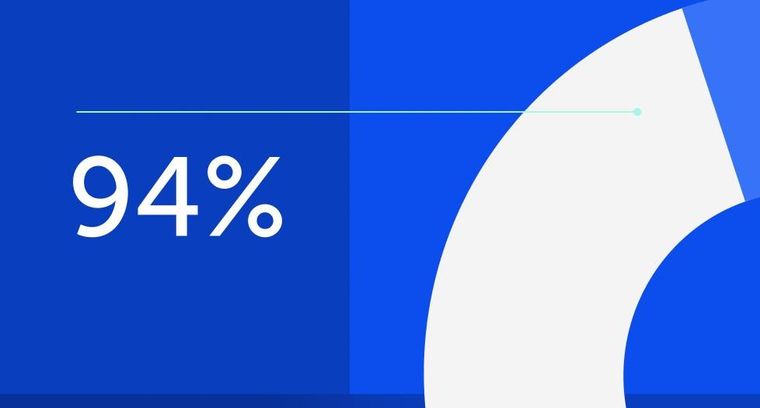
94% of researchers rate our articles as excellent or good
Learn more about the work of our research integrity team to safeguard the quality of each article we publish.
Find out more
REVIEW article
Front. Immunol., 25 March 2022
Sec. NK and Innate Lymphoid Cell Biology
Volume 13 - 2022 | https://doi.org/10.3389/fimmu.2022.859177
This article is part of the Research TopicTranslating NK Cell Scientific Research to Clinical Product ManufacturingView all 10 articles
Natural killer cells constitute a part of the innate immune system that mediates an effective immune response towards virus-infected and malignant cells. In recent years, research has focused on exploring and advancing NK cells as an active immunotherapy platform. Despite major advances, there are several key challenges that need to be addressed for the effective translation of NK cell research to clinical applications. This review highlights some of these challenges and the innovative strategies being developed to overcome them, including in vitro expansion, in vivo persistence, infiltration to the tumor site, and prevention of exhaustion.
Natural killer (NK) cell-based therapies are a promising modality for the treatment of liquid and solid tumors. With their intrinsic ability to mediate both cytotoxicity as well as cytokine secretion that helps mobilize other immune effector cells, NK cells are a powerful tool in the armamentarium of cancer immunotherapy (1, 2). While the initial recognition of their anti-tumor efficacy came from studies of allogeneic stem cell transplantation, numerous other studies have demonstrated their cytotoxic potential against tumor targets even in non-transplant settings (3, 4). In recent years, great progress has been made in the ex vivo activation and expansion of NK cells for clinical applications, as well as their manipulation with exogenous cytokines and genetic methods to further enhance cancer targeting (5–8). While this progress has led to the translation of several NK cell-based immunotherapies into early phase clinical trials, ongoing studies aim to overcome persistent challenges with this therapy. The purpose of this review is to provide an overview of the strategies being developed to address these challenges, including optimization of NK cell expansion, persistence, trafficking, and anti-tumor activity in the tumor microenvironment (TME).
The major goal for NK cell expansion strategies is to generate large numbers of these cells for clinical applications that retain high anti-tumor activity. With substantial NK cells, it is possible to achieve higher desirable NK effector to target ratios, perform multiple infusions, and potentially allow for the development of off-the-shelf NK cell products. There are multiple NK cell expansion strategies and sources for generating clinical grade NK cell products including peripheral blood (PB), cord blood (CB), bone marrow (BM), and human induced pluripotent stem cells (iPSC) (Figure 1) (9). NK cells can be expanded ex vivo before infusion into patients, or in vivo after adoptive transfer with cytokine stimulation. They are typically isolated by CD3+ cell depletion followed by positive selection of CD56+ cells, or in some protocols by single step depletion of CD3+ and CD19+ cells using magnetic beads (CliniMACS) (10). Another approach includes differentiation of functional NK cells from enriched CD34+ progenitors present in CB and BM (Table 1) (11, 29).
Figure 1 Overview of NK cell isolation, expansion, and in vivo persistence. NK cells can be isolated and/or generated from peripheral blood (PB), cord blood (CB), or human induced pluripotent stem cell (iPSC) sources. They are expanded ex vivo using various strategies including cytokine combinations, feeder cells, lipid membranes or biomaterials that carry surface markers to provide biochemical cues. In vivo persistence of NK cells can be accomplished using cytokines like IL-15 and/or cytokine-induced memory-like (CIML) NK cells that have shown long term persistence in an immune compatible environment. Illustration created using BioRender.com.
Table 1 Advantages and disadvantages of different methods of NK cell expansion for adoptive transfer studies.
Although good manufacturing practice guidelines have been defined for NK cell products for clinical applications, the source of NK cells does result in some important differences with respect to NK cell numbers and phenotype (Table 1) (15, 30). It is currently not clear if these differences impact clinical outcomes. For example, while CB has a higher proportion of NK cells than PB, the total NK numbers that can be enriched from CB are significantly lower. Similarly the proportion of less mature CD56bright cells is higher in CB as compared to PB (11). NK cells can also be generated via differentiation of CB-derived CD34+ cells. This process yields a greater expression of CXCR4 on the differentiated cells that mediates bone marrow homing (31). Killer Ig-like receptor (KIR) expression is lower when CD34+ progenitors are differentiated into NK cells that may impact their tumor-targeting ability (20). Furthermore, while CB-derived NK cells seem to have comparable cytokine secretion in response to the in vitro target cell stimulation, they exhibit lower cytotoxicity than PB-derived NK cells (11).
Differentiation and expansion of NK cells from iPSC is another promising approach that has seen significant advancement for clinical application in recent years (13, 14). While the generation of a sufficiently large number of NK cells from iPSC requires a longer time than PB, it is particularly attractive in terms of establishing well characterized master banks, performing multiple gene edits (including CAR arming and CRISPR) and generating functional immune effector cells in large numbers for off-the-shelf use. iPSC-derived NK cells exhibit comparable cytokine-secreting and cytotoxic capacity to PB-derived sources. They are able to infiltrate tumor spheroids in vitro, interact with other effector T-cells in mouse models and are amenable to gene editing with CRISPR technology (13, 32). A phase 1 clinical trial evaluating the feasibility and efficacy of iPSC-derived NK cells is currently ongoing (NCT03841110).
Traditionally IL-2 and more recently IL-15 have been added to culture media to support activation and expansion of purified NK cells (21, 33). IL-2 promotes NK cell expansion and survival as both CD56dim and CD56bright subpopulations express the high affinity IL-2 receptor (34). However, the effect of IL-2 is not specific to NK cells and may also expand regulatory T-cells (Tregs) (35). IL-15, while sharing similar signaling functions with IL-2, is selective in activating NK cells and CD8+ T cells, and is therefore preferable for NK cell activation and expansion efforts (34).
Other ex vivo expansion methods have been developed, including the use of feeder cells and biomaterials (Figure 1). The use of membrane bound IL-15 and 4-1BB on K562 feeder cells have been shown to result in significant expansion of NK cells (36–38). A similar method incorporating membrane-bound IL-21 instead of IL-15 on K562 feeder cells (K562-mbIL21) has the advantage of preserving the telomere length of expanded NK cells, thereby resulting in the infusion of cells with a more sustained proliferative potential (7, 39, 40). A phase 1 trial of adoptively transferred K562-mbIL21 expanded NK cells infused peri-transplant has shown such an approach to be safe and promising (41, 42). Another study evaluated a similar approach of infusing NK cells expanded with cell-free liposomal plasma membrane particles containing mbIL21 and 4-1BB (43). Other feeder cell types have been developed including the Epstein-Barr lymphoblastoid cell line and human B-lymphoblastoid cell line 721.221, with variable effects on the expansion of NK cells while preventing the concurrent expansion of T-cells (44, 45).
Along with targeted drug delivery, unique properties of biomaterials can be exploited for cancer immunotherapy, especially to support the activation and expansion of immune cells. Advances in the engineering of biomaterials have helped to study the interaction of immune cells and precise control of their immunomodulatory functions (46). Various biomaterials including lipid bilayers and nano/micropatterns have been used to study the fundamental mechanisms of immune cell-target interactions in NK cells, providing new insights on the interaction of putative NK ligands with specific activating or inhibitory receptors (22, 47–49). In addition, these materials can be engineered to provide synthetic molecular, physical, and biochemical cues to support the growth of NK cells. Specifically, the surface of the scaffolds generated from these materials can be modified/decorated with specific receptors supporting NK cell expansion or loaded with chemokines/cytokines for their sustained release. Such material designs can provide both co-stimulatory signals and cytokine support for precise immunomodulation, aimed at providing cells with a synthetic environment similar to their natural biological environment (50).
