- 1Department of Pancreatic Surgery, Fudan University Shanghai Cancer Center, Shanghai, China
- 2Department of Oncology, Shanghai Medical College, Fudan University, Shanghai, China
- 3Shanghai Pancreatic Cancer Institute, Shanghai, China
- 4Pancreatic Cancer Institute, Fudan University, Shanghai, China
- 5Institute of Clinical Medicine Research, Zhejiang Provincial People's Hospital, Hangzhou Medical College, Hangzhou, China
- 6Cancer Institute of Integrated Traditional Chinese and Western Medicine, Zhejiang Academy of Traditional Chinese Medicine, Tongde Hospital of Zhejiang Province, Hangzhou, China
Increasingly, patients with gastrointestinal tumors can benefit from immunotherapy, but not patients with pancreatic cancer. While this lack of benefit has been attributed to lower T-cell infiltration in pancreatic cancer, other studies have demonstrated the presence of numerous T cells in pancreatic cancer, suggesting another mechanism for the poor efficacy of immunotherapy. Single-cell RNA sequencing studies on the pancreatic cancer immune microenvironment have demonstrated the predominance of innate immune cells (e.g., macrophages, dendritic cells, mast cells, and innate immune lymphoid cells). Therefore, in-depth research on the source and function of innate immune lymphocytes in pancreatic cancer could guide pancreatic cancer immunotherapy.
1. Introduction
The pancreas is located in the retroperitoneum and connects to the digestive tract only via the pancreatic duct, without direct contact with the external environment. Therefore, the pancreas is relatively “clean” compared with the other digestive organs. Therefore, normal pancreatic tissue contains very few lymphocytes, which has been confirmed by both our research (1) and that of other researchers (2). However, pancreatic cancer initiation and development are followed by substantial immune cell infiltration (Figure 1). Fractionation of the immune cells in pancreatic adenocarcinoma (PDAC) revealed a greater abundance of innate immune cells than adaptive immune cells (2). Innate immune cells are the primary responders to inflammation; therefore, a large proportion of macrophages, myeloid-derived suppressor cells (MDSCs), and dendritic cells (DCs) are observed in pancreatic cancer. The tumor microenvironment includes excessive fibrosis, which further hampers the infiltration of adaptive immune cells.
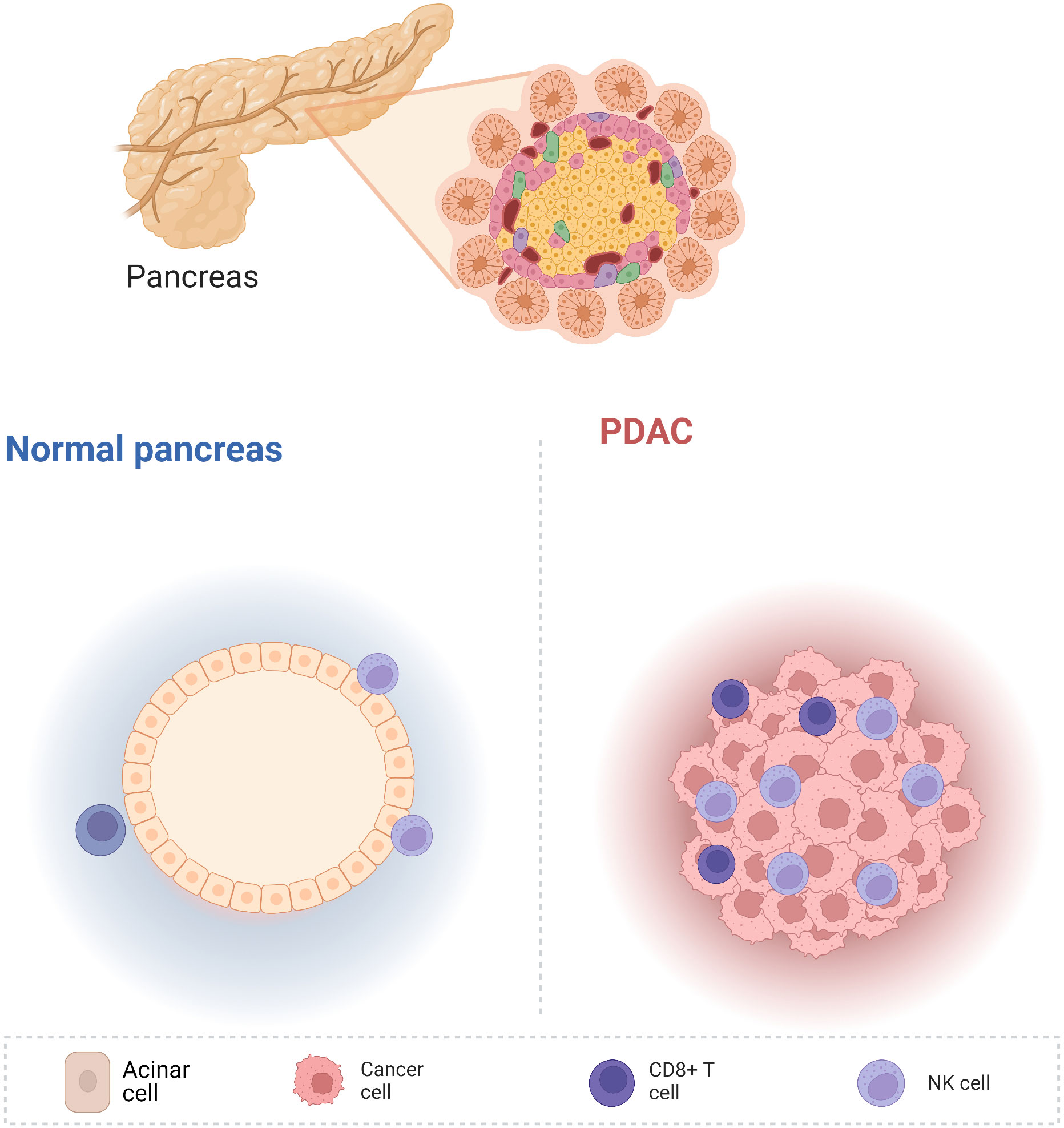
