- 1Clinical Research Development Center, Taleghani and Imam Ali Hospital, Kermanshah University of Medical Sciences, Kermanshah, Iran
- 2Medical Biology Research Center, Health Technology Institute, University of Medical Sciences, Kermanshah, Iran
- 3Medical Technology Research Center (MTRC), School of Medicine, Kermanshah University of Medical Sciences, Kermanshah, Iran
- 4Department of Cellular and Molecular Medicine, The Panum Institute, Faculty of Health and Medical Sciences, University of Copenhagen, Copenhagen, Denmark
- 5Physical Medicine and Rehabilitation Research Center, Aging Research Institute, Tabriz University of Medical Sciences, Tabriz, Iran
- 6Instituto de Investigación Sanitaria de Santiago (IDIS), Complejo Hospitalario Universitario de Santiago (CHUS), Servicio Gallego de Salud (SERGAS), Santiago de Compostela, Spain
- 7Research Center for Molecular Medicine, Hamadan University of Medical Sciences, Hamadan, Iran
- 8Translational Type 1 Diabetes Research, Department of Clinical, Research, Steno Diabetes Center Copenhagen, Gentofte, Denmark
- 9Interventional Regenerative Medicine and Imaging Laboratory, Department of Radiology, Stanford University School of Medicine, Palo Alto, CA, United States
Type 1 diabetes (T1D) is a chronic disorder characterized by immune-mediated destruction of pancreatic insulin-producing β-cells. The primary treatment for T1D is multiple daily insulin injections to control blood sugar levels. Cell-free delivery packets with therapeutic properties, extracellular vesicles (EVs), mainly from stem cells, have recently gained considerable attention for disease treatments. EVs provide a great potential to treat T1D ascribed to their regenerative, anti-inflammatory, and immunomodulatory effects. Here, we summarize the latest EV applications for T1D treatment and highlight opportunities for further investigation.
1 Introduction
Type 1 diabetes (T1D) is caused by immune-mediated destruction of the insulin-producing β-cells resulting in a life-long insulin dependence (1). T1D can occur at any age but mostly arises already at a young age (2). The incidence of T1D has increased through the past three decades worldwide (3).
To establish stable glycemic control, patients with T1D need multiple daily insulin (MDI) injections or continuous insulin infusion through a pump (4). Despite technological advances, unstable glycemic control in patients continues to be a risk factor for diabetes-related metabolic and vascular complications (5, 6). Therefore, novel interventions are needed for the T1D treatment. Protecting and regenerating β-cell mass and improving insulin-producing capacity should be the primary purposes of any in-development and future T1D treatments.
Surgical islet transplantation and/or whole pancreas transplantation are the only current treatments for restoring the β-cells (7). Islet transplantation faces a limited source of islet donors and immune destruction after transplantation, requiring immunosuppression therapy (8, 9).
Ascribed to the autoimmune nature of T1D, immunotherapies aiming at the suppression/regulation of T cells or proinflammatory cytokines have also been developed over the past three decades (1). These treatments remain in active development; however, they have been only partly successful. Technological shortcomings, the limited translational success of adapting preclinical rodent studies to humans, and the ambiguity of the T1D pathogenesis have been involved in the current limitations of immunotherapies for T1D (5, 10, 11). Stem cell-based therapies that aim to replace or regenerate destructed β-cell are broadly investigated treatments (7). Although showing promising results, stem cell-derived β-cells need to be protected from immune-mediated destruction. One way to overcome this is by combining replacement therapy with immunomodulatory treatments (5).
One way to overcome the inflammatory condition and suppress the immune cells is using extracellular vesicles (EVs). EVs are therapeutic agents recently introduced as a new treatment for several autoimmune diseases, especially multiple sclerosis (MS), rheumatoid arthritis (RA), and T1D, with considerable immunomodulatory and regenerative effects (12–17). This review brings together current state-of-the-art advances in applying EVs as a treatment for T1D.
2 Extracellular Vesicles
2.1 Classification and Characterization
Nearly all cells can produce and release endosome-derived vesicles (30–2000 nm) called EVs (18, 19). EVs are important intercellular communication mediators and contain lipids, metabolites, DNA, proteins, and RNA species, such as miRNA, mRNA, tRNAs (20, 21). There are three main subtypes of EVs based on their mechanism of biogenesis: exosomes (EXOs), microvesicles (MVs), and apoptotic bodies (19). EXOs range from 30 to 180nm in size and are characterized by markers including tetraspanins (CD9, CD63, CD81, TSPAN29, and TSPAN30), ESCRT components, HSP70, ALIX, flotillin, MFGE8, and TSG101 (18, 19). EXOs components mainly include ESCRT-related proteins, microRNAs (miRNAs), messenger RNAs (mRNA) and other non-coding RNAs, cytoplasmic and membrane proteins, including receptors and major histocompatibility complex (MHC) molecules (18, 19, 22). EXOs arise from a complex multi-step process in which the plasma membrane is internalized to form a primary endosome, followed by intra-luminal vesicles (ILVs) formation within the endosome. At this stage, specific substrates enter the ILVs via ESCRTs or ESCRT-independent machines. The primary endosome is now considered a mature multivesicular body (MVB), including several ILVs. The MVB attaches to the plasma membrane and releases ILVs into the extracellular space called ‘EXOs’.
MVs’ (50 to 1000nm) markers include integrins, selectins, and CD40 ligand, and their components are mRNA, miRNA, non-coding RNAs, and cytoplasmic and membrane proteins. MVs are generated by the outward budding of the plasma membrane (18, 23) (Figure 1). The apoptotic bodies (500 to 2000nm) are released from apoptotic cells and contain nuclear fractions and cell organelles (18, 24, 25).
Despite recent advances in the understanding of EV, in several published articles, the term “exosome” and “microvesicle” have been used interchangeably due to a lack of a uniform protocol for EV purification and incomplete knowledge of the EVs characteristics (18); as differences in the currently known characteristics such as size, density, and protein markers seem insufficient for a satisfiable distinction between EXOs and MVs (20, 26).
2.2 Interaction With the Target Cell and Biological Significance
EVs are naturally present in body fluids, including blood, urine, saliva, semen, sputum, and milk (21, 27, 28); and are released from many cells such as stem cells (29), progenitor cells (30), mesenchymal stromal cells (MSCs) (17), somatic cells like pancreatic beta cells (31), and many other cells.
EVs are recognized for their unique ability to transport different intact molecules between cells. Cell-to-cell communication mediated by EVs requires traveling and interacting with the plasma membrane. At the target site, EVs can act through membrane receptors and activate downstream intracellular signaling, or they directly fuse with the cell membrane and release their content into the target cell’s cytoplasm. EVs also can internalize by clathrin-mediated or clathrin-independent endocytosis (22, 32).