Various materials have already been designed and investigated for T cell expansion including polymer beads, alginate scaffold, mesoporous silica rods (MSR), polyisocyanopeptide scaffold, and hydrogels (51). Although there are limited studies of such scaffold based systems for NK cells, some studies used CD16-functionalized graphene oxide nanoclusters for the in vitro activation of NK cells (52). In a recent study by Ahn et al, NK cells were expanded using a hyaluronic acid-based biodegradable polymeric scaffold with macro-porous 3D structure (25). The macro-porous architecture supported the cluster formation of loaded NK cells resulting in their proliferation and persistence (25). For the clinical translation of biomaterials, key parameters need to be considered including material composition, biodegradability and the toxicity of chemicals post-degradation, and intrinsic immunogenicity. In addition, the physiochemical properties like surface area and topology, porosity, and ease of functionalization of the material are vital for their application (51, 53).
The efficacy of NK cell therapy depends in part on the ability of the infused cells to persist in vivo after adoptive transfer (54). While NK cells could be expanded in large numbers ex vivo, following infusion these NK cells exhibit limited in vivo persistence and expansion (55). Efforts to expand and activate NK cells after their adoptive transfer have typically relied on the cytokines IL-2 or IL-15, the latter being somewhat favored because of its greater selectivity for NK cells (56). A super-agonist complex (ALT-803) of an IL-15 mutein bound to the sushi domain of the IL-15 receptor alpha (IL-15Rα) and fused to the immunoglobulin G1 Fc region has been generated that in addition to having a longer half-life and improved functionality over the individual cytokine (57), resulted in marked expansion and activation of NK cells in a phase I clinical trial (58).
The expansion of NK cells using feeder cell lines such as K562, Epstein-Barr lymphoblastoid cell line, and human B-lymphoblastoid cell line 721.221 that either express membrane-bound cytokines or in co-culture with exogenously administered cytokines such as IL-21 are methods developed for the generation of activated NK cells with a potential for long-term persistence in vivo (39, 41, 43–45, 59). In addition, genetic manipulation of NK cells via viral and non-viral techniques for autocrine expression of cytokines (IL-15 or IL-2) has been shown to increase the cells’ persistence and expansion post infusion (60).
Differentiation into a memory-like phenotype is another novel approach that results in normally short-lived NK cells persisting for much longer periods with significantly increased in vivo expansion and anti-tumor responses (6, 21, 61–63). Cytokine induced memory-like (CIML) NK cells generated after brief activation with cytokines IL-12, IL-15, and IL-18 exhibit this unique memory-like phenotype (Figure 1). In preclinical and recent clinical settings, CIML NK cells have demonstrated massive expansion, prolonged persistence, and reduced threshold for activation in response to tumor targets (64).
Ongoing clinical studies have shown that the prolonged persistence of the adoptively transferred CIML NK cells is associated with the attainment of clinical responses (6, 21, 61–63). Upon their adoptive transfer into an immune compatible environment in the context of an allogeneic HCT in a phase 1 clinical trial, the infusion of CIML NK cells resulted in a massive expansion of the NK cell compartment that persisted for up to 6 months (26). The use of the CIML NK platform for the prevention of post-allogeneic HCT relapse of myeloid disease is concurrently being explored (65).
In contrast to hematological cancers, solid tumors are characterized by a dense, highly heterogeneous, stroma-rich TME within which crosstalk between the cancer cells and their microenvironment helps drive tumor progression, invasion, and metastasis (66, 67). The TME is composed of cellular and acellular components which often hinder the delivery of small molecules and infiltration of infused adoptive cells (68, 69). NK cell infiltration in the tumor is hindered by lack of chemotactic signaling and activation, as well as by the physical barriers in the TME due to hypo-vasculature and dense extracellular matrix (ECM) deposition (69). In addition, myeloid-derived suppressor cells (MDSCs), tumor associated macrophages (TAMs) and Tregs are often enriched within the advanced tumor sites, and play a key role in inhibiting immune effector cells such as NK and CD8+ T cells (66). The tumor-stromal interaction results in the release of immunosuppressive molecules including IL-10 and TGFβ, the downregulation of antigens targeted by immune effector cells and the upregulation of checkpoint blockade proteins. All these molecules shape the TME to be highly immune-suppressive, thereby hampering effective antitumor immune responses (66). To mediate optimal anti-tumor responses in the solid tumor setting, circulating NK cells need to exit the bloodstream, traffic to tumor sites that include distant metastatic sites, penetrate through the dense ECM, and finally interact with and respond to the tumor cells. Previous reports have shown that only a minority of adoptively-transferred lymphocytes traffic to the tumor sites (70, 71).Various strategies have been investigated to potentially overcome this major barrier, including utilizing chemokine networks for efficient NK cell infiltration into the TME, imparting features to NK cells to overcome the immunosuppressive TME, as well as altering the TME to create a favorable niche for the adoptively transferred immune cells (72).
Interaction between soluble chemokines and their receptors play an essential role in the migration of lymphocytes (73). The role of these receptors on NK cells, including CCR2, CCR5, and CXCR3 was described in a recent review (73). CCR2 has been shown to promote the recruitment of NK cells into lymph nodes infiltrated with melanoma (74). The expression of CCR5 on infiltrating immune cells was correlated with high levels of CCL5 in tumors, and was associated with improved overall survival (75). Alteration of the chemokine receptor profile on NK cells has been shown to drive their migration and infiltration into solid tumors (Figure 2). For example, autophagy inhibition in melanoma leading to PPA2-dependent upregulation of JNK phosphorylation led to increased expression of CCL5 on tumor cells, which in turn increased infiltration of CCR5 expressing NK cells into the TME (73). The latter was associated with improved outcomes in preclinical studies (76).
Figure 2 Strategies involved to improve the tumor infiltration of NK cells, overcome tumor escape and exhaustion. NK cells can be engineered to express CAR and specific chemokine receptors to increase their infiltration in solid tumors. Upon entry into the TME, NK cells can be impaired by its immune suppressive features. Blockade of inhibitory receptors (TIM-3, NKG2A, KIR) on NK cells can increase their antitumor cytotoxicity. Further, inhibition of immune-modulatory molecules (TGF-β) can prevent exhaustion in NK cells and maintain their cytotoxic features. Illustration created using BioRender.com.
Based on the recent advances in our understanding of chemokine networks, NK cells have been genetically engineered with altered expression of chemokine receptors to modulate their homing into solid tumors or metastatic sites (64). Li et al. showed that transduction of primary NK cells with CCR5 resulted in their increased infiltration and subsequent anti-tumor activity in colorectal cancer cells-derived xenograft mice (77). On the other hand, NK cells expanded in the presence of K562 feeder cells engineered to express CCR7 resulted in the acquisition of CCR7 receptors by NK cells via trogocytosis (78). These CCR7+ NK cells, when adoptively transferred into athymic nude mice, exhibited enhanced homing into lymph nodes and increased cytotoxicity against tumor cells (78). In another study, the increased expression of CXCR1 in NK cells resulted in increased migration and infiltration into subcutaneous and intraperitoneal tissue in a model of ovarian cancer (79). Similarly, NK-92 and primary NK cells transduced to overexpress CXCR2 improved their migration and accumulation at sites of disease in models of renal cell carcinoma and lung cancer metastasis, respectively (80). Yang et al. demonstrated that more than one chemokine pathway is needed for the successful infiltration of NK cells in solid tumors (78). The authors showed that increased expression of CXCR4 and CCR7 on NK-92 cells significantly increased their migration in a colon cancer model (81). The level of chemokine receptor expression could also be induced by cytokine activation. For example, expansion of NK cells in the presence of IL-2 significantly increased CXCR3 expression resulting in increased migration towards CXCL10-producing tumor cells (82). In addition to cytokines, treatment of NK cells with TGFβ1 was reported to modulate the expression of chemokine receptors including CXCR3/CXCR4 which further increased their infiltration in tumors (83).
Another approach for enhancing NK cell infiltration into the tumor sites involves manipulation of the immune-suppressive TME to create a favorable niche for infused NK cells (73). A number of studies have demonstrated that intratumorally injected IFNγ induce expression of CXCL9, CXCL10, and CXCL11 in the TME, which in turn increases the local infiltration of NK cells (84). Park et al. developed a very interesting strategy wherein immunomodulatory microspheres, composed of biodegradable PLGA polymer, were engineered to release IFNγ into the TME (85). Intra-arterial transcatheter delivery of these microspheres resulted in local delivery of IFNγ and a significant increase in the NK cell infiltration as demonstrated in an orthotopic liver cancer model (85). Similarly, intratumoral injection of recombinant human IL-12 in patients with head and neck squamous cell carcinoma (HNSCC) showed increased NK cells and IFNγ expression in the tumors and lymph nodes compared to the control arm, which correlated to the overall survival (86).
