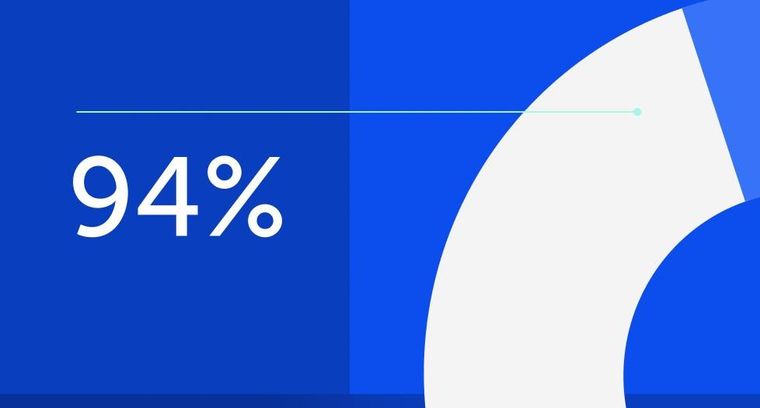
94% of researchers rate our articles as excellent or good
Learn more about the work of our research integrity team to safeguard the quality of each article we publish.
Find out more
PERSPECTIVE article
Front. Immunol., 30 August 2022
Sec. Viral Immunology
Volume 13 - 2022 | https://doi.org/10.3389/fimmu.2022.904342
This article is part of the Research TopicInsights in Viral Immunology: 2021View all 11 articles
The clinical handling of chronic virus infections remains a challenge. Here we describe recent progress in the understanding of virus - host interaction dynamics. Based on the systems biology concept of multi-stability and the prediction of multiplicative cooperativity between virus-specific cytotoxic T cells and neutralising antibodies, we argue for the requirements to engage multiple immune system components for functional cure strategies. Our arguments are derived from LCMV model system studies and are translated to HIV-1 infection.
Chronic virus infections like those with Human Immunodeficiency Viruses (HIV) and Hepatitis B (HBV) and C (HCV) viruses continue to threaten global health. A common feature of these infections is the persistence of virus antigen and the associated exhaustion of virus-specific T lymphocytes (1–5). Although the latter reduces immune-cell-mediated pathology, it is associated with a reduction of virus control that enables antigen persistence and has per se pathological consequences. For example, untreated HIV infection mediates CD4 T cell depletion, chronic immune activation, lymphoid tissue destruction and dysregulation of immune homeostasis (6, 7). Chronic hepatitis virus infections deteriorate liver functions and can lead to liver cirrhosis and hepatocellular carcinoma (8–10). Globally these 3 infections are carried by close to 400 million individuals and thus are a significant burden for public health care systems (11).
The existing approaches to treat chronic infections may be subdivided into 2 fundamentally different categories, (i) targeting of virus replication by antiviral drugs including interferons or therapeutic target cell modifications, and (ii) targeting virus-specific immune responses to improve host control by restoration of immune functions i.e. through therapeutic vaccination or immune checkpoint inhibitors that reinvigorate exhausted lymphocytes. While most of these options are still experimental, antiviral drugs are by far the most common therapeutic modality in use and very successful. For example, the current virus-specific anti-HCV drugs are highly potent and enable virus clearance in around 95% of infected individuals (12). Current antivirals against HIV can reduce virus loads to below detectable levels however fail to eliminate the latently infected cells (13, 14). As a consequence, treatment interruptions lead to rapid viral rebounds from viral reservoirs and the continuation of a high viral load infection state (15). To overcome the need for life-long antiviral HIV therapy with its side effects and the inherent financial burden for health care systems, numerous concepts for curing chronic infections have been developed and experimentally tested. These include “shock-and-kill” strategies that aim to purge the latent virus reservoir by latency reversal agents with subsequent killing of infected cells (16, 17), “block-and-lock” strategies that aim to permanently silence all HIV proviruses (18) and the potential “rinse-and-replace” strategy that predicts a “washing-out” of infected cells by uninfected naive and memory T cells via sequential waves of polyclonal T cell stimulation under combination antiretroviral therapy (19). While still being far from clinical practice, the combined delivery of broadly neutralising antibodies or CD8 T cell-inducing therapeutic vaccines with latency-reversal-agents (LRAs) including TLR7 agonists showed encouraging first results in experimental SIV/SHIV infections of rhesus monkeys (20, 21) and in humans (22).