Several physiological functions have been identified for EVs in recent decades, as they play essential roles in the innate and acquired immune systems response (e.g., antigen presentation and activation of innate antiviral immune responses) (33–35). On the other hand, adverse roles have also been attributed to EVs when the condition is pathological, indicating that EVs are involved in developing or progressing many diseases, including type 1 and 2 diabetes, neurological diseases, autoimmune diseases, and cancers (e.g., primary and metastatic brain tumors, ovarian cancer, breast cancer, and pancreatic cancer) (15, 36–40).
In the last decades, EVs gained interest as a new treatment option in several diseases as they mediate intercellular signaling (20, 41). EV’s content profile and biological activity depend on their cell of origin and the microenvironment (42). They have shown great potential in inducing cell proliferation and tissue repair (43), angiogenesis (31), and improving some cellular functions (43). EVs have also shown considerable properties in modulating the immune system mainly by carrying immunomodulatory effectors, such as transcriptional factors (e.g., Nanog and Oct4), mRNAs, and cytokines (e.g., TGF-β and IL-10) (21, 44–46). For these reasons, diverse therapeutic properties made them popular, especially in drug delivery and regenerative medicine (41).
3 EVs for T1D
The main treatment strategies for T1D are immunotherapies (1) and cell replacement, including islet transplantation and stem cell differentiation into β-cells as a cell replacement method (7). As a new opportunity, EVs, with their therapeutic properties, had emerged (21, 42). EV’s simultaneous regenerative and immunomodulatory abilities align with new hypotheses about the T1D nature and the ideal treatments. According to the new speculations, an ideal treatment for T1D should be able to recover β-cells while modulating the immune system (10) (Figure 2).
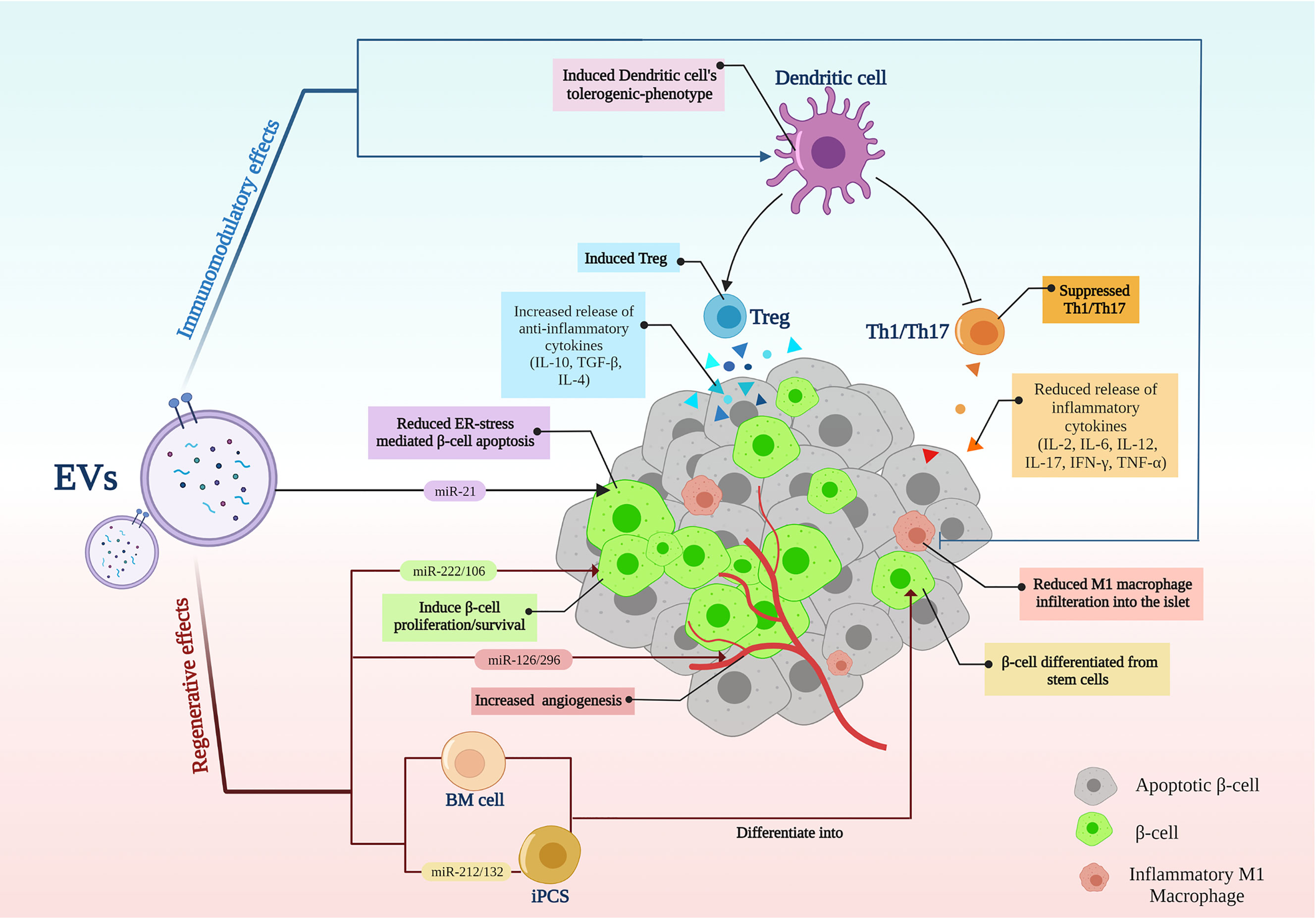
Figure 2 Extracellular vesicle application in type 1 diabetes; EVs modulate inflammation while recovering β-cells. EVs, extracellular vesicles; BM, Bone marrow; iPSCs, induced pluripotent stem cells; Th, T helper cell; Treg, regulatory T cell.
3.1 Immunomodulatory Effects
T1D is essentially an autoimmune disease in which insulin-producing β-cells in the pancreas are invaded and destroyed by the immune system (1); Meanwhile, auto-reactive T cells have been identified as the primary attackers (47, 48). On the other hand, regulatory T cells have lost their efficiency in creating peripheral tolerance to β-cells (49). In T1D, EVs demonstrated immunomodulatory effects, mainly through suppressing reactive T cells and inducing regulatory T cells (Table 1).
3.1.1 Suppressing Auto-Reactive Immune Cells
T cells play a crucial role in the immune attacks on β-cells (47). CD4+ T cells are activated by β-cell antigens, which are presented by antigen-presenting cells (APCs), including macrophages and dendritic cells (DCs); these activated CD4+ T cells by producing cytokines, attract and induce the proliferation of CD8+ T and B cells in the islet, leading to insulitis (48). Insulitis indicates an immune attack on β-cells and is defined by the infiltration of inflammatory cells around and within the islets (52). Suppressing auto-reactive CD4+ T cells delays T1D onset/progression (1).
