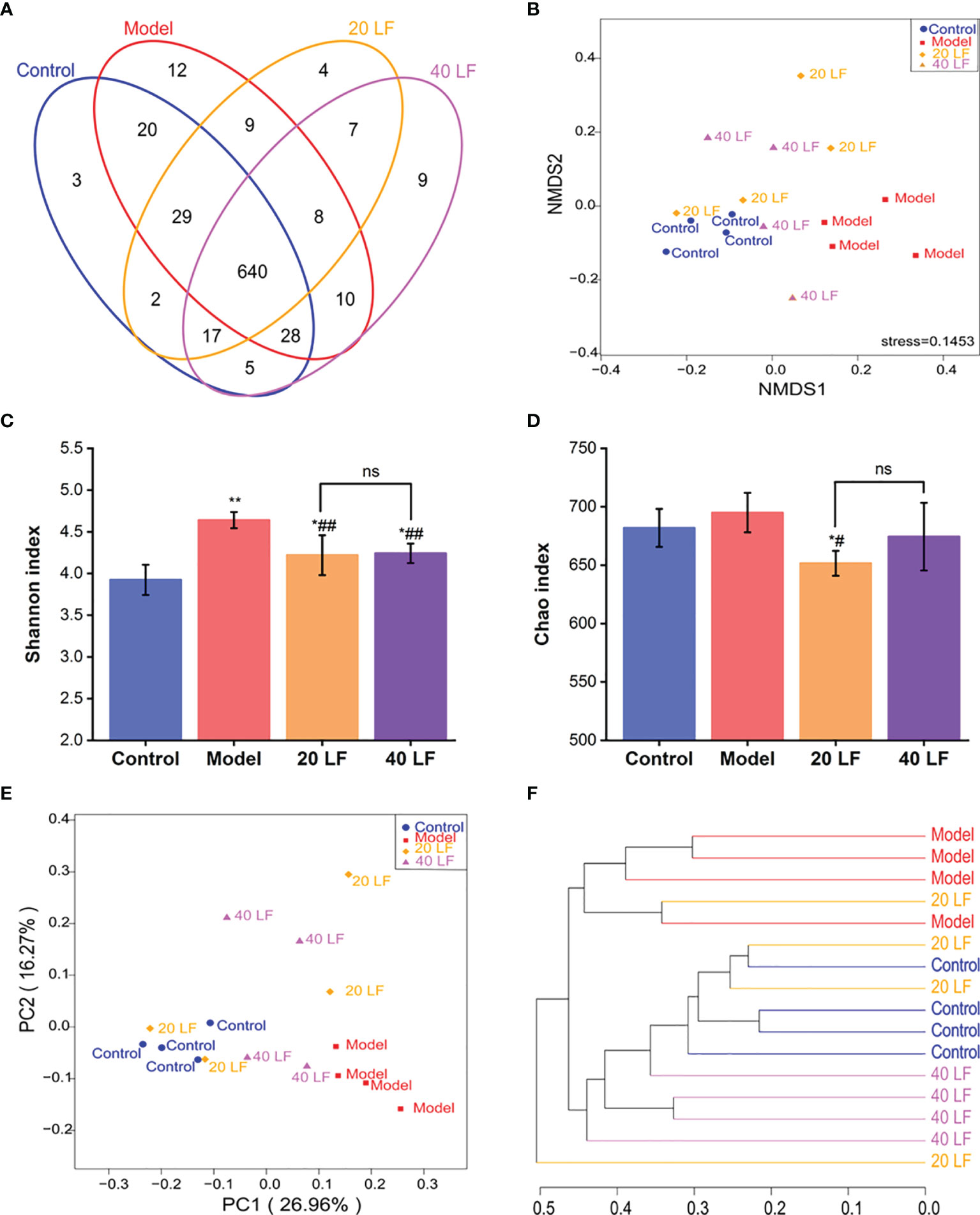
Figure 5 Effects of LF treatment on the gut microbiota composition of the CTX-induced mice (n = 4). (A) Venn diagrams. (B) Non-metric multi-dimensional scaling (NMDS) analysis. (C) Shannon indices. (D) Chao indices. (E) Principal coordinates analysis. (F) Cluster tree analysis based on the unweighted UniFrac at the genus level. *P < 0.05, **P < 0.01 vs control; #P < 0.05, ##P < 0.01 vs model, and ns means no significant difference.
Principal coordinates analysis (PCOA) and cluster tree analysis were performed to analyze the similarity of microbial communities among different groups. The PCOA results suggested that CTX and LF intervention significantly affected the intestinal flora composition (Figure 5E). Furthermore, the distance between the 40 LF and control groups was smaller than that between the control and model groups, suggesting that the intervention of LF could cause a shift in gut flora composition. In addition, the cluster analysis results based on bray curtis distance were consistent with the PCOA results (Figure 5F), suggesting that CTX and LF could effectively influence the intestinal flora composition. In addition, the intestinal flora of the LF group was more similar to the control.