Cure strategies for chronic virus infections can be divided into sterilising and functional cure strategies. While the former attempts to completely eliminate the virus from the host e.g. by HIV-resistant hematopoietic stem cell transplantation (23, 24) or provirus deletion approaches (25–27), the latter solely aims to shift the dynamic virus - host immune system balance into a state in which the virus is sufficiently controlled without causing pathology (Figure 1A). Given that HIV elimination was only successful in possibly 4 cases worldwide with a procedure that has a high mortality rate (30), functional cure approaches appear more feasible. However, is there any evidence that a shift from a high-virus-load to a low-virus-load equilibrium is possible? And what would the requirements be for such a shift? Here, we discuss the evidence that indeed such a shift should be possible and define the conditions under which it may occur.
Figure 1 High and low virus load (VL) equilibrium states in persistent chronic virus infections. (A) Schematic presentation of a high and low virus load state, and the associated pathology. (B) Mathematical model predictions of multiple virus equilibrium states (I to IV) in LCMV infections of mice as a function of the net virus growth rate β. Data are from (28). The four virus equilibrium states differ in their values of the virus load from the highest (state I) to the lowest (state IV). Stability of an equilibrium state or steady state means that the system returns back after some perturbation. Only stable equilibrium states are biologically observable. State I is always stable while state III is stable for a certain range of β (solid lines). States II and IV are unstable (broken lines) and cannot be observed biologically. A possible reduction of β by neutralising antibodies is indicated below. It represents the natural occurrence of a late, specific neutralizing antibody response during chronic LCMV infection that reduces the net virus growth rate represented by β. (C) Evolution of virus neutralising capacity of mouse sera during an experimental LCMV infection of mice. Experimental data are from (29) and converted into this presentation. Medians of viral loads at days 30 and 70 and their interquartile ranges are indicated in red. Solid line, sera from chronic infection; broken line, sera from acute infection.
Many features of virus - immune system interactions within the lymphocytic choriomeningitis virus (LCMV) mice model system resemble those of human chronic HIV and hepatitis virus infections (31, 32). In the early stages, LCMV infections are mainly controlled by CTLs. Infection with a low dose of e.g. LCMV-Docile or a high dose of LCMV-Arm leads to the clearance of virus below the detection level and formation of immune memory (33). In contrast, infection with high doses of LCMV-Docile or LCMV-Cl13 results in chronic viral persistence at high levels and exhaustion of antigen-specific cytotoxic CD8 T cells (CTLs) (1, 34). Nonetheless, they differ in their long-term kinetics of infections i.e. clearance of LCMV Clone 13 by late neutralizing antibodies versus persistence of LCMV Docile (1).
To explore the necessary conditions for the co-existence of virus-host equilibria that differ in viral loads as well as the possibilities for transferring a high-viral load state to a more favourable equilibrium, one can utilise the analytical power of existing mathematical models that have been calibrated using experimental data. Our previously developed mathematical model of LCMV infection considers the population dynamics of viruses, precursor and effector CTLs (35), and utilises LCMV data assimilation procedures and bifurcation analysis (36). The results suggested that the reduction in the net viral growth rate β is a necessary condition for a stable low level LCMV infection state within an immunocompetent host (Figure 1B). Specifically, the existence of replication competent LCMV below the detection limit of about 100 FFU per spleen in immune mice requires a more than 2-times reduction of the exponential virus growth rate of the acute infection phase. Given that LCMV-specific neutralising antibodies (nAbs) can block free virus particles and thus reduce the net virus growth rate, it was hypothesised that such antibodies could be decisive for virus control. And indeed, subsequent experimental work by Greczmiel et al. demonstrated that it is the late appearance of nAbs that finally controls a chronic LCMV infection to below detectable levels (37) (Figure 1C).