Augmentation of NK cell targeting of tumors can be accomplished with a chimeric antigen receptor (CAR) (60, 87, 88). With recent improvements in the efficiency of retroviral transduction of NK cells and the engineering of CARs, this approach has become a reality. The promise of this approach was demonstrated with the use of CAR-transduced NK cells in advanced lymphoma patients (60). NK cells were purified from CB, expanded with IL-21 and 4-1BB expressing K562 feeder cells in the presence of IL-2, and subsequently infused into patients following lymphodepleting chemotherapy. Infusion of these cells was associated with clinical responses in 8/11 patients. Most responses to CAR-NK occurred within the first 1-2 months and attainment of negative minimal residual disease (MRD) complete remission appeared more likely at the higher infused cell doses. The presence of the CAR was detectable for several months after infusion, although the absolute number of persistent NK cells was not quantified in this study. As the maximum tolerated dose of infused CAR-NK cells was not reached in this trial and a higher effector-to-target ratio plays a role in NK cell efficacy, it is possible that a higher infused NK cell dose or improvement in their in vivo persistence may result in the attainment of more sustained MRD-negative CR (60).
Recently there have been efforts to use gene-edited iPSCs for differentiation into NK cell CARs (89, 90). iPSC-derived NK cells can more stably express CARs, and may serve as a better off-the-shelf option for the generation of CAR-NK than CB (13, 14, 20). Whether their more immature phenotype and generally lower KIR expression would be a barrier to efficacy in the context of a CAR remains to be determined. Furthermore, current active research aims to use CIML NK cells as a platform for CAR generated products, with their enhanced cytotoxicity and long-term persistence potentially augmented via the incorporation of a CAR (27, 64). Recent progress and improvements in CAR-NK cell technology have been reviewed in detail by our group and others (87, 88, 91). In addition to CAR, genetic engineering of NK cells has been useful to increase their antitumor efficiency as recently reviewed by our group and others (88, 92).
Early phase NK cell clinical trials have shown favorable safety profiles and promising responses using advances in NK cell engineering, including the differentiation into memory-like phenotype or arming with CARs. However, these studies have a common feature of an initial reduction of tumor burden followed by eventual reappearance of detectable disease in the majority of treated patients (21, 60, 93). These observations can be partially attributed to the tumor escape mechanism and exhaustion of adoptive NK cells (72).
The transformation of normal cells to malignant cells requires elaborate adaptations and oncogenic changes that allow a tumor to bypass detection and elimination by immune effector cells (94). Some of these adaptations involve downregulation or shedding of ligands that activate NK cells (e.g. MICA/B), release of immune-modulatory molecules from tumor cells or supporting stromal cells (e.g. TGFβ), and epigenetic changes (e.g. histone deacetylases, HDACs) (95, 96). Escape of tumors from NK cells must be overcome for NK cell-mediated therapies to become standalone treatments without the need for subsequent consolidation with other therapies. Current strategies to overcome tumor escape include but are not limited to the reversal of NK cell anergy, the combination of NK cell therapy with antibody-mediated therapy that can engage their ADCC function, and the development of off-the-shelf NK cells that may be transduced with CARs that can target escaping tumor cells (Figure 2) (72).
A lack of the KIR-HLA class I interaction (KIR-ligand mismatch) is associated with enhanced NK activity, and one strategy used by tumors to escape NK cell mediated effects involves the increased expression of HLA class I molecules on their surface (97). To overcome this, anti-KIR antibodies such as IPH2101 (previously known as 1-7F9) that bind the inhibitory KIR2DL1, -L2 and -L3 as well as the activating KIR2DS1 and -S2, and interefere with the receptors’ interaction with HLA-C have been developed (98). Lirilumab, a fully human IgG4 anti-KIR2D monoclonal antibody that recognizes the same epitope as IPH2101 and in a similar manner blocks the KIR interaction with HLA-C, has been well-tolerated in early phase clinical trials (99, 100) and has shown some clinical activity when combined with azacytidine in high risk myelodysplastic syndrome (MDS) (101). Similarly, the anti-NKG2A antibody monalizumab has been shown to increase the effector function of NK cells, and its combination with PD-1/PD-L1 blockade yielded evidence of clinical activity in early phase trials (102, 103). An alternative approach to blocking antibodies that targets the KIR-HLA class I interaction is to genetically engineer the NK cells to downregulate inhibitory signals including NKG2A and KIRs (104–107).
The antitumor response of NK cells can also be manipulated using monoclonal antibodies engaging activating receptors like CD16A, NKG2D, and NKp46 (108–110). Antibody dependent cellular cytotoxicity (ADCC) of the target cells coated with IgG molecules is mediated by NK cells through engagement of CD16 (Fc receptor) on NK cells (111). Based on a similar concept, novel NK cell engagers (NKCE), synthetic molecules composed of the fragments of monoclonal antibodies that simultaneously engage NK activating receptors and tumor antigens, have been designed (112, 113). Bispecific killer cell engages (BiKEs) containing ScFv fragments that target CD16 and a tumor antigen are one example of this, and have been used to target EpCAM on carcinomas (114), CD133 on cancer stem cells (115), and CD30 on lymphomas (116). AFM13 (CD16/CD30) is the first-in-class tetravalent bispecific molecule targeting CD30-positive lymphoma, and has shown promising antitumor responses in recent clinical trials when used as a monotherapy as well as in combination with allogenic NK cells (116, 117). This molecule belongs to the class of redirected optimized cell killing (ROCK) platform, which is equipped with high affinity towards specific epitopes on CD16, is independent of individuals’ CD16A allotype, not inhibited by serum IgG, and also prevents NK fratricide (118).
Similarly, trispecific killer engagers (TriKE) have been developed that consist of two scFv fragments connected by an IL-15 domain, enhancing NK cell-mediated anti-tumor efficacy by engaging CD16 on the NK cells and an antigen on the tumor cells. The GTB-3550 TriKE (CD16/IL-15/CD33), for example, has been shown to enhance NK cell proliferation and function, and is being evaluated in clinical trials (119). Other TriKE molecules targeting CD133 and B7-H3 have also shown promising efficacy in cell lines and mouse models (120, 121). The targeting of CD16 on NK cells by BiKEs and TriKEs may be limited by the known process of CD16 shedding (122). However, the combination of the NKCE with novel approaches that interfere with the shedding process are being investigated (113).
While engagement of CD16 has been one successful strategy of NKCE, other engagers have been designed to bridge the NKG2D activating receptor with tumor targets such as CS1 on multiple myeloma (MM) and HER2 on breast cancer cells (123, 124). Alternative NKCE designs, such as those bridging NKp30 and NKp46 with tumor antigens for example, have been reviewed recently (112). Trifunctional NKCE that co-engage two different NK activating receptors, such as CD16 and NKp46, with a tumor target antigen have also been developed, and have shown impressive in vitro activity and suppression of tumor growth in mouse models (110).
A plethora of immune-suppressive pathways in the TME combine to inhibit NK cell function. Exhausted or impaired NK cells in the TME exhibit downregulation of effector cytokines, decreased granulation, downregulation of NKG2D, a decrease in transcription factors like Eomesodermin and T-bet (125), and upregulation of inhibitory receptors such as PD-1, TIM-3, TIGIT and NKG2A (72). Expression of the latter markers on NK cells have been correlated with decreased NK cell functionality and importantly, various studies demonstrate that blockade of these receptors can increase NK cell cytotoxicity and function (72, 126).
TGF-β is one of the important cytokines that plays a critical role in the immune-suppressive TME. It limits NK cell function by inhibiting the T-bet transcription factor (SMAD3) and by targeting the mTOR pathway (127). In addition, TGF-β has been reported to contribute to the selective recruitment of non-cytotoxic CD56bright NK cells by modulating the chemokine repertoire in tumors (127). To potentially overcome TGF-β mediated immune suppression, Otegbeye et al. combined ex vivo expanded NK cells with the TGF-β inhibitor LY2157299, resulting in a significant decrease in liver metastasis as observed in a colon cancer model (128). Another strategy by Burga et al. was to engineer a variant of the TGF-β receptor where the receptor domain was coupled with NK specific activating domains (129). The authors showed that these engineered NK cells could efficiently convert inhibitory TGF-β signals into activating signals leading to augmented cytotoxic function in a neuroblastoma model (129).
Impaired cellular metabolism in the nutrient-poor TME is another important factor responsible for the dysregulation of NK cells (130). Glycolysis is a critical metabolic process for NK cells, especially for ex vivo expanded NK cells. A study by Cong et al. showed that aberrant expression of fructose bisphosphatase 1 (FBP1) in intratumoral NK cells impaired their cytotoxic function and could be a potential target to improve NK-based therapy (131). Recently, cytokine-inducible SH2-containing protein (CISH)-knockout in iPSC-derived NK cells using the CRISPR/cas9 system exhibited improved metabolic fitness as exemplified by increased basal glycolysis and mitochondrial activity, and by increased mTOR signaling which contributed to the enhanced NK cell function (132). Overall, metabolic reprograming of the TME or adoptive NK cells is a promising strategy to prevent NK cell dysfunction.