EVs have shown suppressive effects on auto-reactive T cells in T1D animal models, while MSC-derived EVs were shown to suppress the proliferation/activation of APCs, Th1 and Th17 cells in vitro and delayed T1D onset in mice (16). In line with these findings, releasing inflammatory cytokines by reactive CD4+ T cells including IFN‐γ, IL-12, TNF-α, IL-6, and IL-17 were reduced following EV treatment. The authors suggested that MSC-EVs might induce IL-10-secreting regulatory DCs; thereby, DCs subsequently suppressed Th1 and Th17 cells development (16). In another study, DCs preconditioned with MSC-derived EXOs represented an immature IL-10-secreting phenotype associated with reduced co-stimulatory molecules expression, which could reduce Th17 numbers in the islet-antigen-stimulated T cells in vitro (17). Therefore, Th17 cells are believed to contribute to the pathogenesis of autoimmune diseases; however, their role in T1D is not fully understood (53)
In addition to T cells, macrophages also infiltrate the pancreatic islets in the early stages of T1D and induce an inflammatory response resulting in insulitis and β-cell death (48). EXOs isolated from β-cells showed lower proinflammatory macrophage infiltration into the pancreatic islets in diabetic mice (31). In a study by Hu et al., EXOs isolated from cord blood-derived stem cells converted patients’ blood monocytes into M2 macrophages with anti-inflammatory properties in vitro. M2 macrophages, called “educated” immune cells, can be re-injected into the T1D patients’ blood, thus modulating the immune system (29).
3.1.2 Inducing Regulatory T Cells
Regulatory T cells (Tregs) are essential in establishing peripheral immune tolerance (54). Defective Treg-mediated immune regulation has been shown in numerous autoimmune disorders, including T1D (55). At least a subset of individuals with T1D have reduced FOXP3+ Treg frequency and function (49). Tregs from patients with T1D showed to be less potent to regulate the proliferation of autologous effector T cells (56) and produce mainly proinflammatory cytokines (57). However, T1D patients are believed to benefit from reinforcement of Tregs, even individuals who do not have reduced Treg frequency or function (49). Accordingly, EVs have demonstrated the potential to induce Tregs. EXOs derived from adipose-MSCs have been shown to restrain the autoimmune response of the streptozotocin (STZ)-induced T1D mouse model (50). These EXOs led to an increase in the splenic production of anti‐inflammatory cytokines TGF‐β, IL‐4, and IL‐10, with a decrease in the production of proinflammatory cytokines IL‐17 and IFN‐γ, as the results of an increased splenic CD25+FOXP3+ Treg population (50). Wen et al. suggested that EXOs isolated from human bone marrow stromal cells (hBMSC) and peripheral blood mononuclear cell (PBMC) co-culture could suppress immune reaction by amplifying Treg function in a humanized NOD SCID gamma (NSG) mouse model (51). In another study, T1D patients-isolated DCs preconditioned with MSC-derived EXOs induced a higher proportion of FOXP3+ Tregs in the islet-antigen-stimulated T cell population in vitro. These T cells also exerted an increased secretion of anti-inflammatory IL-10, TGF-β, and IL-6 molecules (17).
3.2 Inducing β-Cell Regeneration
Pancreatic β-cells regeneration is a promising strategy for T1D treatment. In this regard, EVs have shown considerable potential in inducing β-cell proliferation (Table 2). EXOs isolated from MSCs have been shown to induce islet cell regeneration and insulin secretion through upregulating pancreatic and duodenal homeobox1 (pdx1), TGF-β, and smad1/2 (12). Pdx1 is involved in β-cell differentiation, survival, and functional maintenance (62), and TGF-β is also responsible for cell proliferation and differentiation (63). Moreover, MSC-EXOs demonstrated higher regenerative potential when compared with parental MSCs (58). EXOs derived from menstrual blood-derived MSCs have also shown similar results in inducing β-cell regeneration through pdx1 upregulation in T1D rats (59). Healthy adipocyte-derived EVs also improved the survival, proliferation, and insulin-producing function of pancreatic β-cells (43). In a study by Tsukita et al., bone marrow transplantation promoted β-cell regeneration in T1D mice by releasing EXOs containing miR-106b-5p and miR-222-3p into the bloodstream. These microRNAs encapsulated in EXOs reached β-cells and downregulated p21Cip1 and p27Kip1, which are negative controllers of β-cell regeneration after injury, inducing β-cell proliferation (60).
Pathfinder cells (PC) (pancreas-derived progenitor cells) were recently introduced as a novel cell type that can be isolated from the adult rat pancreatic ducts and induced to form islet-like structures in vitro (64). PCs have been shown to stimulate regeneration of damaged mice pancreatic islets (65). In order to extend the latter study, McGuinness et al. suggested that PC-derived MVs (not EXOs) induce islet regeneration and restore their function in the STZ diabetic mouse model. The results of the PC-MVs were similar to the PC treatment (61), which demonstrated that PCs in the Stevenson et al. study (65) possibly acted through releasing MVs.
3.3 Improving Islet Transplantation Outcome
Destruction of transplanted islets due to immune rejection and poor vascularity limits the broad application of islet transplantation in T1D patients (66). Therefore, improving transplanted islet cell survival by protecting these islets against the immune system and enhancing angiogenesis within and/or around them seem beneficial and essential. In this context, EVs showed promising effects (Table 3).
hBMSCs derived EXOs suppressed transplanted islet apoptosis and improved their function in a humanized NSG mouse model by co-delivering short interfering RNA (siRNA) against Fas receptor and miR-375 inhibitor (51). Evidence suggests that Fas activity and miR-375 impair islet cell proliferation and insulin secretion (73–75).
Foreign body response (FBR) is an inflammatory reaction to the transplantation, leading to dense fibrotic tissue forming around the microcapsules-containing islets, restricting the survival and insulin-producing function of islets in rodent models (76, 77). Xenotransplantation of rat islets encapsulated in hybrid alginate microcapsule-loaded by EXOs derived from human umbilical cord MSCs (UC-MSCs) attenuated the immune-based FBR and islet-engulfed fibrosis after transplantation. It increased the survival of transplanted islets in an immunocompetent mouse model of T1D. These EXOs suppressed inflammatory macrophage and T cell activation/proliferation followed by a reduced production of inflammatory cytokines, including MCP-1, IL-2, IL-6, IL-12, IL-22, and TNF-α (68).
Transplanted islets often experience hypoxia due to poor vascularity (78). Hypoxia causes β-cells death and plays a crucial role in destroying the transplanted islets (79). EXOs derived from human UC-MSCs have been demonstrated to improve β-cell survival under a hypoxic condition by delivering miR-21, resulting in reduced endoplasmic reticulum (ER) stress and p38 MAPK suppression (70). As ER stress activates p38-dependent apoptosis (80), ER stress reduction leads to a decrease in β-cell apoptosis (70). EXOs derived from human UC-MSC were also able to protect the survival and function of porcine islets from hypoxia by inducing hypoxia-inducible factor 1α (HIF-1α) expression (71). In another similar study, Keshtkar et al. also observed similar results for UC-MSC-derived EXOs in improving the survival and function of mouse islets; but this time, they introduced VEGF as the leading player in these results. They showed that UC-MSC-derived EXOs contained VEGF (mRNA and protein) and increased VEGF expression in islet cells (72). VEGF can enhance the viability and function of transplanted islets in the early days after transplantation, and this effect of VEGF is independent of neovascularization since neovascularization in transplanted islets occurs days to weeks later (81–83).