A conceptual dynamic view of the above observations is summarized in Figure 2A which considers the outcome of virus-host interactions as a ‘numbers game’ between the rate of infection growth and the activation of the immune system (38). If the virus outcompetes the CTL response, a high virus load state is established that is characterised by T cell exhaustion and maintained through the interaction of inhibitory receptors on T cells with their ligands on antigen-presenting cells (APCs) (5). However, this harmful equilibrium can be shifted in favour of the host by inducing a virus-specific neutralising antibody response or by providing antibodies as a therapeutic intervention (37, 39). Since the cooperativity of remaining CTLs and the newly induced antibody response can be considered as multiplicative rather than just additive, the demand for both specific immune response components is less stringent in absolute numbers (40).
Figure 2 Dynamic views of virus-host interactions. Lines with an arrow or T end represent expansion or reduction/suppression of the corresponding virus or cell populations, respectively. The specific processes by which this occurs are specified above the lines. (A) Conceptual dynamic view of a high and a low virus load state within an infected host. The underlying processes are indicated. A high VL state drives CTL exhaustion (grey cells) and reduces the population of effector CTL (green cells). B cells (blue cells) produce antibodies that eliminate infectious viruses. Both, effector CTL and antibodies from B cells, depending on their strength, contribute to the control of the virus load. (B) Cooperative engagement of individual immune components for functional cure strategies in HIV-1 infection. The combination of immune checkpoint inhibitors with CTL-based immunotherapy and neutralising antibody responses is indicated. aPDL1, anti-PDL1 antibodies; CTL, cytotoxic T cells; CTLex, exhausted CTL; CD4i, infected CD4 T cells; VL, virus load. The figure was created with BioRender.com.
To translate these results and considerations from the LCMV mouse model system to human infections like HIV infections and functional cure strategies, two fundamental questions arise: Is there evidence for different dynamic steady states in HIV infection? How can one reduce viral growth rate β and restore functional immune control in a therapeutic setting? Answer to the first question is yes. There are several different dynamic states that can be defined by viral loads and disease progression rates (41). These are related to the virus set point, the dynamic equilibrium state at which the virus settles after the primary infection phase (42–44). For example, from the infected individuals in which the virus load settles to above 36,000 virions/ml blood, more than 62% will develop AIDS within 5 years. In contrast, only 8% of individuals with a virus set point below 4,500 virions/ml blood will develop AIDS in this time frame. Thus, at least in HIV-1 infection, virus loads are directly linked to pathology and the low virus load stage, as observed in so-called “elite controllers” (EC) (45, 46), may be regarded as a non-pathogenic virus infection state that should be the target for functional cure strategies (Figure 1A). Indeed, elite controllers are exceptional HIV-infected individuals that control virus replication without the requirement of antiretroviral therapy. Based on studies during their chronic steady state, many potential immunological and virological factors have been linked to this. It includes virus-specific CTLs and CD4 T cells, innate immune responses like NK cells and plasmacytoid dendritic cells as well as virus attenuation and provirus integration into repressed chromatin sites (47–49). Since the EC state is associated with certain HLA types, and the antiviral functionality of CTLs from EC against HIV-infected autologous CD4 T cells is superior to those of HIV non-controllers (50), HIV-specific CTL seem to be a prime component in achieving virus control. The role of virus-specific neutralizing antibody responses in EC is less clear as high titres of these are often lacking in respective individuals (47).