NK cells have emerged as an attractive platform for adoptive cell immunotherapy in recent years. Key advances, including the development of efficient ex vivo expansion systems, the use of CIML NK cells for prolonged in vivo persistence and genetic manipulations strategies involving CARs have helped to catapult the field toward more effective clinical translation. However, more work needs to be done to further enhance adoptively transferred NK cells’ tumor targeting, overcome their immune suppression and exhaustion in the TME, increase their persistence in an allogeneic setting, and augment their capacity for long-term surveillance against tumor relapse. Taking advantage of recent advances in our understanding of basic NK cell biology and genetic manipulation techniques, we expect a bright future for the NK cell immunotherapy field.
MT, RR, and RS conceived of the topics to include in the review. MT and RS wrote the manuscript. MT designed the figures. RR provided critical edits to the manuscript. All authors approved of the final version.
Funding support was provided by the Michelle D. and Douglas W. Bell Fund for Stem Cell Transplant (grant# 9618342) and the Ted and Eileen Pasquarello Research Fund (grant #9616306).
The authors declare that the research was conducted in the absence of any commercial or financial relationships that could be construed as a potential conflict of interest
All claims expressed in this article are solely those of the authors and do not necessarily represent those of their affiliated organizations, or those of the publisher, the editors and the reviewers. Any product that may be evaluated in this article, or claim that may be made by its manufacturer, is not guaranteed or endorsed by the publisher.
1. Hu Y, Tian Z, Zhang C. Natural Killer Cell-Based Immunotherapy for Cancer: Advances and Prospects. Engineering (2019) 5:106–14. doi: 10.1016/j.eng.2018.11.015
2. Liu S, Galat V, Galat4 Y, Lee YKA, Wainwright D, Wu J. NK Cell-Based Cancer Immunotherapy: From Basic Biology to Clinical Development. J Hematol Oncol (2021) 14:7. doi: 10.1186/s13045-020-01014-w
3. Ruggeri L, Capanni M, Urbani E, Perruccio K, Shlomchik WD, Tosti A, et al. Effectiveness of Donor Natural Killer Cell Alloreactivity in Mismatched Hematopoietic Transplants. Science (2002) 295:2097–100. doi: 10.1126/science.1068440
4. Ruggeri L, Mancusi A, Burchielli E, Capanni M, Carotti A, Aloisi T, et al. NK Cell Alloreactivity and Allogeneic Hematopoietic Stem Cell Transplantation. Blood Cells Mol Dis (2008) 40:84–90. doi: 10.1016/j.bcmd.2007.06.029
5. Lee DA. Cellular Therapy: Adoptive Immunotherapy With Expanded Natural Killer Cells. Immunol Rev (2019) 290:85–99. doi: 10.1111/imr.12793
6. Romee R, Schneider SE, Leong JW, Chase JM, Keppel CR, Sullivan RP, et al. Cytokine Activation Induces Human Memory-Like NK Cells. Blood (2012) 120:4751–60. doi: 10.1182/blood-2012-04-419283
7. Denman CJ, Senyukov VV, Somanchi SS, Phatarpekar PV, Kopp LM, Johnson JL, et al. Membrane-Bound IL-21 Promotes Sustained Ex Vivo Proliferation of Human Natural Killer Cells. PloS One (2012) 7:e30264. doi: 10.1371/journal.pone.0030264
8. Yilmaz A, Cui H, Caligiuri MA, Yu J. Chimeric Antigen Receptor-Engineered Natural Killer Cells for Cancer Immunotherapy. J Hematol Oncol (2020) 13:168. doi: 10.1186/s13045-020-00998-9
9. Kundu S, Gurney M, O’Dwyer M. Generating Natural Killer Cells for Adoptive Transfer: Expanding Horizons. Cytotherapy (2021) 23:559–66. doi: 10.1016/j.jcyt.2020.12.002
10. Eissens DN, Schaap NPM, Preijers FWMB, Dolstra H, van Cranenbroek B, Schattenberg AVM, et al. CD3+/CD19+-Depleted Grafts in HLA-Matched Allogeneic Peripheral Blood Stem Cell Transplantation Lead to Early NK Cell Cytolytic Responses and Reduced Inhibitory Activity of NKG2A. Leukemia (2010) 24:583–91. doi: 10.1038/leu.2009.269
11. Sarvaria A, Jawdat D, Madrigal JA, Saudemont A. Umbilical Cord Blood Natural Killer Cells, Their Characteristics, and Potential Clinical Applications. Front Immunol (2017) 8:329. doi: 10.3389/fimmu.2017.00329
12. Chouaib S, Pittari G, Nanbakhsh A, El Ayoubi H, Amsellem S, Bourhis J-H, et al. Improving the Outcome of Leukemia by Natural Killer Cell-Based Immunotherapeutic Strategies. Front Immunol (2014) 5:95. doi: 10.3389/fimmu.2014.00095
13. Cichocki F, Bjordahl R, Gaidarova S, Mahmood S, Abujarour R, Wang H, et al. iPSC-Derived NK Cells Maintain High Cytotoxicity and Enhance In Vivo Tumor Control in Concert With T Cells and Anti-PD-1 Therapy. Sci Transl Med (2020) 12(586). doi: 10.1126/scitranslmed.aaz5618
14. Hermanson DL, Bendzick L, Pribyl L, McCullar V, Vogel RI, Miller JS, et al. Induced Pluripotent Stem Cell-Derived Natural Killer Cells for Treatment of Ovarian Cancer. Stem Cells (2016) 34:93–101. doi: 10.1002/stem.2230
15. Granzin M, Wagner J, Köhl U, Cerwenka A, Huppert V, Ullrich E. Shaping of Natural Killer Cell Antitumor Activity by Ex Vivo Cultivation. Front Immunol (2017) 8:458. doi: 10.3389/fimmu.2017.00458
16. Lupo KB, Matosevic S. Natural Killer Cells as Allogeneic Effectors in Adoptive Cancer Immunotherapy. Cancers (Basel) (2019) 11(6):769. doi: 10.3390/cancers11060769
17. Heinze A, Grebe B, Bremm M, Huenecke S, Munir TA, Graafen L, et al. The Synergistic Use of IL-15 and IL-21 for the Generation of NK Cells From CD3/CD19-Depleted Grafts Improves Their Ex Vivo Expansion and Cytotoxic Potential Against Neuroblastoma: Perspective for Optimized Immunotherapy Post Haploidentical Stem Cell Trans. Front Immunol (2019) 10:2816. doi: 10.3389/fimmu.2019.02816
18. Williams SM, Sumstad D, Kadidlo D, Curtsinger J, Luo X, Miller JS, et al. Clinical-Scale Production of cGMP Compliant CD3/CD19 Cell-Depleted NK Cells in the Evolution of NK Cell Immunotherapy at a Single Institution. Transfusion (2018) 58:1458–67. doi: 10.1111/trf.14564
19. Moretta F, Petronelli F, Lucarelli B, Pitisci A, Bertaina A, Locatelli F, et al. The Generation of Human Innate Lymphoid Cells is Influenced by the Source of Hematopoietic Stem Cells and by the Use of G-CSF. Eur J Immunol (2016) 46:1271–8. doi: 10.1002/eji.201546079
20. Goldenson BH, Zhu H, Wang YM, Heragu N, Bernareggi D, Ruiz-Cisneros A, et al. Umbilical Cord Blood and iPSC-Derived Natural Killer Cells Demonstrate Key Differences in Cytotoxic Activity and KIR Profiles. Front Immunol (2020) 11:561553. doi: 10.3389/fimmu.2020.561553
21. Romee R, Rosario M, Berrien-Elliott MM, Wagner JA, Jewell BA, Schappe T, et al. Cytokine-Induced Memory-Like Natural Killer Cells Exhibit Enhanced Responses Against Myeloid Leukemia. Sci Transl Med (2016) 8:357ra123. doi: 10.1126/scitranslmed.aaf2341
22. Keydar Y, Le Saux G, Pandey A, Avishay E, Bar-Hanin N, Esti T, et al. Natural Killer Cells’ Immune Response Requires a Minimal Nanoscale Distribution of Activating Antigens. Nanoscale (2018) 10:14651–9. doi: 10.1039/c8nr04038a