EVs also demonstrated potential in improving islet revascularization after transplantation. MVs derived from endothelial progenitor cells (EPCs) induced angiogenic behavior in islet endothelium in vitro and enhanced vascularization of transplanted islets in severe combined immunodeficient (SCID) mice. This proangiogenic effect was acquired by delivering miR-126 and miR-296 (known as proangiogenic microRNAs) and activating the PI3K-Akt and eNOS signaling pathways further. Indeed, EPC-derived MVs improved insulin secretion and survival of the transplanted islets (30). Moreover, MVs isolated from the β-cell line preserved islet cells’ function by inducing islet neovascularization in streptozotocin-diabetic mice (31).
Another problem in islet transplantation is limited sources of functional islets for transplantation. Therefore, several studies have developed alternative insulin-producing cells by inducing differentiation of stem/progenitor cells (84). In this regard, applying EV-mimetic nanovesicles (EVMNs) derived from a pancreatic β-cell line to a subcutaneous matrigel platform containing bone marrow cells in diabetic NSG mice induced differentiation of islet-like clusters of insulin-producing cells with capillary networks from bone marrow cells. These islet-like insulin-producing cells could control the blood glucose levels over 60 days (69).
Bai et al. used β cell-derived EVs to induce β-cell differentiation from induced pluripotent stem cells (iPSCs) and suggested the critical role of miR-212/132 (encapsulated in these EVs) in this process, which inhibited FBW7 to reinstate neurogenin 3 (NGN3) expression. In the following, NGN3 is bound to PDX1 to induce endogenous miR-212/132 expression resulting in the insulin-producing function of β-cells (67). NGN3 is a transcription factor involved in pancreas development (85, 86), which induces differentiation of islet cell precursors, and FBW7 is a ubiquitin ligase that has a negative impact on the NGN3 stability (87).
4 Future Considerations
While it is widely believed that T1D is caused by immune system dysregulation and autoimmunity, another hypothesis has been put forward stating that the pathogenesis of T1D is triggered via β-cell itself and consequently causes an unregulated autoimmune response (10), a phenomenon entitled “un-masked β-cell” (5). This might partially explain why immunotherapies alone are not satisfactory. One possible cause of the “un-masked β-cell” is β-cell stress. Stressed β-cells through HLA-I and chemokine CXCL10 secretion hyper-expression attract leukocytes to the islet and precede insulitis. Therefore, reducing this cellular stress in β-cells may effectively mitigate this phenomenon (10, 88). As mentioned, EVs can efficiently reduce β-cell stress (70). The “un-masked β-cell” hypothesis supports therapies that help β-cell recovery/regeneration while modulating the immune system. Since immune system dysregulation is thought to result from an initial β-cells misbehave; thus, the consequent autoimmunity cannot be resolved before β-cell recovery (10). In this regard, EV therapy suggests both effects.
Accordingly, one practical approach for T1D would be inducing selective immune tolerance to β-cell autoantigens (10). Nanoparticles and microparticles have been used as delivery packets for autoantigens such as proinsulin or GAD65 to induce a tolerogenic phenotype in DCs, characterized by the reduced ability of DCs to activate inflammatory auto-reactive T cells resulting in increased differentiation of FOXP3+ Treg cells (89). In this regard, EV, as a drug delivery tool (18), can also induce immune tolerance in DCs via delivering β-cell autoantigens. Indeed, MSC-derived EVs were shown to induce a tolerogenic phenotype in DCs resulting in anti-islet T cells reduction, although not by β-cell autoantigen transfer (16, 17).
Although knowledge on EV therapy for T1D is not yet leading to a conclusive outcome, based on the studies we reviewed here, the most prominent cell sources for EV isolation for therapeutic applications in T1D include BMSCs, β-cells, and UC-MSCs. When it comes to inducing proliferation and improving the insulin production/secretion function of β-cells, BMSCs-derived EVs showed considerable potency (12, 58, 60). Moreover, when it comes to inducing differentiation of β-cells from pluripotent cells, β-cells-derived EVs are the most used (67, 69). EVs derived from UC-MSCs were also reported to protect β-cells after transplantation with high efficiency (70–72).
However, interpretation of the results of current studies should be made with care; since most studies did not adequately address the potential effects associated with EVs co-isolates (22); therefore, it is possible that some of the obtained results were affected or caused by the co-isolates. In addition, there are still many other considerations associated with EV isolation, evaluation, and functional assays, which are not adequately addressed in most studies. EV isolation and concentration methods, including ultracentrifugation, ultrafiltration, density gradients, precipitation, size exclusion chromatography, and immuno-isolation, cannot effectively isolate various EV subtypes (including EXOs and MVs) and are partially successful in specificity to EV isolation (6, 22). In addition, there is still no standard way to evaluate the stability (90) and functionality of these isolated EVs, so it is not clear how many EVs are ultimately involved in the reported results.
Clinical application of EVs for T1D has been limited so far. This could be partially because much preparation is needed to bring EV therapy into the clinical practice; upgrading and standardizing EV isolation/purification methods, obtaining the most efficient dose and the number of EV injections, producing EV on a large scale, and ensuring EV safety are some of the most prominent issues that must be addressed before the entrance of EV to the clinics (6). To study the effect of EV therapy on β-cell mass and glycemic control, one study at phase 1 was conducted in 2014, where cord blood MSC-derived EVs were used to treat twenty T1D patients (NCT02138331). However, this study seems never to be completed, and the data is not published. This is the only clinical trial performed in T1D using EVs to the best of our knowledge. Obviously, more clinical trials are needed to evaluate EV effectiveness for T1D.
5 Conclusion
Here we reviewed the studies that used EVs for T1D treatment in pre-clinical settings and further highlighted the lack of clinical application knowledge in the field. As an effective treatment for T1D, EVs are thought to regulate the immune system at innate and adaptive levels, possibly by inducing tolerance in DCs to β-cell autoantigens. Based on the recent speculation about the pathogenesis of T1D, which emphasizes the β-cell as a key player in initiating autoimmunity, EV therapy could continue to present itself as an effective and scalable treatment option. It was “postulated” that EVs can reduce cellular stress in β-cells and, therefore, reduce the immune system attack. By inducing proliferation and regeneration in β-cells, EVs also restore destructed islet mass. Moreover, they can increase the survival rate of transplanted islets by diminishing immune rejection and by enhancing angiogenesis.
In a nutshell, studies reviewed here have provided excellent guidelines to use EVs features to design specific clinical trials that utterly could generate an alternative to standard treatment. However, EVs continue to present some practical challenges for their use as a clinical-grade therapy, which must be addressed in the future.
Author Contributions
All authors listed have made a substantial, direct, and intellectual contribution to the work, and approved it for publication.
Funding
The Lundbeck foundation grant R303-2018-3148 supported this work.
Conflict of Interest
The authors declare that the research was conducted in the absence of any commercial or financial relationships that could be construed as a potential conflict of interest.