Figure 1 Compared with other digestive tract organs, the non-cancerous pancreas is relatively clean and contains very few immune cells. Nevertheless, pancreatic cancer development is accompanied by the infiltration of numerous immune cells, including innate and adaptive immune cells.
Current immunotherapy, including immune checkpoint blockade, chimeric antigen receptor-T, and T-cell receptor-T, has shown a low response rate in PDAC so far. The non-responsiveness to immunotherapy in pancreatic cancers can be explained by these tumors being less immunogenic (harboring fewer somatic mutations) compared with breast and lung tumors, which tend to have better immunotherapy responsive rates.
Furthermore, compared with adaptive immunity, innate immune cells do not require antigen presentation, and can be rapidly and directly activated by a large number of cytokines. Therefore, in light of the predominance infiltration, decreased antigenicity and instant activation, innate immunity might play a more important role than adaptive immunity in the PDAC immune microenvironment.
In this review, we categorize the innate immune lymphocytes in pancreatic cancer and describe their origin and function. We believe that in-depth study of the innate immune function and characterizing the pancreatic cancer immune microenvironment is crucial to improve the efficacy of pancreatic cancer immunotherapy.
2. Macrophages
Single-cell RNA sequencing (RNA-seq) data from Magliano’s group (2) demonstrated that macrophages are the dominant immune cells among the CD45+ population in PDAC. Previously, it was believed that peripheral tissue macrophages were derived from circulating monocytes, which originate from bone marrow progenitors. Recently, accumulating studies have demonstrated two independent origins for macrophages (3–7). The first comprises early erythroid-myeloid precursor cells (EMPs) of the yolk sac (embryonic day [E]7) or the late EMPs of the liver (E8–E9.5). The EMPs migrate into the peripheral tissues during the embryonic period, differentiate, and mature, and have self-renewal and maintenance functions in adults. The other macrophage origin is hematopoietic stem cells (HSCs) from the bone marrow that enter the peripheral tissues through the blood circulation, and then mature and differentiate into monocytes. The proportions of these two macrophage origins differ quite significantly between different organs (Figure 2).