23. Oyer JL, Igarashi RY, Kulikowski AR, Colosimo DA, Solh MM, Zakari A, et al. Generation of Highly Cytotoxic Natural Killer Cells for Treatment of Acute Myelogenous Leukemia Using a Feeder-Free, Particle-Based Approach. Biol Blood Marrow Transplant J Am Soc Blood Marrow Transplant (2015) 21:632–9. doi: 10.1016/j.bbmt.2014.12.037
24. North J, Bakhsh I, Marden C, Pittman H, Addison E, Navarrete C, et al. Tumor-Primed Human Natural Killer Cells Lyse NK-Resistant Tumor Targets: Evidence of a Two-Stage Process in Resting NK Cell Activation. J Immunol (2007) 178:85–94. doi: 10.4049/jimmunol.178.1.85
25. Ahn YH, Ren L, Kim SM, Seo S-H, Jung C-R, Kim DS, et al. A Three-Dimensional Hyaluronic Acid-Based Niche Enhances the Therapeutic Efficacy of Human Natural Killer Cell-Based Cancer Immunotherapy. Biomaterials (2020) 247:119960. doi: 10.1016/j.biomaterials.2020.119960
26. Shapiro RM, Nikiforow S, Rambaldi B, Vergara J, Daley H, Kim HT, et al. Cytokine-Induced Memory-Like NK Cells Exhibit Massive Expansion and Long-Term Persistence After Infusion Post-Haploidentical Stem Cell Transplantation: A Report of the First Three Cases in a Phase I Trial. Blood (2020) 136:8–9. doi: 10.1182/blood-2020-133933
27. Dong H, Xie G, Liang Y, Dongjoo Ham J, Vergara J, Chen J, et al. Engineered Memory-Like NK Cars Targeting a Neoepitope Derived From Intracellular NPM1c Exhibit Potent Activity and Specificity Against Acute Myeloid Leukemia. Blood (2020) 136:3–4. doi: 10.1182/blood-2020-134148
28. Berraondo P, Sanmamed MF, Ochoa MC, Etxeberria I, Aznar MA, Pérez-Gracia JL, et al. Cytokines in Clinical Cancer Immunotherapy. Br J Cancer (2019) 120:6–15. doi: 10.1038/s41416-018-0328-y
29. Yang C, Siebert JR, Burns R, Gerbec ZJ, Bonacci B, Rymaszewski A, et al. Heterogeneity of Human Bone Marrow and Blood Natural Killer Cells Defined by Single-Cell Transcriptome. Nat Commun (2019) 10:3931. doi: 10.1038/s41467-019-11947-7
30. Childs RW, Berg M. Bringing Natural Killer Cells to the Clinic: Ex Vivo Manipulation. Hematol Am Soc Hematol Educ Progr (2013) 2013:234–46. doi: 10.1182/asheducation-2013.1.234
31. Luevano M, Daryouzeh M, Alnabhan R, Querol S, Khakoo S, Madrigal A, et al. The Unique Profile of Cord Blood Natural Killer Cells Balances Incomplete Maturation and Effective Killing Function Upon Activation. Hum Immunol (2012) 73:248–57. doi: 10.1016/j.humimm.2011.12.015
32. Karagiannis P, Kim S-I. iPSC-Derived Natural Killer Cells for Cancer Immunotherapy. Mol Cells (2021) 44:541–8. doi: 10.14348/molcells.2021.0078
33. Cooley S, He F, Bachanova V, Vercellotti GM, DeFor TE, Curtsinger JM, et al. First-In-Human Trial of rhIL-15 and Haploidentical Natural Killer Cell Therapy for Advanced Acute Myeloid Leukemia. Blood Adv (2019) 3:1970–80. doi: 10.1182/bloodadvances.2018028332
34. Waldmann TA. Cytokines in Cancer Immunotherapy. Cold Spring Harb Perspect Biol (2018) 10. doi: 10.1101/cshperspect.a028472
35. Sitrin J, Ring A, Garcia KC, Benoist C, Mathis D. Regulatory T Cells Control NK Cells in an Insulitic Lesion by Depriving Them of IL-2. J Exp Med (2013) 210:1153–65. doi: 10.1084/jem.20122248
36. Fujisaki H, Kakuda H, Imai C, Mullighan CG, Campana D. Replicative Potential of Human Natural Killer Cells. Br J Haematol (2009) 145:606–13. doi: 10.1111/j.1365-2141.2009.07667.x
37. Fujisaki H, Kakuda H, Shimasaki N, Imai C, Ma J, Lockey T, et al. Expansion of Highly Cytotoxic Human Natural Killer Cells for Cancer Cell Therapy. Cancer Res (2009) 69:4010–7. doi: 10.1158/0008-5472.CAN-08-3712
38. Lapteva N, Durett AG, Sun J, Rollins LA, Huye LL, Fang J, et al. Large-Scale Ex Vivo Expansion and Characterization of Natural Killer Cells for Clinical Applications. Cytotherapy (2012) 14:1131–43. doi: 10.3109/14653249.2012.700767
39. Kweon S, Phan M-TT, Chun S, Yu H, Kim J, Kim S, et al. Expansion of Human NK Cells Using K562 Cells Expressing OX40 Ligand and Short Exposure to IL-21. Front Immunol (2019) 10:879. doi: 10.3389/fimmu.2019.00879
40. Granzin M, Soltenborn S, Müller S, Kollet J, Berg M, Cerwenka A, et al. Fully Automated Expansion and Activation of Clinical-Grade Natural Killer Cells for Adoptive Immunotherapy. Cytotherapy (2015) 17:621–32. doi: 10.1016/j.jcyt.2015.03.611
41. Ciurea SO, Schafer JR, Bassett R, Denman CJ, Cao K, Willis D, et al. Phase 1 Clinical Trial Using Mbil21 Ex Vivo-Expanded Donor-Derived NK Cells After Haploidentical Transplantation. Blood (2017) 130:1857–68. doi: 10.1182/blood-2017-05-785659
42. Ciurea SO, Kongtim P, Soebbing D, Trikha P, Behbehani G, Rondon G, et al. Decrease Post-Transplant Relapse Using Donor-Derived Expanded NK-Cells. Leukemia (2022) 36:155–64. doi: 10.1038/s41375-021-01349-4
43. Vasu S, Bejanyan N, Devine S, Krakow E, Krakow E, Logan B, et al. BMT CTN 1803: Haploidentical Natural Killer Cells (CSTD002) to Prevent Post-Transplant Relapse in AML and MDS (NK-REALM). Blood (2019) 134:1955. doi: 10.1182/blood-2019-128005
44. Bae DS, Lee JK. Development of NK Cell Expansion Methods Using Feeder Cells From Human Myelogenous Leukemia Cell Line. Blood Res (2014) 49:154–61. doi: 10.5045/br.2014.49.3.154
45. Granzin M, Stojanovic A, Miller M, Childs R, Huppert V, Cerwenka A. Highly Efficient IL-21 and Feeder Cell-Driven Ex Vivo Expansion of Human NK Cells With Therapeutic Activity in a Xenograft Mouse Model of Melanoma. Oncoimmunology (2016) 5:e1219007. doi: 10.1080/2162402X.2016.1219007
46. Li J, Luo Y, Li B, Xia Y, Wang H, Fu C. Implantable and Injectable Biomaterial Scaffolds for Cancer Immunotherapy. Front Bioeng Biotechnol (2020) 8:612950. doi: 10.3389/fbioe.2020.612950
47. Zheng P, Bertolet G, Chen Y, Huang S, Liu D. Super-Resolution Imaging of the Natural Killer Cell Immunological Synapse on a Glass-Supported Planar Lipid Bilayer. J Vis Exp (2015) (96). doi: 10.3791/52502
48. Liu D, Bryceson YT, Meckel T, Vasiliver-Shamis G, Dustin ML, Long EO. Integrin-Dependent Organization and Bidirectional Vesicular Traffic at Cytotoxic Immune Synapses. Immunity (2009) 31:99–109. doi: 10.1016/j.immuni.2009.05.009
49. Delcassian D, Depoil D, Rudnicka D, Liu M, Davis DM, Dustin ML, et al. Nanoscale Ligand Spacing Influences Receptor Triggering in T Cells and NK Cells. Nano Lett (2013) 13:5608–14. doi: 10.1021/nl403252x
50. Anderson DG, Burdick JA, Langer R. Materials Science. Smart Biomaterials. Science (2004) 305:1923–4. doi: 10.1126/science.1099987
51. Schluck M, Hammink R, Figdor CG, Verdoes M, Weiden J. Biomaterial-Based Activation and Expansion of Tumor-Specific T Cells. Front Immunol (2019) 10:931:931. doi: 10.3389/fimmu.2019.00931
52. Loftus C, Saeed M, Davis DM, Dunlop IE. Activation of Human Natural Killer Cells by Graphene Oxide-Templated Antibody Nanoclusters. Nano Lett (2018) 18:3282–9. doi: 10.1021/acs.nanolett.8b01089
53. Mitragotri S, Lahann J. Physical Approaches to Biomaterial Design. Nat Mater (2009) 8:15–23. doi: 10.1038/nmat2344