Analysis of Community Differences of Intestinal Flora
To identify specific taxa associated with LF, we assessed the relative abundance of species at phylum and family levels. The mouse intestinal flora comprises Firmicutes, Bacteroidetes, and Proteobacteria, together accounting for over 90% (Figure 6A). The differences in the relative abundance of these three major bacteria were further analyzed (Figure 6B). It was observed that CTX treatment significantly decreased the abundance of Firmicutes and significantly increased the abundance of Proteobacteria (P < 0.05). After LF treatment, the changes of relative abundance of the three phyla were reversed, but only changes of Proteobacteria abundance in the 20 LF group were significantly different. The gut flora varied significantly at the family level (Figure 6C), where the top 10 species with relative abundance were Lactobacillaceae, Lachnospiraceae, Bacteroidales_S24-7_group, Ruminococcaceae, Rikenellaceae, Porphyromonadaceae, Enterobacteriaceae, Helicobacteraceae, Bacteroidaceae, and Peptostreptococcaceae (Table 1). CTX significantly altered the relative abundance of Lactobacillaceae, Bacteroidales_S24-7_group, and Peptostreptococcaceae compared to the control, and LF treatment at different doses reversed these changes caused by CTX (Figure 6D, P < 0.01).
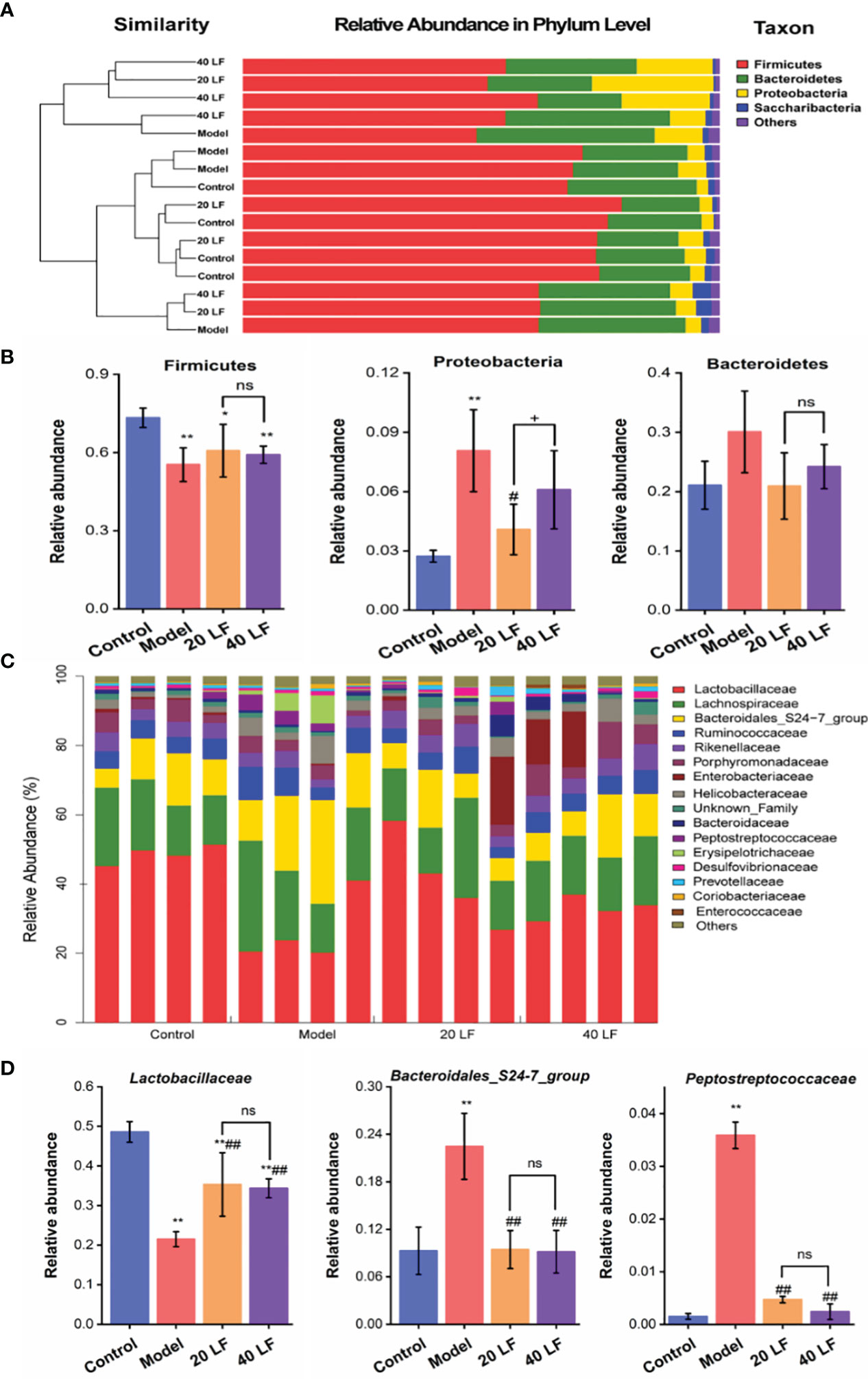
Figure 6 Comparisons of the gut microbiota at phylum and family taxonomic levels (n = 4). (A) Gut microbiota composition at the phylum level. (B) Relative abundance of the gut microbiota at the phylum level. (C) Gut microbiota composition at the family level. (D) Relative abundance of the gut microbiota at the family level. *P < 0.05, **P < 0.01 vs control; #P < 0.05, ##P < 0.01 model, +P < 0.05 compared between 20 LF and 40 LF, and ns means no significant difference.