The answer to the second question is much more demanding because one needs to consider the state of the whole immune system and virus population at the time the functional cure strategy is to be initiated. In the chronic infection state in which T cells are exhausted (5) and the virus is a complex quasispecies population (51, 52) and partially hiding within latently infected cells (53, 54), the simple reduction of the virus growth rate by antiviral drugs is not sufficient to self-maintain a stable low virus load state. Likewise, it is not sufficient to invigorate exhausted T and B cells by checkpoint inhibitors in the presence of antiviral drugs because only a fraction of HIV disease phenotypes would benefit. This was demonstrated in our modelling study of anti-PD-L1 blockage in HIV-1 infection (55). In this we showed that a favourable effect in terms of viral load reduction and restoration of functional T cells strongly depends on the antibody-mediated elimination rate of infectious virus in a threshold dependent manner. Furthermore, taking into account spatial aspects of HIV-1 infection spreading within lymphatic tissue and CTL motility, we estimated that the minimum frequency of HIV-specific effector CTLs should be above 5% to ensure localisation and elimination of an infected cell within a virus life cycle time (56). Recent vaccine studies against simian–human immunodeficiency virus (SHIV) infection in macaques demonstrated that the threshold requirements for virus infection control were much lower when neutralizing antibodies and CTLs were induced (57) suggesting multiplicative cooperation between both arms of the adaptive immune system (40). Taken together and considering the multiplicative cooperativity between cellular and humoral responses, it would appear that only a multi-modal empowerment of antiviral immunity could enable a permanent shift from a high virus load to a low virus load state in HIV-1 infection. This would require invigoration of exhausted T cells by checkpoint inhibitors in the presence of antiviral drugs (58) and induction of novel CTLs together with neutralising antibodies that cover a broad spectrum of viral epitopes (Figure 2B).
Here we summarise the evidence for multiple stable virus load states in persistent chronic virus infections and provide a perspective for a functional cure.
Multi-stability is a relevant property of complex biological systems with normal feedback regulation (59) to which virus infections belong to. It provides the possibility of switching between different virus load states. Computational models are helpful in this context as they can identify the required parameter values for the multiple steady states (28). For example, a 2-times reduction of the net virus growth rate β is the necessary condition for the existence of the low virus load state in persistent LCMV infection (Figure 1B). Once this condition is identified, the challenge becomes to define the required manipulations for shifting the whole system to that favourable state. Again, computational approaches can provide useful insight. Amongst them is the recently developed optimal disturbance approach which can predict multi-modal impacts (combination therapies) with maximal effects on the immune system (60). When applied to LCMV infection, the results demonstrated that a systems shift is possible and requires a combination of different initial state perturbations like virus load and functional T cell state. While this will translate to a combination therapy e.g. of checkpoint inhibitors and neutralising antibodies, the respective quantities and time lines are not yet determined and await experimental elaboration. Nonetheless, these mathematically-driven experimental LCMV system-based studies provide a proof-of-concept for a possible system shift to a favourable virus-host interaction dynamics. Respective analyses of multi-stability and optimal disturbances for HIV-1 or other persistent chronic infections in humans are still lacking and clearly represent a challenge and direction for further interdisciplinary research. The recent progress of immunotherapies to induce and boost antiviral immunity are encouraging but also highlight the need to cooperatively engage individual immune system components that may eventually allow moving from a drug-based virus containment to a long-term immune system-based functional cure.
In summary, the currently explored strategies for functionally curing an HIV infection are “shock-and-kill”, “block-and-lock” and “rinse-and-replace”. None of them considers and explores the concept of multiplicative cooperativity between individual immune system components that is proposed here. A proof of concept in a clinical setting is eagerly waited for.
The original contributions presented in the study are included in the article/supplementary material. Further inquiries can be directed to the corresponding authors.
All authors listed have made a substantial, direct and intellectual contribution to the work, and approved it for publication. All authors contributed to the article and approved the submitted version.
The authors are supported by the Russian Science Foundation (RSF grant no. 18-11-00171), the Russian Foundation of Basic Research (RFBR grant no. 20-01-00352), “la Caixa” Foundation under the project code HR17-00199, the Spanish Ministry of Science and Innovation grant no. PID2019-106323RB-I00 AEI//10.13039/501100011033, and “Unidad de Excelencia María de Maeztu”, funded by the MCIN and the AEI (DOI: 10.13039/501100011033) Ref: CEX2018-000792-M.
The authors declare that the research was conducted in the absence of any commercial or financial relationships that could be construed as a potential conflict of interest.
All claims expressed in this article are solely those of the authors and do not necessarily represent those of their affiliated organizations, or those of the publisher, the editors and the reviewers. Any product that may be evaluated in this article, or claim that may be made by its manufacturer, is not guaranteed or endorsed by the publisher.