Publisher’s Note
All claims expressed in this article are solely those of the authors and do not necessarily represent those of their affiliated organizations, or those of the publisher, the editors and the reviewers. Any product that may be evaluated in this article, or claim that may be made by its manufacturer, is not guaranteed or endorsed by the publisher.
References
1. Warshauer JT, Bluestone JA, Anderson MS. New Frontiers in the Treatment of Type 1 Diabetes. Cell Metab (2020) 31(1):46–61. doi: 10.1016/j.cmet.2019.11.017
2. Gale EA. The Rise of Childhood Type 1 Diabetes in the 20th Century. Diabetes (2002) 51(12):3353–61. doi: 10.2337/diabetes.51.12.3353
3. Norris JM, Johnson RK, Stene LC. Type 1 Diabetes-Early Life Origins and Changing Epidemiology. Lancet Diabetes Endocrinol (2020) 8(3):226–38. doi: 10.1016/S2213-8587(19)30412-7
4. Guo X, Wang W. Challenges and Recent Advances in the Subcutaneous Delivery of Insulin. Expert Opin Drug Deliv (2017) 14(6):727–34. doi: 10.1080/17425247.2016.1232247
5. von Scholten BJ, Kreiner FF, Gough SCL, von Herrath M. Current and Future Therapies for Type 1 Diabetes. Diabetologia (2021) 64(5):1037–48. doi: 10.1007/s00125-021-05398-3
6. Soltani S, Mansouri K, Parvaneh S, Thakor AS, Pociot F, Yarani R. Diabetes Complications and Extracellular Vesicle Therapy. Rev Endocr Metab Disord (2021). doi: 10.1007/s11154-021-09680-y
7. Brovkina O, Dashinimaev E. Advances and Complications of Regenerative Medicine in Diabetes Therapy. PeerJ (2020) 8:e9746. doi: 10.7717/peerj.9746
8. Posselt AM, Bellin MD, Tavakol M, Szot GL, Frassetto LA, Masharani U, et al. Islet Transplantation in Type 1 Diabetics Using an Immunosuppressive Protocol Based on the Anti-LFA-1 Antibody Efalizumab. Am J Transplant (2010) 10(8):1870–80. doi: 10.1111/j.1600-6143.2010.03073.x
9. Wang X, Brown NK, Wang B, Shariati K, Wang K, Fuchs S, et al. Local Immunomodulatory Strategies to Prevent Allo-Rejection in Transplantation of Insulin-Producing Cells. Adv Sci (Weinh) (2021) 8(17):e2003708. doi: 10.1002/advs.202003708
10. Roep BO, Thomaidou S, van Tienhoven R, Zaldumbide A. Type 1 Diabetes Mellitus as a Disease of the β-Cell (do Not Blame the Immune System)? Nat Rev Endocrinol (2021) 17(3):150–61. doi: 10.1038/s41574-020-00443-4
11. Atkinson MA, Roep BO, Posgai A, Wheeler DCS, Peakman M. The Challenge of Modulating β-Cell Autoimmunity in Type 1 Diabetes. Lancet Diabetes Endocrinol (2019) 7(1):52–64. doi: 10.1016/S2213-8587(18)30112-8
12. Sabry D, Marzouk S, Zakaria R, Ibrahim HA, Samir M. The Effect of Exosomes Derived From Mesenchymal Stem Cells in the Treatment of Induced Type 1 Diabetes Mellitus in Rats. Biotechnol Lett (2020) 42(8):1597–610. doi: 10.1007/s10529-020-02908-y
13. Yu L, Yang F, Jiang L, Chen Y, Wang K, Xu F, et al. Exosomes With Membrane-Associated TGF-β1 From Gene-Modified Dendritic Cells Inhibit Murine EAE Independently of MHC Restriction. Eur J Immunol (2013) 43(9):2461–72. doi: 10.1002/eji.201243295
14. Kim SH, Lechman ER, Bianco N, Menon R, Keravala A, Nash J, et al. Exosomes Derived From IL-10-Treated Dendritic Cells can Suppress Inflammation and Collagen-Induced Arthritis. J Immunol (2005) 174(10):6440–8. doi: 10.4049/jimmunol.174.10.6440
15. Tian J, Casella G, Zhang Y, Rostami A, Li X. Potential Roles of Extracellular Vesicles in the Pathophysiology, Diagnosis, and Treatment of Autoimmune Diseases. Int J Biol Sci (2020) 16(4):620–32. doi: 10.7150/ijbs.39629
16. Shigemoto-Kuroda T, Oh JY, Kim DK, Jeong HJ, Park SY, Lee HJ, et al. MSC-Derived Extracellular Vesicles Attenuate Immune Responses in Two Autoimmune Murine Models: Type 1 Diabetes and Uveoretinitis. Stem Cell Rep (2017) 8(5):1214–25. doi: 10.1016/j.stemcr.2017.04.008
17. Favaro E, Carpanetto A, Caorsi C, Giovarelli M, Angelini C, Cavallo-Perin P, et al. Human Mesenchymal Stem Cells and Derived Extracellular Vesicles Induce Regulatory Dendritic Cells in Type 1 Diabetic Patients. Diabetologia (2016) 59(2):325–33. doi: 10.1007/s00125-015-3808-0
18. ELA S, Mäger I, Breakefield XO, Wood MJ. Extracellular Vesicles: Biology and Emerging Therapeutic Opportunities. Nat Rev Drug Discovery (2013) 12(5):347–57. doi: 10.1038/nrd3978
19. Yang Y, Hong Y, Cho E, Kim GB, Kim IS. Extracellular Vesicles as a Platform for Membrane-Associated Therapeutic Protein Delivery. J Extracell Vesicles (2018) 7(1):1440131. doi: 10.1080/20013078.2018.1440131
20. Raposo G, Stoorvogel W. Extracellular Vesicles: Exosomes, Microvesicles, and Friends. J Cell Biol (2013) 200(4):373–83. doi: 10.1083/jcb.201211138
21. Robbins PD, Morelli AE. Regulation of Immune Responses by Extracellular Vesicles. Nat Rev Immunol (2014) 14(3):195–208. doi: 10.1038/nri3622
22. Théry C, Witwer KW, Aikawa E, Alcaraz MJ, Anderson JD, Andriantsitohaina R, et al. Minimal Information for Studies of Extracellular Vesicles 2018 (MISEV2018): A Position Statement of the International Society for Extracellular Vesicles and Update of the MISEV2014 Guidelines. J Extracell Vesicles (2018) 7(1):1535750. doi: 10.1080/20013078.2018.1535750
23. Sedgwick AE, D’Souza-Schorey C. The Biology of Extracellular Microvesicles. Traffic (2018) 19(5):319–27. doi: 10.1111/tra.12558