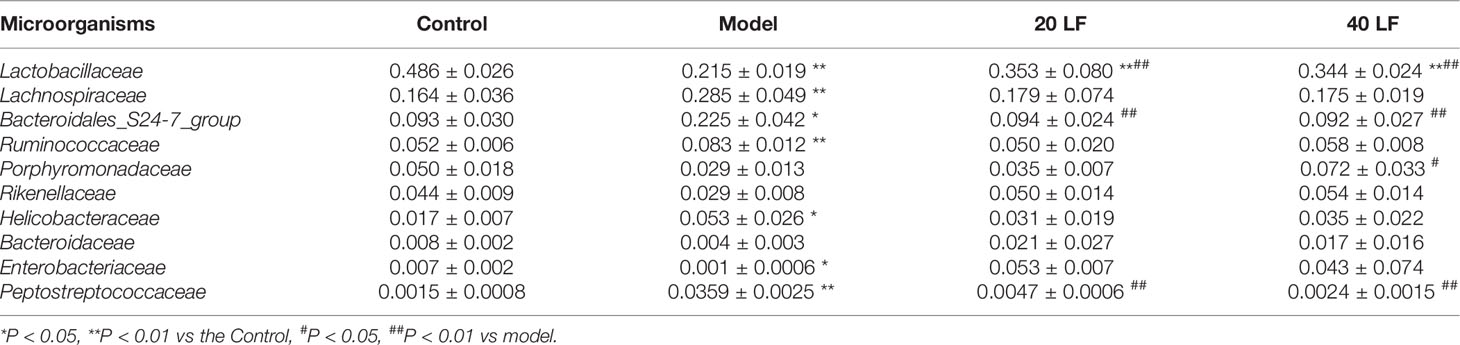
Table 1 Changes of intestinal microorganisms in the top 10 relative abundance at the family level (n = 4).
LEfSe Analysis of Intestinal Flora in Mice
LEfSe was performed to identify taxa with significant differences in abundance, while linear discriminant analysis (LDA) was performed to estimate the influence of the abundance of each component on the differential effect. From the Lefse results (Figure 7), it was observed that the CTX treatment mainly suppressed the relative abundance of Lactobacillales, Bacilli, Enterobacteriales, and Gammaproteobacteria, and promoted the relative abundance of Family_XIII_UCG_001, Eubacterium_brachy_group, Blautia, Lachnoclostridium, Romboutsia, Peptostreptococcaceae, Anaerotruncus, Ruminococcaceae_UCG_010, Faecalibaculum, Turicibacter, Erysipelotrichia, Helicobacteraceae, Campylobacterales, and Epsilonproteobacteria. Compared to the model, 40 LF treatment significantly suppressed the relative abundance of the CTX-stimulated microorganisms, especially the relative abundance of Erysipelotrichia-related groups, and promoted the relative abundance of Alistipes, Gastranaerophilales, Cyanobacteria, and Streptococcus.
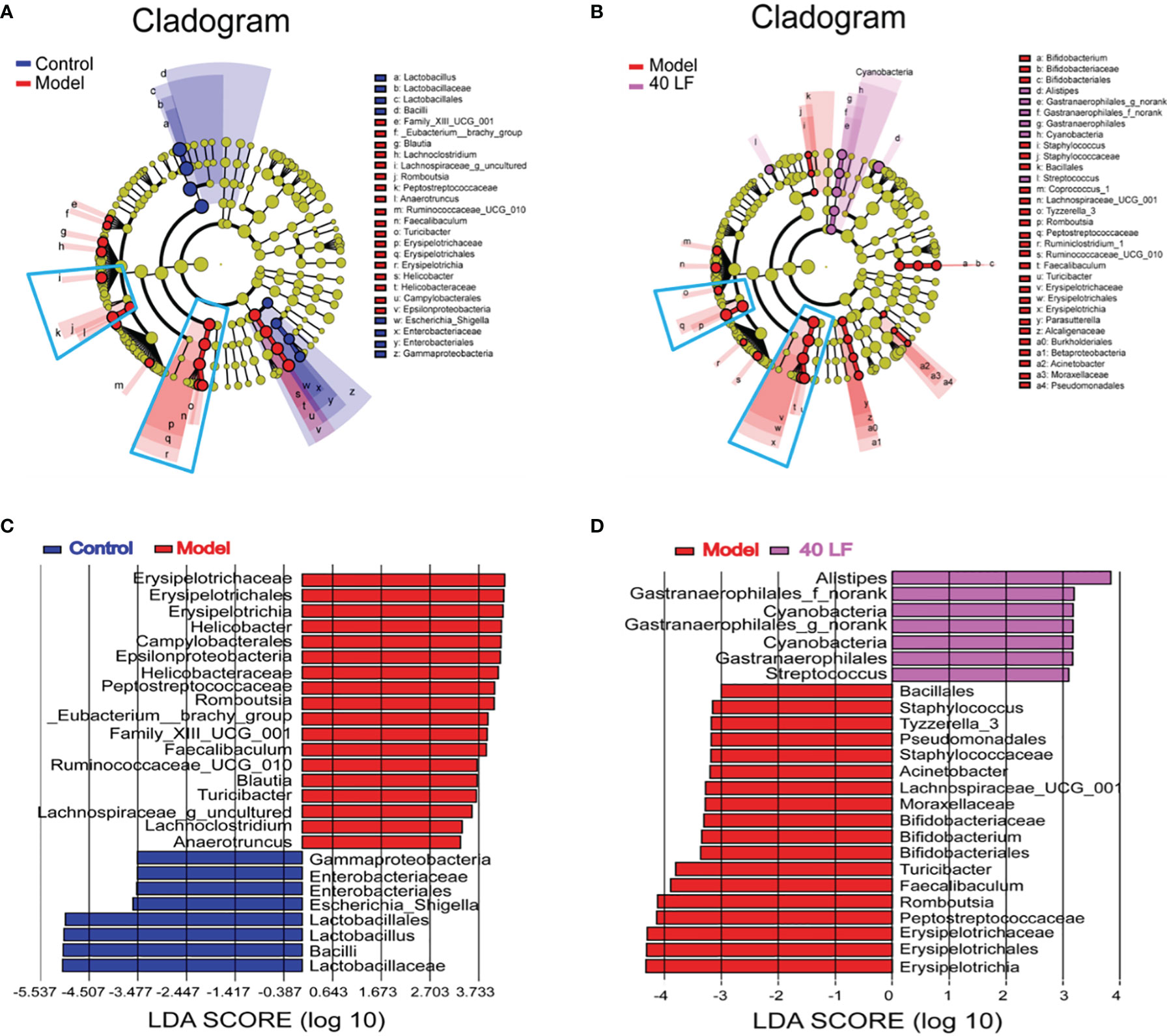
Figure 7 The LEfSe and Spearman correlation analyses of the fecal microbes of the different mice groups (n = 4). (A) LEfSe analysis showing the key differential microbes of the CTX-induced mice. (B) LEfSe analysis showing the key differential microbes of the CTX-induced immunosuppressed mice subjected to the 40 LF intervention. (C) The LDA score between the control and the model groups. (D) The LDA score between the model and the 40-LF treated groups.