1. Moskophidis D, Lechner F, Pircher H, Zinkernagel RM. Virus persistence in acutely infected immunocompetent mice by exhaustion of antiviral cytotoxic effector T cells. Nature (1993) 362:758–61. doi: 10.1038/362758a0
2. Day CL, Kaufmann DE, Kiepiela P, Brown JA, Moodley ES, Reddy S, et al. PD-1 expression on HIV-specific T cells is associated with T-cell exhaustion and disease progression. Nature (2006) 443:350–4. doi: 10.1038/nature05115
3. Boni C, Fisicaro P, Valdatta C, Amadei B, Vincenzo PD, Giuberti T, et al. Characterization of hepatitis B virus (HBV)-specific T-cell dysfunction in chronic HBV infection. J Virol (2007) 81:4215–25. doi: 10.1128/jvi.02844-06
4. Bengsch B, Seigel B, Ruhl M, Timm J, Kuntz M, Blum HE, et al. Coexpression of PD-1, 2B4, CD160 and KLRG1 on exhausted HCV-specific CD8+ T cells is linked to antigen recognition and T cell differentiation. PLoS Pathog (2010) 6:e1000947. doi: 10.1371/journal.ppat.1000947
5. Wherry EJ, Kurachi M. Molecular and cellular insights into T cell exhaustion. Nat Rev Immunol (2015) 15:486–99. doi: 10.1038/nri3862
7. Lackner AA, Lederman MM, Rodriguez B. HIV Pathogenesis: The host. Csh Perspect Med (2012) 2:a007005. doi: 10.1101/cshperspect.a007005
8. Liang TJ, Rehermann B, Seeff LB, Hoofnagle JH. Pathogenesis, natural history, treatment, and prevention of hepatitis C. Ann Intern Med (2000) 132:296. doi: 10.7326/0003-4819-132-4-200002150-00008
9. Chisari FV, Isogawa M, Wieland. SF. Pathogenesis of hepatitis b virus infection. Pathol Biol (2010) 58:258–66. doi: 10.1016/j.patbio.2009.11.001
10. Iannacone M, Guidotti LG. Immunobiology and pathogenesis of hepatitis B virus infection. Nat Rev Immunol (2022) 22:19–32. doi: 10.1038/s41577-021-00549-4
11. Global progress report on HIV, viral hepatitis and sexually transmitted infections. In: Accountability for the global health sector strategies 2016–2021: actions for impact. Geneva: World Health Organization. Available at: https://www.who.int/publications/i/item/9789240027077.
12. Villani R, Monami M, Cosimo FD, Fioravanti G, Mannucci E, Vendemiale G, et al. Direct-acting antivirals for HCV treatment in older patients: A systematic review and meta-analysis. J Viral Hepat (2019) 26:1249–56. doi: 10.1111/jvh.13169
13. Cohn LB, Chomont N, Deeks SG. The biology of the HIV-1 latent reservoir and implications for cure strategies. Cell Host Microbe (2020) 27:519–30. doi: 10.1016/j.chom.2020.03.014
14. Saag MS, Gandhi RT, Hoy JF, Landovitz RJ, Thompson MA, Sax PE, et al. Antiretroviral drugs for treatment and prevention of HIV infection in adults. JAMA (2020) 324:1651–69. doi: 10.1001/jama.2020.17025
15. Wen Y, Bar KJ, Li JZ. Lessons learned from HIV antiretroviral treatment interruption trials. Curr Opin HIV AIDS (2018) 13:416–21. doi: 10.1097/coh.0000000000000484
17. Siliciano JD, Siliciano RF. In vivo dynamics of the latent reservoir for HIV-1: New insights and implications for cure. Annu Rev Pathol Mech Dis (2021) 17:1–24. doi: 10.1146/annurev-pathol-050520-112001
18. Vansant G, Bruggemans A, Janssens J, Debyser Z. Block-And-Lock strategies to cure HIV infection. Viruses (2020) 12:84. doi: 10.3390/v12010084
19. Grossman Z, Singh NJ, Simonetti FR, Lederman MM, Douek DC, Deeks SG, et al. ‘Rinse and replace’: Boosting T cell turnover to reduce HIV-1 reservoirs. Trends Immunol (2020) 41:466–80. doi: 10.1016/j.it.2020.04.003