54. Vahedi F, Nham T, Poznanski SM, Chew MV, Shenouda MM, Lee D, et al. Ex Vivo Expanded Human NK Cells Survive and Proliferate in Humanized Mice With Autologous Human Immune Cells. Sci Rep (2017) 7:12083. doi: 10.1038/s41598-017-12223-8
55. Nguyen R, Wu H, Pounds S, Inaba H, Ribeiro RC, Cullins D, et al. A Phase II Clinical Trial of Adoptive Transfer of Haploidentical Natural Killer Cells for Consolidation Therapy of Pediatric Acute Myeloid Leukemia. J Immunother Cancer (2019) 7:81. doi: 10.1186/s40425-019-0564-6
56. Fehniger TA, Cooper MA, Caligiuri MA. Interleukin-2 and Interleukin-15: Immunotherapy for Cancer. Cytokine Growth Factor Rev (2002) 13:169–83. doi: 10.1016/s1359-6101(01)00021-1
57. Romee R, Cooley S, Berrien-Elliott MM, Westervelt P, Verneris MR, Wagner JE, et al. First-In-Human Phase 1 Clinical Study of the IL-15 Superagonist Complex ALT-803 to Treat Relapse After Transplantation. Blood (2018) 131:2515–27. doi: 10.1182/blood-2017-12-823757
58. Xu W, Jones M, Liu B, Zhu X, Johnson CB, Edwards AC, et al. Efficacy and Mechanism-Of-Action of a Novel Superagonist Interleukin-15: Interleukin-15 Receptor αsu/Fc Fusion Complex in Syngeneic Murine Models of Multiple Myeloma. Cancer Res (2013) 73:3075 LP – 3086. doi: 10.1158/0008-5472.CAN-12-2357
59. Yang Y, Badeti S, Tseng H, Ma MT, Liu T, Jiang J-G, et al. Superior Expansion and Cytotoxicity of Human Primary NK and CAR-NK Cells From Various Sources via Enriched Metabolic Pathways. Mol Ther - Methods Clin Dev (2020) 18:428–45. doi: 10.1016/j.omtm.2020.06.014
60. Liu E, Marin D, Banerjee P, Macapinlac HA, Thompson P, Basar R, et al. Use of CAR-Transduced Natural Killer Cells in CD19-Positive Lymphoid Tumors. N Engl J Med (2020) 382:545–53. doi: 10.1056/NEJMoa1910607
61. Cooper MA, Elliott JM, Keyel PA, Yang L, Carrero JA, Yokoyama WM. Cytokine-Induced Memory-Like Natural Killer Cells. Proc Natl Acad Sci (2009) 106:1915–9. doi: 10.1073/pnas.0813192106
62. Berrien-Elliott MM, Cashen AF, Cubitt CC, Neal CC, Wong P, Wagner JA, et al. Multidimensional Analyses of Donor Memory-Like NK Cells Reveal New Associations With Response After Adoptive Immunotherapy for Leukemia. Cancer Discov (2020) 10:1854–71. doi: 10.1158/2159-8290.CD-20-0312
63. Shapiro RM, Birch G, Vergara J, Hu G, Nikiforow S, Baginska J, et al. Expansion, Persistence and Efficacy of Donor Memory-Like NK Cells for the Treatment of Post-Transplant Relapse. medRxiv (2021). doi: 10.1101/2021.08.24.21262547
64. Tarannum M, Romee R. Cytokine-Induced Memory-Like Natural Killer Cells for Cancer Immunotherapy. Stem Cell Res Ther (2021) 12:592. doi: 10.1186/s13287-021-02655-5
65. Foltz JA, Berrien-Elliott MM, Neal C, Foster M, McClain E, Schappe T, et al. Cytokine-Induced Memory-Like (ML) NK Cells Persist for \\< 2 Months Following Adoptive Transfer Into Leukemia Patients With a MHC-Compatible Hematopoietic Cell Transplant (HCT). Blood (2019) 134:1954. doi: 10.1182/blood-2019-126004
66. Labani-Motlagh A, Ashja-Mahdavi M, Loskog A. The Tumor Microenvironment: A Milieu Hindering and Obstructing Antitumor Immune Responses. Front Immunol (2020) 11:940. doi: 10.3389/fimmu.2020.00940
67. Giraldo NA, Sanchez-Salas R, Peske JD, Vano Y, Becht E, Petitprez F, et al. The Clinical Role of the TME in Solid Cancer. Br J Cancer (2019) 120:45–53. doi: 10.1038/s41416-018-0327-z
68. Melero I, Rouzaut A, Motz GT. Coukos G. T-Cell and NK-Cell Infiltration Into Solid Tumors: A Key Limiting Factor for Efficacious Cancer Immunotherapy. Cancer Discov (2014) 4:522–6. doi: 10.1158/2159-8290.CD-13-0985
69. Vitale M, Cantoni C, Pietra G, Mingari MC, Moretta L. Effect of Tumor Cells and Tumor Microenvironment on NK-Cell Function. Eur J Immunol (2014) 44:1582–92. doi: 10.1002/eji.201344272
70. Fisher B, Packard BS, Read EJ, Carrasquillo JA, Carter CS, Topalian SL, et al. Tumor Localization of Adoptively Transferred Indium-111 Labeled Tumor Infiltrating Lymphocytes in Patients With Metastatic Melanoma. J Clin Oncol Off J Am Soc Clin Oncol (1989) 7:250–61. doi: 10.1200/JCO.1989.7.2.250
71. Carrega P, Bonaccorsi I, Di Carlo E, Morandi B, Paul P, Rizzello V, et al. CD56(bright)perforin(low) Noncytotoxic Human NK Cells are Abundant in Both Healthy and Neoplastic Solid Tissues and Recirculate to Secondary Lymphoid Organs via afferent lymph. J Immunol (2014) 192:3805–15. doi: 10.4049/jimmunol.1301889
72. Ben-Shmuel A, Biber G, Barda-Saad M. Unleashing Natural Killer Cells in the Tumor Microenvironment–The Next Generation of Immunotherapy? Front Immunol (2020) 11:275. doi: 10.3389/fimmu.2020.00275
73. Yao X, Matosevic S. Chemokine Networks Modulating Natural Killer Cell Trafficking to Solid Tumors. Cytokine Growth Factor Rev (2021) 59:36–45. doi: 10.1016/j.cytogfr.2020.12.003
74. Ali TH, Pisanti S, Ciaglia E, Mortarini R, Anichini A, Garofalo C, et al. Enrichment of CD56(dim)KIR + CD57 + Highly Cytotoxic NK Cells in Tumour-Infiltrated Lymph Nodes of Melanoma Patients. Nat Commun (2014) 5:5639. doi: 10.1038/ncomms6639
75. Weiss ID, Shoham H, Wald O, Wald H, Beider K, Abraham M, et al. Ccr5 Deficiency Regulates the Proliferation and Trafficking of Natural Killer Cells Under Physiological Conditions. Cytokine (2011) 54:249–57. doi: 10.1016/j.cyto.2011.01.011
76. Mgrditchian T, Arakelian T, Paggetti J, Noman MZ, Viry E, Moussay E, et al. Targeting Autophagy Inhibits Melanoma Growth by Enhancing NK Cells Infiltration in a CCL5-Dependent Manner. Proc Natl Acad Sci USA (2017) 114:E9271–9. doi: 10.1073/pnas.1703921114
77. Li F, Sheng Y, Hou W, Sampath P, Byrd D, Thorne S, et al. CCL5-Armed Oncolytic Virus Augments CCR5-Engineered NK Cell Infiltration and Antitumor Efficiency. J Immunother Cancer (2020) 8:e000131. doi: 10.1136/jitc-2019-000131
78. Somanchi SS, Somanchi A, Cooper LJN, Lee DA. Engineering Lymph Node Homing of Ex Vivo-Expanded Human Natural Killer Cells via Trogocytosis of the Chemokine Receptor CCR7. Blood (2012) 119:5164–72. doi: 10.1182/blood-2011-11-389924
79. Ng YY, Tay JCK, Wang S. CXCR1 Expression to Improve Anti-Cancer Efficacy of Intravenously Injected CAR-NK Cells in Mice With Peritoneal Xenografts. Mol Ther Oncol (2020) 16:75–85. doi: 10.1016/j.omto.2019.12.006
80. Kremer V, Ligtenberg MA, Zendehdel R, Seitz C, Duivenvoorden A, Wennerberg E, et al. Genetic Engineering of Human NK Cells to Express CXCR2 Improves Migration to Renal Cell Carcinoma. J Immunother Cancer (2017) 5:73. doi: 10.1186/s40425-017-0275-9
81. Yang L, Huang C, Wang C, Zhang S, Li Z, Zhu Y, et al. Overexpressed CXCR4 and CCR7 on the Surface of NK92 Cell Have Improved Migration and Anti-Tumor Activity in Human Colon Tumor Model. Anticancer Drugs (2020) 31:333–44. doi: 10.1097/CAD.0000000000000868
82. Wennerberg E, Kremer V, Childs R, Lundqvist A. CXCL10-Induced Migration of Adoptively Transferred Human Natural Killer Cells Toward Solid Tumors Causes Regression of Tumor Growth In Vivo. Cancer Immunol Immunother (2015) 64:225–35. doi: 10.1007/s00262-014-1629-5