Correlation Between Key Microbes and Host Parameters
The correlation heatmap analysis was used to calculate the Spearman rank correlation coefficient between the intestinal key microbial species and host parameters. In addition, the key microorganisms expressing the highest correlation with LF to enhance the CTX-induced immunosuppression were screened out. As depicted in Figure 8, 5 types of microbes (Romboutsia, Peptostreptococcaceae, Faecalibaculum, Turicibacter, and Erysipelotrichia) presented a significant negative correlation with the CAT, SOD, and GSH-Px activities, pro-inflammatory cytokine levels (IL-1β and TNF-α), while also being positively correlated with the MDA content (P < 0.05). These bacteria were the key species of intestinal microbiota in the model group and were also inhibited by LF. In contrast, 3 types of key microorganisms (Lactobacillaceae, Bacilli, and Lactobacillus) presented a positively correlated with pro-inflammatory cytokine levels (IL-6 and TNF-α), IgG, CAT activity. Moreover, the LF group was also enriched with Alistipes, presenting a significant negative correlation with MDA levels and positively correlated with SOD and GSH-Px activities.
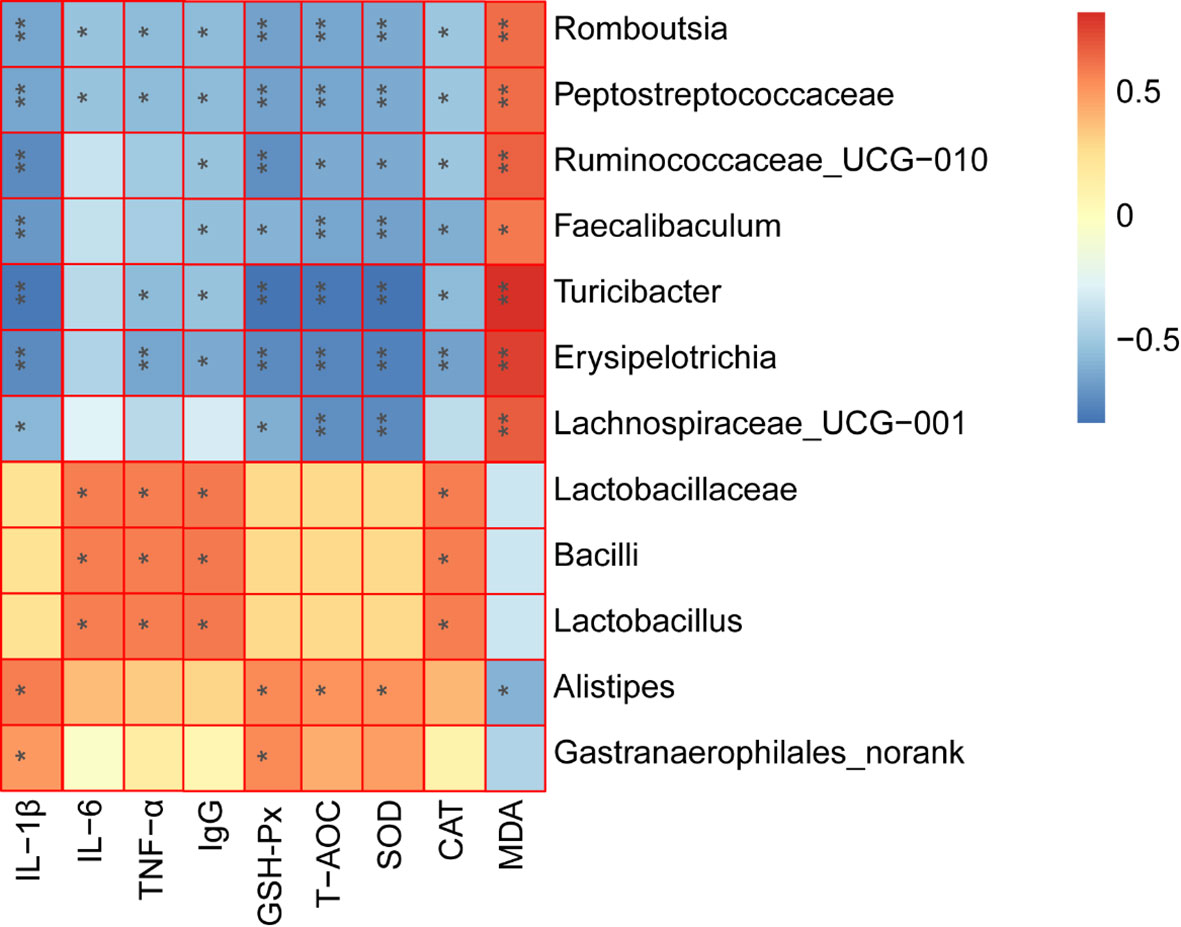
Figure 8 Spearman correlation analysis between the key microbial communities and immune-related biochemistry parameters. *P < 0.05, **P < 0.01.