24. Akers JC, Gonda D, Kim R, Carter BS, Chen CC. Biogenesis of Extracellular Vesicles (EV): Exosomes, Microvesicles, Retrovirus-Like Vesicles, and Apoptotic Bodies. J Neurooncol (2013) 113(1):1–11. doi: 10.1007/s11060-013-1084-8
25. Jiang L, Paone S, Caruso S, Atkin-Smith GK, Phan TK, Hulett MD, et al. Determining the Contents and Cell Origins of Apoptotic Bodies by Flow Cytometry. Sci Rep (2017) 7(1):14444. doi: 10.1038/s41598-017-14305-z
26. Bobrie A, Colombo M, Raposo G, Théry C. Exosome Secretion: Molecular Mechanisms and Roles in Immune Responses. Traffic (2011) 12(12):1659–68. doi: 10.1111/j.1600-0854.2011.01225.x
27. Yáñez-Mó M, Siljander PR, Andreu Z, Zavec AB, Borràs FE, Buzas EI, et al. Biological Properties of Extracellular Vesicles and Their Physiological Functions. J Extracell Vesicles (2015) 4:27066. doi: 10.3402/jev.v4.27066
28. Mirza AH, Kaur S, Nielsen LB, Størling J, Yarani R, Roursgaard M, et al. Breast Milk-Derived Extracellular Vesicles Enriched in Exosomes From Mothers With Type 1 Diabetes Contain Aberrant Levels of microRNAs. Front Immunol (2019) 10:2543. doi: 10.3389/fimmu.2019.02543
29. Hu W, Song X, Yu H, Sun J, Zhao Y. Differentiation of Monocytes Into Phenotypically Distinct Macrophages After Treatment With Human Cord Blood Stem Cell (CB-SC)-Derived Exosomes. J Vis Exp (2020) (165):e61562. doi: 10.3791/61562
30. Cantaluppi V, Biancone L, Figliolini F, Beltramo S, Medica D, Deregibus MC, et al. Microvesicles Derived From Endothelial Progenitor Cells Enhance Neoangiogenesis of Human Pancreatic Islets. Cell transplantation (2012) 21(6):1305–20. doi: 10.3727/096368911X627534
31. Sun Y, Mao Q, Shen C, Wang C, Jia W. Exosomes From β-Cells Alleviated Hyperglycemia and Enhanced Angiogenesis in Islets of Streptozotocin-Induced Diabetic Mice. Diabetes Metab Syndr Obes (2019) 12:2053–64. doi: 10.2147/DMSO.S213400
32. Mulcahy LA, Pink RC, Carter DR. Routes and Mechanisms of Extracellular Vesicle Uptake. J Extracell Vesicles (2014) 3(1):24641. doi: 10.3402/jev.v3.24641
33. Lindenbergh MFS, Stoorvogel W. Antigen Presentation by Extracellular Vesicles From Professional Antigen-Presenting Cells. Annu Rev Immunol (2018) 36:435–59. doi: 10.1146/annurev-immunol-041015-055700
34. Nocera AL, Mueller SK, Stephan JR, Hing L, Seifert P, Han X, et al. Exosome Swarms Eliminate Airway Pathogens and Provide Passive Epithelial Immunoprotection Through Nitric Oxide. J Allergy Clin Immunol (2019) 143(4):1525–35.e1. doi: 10.1016/j.jaci.2018.08.046
35. Zhou X, Xie F, Wang L, Zhang L, Zhang S, Fang M, et al. The Function and Clinical Application of Extracellular Vesicles in Innate Immune Regulation. Cell Mol Immunol (2020) 17(4):323–34. doi: 10.1038/s41423-020-0391-1
36. Xiao Y, Zheng L, Zou X, Wang J, Zhong J, Zhong T. Extracellular Vesicles in Type 2 Diabetes Mellitus: Key Roles in Pathogenesis, Complications, and Therapy. J Extracell Vesicles (2019) 8(1):1625677. doi: 10.1080/20013078.2019.1625677
37. Morad G, Moses MA. Brainwashed by Extracellular Vesicles: The Role of Extracellular Vesicles in Primary and Metastatic Brain Tumour Microenvironment. J Extracell Vesicles (2019) 8(1):1627164. doi: 10.1080/20013078.2019.1627164
38. Becker A, Thakur BK, Weiss JM, Kim HS, Peinado H, Lyden D. Extracellular Vesicles in Cancer: Cell-To-Cell Mediators of Metastasis. Cancer Cell (2016) 30(6):836–48. doi: 10.1016/j.ccell.2016.10.009
39. Shah R, Patel T, Freedman JE. Circulating Extracellular Vesicles in Human Disease. N Engl J Med (2018) 379(10):958–66. doi: 10.1056/NEJMra1704286
40. Grieco GE, Fignani D, Formichi C, Nigi L, Licata G, Maccora C, et al. Extracellular Vesicles in Immune System Regulation and Type 1 Diabetes: Cell-To-Cell Communication Mediators, Disease Biomarkers, and Promising Therapeutic Tools. Front Immunol (2021) 12:682948. doi: 10.3389/fimmu.2021.682948
41. Murphy DE, de Jong OG, Brouwer M, Wood MJ, Lavieu G, Schiffelers RM, et al. Extracellular Vesicle-Based Therapeutics: Natural Versus Engineered Targeting and Trafficking. Exp Mol Med (2019) 51(3):1–12. doi: 10.1038/s12276-019-0223-5
42. Bjørge IM, Kim SY, Mano JF, Kalionis B, Chrzanowski W. Extracellular Vesicles, Exosomes and Shedding Vesicles in Regenerative Medicine - a New Paradigm for Tissue Repair. Biomater Sci (2017) 6(1):60–78. doi: 10.1039/C7BM00479F
43. Gesmundo I, Pardini B, Gargantini E, Gamba G, Birolo G, Fanciulli A, et al. Adipocyte-Derived Extracellular Vesicles Regulate Survival and Function of Pancreatic β Cells. JCI Insight (2021) 6(5):e141962. doi: 10.1172/jci.insight.141962
44. Burrello J, Monticone S, Gai C, Gomez Y, Kholia S, Camussi G. Stem Cell-Derived Extracellular Vesicles and Immune-Modulation. Front Cell Dev Biol (2016) 4:83. doi: 10.3389/fcell.2016.00083
45. Bruno S, Deregibus MC, Camussi G. The Secretome of Mesenchymal Stromal Cells: Role of Extracellular Vesicles in Immunomodulation. Immunol Lett (2015) 168(2):154–8. doi: 10.1016/j.imlet.2015.06.007
46. Katsman D, Stackpole EJ, Domin DR, Farber DB. Embryonic Stem Cell-Derived Microvesicles Induce Gene Expression Changes in Müller Cells of the Retina. PloS One (2012) 7(11):e50417. doi: 10.1371/journal.pone.0050417
47. Bluestone JA, Herold K, Eisenbarth G. Genetics, Pathogenesis and Clinical Interventions in Type 1 Diabetes. Nature (2010) 464(7293):1293–300. doi: 10.1038/nature08933