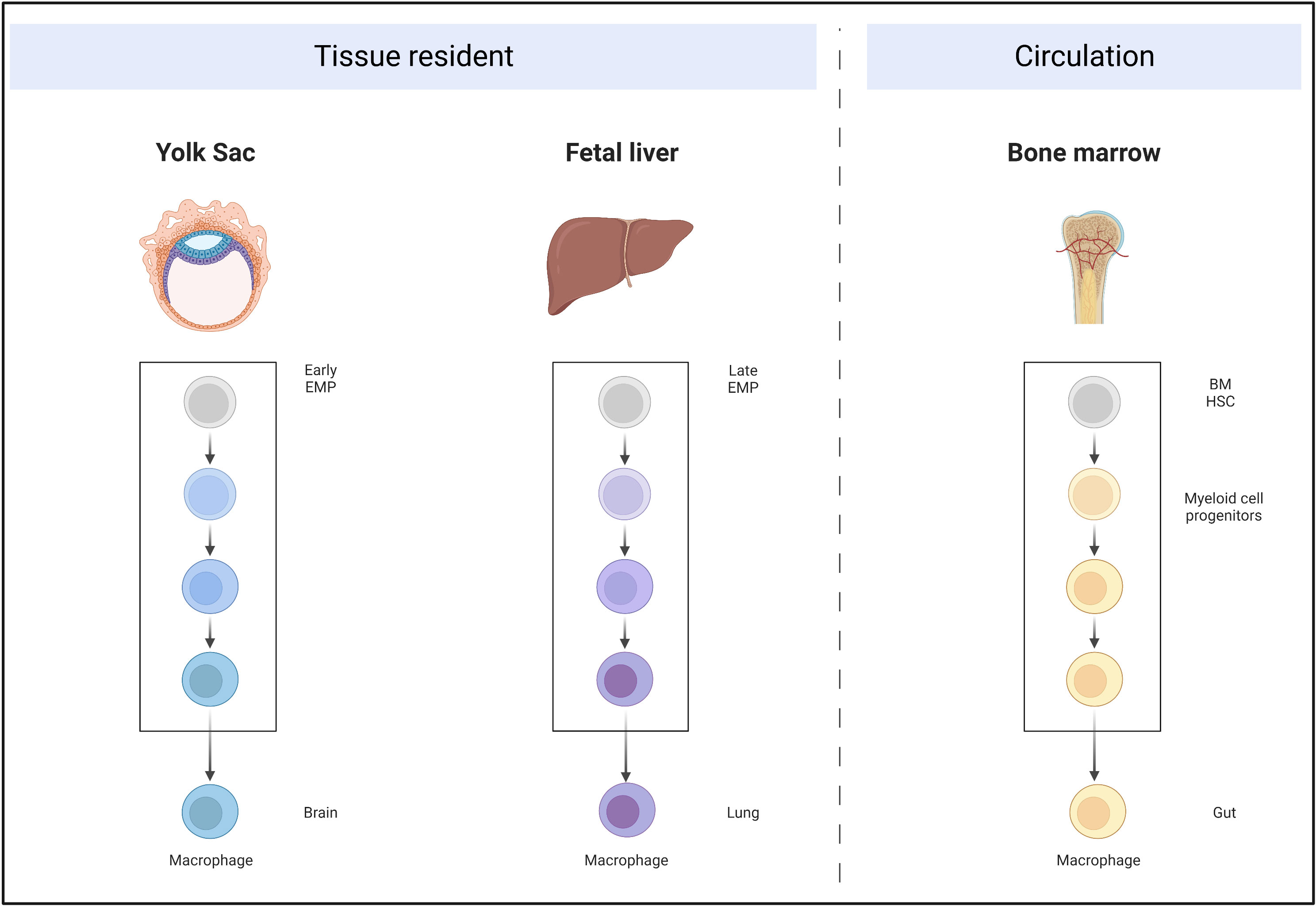
Figure 2 Self-proliferative tissue-resident macrophages originate from the yolk sac and fetal liver, migrate into the peripheral organs during embryonic development, and mature. Circulating macrophages are derived from bone marrow HSCs, which develop into myeloid-committed stem cells and further develop into macrophages.
In brain tissue, microglia are derived from the early EMPs of the yolk sac and are not replenished by circulating monocytes (3). By contrast, most adult intestinal macrophages are constantly replenished by peripheral monocytes, for which Bain et al. (7) demonstrated that the F4/80hiCD11blo subset (derived from embryonic precursors) was dominant in newborn mice, but was progressively lost in adult mice, in which almost all macrophages were F4/80lowCD11bhi (derived from the circulation).