Discussion
CTX is an effective immunosuppressant, widely applied as a vital drug in cancer treatment and both blood and bone marrow transplants (5). However, CTX treatment can induce immunosuppression and intestinal flora dysfunction (32, 33). Polysaccharides could be used to alleviate the CTX-induced immunosuppression and intestinal dysbacteriosis (16, 17). For example, Mulberry leaf-derived polysaccharide has the potential to modulate the immune response and gut microbiota composition in immunosuppressed mice (16). Millettia Speciosa Champ roots polysaccharides could modulate gut health and ameliorate CTX-induced intestinal injury and immunosuppression (17). In this work, immunosuppression was induced in mice to investigate the possible positive effects of LF treatment on such immunosuppression, as well as the intestinal flora dysfunction caused by CTX.
The ability of the spleen to induce lymphocytic proliferation is an important index of the body’s immune response (26). As another important immune cell, macrophages exist in various cellular tissues and, together with neutrophils, constitute the first line of the body’s immune defense, playing vital roles in non-specific and specific immunity (30). Macrophages are phagocytes derived from monocytes and play key roles in natural and specific immune systems (31). Activated macrophages can actively phagocytose and remove foreign antigens or directly kill pathogenic microorganisms. In this study, CTX significantly decreased the mice’s T and B lymphocyte proliferation, suggesting an impaired cellular immune response, consistent with previous studies (26, 34). However, LF (20 mg/kg or 40 mg/kg) treatment could significantly promote the T and B lymphocytes proliferation. Moreover, the proliferative capacity and phagocytosis indices of peritoneal macrophages were also significantly increased after LF treatment. These results showed that LF treatment ameliorated the CTX injury to immune cells and enhanced the immune response of mice.
Cytokines are soluble extracellular polypeptides or glycoproteins indispensable in the immune response (29, 32). Immunoglobulins, secreted by B cells, are an important part of the immune response (29). CTX could decrease the serum levels of cytokines or immunoglobulins, causing a decrease in immunity (29, 31). Our results indicated that the IL-6, IL-1β, TNF-α, and IgG serum levels were decreased in the model group, which was consistent with previous studies (29, 31). However, after treatment with LF, both cytokines (IL-6, IL-1β, TNF-α) and IgG serum levels were significantly increased compared with the model, indicating that LF could promote IL-6, IL-1β, TNF-α, and IgG serum levels to improve immune function, consistent with the previous studies (23). The spleen and thymus are two important immune organs (35). In this work, the spleen and thymus indices were decreased significantly after CTX treatment, and HE staining results also indicated that CTX could induce apoptosis of the spleen and thymus, but LF treatment could reverse these adverse effects, suggesting that LF had a rescue effect. Sun et al. (24) that low-molecular-weight fucoidan from Laminaria japonica could increase the thymus and spleen index in the virus-infected mice, and our results were consistent with their studies. Furthermore, previous studies have shown a correlation between immune ecology and oxidative stress, and immune organ damage is closely related to oxidative stress (29, 36). Moreover, the dynamic balance between the oxidative and antioxidant states of the body plays a vital role in safeguarding the organism’s health (30, 37). Polysaccharides have strong antioxidant properties and can ameliorate the CTX-induced low immunity by alleviating oxidative stress damage (30, 38). In this work, the spleen levels of GSH-Px, CAT, and SOD were significantly decreased, and MDA levels were significantly increased in the model group, consistent with previous studies (38). While GSH-Px, CAT, and SOD levels were significantly enhanced after LF treatment, MDA levels were significantly reduced. These results suggested that LF could ameliorate the CTX-induced oxidative stress, consistent with our previous studies (25).
Gut flora plays key roles in shaping the immune system (39). CTX could modulate the intestinal flora composition, while polysaccharides also can modulate intestinal flora composition after CTX treatment, thus regulating the host’s immunity (11, 29). In this work, after CTX treatment, the relative abundances of Lactobacillaceae, Porphyromonadaceae, Rikenellaceae, Bacteroidaceae, and Enterobacteriaceae were decreased, and the relative abundances of Bacteroidales_S24-7_group, Ruminococcaceae, Lachnospiraceae, Helicobacteraceae, and Peptostreptococcaceae were significantly increased. These results are in agreement with the work performed by Ding and collaborators (40). However, LF can reverse the CTX-induced changes in the abundance of bacteria at the family level. A comprehensive analysis of Lefse and community composition found that LF treatment significantly reversed the intestinal flora disturbance caused by CTX by significantly inhibiting the relative abundance of Erysipelotrichia, Peptostreptococcaceae, Faecalibaculum, Turicibacter, Romboutsia, and Helicobacteraceae. The relative abundance of Erysipelotrichia related groups, and beneficial strains, including Lactobacillaceae, Alistipes, Gastranaerophilales, Cyanobacteria, and Streptococcus, increased significantly. Further, the Spearman rank correlation coefficient was used to calculate the association between the intestinal key microbial species and host parameters. The results showed that 5 types of key microorganisms (Romboutsia, Peptostreptococcaceae, Faecalibaculum, Turicibacter, and Erysipelotrichi) were negatively correlated with immune characteristics, and 4 types of key microorganisms (Lactobacillaceae, Bacilli, Lactobacillus, and Alistipes) were positively correlated with immune characteristics.