20. Borducchi EN, Cabral C, Stephenson KE, Liu J, Abbink P, Ng’ang’a D, et al. Ad26/MVA therapeutic vaccination with TLR7 stimulation in SIV-infected rhesus monkeys. Nature (2016) 540:284–7. doi: 10.1038/nature20583
21. Borducchi EN, Liu J, Nkolola JP, Cadena AM, Yu W-H, Fischinger S, et al. Antibody and TLR7 agonist delay viral rebound in SHIV-infected monkeys. Nature (2018) 563:360–4. doi: 10.1038/s41586-018-0600-6
22. Mothe B, Rosás-Umbert M, Coll P, Manzardo C, Puertas MC, Morón-López S, et al. HIVconsv vaccines and romidepsin in early-treated HIV-1-Infected individuals: Safety, immunogenicity and effect on the viral reservoir (Study BCN02). Front Immunol (2020) 11:823. doi: 10.3389/fimmu.2020.00823
23. Hütter G, Nowak D, Mossner M, Ganepola S, Müßig A, Allers K, et al. Long-term control of HIV by CCR5 Delta32/Delta32 stem-cell transplantation. New Engl J Med (2009) 360:692–8. doi: 10.1056/nejmoa0802905
24. Gupta RK, Abdul-Jawad S, McCoy LE, Mok HP, Peppa D, Salgado M, et al. HIV-1 remission following CCR5Δ32/Δ32 haematopoietic stem-cell transplantation. Nature (2019) 568:244–8. doi: 10.1038/s41586-019-1027-4
25. Mancuso P, Chen C, Kaminski R, Gordon J, Liao S, Robinson JA, et al. CRISPR based editing of SIV proviral DNA in ART treated non-human primates. Nat Commun (2020) 11:6065. doi: 10.1038/s41467-020-19821-7
26. Excision BioTherapeutics (U.S.). (2022, January -). study of EBT-101 in aviremic HIV-1 infected adults on stable ART. Available at: https://clinicaltrials.gov/ct2/show/NCT05144386.
27. Sarkar I, Hauber I, Hauber J, Buchholz F. HIV-1 proviral DNA excision using an evolved recombinase. Science (2007) 316:1912–5. doi: 10.1126/science.1141453
28. Nechepurenko Y, Khristichenko M, Grebennikov D, Bocharov G. Bistability analysis of virus infection models with time delays. Discret Contin Dyn Syst - S (2020) 13:2385–401. doi: 10.3934/dcdss.2020166
29. Kräutler NJ, Yermanos A, Pedrioli A, Welten SPM, Lorgé D, Greczmiel U, et al. Quantitative and qualitative analysis of humoral immunity reveals continued and personalized evolution in chronic viral infection. Cell Rep (2020) 30:997–1012.e6. doi: 10.1016/j.celrep.2019.12.088
30. Styczyński J, Tridello G, Koster L, Iacobelli S, van Biezen A, van der Werf S, et al. Death after hematopoietic stem cell transplantation: changes over calendar year time, infections and associated factors. Bone Marrow Transpl (2020) 55:126–36. doi: 10.1038/s41409-019-0624-z
31. Klenerman P, Zinkernagel RM. What can we learn about human immunodeficiency virus infection from a study of lymphocytic choriomeningitis virus? Immunol Rev (1997) 159:5–16. doi: 10.1111/j.1600-065x.1997.tb01003.x
32. Abdel-Hakeem MS. Viruses teaching immunology: Role of LCMV model and human viral infections in immunological discoveries. Viruses (2019) 11:106. doi: 10.3390/v11020106
33. Ciurea A, Klenerman P, Hunziker L, Horvath E, Odermatt B, Ochsenbein AF, et al. Persistence of lymphocytic choriomeningitis virus at very low levels in immune mice. Proc Natl Acad Sci (1999) 96:11964–9. doi: 10.1073/pnas.96.21.11964
34. Zajac AJ, Blattman JN, Murali-Krishna K, Sourdive DJD, Suresh M, Altman JD, et al. Viral immune evasion due to persistence of activated T cells without effector function. J Exp Med (1998) 188:2205–13. doi: 10.1084/jem.188.12.2205
35. Bocharov GA. Modelling the dynamics of LCMV infection in mice: Conventional and exhaustive CTL responses. J Theor Biol (1998) 192:283–308. doi: 10.1006/jtbi.1997.0612