83. Castriconi R, Dondero A, Bellora F, Moretta L, Castellano A, Locatelli F, et al. Neuroblastoma-Derived TGF-β1 Modulates the Chemokine Receptor Repertoire of Human Resting NK Cells. J Immunol (2013) 190:5321–8. doi: 10.4049/jimmunol.1202693
84. Wendel M, Galani IE, Suri-Payer E, Cerwenka A. Natural Killer Cell Accumulation in Tumors Is Dependent on IFN-γ and CXCR3 Ligands. Cancer Res (2008) 68:8437–45. doi: 10.1158/0008-5472.CAN-08-1440
85. Park W, Gordon AC, Cho S, Huang X, Harris KR, Larson AC, et al. Immunomodulatory Magnetic Microspheres for Augmenting Tumor-Specific Infiltration of Natural Killer (NK) Cells. ACS Appl Mater Interfaces (2017) 9:13819–24. doi: 10.1021/acsami.7b02258
86. van Herpen CML, van der Laak JAWM, de Vries IJM, van Krieken JH, de Wilde PC, Balvers MGJ, et al. Intratumoral Recombinant Human Interleukin-12 Administration in Head and Neck Squamous Cell Carcinoma Patients Modifies Locoregional Lymph Node Architecture and Induces Natural Killer Cell Infiltration in the Primary Tumor. Clin Cancer Res an Off J Am Assoc Cancer Res (2005) 11:1899–909. doi: 10.1158/1078-0432.CCR-04-1524
87. Xie G, Dong H, Liang Y, Ham JD, Rizwan R, Chen J. CAR-NK Cells: A Promising Cellular Immunotherapy for Cancer. EBioMedicine (2020) 59:102975. doi: 10.1016/j.ebiom.2020.102975
88. Ali AK, Tarannum M, Romee R. Is Adoptive Cellular Therapy With Non–T-Cell Immune Effectors the Future? Cancer J (2021) 27(79):168–75. doi: 10.1097/PPO.0000000000000517
89. Boyd NR, Tiedemann M, Cartledge K, Cao M, Evtimov V, Shu R, et al. Off-The-Shelf Ipsc Derived Car-Nk Immunotherapy for Solid Tumors. Cytotherapy (2020) 22:S199. doi: 10.1016/j.jcyt.2020.04.067
90. Li Y, Hermanson DL, Moriarity BS, Kaufman DS. Human iPSC-Derived Natural Killer Cells Engineered With Chimeric Antigen Receptors Enhance Anti-Tumor Activity. Cell Stem Cell (2018) 23:181–192.e5. doi: 10.1016/j.stem.2018.06.002
91. Marofi F, Al-Awad AS, Sulaiman Rahman H, Markov A, Abdelbasset WK, Ivanovna Enina Y, et al. CAR-NK Cell: A New Paradigm in Tumor Immunotherapy. Front Oncol (2021) 11:673276. doi: 10.3389/fonc.2021.673276
92. Mantesso S, Geerts D, Spanholtz J, Kučerová L. Genetic Engineering of Natural Killer Cells for Enhanced Antitumor Function. Front Immunol (2020) 11:607131. doi: 10.3389/fimmu.2020.607131
93. Bednarski JJ, Zimmerman C, Berrien-Elliott MM, Foltz JA, Becker-Hapak M, Neal CC, et al. Donor Memory-Like NK Cells Persist and Induce Remissions in Pediatric Patients With Relapsed AML After Transplant. Blood (2021). doi: 10.1182/blood.2021013972
94. Vesely MD, Kershaw MH, Schreiber RD, Smyth MJ. Natural Innate and Adaptive Immunity to Cancer. Annu Rev Immunol (2011) 29:235–71. doi: 10.1146/annurev-immunol-031210-101324
95. Groth A, Klöss S, von Strandmann EP, Koehl U, Koch J. Mechanisms of Tumor and Viral Immune Escape From Natural Killer Cell-Mediated Surveillance. J Innate Immun (2011) 3:344–54. doi: 10.1159/000327014
96. Ge Z, Wu S, Zhang Z, Ding S. Mechanism of Tumor Cells Escaping From Immune Surveillance of NK Cells. Immunopharmacol Immunotoxicol (2020) 42:187–98. doi: 10.1080/08923973.2020.1742733
97. Doubrovina ES, Doubrovin MM, Vider E, Sisson RB, O’Reilly RJ, Dupont B, et al. Evasion From NK Cell Immunity by MHC Class I Chain-Related Molecules Expressing Colon Adenocarcinoma. J Immunol (2003) 171:6891–9. doi: 10.4049/jimmunol.171.12.6891
98. Romagné F, André P, Spee P, Zahn S, Anfossi N, Gauthier L, et al. Preclinical Characterization of 1-7F9, a Novel Human Anti-KIR Receptor Therapeutic Antibody That Augments Natural Killer-Mediated Killing of Tumor Cells. Blood (2009) 114:2667–77. doi: 10.1182/blood-2009-02-206532
99. Segal NH, Infante JR, Sanborn RE, Gibney GT, Lawrence DP, Rizvi N, et al. 1086p - Safety of the Natural Killer (NK) Cell-Targeted Anti-KIR Antibody, Lirilumab (Liri), in Combination With Nivolumab (Nivo) or Ipilimumab (Ipi) in Two Phase 1 Studies in Advanced Refractory Solid Tumors. Ann Oncol (2016) 27:vi372. doi: 10.1093/annonc/mdw378.40
100. Vey N, Karlin L, Sadot-Lebouvier S, Broussais F, Berton-Rigaud D, Rey J, et al. A Phase 1 Study of Lirilumab (Antibody Against Killer Immunoglobulinlike Receptor Antibody KIR2D; IPH2102) in Patients With Solid Tumors and Hematologic Malignancies. Oncotarget (2018) 9:17675–88. doi: 10.18632/oncotarget.24832
101. Yalniz FF, Daver N, Rezvani K, Kornblau S, Ohanian M, Borthakur G, et al. A Pilot Trial of Lirilumab With or Without Azacitidine for Patients With Myelodysplastic Syndrome. Clin Lymph Myeloma Leuk (2018) 18:658–663.e2. doi: 10.1016/j.clml.2018.06.011
102. Segal NH, Naidoo J, Curigliano G, Patel S, Sahebjam S, Papadopoulos KP, et al. First-In-Human Dose Escalation of Monalizumab Plus Durvalumab, With Expansion in Patients With Metastatic Microsatellite-Stable Colorectal Cancer. J Clin Oncol (2018) 36:3540. doi: 10.1200/JCO.2018.36.15_suppl.3540
103. André P, Denis C, Soulas C, Bourbon-Caillet C, Lopez J, Arnoux T, et al. Anti-NKG2A mAb Is a Checkpoint Inhibitor That Promotes Anti-Tumor Immunity by Unleashing Both T and NK Cells. Cell (2018) 175(7):1731–43. doi: 10.1016/j.cell.2018.10.014
104. Figueiredo C, Seltsam A, Blasczyk R. Permanent Silencing of NKG2A Expression for Cell-Based Therapeutics. J Mol Med (2009) 87:199–210. doi: 10.1007/s00109-008-0417-0
105. Carlsten M, Childs RW. Genetic Manipulation of NK Cells for Cancer Immunotherapy: Techniques and Clinical Implications. Front Immunol (2015) 6:266. doi: 10.3389/fimmu.2015.00266
106. Imai C, Iwamoto S, Campana D. Genetic Modification of Primary Natural Killer Cells Overcomes Inhibitory Signals and Induces Specific Killing of Leukemic Cells. Blood (2005) 106:376–83. doi: 10.1182/blood-2004-12-4797
107. Quintarelli C, Sivori S, Caruso S, Carlomagno S, Falco M, Boffa I, et al. Efficacy of Third-Party Chimeric Antigen Receptor Modified Peripheral Blood Natural Killer Cells for Adoptive Cell Therapy of B-Cell Precursor Acute Lymphoblastic Leukemia. Leukemia (2020) 34:1102–15. doi: 10.1038/s41375-019-0613-7
108. Capuano C, Pighi C, Battella S, De Federicis D, Galandrini R, Palmieri G. Harnessing CD16-Mediated NK Cell Functions to Enhance Therapeutic Efficacy of Tumor-Targeting Mabs. Cancers (Basel) (2021) 13(10):2500. doi: 10.3390/cancers13102500
109. Deguine J, Breart B, Lemaître F, Bousso P. Cutting Edge: Tumor-Targeting Antibodies Enhance NKG2D-Mediated NK Cell Cytotoxicity by Stabilizing NK Cell–Tumor Cell Interactions. J Immunol (2012) 189:5493 LP – 5497. doi: 10.4049/jimmunol.1202065
110. Gauthier L, Morel A, Anceriz N, Rossi B, Blanchard-Alvarez A, Grondin G, et al. Multifunctional Natural Killer Cell Engagers Targeting NKp46 Trigger Protective Tumor Immunity. Cell (2019) 177:1701–1713.e16. doi: 10.1016/j.cell.2019.04.041