Conclusion
In summary, LF enhanced the immune response by enhancing the secretion of cytokines and IgG, alleviating spleen and thymus injury in CTX-treated mice. In addition, LF could also regulate the intestinal flora disorder caused by CTX. These findings indicated that LF has the potential to be used as an immunoregulatory adjuvant or functional food additive to ameliorate CTX-induced immunosuppression.
Data Availability Statement
The datasets presented in this study can be found in online repositories. The names of the repository/repositories and accession number(s) can be found below: https://www.ncbi.nlm.nih.gov/genbank/, PRJNA830087.
Ethics Statement
The animal study was reviewed and approved by Animal Ethics Committee of Zhejiang Ocean University.
Author Contributions
YT and TH conceived the study and designed the project. YT, QP, QZ, YZ, and XJ performed the experiment and analyzed the data. YT drafted the manuscript. YT and TH revised the manuscript and supervised the whole study. All authors contributed to the article and approved the submitted version.
Funding
This work was financially supported by the Zhoushan Science and Technology Project (No.2022C41004), and the National Natural Science Foundation of China (No. 41806153).
Conflict of Interest
The authors declare that the research was conducted in the absence of any commercial or financial relationships that could be construed as a potential conflict of interest.
Publisher’s Note
All claims expressed in this article are solely those of the authors and do not necessarily represent those of their affiliated organizations, or those of the publisher, the editors and the reviewers. Any product that may be evaluated in this article, or claim that may be made by its manufacturer, is not guaranteed or endorsed by the publisher.
References
1. Chen XH, Wang SS, Chen GJ, Wang ZR, Kan JQ. The Immunomodulatory Effects of Carapax Trionycisultrafine Powder on Cyclophosphamide-Induced Immunosuppression in Balb/c Mice. J Sci Food Agri (2021) 101(5):2014–26. doi: 10.1002/jsfa.10819
2. Berkoz M, Yalin S, Ozkan-Yilmaz F, Ozluer-Hunt A, Krosniak M, Francik R, et al. Protective Effect of Myricetin, Apigenin, and Hesperidin Pretreatments on Cyclophosphamide-Induced Immunosuppression. Immunopharmacol Immunotoxicol (2021) 43(3):353–69. doi: 10.1080/08923973.2021.1916525
3. Lv L, Mu D, Du Y, Yan R, Jiang H. Mechanism of the Immunomodulatory Effect of the Combination of Live Bifidobacterium, Lactobacillus, Enterococcus, and Bacillus on Immunocompromised Rats. Front Immunol (2021) 12:694344. doi: 10.3389/fimmu.2021.694344
4. Parkin J, Cohen B. An Overview of the Immune System. Lancet (2001) 357(9270):1777–89. doi: 10.1016/S0140-6736(00)04904-7
5. Ahlmann M, Hempel G. The Effect of Cyclophosphamide on the Immune System: Implications for Clinical Cancer Therapy. Cancer Chemother Pharmacol (2016) 78(4):661–71. doi: 10.1007/s00280-016-3152-1
6. Li CP, Duan SS, Li YP, Pan X, Han LR. Polysaccharides in Natural Products That Repair the Damage to Intestinal Mucosa Caused by Cyclophosphamide and Their Mechanisms: A Review. Carbohydr Polym (2021) 261:117876. doi: 10.1016/j.carbpol.2021.117876
7. Deng J, Zhong YF, Wu YP, Luo Z, Sun YM, Wang GE, et al. Carnosine Attenuates Cyclophosphamide-Induced Bone Marrow Suppression by Reducing Oxidative DNA Damage. Redox Biol (2018) 14:1–6. doi: 10.1016/j.redox.2017.08.003
8. Yu Y, Mo SR, Shen MY, Chen Y, Yu Q, Li ZD, et al. Sulfated Modification Enhances the Immunomodulatory Effect of Cyclocarya Paliurus Polysaccharide on Cyclophosphamide-Induced Immunosuppressed Mice Through MyD88-Dependent MAPK/NF-Kappa B and PI3K-Akt Signaling Pathways. Food Res Int (2021) 150(Pt A):110756. doi: 10.1016/j.foodres.2021.110756
9. Liu T, Wu Y, Wang L, Pang X, Zhao L, Yuan H, et al. A More Robust Gut Microbiota in Calorie-Restricted Mice Is Associated With Attenuated Intestinal Injury Caused by the Chemotherapy Drug Cyclophosphamide. mBio (2019) 10(2):02903–18. doi: 10.1128/mBio.02903-18
10. Shi H, Chang Y, Gao Y, Wang X, Chen X, Wang Y, et al. Dietary Fucoidan of Acaudina Molpadioides Alters Gut Microbiota and Mitigates Intestinal Mucosal Injury Induced by Cyclophosphamide. Food Funct (2017) 8(9):3383–93. doi: 10.1039/C7FO00932A