36. Luzyanina T, Engelborghs K, Ehl S, Klenerman P, Bocharov G. Low level viral persistence after infection with LCMV: A quantitative insight through numerical bifurcation analysis. Math Biosci (2001) 173:1–23. doi: 10.1016/s0025-5564(01)00072-4
37. Greczmiel U, Kräutler NJ, Pedrioli A, Bartsch I, Agnellini P, Bedenikovic G, et al. Sustained T follicular helper cell response is essential for control of chronic viral infection. Sci Immunol (2017) 2(18):eaam8686. doi: 10.1126/sciimmunol.aam8686
38. Zinkernagel RM, Hengartner H, Stitz L. ON THE ROLE OF VIRUSES IN THE EVOLUTION OF IMMUNE RESPONSES. Brit Med Bull (1985) 41:92–7. doi: 10.1093/oxfordjournals.bmb.a072033
39. Ertuna YI, Fallet B, Marx A-F, Dimitrova M, Kastner AL, Wagner I, et al. Vectored antibody gene delivery restores host B and T cell control of persistent viral infection. Cell Rep (2021) 37:110061. doi: 10.1016/j.celrep.2021.110061
40. Bocharov G, Grebennikov D, Argilaguet J, Meyerhans A. Examining the cooperativity mode of antibody and CD8+ T cell immune responses for vaccinology. Trends Immunol (2021) 42(10):852–5. doi: 10.1016/j.it.2021.08.003
41. Gurdasani D, Iles L, Dillon DG, Young EH, Olson AD, Naranbhai V, et al. A systematic review of definitions of extreme phenotypes of HIV control and progression. Aids (2014) 28:149–62. doi: 10.1097/qad.0000000000000049
42. Mellors JW, Rinaldo CR Jr, Gupta P, White RM, Todd JA, Kingsley LA. Prognosis in HIV-1 infection predicted by the quantity of virus in plasma. Science (1996) 272:1167–70. doi: 10.1126/science.272.5265.1167
43. Ho DD. Viral counts count in HIV infection. Science (1996) 272:1124–5. doi: 10.1126/science.272.5265.1124
44. Robb ML, Eller LA, Kibuuka H, Rono K, Maganga L, Nitayaphan S, et al. Prospective study of acute HIV-1 infection in adults in East Africa and Thailand. New Engl J Med (2016) 374:2120–30. doi: 10.1056/nejmoa1508952
45. Baker B, Block B, Rothchild A, Walker B. Elite control of HIV infection: implications for vaccine design. Expert Opin Biol Th (2008) 9:55–69. doi: 10.1517/14712590802571928
46. Moyano A, Ndungu T, Mann JK. Determinants of natural HIV-1 control. AIDS Rev (2022) 24(2):51–8. doi: 10.24875/aidsrev.21000048
47. Deeks SG, Walker BD. Human immunodeficiency virus controllers: mechanisms of durable virus control in the absence of antiretroviral therapy. Immunity (2007) 27(3):406–16. doi: 10.1016/j.immuni.2007.08.010
48. Navarrete-Muñoz MA, Restrepo C, Benito JM, Rallón N. Elite controllers: A heterogeneous group of HIV-infected patients. Virulence (2020) 11(1):889–97. doi: 10.1080/21505594.2020.1788887
49. Jiang C, Lian X, Gao C, Sun X, Einkauf KB, Chevalier JM, et al. Distinct viral reservoirs in individuals with spontaneous control of HIV-1. Nature (2020) 585(7824):261–7. doi: 10.1038/s41586-020-2651-8
50. Domingo E, García-Crespo C, Perales C. Historical perspective on the discovery of the quasispecies concept. Ann Rev Virol (2021) 8:51–72. doi: 10.1146/annurev-virology-091919-105900
51. Chun T-W, Fauci AS. Latent reservoirs of HIV: Obstacles to the eradication of virus. Proc Natl Acad Sci (1999) 96:10958–61. doi: 10.1073/pnas.96.20.10958
52. Siliciano RF, Greene WC. HIV Latency. Csh Perspect Med (2011) 1:a007096. doi: 10.1101/cshperspect.a007096
53. Zheltkova V, Argilaguet J, Peligero C, Bocharov G, Meyerhans A. Prediction of PD-L1 inhibition effects for HIV-infected individuals. PLoS Comput Biol (2019) 15:e1007401. doi: 10.1371/journal.pcbi.1007401