111. Lo Nigro C, Macagno M, Sangiolo D, Bertolaccini L, Aglietta M, Merlano MC. NK-Mediated Antibody-Dependent Cell-Mediated Cytotoxicity in Solid Tumors: Biological Evidence and Clinical Perspectives. Ann Transl Med (2019) 7:105. doi: 10.21037/atm.2019.01.42
112. Demaria O, Gauthier L, Debroas G, Vivier E. Natural Killer Cell Engagers in Cancer Immunotherapy: Next Generation of Immuno-Oncology Treatments. Eur J Immunol (2021) 51:1934–42. doi: 10.1002/eji.202048953
113. Felices M, Lenvik TR, Davis ZB, Miller JS, Vallera DA. “Generation of BiKEs and TriKEs to Improve NK Cell-Mediated Targeting of Tumor Cells,”. Methods Mol Biol (2016) (1441):333–46. doi: 10.1007/978-1-4939-3684-7_28
114. Vallera DA, Zhang B, Gleason MK, Oh S, Weiner LM, Kaufman DS, et al. Heterodimeric Bispecific Single-Chain Variable-Fragment Antibodies Against EpCAM and CD16 Induce Effective Antibody-Dependent Cellular Cytotoxicity Against Human Carcinoma Cells. Cancer Biother Radiopharm (2013) 28:274–82. doi: 10.1089/cbr.2012.1329
115. Schmohl JU, Gleason MK, Dougherty PR, Miller JS, Vallera DA. Heterodimeric Bispecific Single Chain Variable Fragments (scFv) Killer Engagers (BiKEs) Enhance NK-Cell Activity Against CD133+ Colorectal Cancer Cells. Target Oncol (2016) 11(3):353–61. doi: 10.1007/s11523-015-0391-8
116. Rothe A, Sasse S, Topp MS, Eichenauer DA, Hummel H, Reiners KS, et al. A Phase 1 Study of the Bispecific Anti-CD30/CD16A Antibody Construct AFM13 in Patients With Relapsed or Refractory Hodgkin Lymphoma. Blood (2015) 125(26):4024–31. doi: 10.1182/blood-2014-12-614636
117. Reusch U, Burkhardt C, Fucek I, Le Gall F, Le Gall M, Hoffmann K, et al. A Novel Tetravalent Bispecific TandAb (CD30/CD16A) Efficiently Recruits NK Cells for the Lysis of CD30+ Tumor Cells. MAbs (2014) 6(3):727–38. doi: 10.4161/mabs.28591
118. Ellwanger K, Reusch U, Fucek I, Wingert S, Ross T, Müller T, et al. Redirected Optimized Cell Killing (ROCK®): A Highly Versatile Multispecific Fit-for-Purpose Antibody Platform for Engaging Innate Immunity. MAbs (2019) 11:899–918. doi: 10.1080/19420862.2019.1616506
119. Vallera DA, Felices M, McElmurry R, McCullar V, Zhou X, Schmohl JU, et al. IL15 Trispecific Killer Engagers (TriKE) Make Natural Killer Cells Specific to CD33+ Targets While Also Inducing Persistence, In Vivo Expansion, and Enhanced Function. Clin Cancer Res an Off J Am Assoc Cancer Res (2016) 22:3440–50. doi: 10.1158/1078-0432.CCR-15-2710
120. Schmohl JU, Felices M, Oh F, Lenvik AJ, Lebeau AM, Panyam J, et al. Engineering of Anti-CD133 Trispecific Molecule Capable of Inducing NK Expansion and Driving Antibody-Dependent Cell-Mediated Cytotoxicity. Cancer Res Treat (2017) 49:1140–52. doi: 10.4143/crt.2016.491
121. Vallera DA, Ferrone S, Kodal B, Hinderlie P, Bendzick L, Ettestad B, et al. NK-Cell-Mediated Targeting of Various Solid Tumors Using a B7-H3 Tri-Specific Killer Engager in vitro and in vivo. Cancers (Basel) (2020) 12(9):2659. doi: 10.3390/cancers12092659
122. Romee R, Foley B, Lenvik T, Wang Y, Zhang B, Ankarlo D, et al. NK Cell CD16 Surface Expression and Function is Regulated by a Disintegrin and Metalloprotease-17 (ADAM17). Blood (2013) 121:3599–608. doi: 10.1182/blood-2012-04-425397
123. Chan WK, Kang S, Youssef Y, Glankler EN, Barrett ER, Carter AM, et al. A CS1-NKG2D Bispecific Antibody Collectivel Activates Cytolytic Immune Cells Against Multiple Myeloma. Cancer Immunol Res (2018) 6(7):776–87. doi: 10.1158/2326-6066.CIR-17-0649
124. Raynaud A, Desrumeaux K, Vidard L, Termine E, Baty D, Chames P, et al. Anti-NKG2D Single Domain-Based Antibodies for the Modulation of Anti-Tumor Immune Response. Oncoimmunology (2021) 10(1). doi: 10.1080/2162402X.2020.1854529
125. Gill S, Vasey AE, De Souza A, Baker J, Smith AT, Kohrt HE, et al. Rapid Development of Exhaustion and Down-Regulation of Eomesodermin Limit the Antitumor Activity of Adoptively Transferred Murine Natural Killer Cells. Blood (2012) 119:5758–68. doi: 10.1182/blood-2012-03-415364
126. Marvel D, Gabrilovich DI. Myeloid-Derived Suppressor Cells in the Tumor Microenvironment: Expect the Unexpected. J Clin Invest (2015) 125:3356–64. doi: 10.1172/JCI80005
127. Melaiu O, Lucarini V, Cifaldi L, Fruci D. Influence of the Tumor Microenvironment on NK Cell Function in Solid Tumors. Front Immunol (2020) 10:3038:3038. doi: 10.3389/fimmu.2019.03038
128. Otegbeye F, Ojo E, Moreton S, Mackowski N, Lee DA, de Lima M, et al. Inhibiting TGF-Beta Signaling Preserves the Function of Highly Activated, In Vitro Expanded Natural Killer Cells in AML and Colon Cancer Models. PloS One (2018) 13:1–13. doi: 10.1371/journal.pone.0191358
129. Burga RA, Yvon E, Chorvinsky E, Fernandes R, Cruz CRY, Bollard CM. Engineering the Tgfβ Receptor to Enhance the Therapeutic Potential of Natural Killer Cells as an Immunotherapy for Neuroblastoma. Clin Cancer Res (2019) 25:4400–12. doi: 10.1158/1078-0432.CCR-18-3183
130. O’Sullivan D, Sanin DE, Pearce EJ, Pearce EL. Metabolic Interventions in the Immune Response to Cancer. Nat Rev Immunol (2019) 19:324–35. doi: 10.1038/s41577-019-0140-9
131. Cong J, Wang X, Zheng X, Wang D, Fu B, Sun R, et al. Dysfunction of Natural Killer Cells by FBP1-Induced Inhibition of Glycolysis During Lung Cancer Progression. Cell Metab (2018) 28:243–255.e5. doi: 10.1016/j.cmet.2018.06.021
Keywords: natural Killer (NK) cell, immunotherapy, in vitro expansion, tumor infiltrating cell, NK cell exhaustion
Citation: Tarannum M, Romee R and Shapiro RM (2022) Innovative Strategies to Improve the Clinical Application of NK Cell-Based Immunotherapy. Front. Immunol. 13:859177. doi: 10.3389/fimmu.2022.859177
Received: 21 January 2022; Accepted: 28 February 2022;
Published: 25 March 2022.
Edited by:
Evelyn Ulrich, Goethe University Frankfurt, GermanyReviewed by:
Daniela Pende, San Martino Hospital (IRCCS), ItalyCopyright © 2022 Tarannum, Romee and Shapiro. This is an open-access article distributed under the terms of the Creative Commons Attribution License (CC BY). The use, distribution or reproduction in other forums is permitted, provided the original author(s) and the copyright owner(s) are credited and that the original publication in this journal is cited, in accordance with accepted academic practice. No use, distribution or reproduction is permitted which does not comply with these terms.
*Correspondence: Roman M. Shapiro, Um9tYW5fU2hhcGlyb0BkZmNpLmhhcnZhcmQuZWR1
Disclaimer: All claims expressed in this article are solely those of the authors and do not necessarily represent those of their affiliated organizations, or those of the publisher, the editors and the reviewers. Any product that may be evaluated in this article or claim that may be made by its manufacturer is not guaranteed or endorsed by the publisher.
Research integrity at Frontiers
Learn more about the work of our research integrity team to safeguard the quality of each article we publish.