11. Huang R, Xie J, Liu X, Shen M. Sulfated Modification Enhances the Modulatory Effect of Yam Polysaccharide on Gut Microbiota in Cyclophosphamide-Treated Mice. Food Res Int (2021) 145:110393. doi: 10.1016/j.foodres.2021.110393
12. Chu Q, Zhang Y, Chen W, Jia R, Yu X, Wang Y, et al. Apios Americana Medik Flowers Polysaccharide (AFP) Alleviate Cyclophosphamide-Induced Immunosuppression in ICR Mice. Int J Biol Macromol (2020) 144:829–36. doi: 10.1016/j.ijbiomac.2019.10.035
13. Jie D, Gao T, Shan Z, Song J, Zhang M, Kurskaya O, et al. Immunostimulating Effect of Polysaccharides Isolated From Ma-Nuo-Xi Decoction in Cyclophosphamide-Immunosuppressed Mice. Int J Biol Macromol (2020) 146:45–52. doi: 10.1016/j.ijbiomac.2019.12.042
14. Li C, Duan S, Li Y, Pan X, Han L. Polysaccharides in Natural Products That Repair the Damage to Intestinal Mucosa Caused by Cyclophosphamide and Their Mechanisms: A Review. Carbohydr Polym (2021) 261:117876. doi: 10.1016/j.carbpol.2021.117876
15. Shu G, Xu D, Zhao J, Yin L, Lin J, Fu H, et al. Protective Effect of Polygonatum Sibiricum Polysaccharide on Cyclophosphamide-Induced Immunosuppression in Chickens. Res Vet Sci (2021) 135:96–105. doi: 10.1016/j.rvsc.2020.12.025
16. Chen X, Cai B, Wang J, Sheng Z, Yang H, Wang D, et al. Mulberry Leaf-Derived Polysaccharide Modulates the Immune Response and Gut Microbiota Composition in Immunosuppressed Mice. J Funct Food (2021) 83(25):104545. doi: 10.1016/j.jff.2021.104545
17. Chen X, Sun W, Xu B, Wu E, Cui Y, Hao K, et al. Polysaccharides From the Roots of Millettia Speciosa Champ Modulate Gut Health and Ameliorate Cyclophosphamide-Induced Intestinal Injury and Immunosuppression. Front Immunol (2021) 12:766296. doi: 10.3389/fimmu.2021.766296
18. Huang W, Tan H, Nie S. Beneficial Effects of Seaweed-Derived Dietary Fiber: Highlights of the Sulfated Polysaccharides. Food Chem (2022) 373(Pt B):131608. doi: 10.1016/j.foodchem.2021.131608
19. Chen Y, Jin H, Yang F, Jin S, Liu C, Zhang L, et al. Physicochemical, Antioxidant Properties of Giant Croaker (Nibea Japonica) Swim Bladders Collagen and Wound Healing Evaluation. Int J Biol Macromol (2019) 138:483–91. doi: 10.1016/j.ijbiomac.2019.07.111
20. Yu FM, He K, Dong XZ, Zhang ZW, Wang FL, Tang YP, et al. Immunomodulatory Activity of Low Molecular-Weight Peptides From Nibea Japonica Skin in Cyclophosphamide-Induced Immunosuppressed Mice. J Funct Food (2020) 68:103888. doi: 10.1016/j.jff.2020.103888
21. Li HY, Yi YL, Guo S, Zhang F, Yan H, Zhan ZL, et al. Isolation, Structural Characterization and Bioactivities of Polysaccharides From Laminaria Japonica: A Review. Food Chem (2021) 370:131010. doi: 10.1016/j.foodchem.2021.131010
22. Peng Y, Song Y, Wang Q, Hu Y, He Y, Ren D, et al. In Vitro and In Vivo Immunomodulatory Effects of Fucoidan Compound Agents. Int J Biol Macromol (2019) 127:48–56. doi: 10.1016/j.ijbiomac.2018.12.197
23. Hwang PA, Lin HTV, Lin HY, Lo SK. Dietary Supplementation With Low-Molecular-Weight Fucoidan Enhances Innate and Adaptive Immune Responses and Protects Against Mycoplasma Pneumoniae Antigen Stimulation. Mar Drugs (2019) 17(3):175. doi: 10.3390/md17030175
24. Sun TH, Zhang XH, Miao Y, Zhou Y, Shi J, Yan MX, et al. Studies on Antiviral and Immuno-Regulation Activity of Low Molecular Weight Fucoidan From. Laminaria Japonic J Ocean U China (2018) 17(3):705–11. doi: 10.1007/s11802-018-3794-1
25. Tian SS, Jiang XX, Tang YP, Han T. Laminaria Japonica Fucoidan Ameliorates Cyclophosphamide-Induced Liver and Kidney Injury Possibly by Regulating Nrf2/HO-1 and TLR4/NF-Kappa B Signaling Pathways. J Sci Food Agr (2021) 102(6):2604–12. doi: 10.1002/jsfa.11602
26. Han L, Lei H, Tian Z, Wang X, Cheng D, Wang C. The Immunomodulatory Activity and Mechanism of Docosahexenoic Acid (DHA) on Immunosuppressive Mice Models. Food Funct (2018) 9(6):3254–63. doi: 10.1039/C8FO00269J
27. Zhou YF, Tian SS, Qian L, Jiang S, Tang YP, Han T. DHA-Enriched Phosphatidylserine Ameliorates Non-Alcoholic Fatty Liver Disease and Intestinal Dysbacteriosis in Mice Induced by a High-Fat Diet. Food Funct (2021) 12(9):4021–33. doi: 10.1039/D0FO03471A