54. Grebennikov D, Bouchnita A, Volpert V, Bessonov N, Meyerhans A, Bocharov G. Spatial lymphocyte dynamics in lymph nodes predicts the cytotoxic T cell frequency needed for HIV infection control. Front Immunol (2019) 10:1213. doi: 10.3389/fimmu.2019.01213
55. Sáez-Cirión A, Lacabaratz C, Lambotte O, Versmisse P, Urrutia A, Boufassa F, et al. HIV Controllers exhibit potent CD8 T cell capacity to suppress HIV infection ex vivo and peculiar cytotoxic T lymphocyte activation phenotype. Proc Natl Acad Sci USA (2007) 104(16):6776–81. doi: 10.1073/pnas.0611244104
56. Meyerhans A, Cheynier R, Albert J, Seth M, Kwok S, Sninsky J, et al. Temporal fluctuations in HIV quasispecies in vivo are not reflected by sequential HIV isolations. Cell (1989) 58:901–10. doi: 10.1016/0092-8674(89)90942-2
57. Arunachalam PS, Charles TP, Joag V, Bollimpelli VS, Scott MKD, Wimmers F, et al. Pulendran b. T cell-inducing vaccine durably prevents mucosal SHIV infection even with lower neutralizing antibody titers. Nat Med (2020) 26(6):932–40. doi: 10.1038/s41591-020-0858-8
58. Peligero C, Argilaguet J, Güerri-Fernandez R, Torres B, Ligero C, Colomer P, et al. PD-L1 blockade differentially impacts regulatory T cells from HIV-infected individuals depending on plasma viremia. PLoS Pathog (2015) 11:e1005270. doi: 10.1371/journal.ppat.1005270
59. Angeli D, Ferrell JE, Sontag ED. Detection of multistability, bifurcations, and hysteresis in a large class of biological positive-feedback systems. Proc Natl Acad Sci (2004) 101:1822–7. doi: 10.1073/pnas.0308265100
Keywords: Chronic virus infections, multi-stability, shifting equilibrium states, cure strategies, LCMV (lymphocytic choriomeningitis virus), HIV (human immunodeficiency virus)
Citation: Bocharov G, Grebennikov D, Cebollada Rica P, Domenjo-Vila E, Casella V and Meyerhans A (2022) Functional cure of a chronic virus infection by shifting the virus - host equilibrium state. Front. Immunol. 13:904342. doi: 10.3389/fimmu.2022.904342
Received: 25 March 2022; Accepted: 12 August 2022;
Published: 30 August 2022.
Edited by:
Eric Hunter, Emory University, United StatesReviewed by:
Juan C. De La Torre, The Scripps Research Institute, United StatesCopyright © 2022 Bocharov, Grebennikov, Cebollada Rica, Domenjo-Vila, Casella and Meyerhans. This is an open-access article distributed under the terms of the Creative Commons Attribution License (CC BY). The use, distribution or reproduction in other forums is permitted, provided the original author(s) and the copyright owner(s) are credited and that the original publication in this journal is cited, in accordance with accepted academic practice. No use, distribution or reproduction is permitted which does not comply with these terms.
*Correspondence: Gennady Bocharov, Z2JvY2hhcm92QGdtYWlsLmNvbQ==; Andreas Meyerhans, YW5kcmVhcy5tZXllcmhhbnNAdXBmLmVkdQ==
Disclaimer: All claims expressed in this article are solely those of the authors and do not necessarily represent those of their affiliated organizations, or those of the publisher, the editors and the reviewers. Any product that may be evaluated in this article or claim that may be made by its manufacturer is not guaranteed or endorsed by the publisher.
Research integrity at Frontiers
Learn more about the work of our research integrity team to safeguard the quality of each article we publish.