48. Szablewski L. Role of Immune System in Type 1 Diabetes Mellitus Pathogenesis. Int Immunopharmacol (2014) 22(1):182–91. doi: 10.1016/j.intimp.2014.06.033
49. Hull CM, Peakman M, Tree TIM. Regulatory T Cell Dysfunction in Type 1 Diabetes: What’s Broken and How can We Fix it? Diabetologia (2017) 60(10):1839–50. doi: 10.1007/s00125-017-4377-1
50. Nojehdehi S, Soudi S, Hesampour A, Rasouli S, Soleimani M, Hashemi SM. Immunomodulatory Effects of Mesenchymal Stem Cell-Derived Exosomes on Experimental Type-1 Autoimmune Diabetes. J Cell Biochem (2018) 119(11):9433–43. doi: 10.1002/jcb.27260
51. Wen D, Peng Y, Liu D, Weizmann Y, Mahato RI. Mesenchymal Stem Cell and Derived Exosome as Small RNA Carrier and Immunomodulator to Improve Islet Transplantation. J Control Release (2016) 238:166–75. doi: 10.1016/j.jconrel.2016.07.044
52. Pugliese A. Insulitis in the Pathogenesis of Type 1 Diabetes. Pediatr Diabetes (2016) 17 Suppl 22(Suppl Suppl 22):31–6. doi: 10.1111/pedi.12388
53. Pietropaolo M, Surhigh JM, Nelson PW, Eisenbarth GS. Primer: Immunity and Autoimmunity. Diabetes (2008) 57(11):2872–82. doi: 10.2337/db07-1691
54. Lu J, Li P, Du X, Liu Y, Zhang B, Qi F. Regulatory T Cells Induce Transplant Immune Tolerance. Transpl Immunol (2021) 67:101411. doi: 10.1016/j.trim.2021.101411
55. Dominguez-Villar M, Hafler DA. Regulatory T Cells in Autoimmune Disease. Nat Immunol (2018) 19(7):665–73. doi: 10.1038/s41590-018-0120-4
56. Lindley S, Dayan CM, Bishop A, Roep BO, Peakman M, Tree TI. Defective Suppressor Function in CD4(+)CD25(+) T-Cells From Patients With Type 1 Diabetes. Diabetes (2005) 54(1):92–9. doi: 10.2337/diabetes.54.1.92
57. McClymont SA, Putnam AL, Lee MR, Esensten JH, Liu W, Hulme MA, et al. Plasticity of Human Regulatory T Cells in Healthy Subjects and Patients With Type 1 Diabetes. J Immunol (2011) 186(7):3918–26. doi: 10.4049/jimmunol.1003099
58. Mostafa-Hedeab G, Hassan ME, Sabry D, Ali RI. Anti-Diabetic Therapeutic Efficacy of Mesenchymal Stem Cells-Derived Exosomes. Int J OF Pharmacol (2020) 16(6):437–46. doi: 10.3923/ijp.2020.437.446
59. Mahdipour E, Salmasi Z, Sabeti N. Potential of Stem Cell-Derived Exosomes to Regenerate β Islets Through Pdx-1 Dependent Mechanism in a Rat Model of Type 1 Diabetes. J Cell Physiol (2019) 234(11):20310–21. doi: 10.1002/jcp.28631
60. Tsukita S, Yamada T, Takahashi K, Munakata Y, Hosaka S, Takahashi H, et al. MicroRNAs 106b and 222 Improve Hyperglycemia in a Mouse Model of Insulin-Deficient Diabetes via Pancreatic β-Cell Proliferation. EBioMedicine (2017) 15:163–72. doi: 10.1016/j.ebiom.2016.12.002
61. McGuinness D, Anthony DF, Moulisova V, MacDonald AI, MacIntyre A, Thomson J, et al. Microvesicles But Not Exosomes From Pathfinder Cells Stimulate Functional Recovery of the Pancreas in a Mouse Streptozotocin-Induced Diabetes Model. Rejuvenation Res (2016) 19(3):223–32. doi: 10.1089/rej.2015.1723
62. Fujimoto K, Polonsky KS. Pdx1 and Other Factors That Regulate Pancreatic Beta-Cell Survival. Diabetes Obes Metab (2009) 11 Suppl 4(Suppl 4):30–7. doi: 10.1111/j.1463-1326.2009.01121.x
63. David CJ, Massagué J. Publisher Correction: Contextual Determinants of Tgfβ Action in Development, Immunity and Cancer. Nat Rev Mol Cell Biol (2018) 19(7):479. doi: 10.1038/s41580-018-0018-x
64. McGlynn LM, Eller K, MacDonald AI, Macintyre A, Russell D, Koppelstaetter C, et al. Pathfinder Cells Provide a Novel Therapeutic Intervention for Acute Kidney Injury. Rejuvenation Res (2013) 16(1):11–20. doi: 10.1089/rej.2012.1350
65. Stevenson K, Chen D, MacIntyre A, McGlynn LM, Montague P, Charif R, et al. Pancreatic-Derived Pathfinder Cells Enable Regeneration of Critically Damaged Adult Pancreatic Tissue and Completely Reverse Streptozotocin-Induced Diabetes. Rejuvenation Res (2011) 14(2):163–71. doi: 10.1089/rej.2010.1099
66. Barshes NR, Wyllie S, Goss JA. Inflammation-Mediated Dysfunction and Apoptosis in Pancreatic Islet Transplantation: Implications for Intrahepatic Grafts. J Leukoc Biol (2005) 77(5):587–97. doi: 10.1189/jlb.1104649
67. Bai C, Ren Q, Liu H, Li X, Guan W, Gao Y. miR-212/132-Enriched Extracellular Vesicles Promote Differentiation of Induced Pluripotent Stem Cells Into Pancreatic Beta Cells. Front Cell Dev Biol (2021) 9:673231. doi: 10.3389/fcell.2021.673231
68. Mohammadi MR, Rodriguez SM, Luong JC, Li S, Cao R, Alshetaiwi H, et al. Exosome Loaded Immunomodulatory Biomaterials Alleviate Local Immune Response in Immunocompetent Diabetic Mice Post Islet Xenotransplantation. Commun Biol (2021) 4(1):685. doi: 10.1038/s42003-021-02229-4
69. Oh K, Kim SR, Kim DK, Seo MW, Lee C, Lee HM, et al. In Vivo Differentiation of Therapeutic Insulin-Producing Cells From Bone Marrow Cells via Extracellular Vesicle-Mimetic Nanovesicles. ACS Nano (2015) 9(12):11718–27. doi: 10.1021/acsnano.5b02997
70. Chen J, Chen J, Cheng Y, Fu Y, Zhao H, Tang M, et al. Mesenchymal Stem Cell-Derived Exosomes Protect Beta Cells Against Hypoxia-Induced Apoptosis via miR-21 by Alleviating ER Stress and Inhibiting P38 MAPK Phosphorylation. Stem Cell Res Ther (2020) 11(1):97. doi: 10.1186/s13287-020-01610-0
71. Nie W, Ma X, Yang C, Chen Z, Rong P, Wu M, et al. Human Mesenchymal-Stem-Cells-Derived Exosomes are Important in Enhancing Porcine Islet Resistance to Hypoxia. Xenotransplantation (2018) 25(5):e12405. doi: 10.1111/xen.12405