28. Liu ZB, Chen Q, Zhang C, Ni L. Comparative Study of the Anti-Obesity and Gut Microbiota Modulation Effects of Green Tea Phenolics and Their Oxidation Products in High-Fat-Induced Obese Mice. Food Chem (2022) 367:130735. doi: 10.1016/j.foodchem.2021.130735
29. Zhao Y, Yan Y, Zhou W, Chen D, Huang K, Yu S, et al. Effects of Polysaccharides From Bee Collected Pollen of Chinese Wolfberry on Immune Response and Gut Microbiota Composition in Cyclophosphamide-Treated Mice. J Funct Food (2020) 72(3):104057. doi: 10.1016/j.jff.2020.104057
30. Xia XW, Hao H, Zhang XY, Wong IN, Chung SK, Chen ZX, et al. Immunomodulatory Sulfated Polysaccharides From Caulerpa Racemosa Var. Peltata Induces Metabolic Shifts in NF-KB Signaling Pathway in RAW 264.7 Macrophages. Int J Biol Macromol (2021) 182:321–32. doi: 10.1016/j.ijbiomac.2021.04.025
31. Yu F, Zhang Z, Ye S, Hong X, Jin H, Huang F, et al. Immunoenhancement Effects of Pentadecapeptide Derived From Cyclina Sinensis on Immune-Deficient Mice Induced by Cyclophosphamide. J Funct Food (2019) 60(1):103408. doi: 10.1016/j.jff.2019.06.010
32. Zhu G, Jiang Y, Yao Y, Wu N, Luo J, Hu M, et al. Ovotransferrin Ameliorates the Dysbiosis of Immunomodulatory Function and Intestinal Microbiota Induced by Cyclophosphamide. Food Funct (2019) 10(2):1109–22. doi: 10.1039/C8FO02312C
33. Zhao S, Peng X, Zhou Q, Huang Y, Rao X, Tu J, et al. Bacillus Coagulans 13002 and Fructo-Oligosaccharides Improve the Immunity of Mice With Immunosuppression Induced by Cyclophosphamide Through Modulating Intestinal-Derived and Fecal Microbiota. Food Res Int (2021) 140:109793. doi: 10.1016/j.foodres.2020.109793
34. Liu Y, Wu X, Wang Y, Jin W, Guo Y. The Immunoenhancement Effects of Starfish Asterias Rollestoni Polysaccharides in Macrophages and Cyclophosphamide-Induced Immunosuppression Mouse Models. Food Funct (2020) 11(12):10700–8. doi: 10.1039/D0FO01488E
35. Ma T, Li C, Zhao F, Cao J, Zhang X, Shen X. Effects of Co-Fermented Collagen Peptide-Jackfruit Juice on the Immune Response and Gut Microbiota in Immunosuppressed Mice. Food Chem (2021) 365:130487. doi: 10.1016/j.foodchem.2021.130487
36. Shi J, Zhang Q, Zhao X-H, Wang L. The Impact of Caseinate Oligochitosan-Glycation by Transglutaminase on Amino Acid Compositions and Immune-Promoting Activity in BALB/c Mice of the Tryptic Caseinate Hydrolysate. Food Chem (2021) 350:129302. doi: 10.1016/j.foodchem.2021.129302
37. Jiang S, Zhang Z, Huang F, Yang Z, Yu F, Tang Y, et al. Protective Effect of Low Molecular Weight Peptides From Solenocera Crassicornis Head Against Cyclophosphamide-Induced Nephrotoxicity in Mice via the Keap1/Nrf2 Pathway. Antioxidants (2020) 9(8):745. doi: 10.3390/antiox9080745
38. Han L, Meng M, Guo M, Cheng D, Shi L, Wang X, et al. Immunomodulatory Activity of a Water-Soluble Polysaccharide Obtained From Highland Barley on Immunosuppressive Mice Models. Food Funct (2019) 10(1):304–14. doi: 10.1039/C8FO01991F
39. Geuking MB, Koller Y, Rupp S, McCoy KD. The Interplay Between the Gut Microbiota and the Immune System. Gut Microbes (2014) 5(3):411–8. doi: 10.4161/gmic.29330
Keywords: fucoidan, cyclophosphamide, immunosuppression, immunomodulatory, gut microbiota
Citation: Tang Y, Pu Q, Zhao Q, Zhou Y, Jiang X and Han T (2022) Effects of Fucoidan Isolated From Laminaria japonica on Immune Response and Gut Microbiota in Cyclophosphamide-Treated Mice. Front. Immunol. 13:916618. doi: 10.3389/fimmu.2022.916618
Received: 09 April 2022; Accepted: 25 April 2022;
Published: 19 May 2022.
Edited by:
Bin Du, Hebei Normal University of Science and Technology, ChinaReviewed by:
Fei Peng, Hebei Normal University of Science and Technology, ChinaXiaoting Fu, Ocean University of China, China
Copyright © 2022 Tang, Pu, Zhao, Zhou, Jiang and Han. This is an open-access article distributed under the terms of the Creative Commons Attribution License (CC BY). The use, distribution or reproduction in other forums is permitted, provided the original author(s) and the copyright owner(s) are credited and that the original publication in this journal is cited, in accordance with accepted academic practice. No use, distribution or reproduction is permitted which does not comply with these terms.
*Correspondence: Yunping Tang, dGFuZ3l1bnBpbmcxOTg1QDE2My5jb20=; Tao Han, Z29vZGhhbnRhb0BnbWFpbC5jb20=