72. Keshtkar S, Kaviani M, Sarvestani FS, Ghahremani MH, Aghdaei MH, Al-Abdullah IH, et al. Exosomes Derived From Human Mesenchymal Stem Cells Preserve Mouse Islet Survival and Insulin Secretion Function. EXCLI J (2020) 19:1064–80. doi: 10.1155/2020/8857457
73. Feng ZC, Riopel M, Li J, Donnelly L, Wang R. Downregulation of Fas Activity Rescues Early Onset of Diabetes in C-Kit(Wv/+) Mice. Am J Physiol Endocrinol Metab (2013) 304(6):E557–65. doi: 10.1152/ajpendo.00453.2012
74. Choi D, Radziszewska A, Schroer SA, Liadis N, Liu Y, Zhang Y, et al. Deletion of Fas in the Pancreatic Beta-Cells Leads to Enhanced Insulin Secretion. Am J Physiol Endocrinol Metab (2009) 297(6):E1304–12. doi: 10.1152/ajpendo.00217.2009
75. Dumortier O, Hinault C, Gautier N, Patouraux S, Casamento V, Van Obberghen E. Maternal Protein Restriction Leads to Pancreatic Failure in Offspring: Role of Misexpressed microRNA-375. Diabetes (2014) 63(10):3416–27. doi: 10.2337/db13-1431
76. Doloff JC, Veiseh O, Vegas AJ, Tam HH, Farah S, Ma M, et al. Colony Stimulating Factor-1 Receptor is a Central Component of the Foreign Body Response to Biomaterial Implants in Rodents and non-Human Primates. Nat Mater (2017) 16(6):671–80. doi: 10.1038/nmat4866
77. Mohammadi MR, Rodrigez S, Cao R, Alexander M, Lakey JR. Immune Response to Subcutaneous Implants of Alginate Microcapsules. Materials Today: Proc (2018) 5(7):15580–5. doi: 10.1016/j.matpr.2018.04.166
78. Rodriguez-Brotons A, Bietiger W, Peronet C, Magisson J, Sookhareea C, Langlois A, et al. Impact of Pancreatic Rat Islet Density on Cell Survival During Hypoxia. J Diabetes Res (2016) 2016:3615286. doi: 10.1155/2016/3615286
79. Kim DS, Song L, Wang J, Wu H, Gou W, Cui W, et al. Carbon Monoxide Inhibits Islet Apoptosis via Induction of Autophagy. Antioxid Redox Signal (2018) 28(14):1309–22. doi: 10.1089/ars.2016.6979
80. Lumley EC, Osborn AR, Scott JE, Scholl AG, Mercado V, McMahan YT, et al. Moderate Endoplasmic Reticulum Stress Activates a PERK and P38-Dependent Apoptosis. Cell Stress Chaperones (2017) 22(1):43–54. doi: 10.1007/s12192-016-0740-2
81. Cross SE, Richards SK, Clark A, Benest AV, Bates DO, Mathieson PW, et al. Vascular Endothelial Growth Factor as a Survival Factor for Human Islets: Effect of Immunosuppressive Drugs. Diabetologia (2007) 50(7):1423–32. doi: 10.1007/s00125-007-0670-8
82. Sigrist S, Mechine-Neuville A, Mandes K, Calenda V, Braun S, Legeay G, et al. Influence of VEGF on the Viability of Encapsulated Pancreatic Rat Islets After Transplantation in Diabetic Mice. Cell transplantation (2003) 12(6):627–35. doi: 10.3727/000000003108747109
83. Yamada S, Shimada M, Utsunomiya T, Ikemoto T, Saito Y, Morine Y, et al. Trophic Effect of Adipose Tissue-Derived Stem Cells on Porcine Islet Cells. J Surg Res (2014) 187(2):667–72. doi: 10.1016/j.jss.2013.10.031
84. Oliver-Krasinski JM, Stoffers DA. On the Origin of the Beta Cell. Genes Dev (2008) 22(15):1998–2021. doi: 10.1101/gad.1670808
85. Kaneto H, Nakatani Y, Miyatsuka T, Matsuoka TA, Matsuhisa M, Hori M, et al. PDX-1/VP16 Fusion Protein, Together With NeuroD or Ngn3, Markedly Induces Insulin Gene Transcription and Ameliorates Glucose Tolerance. Diabetes (2005) 54(4):1009–22. doi: 10.2337/diabetes.54.4.1009
86. Schwitzgebel VM, Scheel DW, Conners JR, Kalamaras J, Lee JE, Anderson DJ, et al. Expression of Neurogenin3 Reveals an Islet Cell Precursor Population in the Pancreas. Development (2000) 127(16):3533–42. doi: 10.1242/dev.127.16.3533
87. Sancho R, Gruber R, Gu G, Behrens A. Loss of Fbw7 Reprograms Adult Pancreatic Ductal Cells Into α, δ, and β Cells. Cell Stem Cell (2014) 15(2):139–53. doi: 10.1016/j.stem.2014.06.019
88. Piganelli JD, Mamula MJ, James EA. The Role of β Cell Stress and Neo-Epitopes in the Immunopathology of Type 1 Diabetes. Front Endocrinol (Lausanne) (2020) 11:624590. doi: 10.3389/fendo.2020.624590
89. Baekkeskov S, Hubbell JA, Phelps EA. Bioengineering Strategies for Inducing Tolerance in Autoimmune Diabetes. Adv Drug Deliv Rev (2017) 114:256–65. doi: 10.1016/j.addr.2017.06.007
Keywords: extracellular vesicle, type 1 diabetes, exosomes, β-cell, immunomodulation, therapy
Citation: Soltani S, Mansouri K, Emami Aleagha MS, Moasefi N, Yavari N, Shakouri SK, Notararigo S, Shojaeian A, Pociot F and Yarani R (2022) Extracellular Vesicle Therapy for Type 1 Diabetes. Front. Immunol. 13:865782. doi: 10.3389/fimmu.2022.865782
Received: 30 January 2022; Accepted: 17 March 2022;
Published: 08 April 2022.
Edited by:
Baharak Hosseinkhani, University of Hasselt, BelgiumReviewed by:
Kenneth Verboven, Universiteit Hasselt, BelgiumMario Galgani, University of Naples Federico II, Italy
Copyright © 2022 Soltani, Mansouri, Emami Aleagha, Moasefi, Yavari, Shakouri, Notararigo, Shojaeian, Pociot and Yarani. This is an open-access article distributed under the terms of the Creative Commons Attribution License (CC BY). The use, distribution or reproduction in other forums is permitted, provided the original author(s) and the copyright owner(s) are credited and that the original publication in this journal is cited, in accordance with accepted academic practice. No use, distribution or reproduction is permitted which does not comply with these terms.
*Correspondence: Reza Yarani, cmV6YS55YXJhbmkuMDFAcmVnaW9uaC5kaw==; cnlhcmFuaUBzdGFuZm9yZC5lZHU=