Similarly, in the pancreas, macrophages have an obvious dual origin. Using lineage tracing mice (Flt3-CreYFP; Flt3, Fms related receptor tyrosine kinase 3; YFP, yellow fluorescent protein) in which HSC-derived cells were YFP+ and embryo-derived cells were YFP-, Zhu et al. demonstrated that approximately 32% of macrophages in the pancreas were YFP-. Furthermore, they established orthotopic KPC (LSL-KrasG12D/+LSL-Trp53R172H/+Pdx1-Cre) tumor mice. Similar to the results in the non-tumorous pancreas, almost one-third of macrophages were YFP-, indicating that they were tissue-resident (8).
The cells from these two origins have entirely different development environments, which means that they might have different functions. Using Ccr2 (encoding C-C motif chemokine receptor 2) and Nur77 (encoding nuclear hormone receptor NUR/77) knockout mice, Zhu et al. demonstrated that bone marrow-derived macrophages were more prone to expressing human leucocyte antigen DR (HLA-DR), and its absence did not impair PDAC development (8). By contrast, embryonically derived tumor-associated macrophages (TAMs) exhibited unique pro-fibrotic activity and promoted cancer progression. Macrophages of these different origins appear to be distinguished by C-X-C motif chemokine receptor 4 (CXCR4) in human pancreatic cancer, where CXCR4-positive macrophages appear to be tissue-resident and have a pro-fibrotic effect. Bockorny et al. used the CXCR4 inhibitor, BL-8040, in combination with pembrolizumab and chemotherapy, which was initially successful; however, the specific mechanism is unknown (9). Whether CXCR4 is merely a downstream marker or functions as a driving gene requires further exploration.
Traditionally, macrophage function is divided into the M1 and M2 types; however, this division might have some limitations. Single-cell RNA-seq data suggested that macrophage function appears to present as a continuous, rather than a separate state (10–12) and is highly susceptible to the influence of the microenvironment.
Macrophages are generally inclined to the M2 phenotype in pancreatic cancer. Their infiltration density was associated with disease progression, recurrence, and metastasis in patients with PDAC (13). TAM-derived interleukin (IL)6, tumor necrosis factor alpha (TNFα), and regulated upon activation, normally t-expressed, and presumably secreted (RANTES) could facilitate acinar-to-ductal metaplasia and pancreatic intraepithelial neoplasia development via Janus kinase (JAK)-signal transducer and activator of transcription3 (STAT3) (14) or nuclear factor kappa B (NF-κB) (15) signaling. Depletion of TAMs could dramatically decrease tumorigenesis (16). In addition, TAMs could also express a series of immunosuppressive factors (e.g., IL-10, transforming growth factor beta (TGF-β) and C-C motif chemokine ligand (CCL)2) and negative immune checkpoints (e.g., cytotoxic T-lymphocyte associated protein 4 (CTLA-4) and programmed cell death 1 ligand 1 (PD-L1)) to promote immune escape in PDAC (17). With regard to fibrosis, macrophages could secrete growth factors (e.g., TGFβ1), cytokines (e.g., TNFα) and chemokines (e.g., CCL2), which directly activate fibroblasts to form fibrous desmoplastic tissue (18). This interstitial fibrosis leads to extreme hypoxia, which in turn induces polarization toward the M2 phenotype, promotes the expression of vascular endothelial growth factor (VEGF) and tunica interna endothelial cell kinase (TIE2), and stimulates PDAC angiogenesis (19, 20). On the other hand, TAMs will also secrete chemokines including CCL20 and CCL18, induce the upregulation of matrix metalloproteinase 9 (MMP9) and vascular cell adhesion molecule 1 (VCAM-1) expression in pancreatic cancer cells, and enhance metastasis (21, 22). Nevertheless, TAMs can activate cancer-associated fibroblasts to promote fibrosis and increase the difficulty of drug delivery (23). Xian et al. also reported that TAMs directly induced resistance to gemcitabine in PDAC cells (24). Furthermore, D’Erico et al. suggested that TAMs can induce regulatory T cell infiltration, thereby inhibiting the efficacy of chemotherapy (25). Therefore, TAMs are one of the most important regulators in the tumor microenvironment, including tumorigenesis, immune escape, interstitial fibrosis, angiogenesis, and chemoresistance.
3. Dendritic cells
Dendritic cells (DCs) were previously classified as belonging to the mononuclear phagocyte system, until Steinman et al. distinguished them from macrophages (26). DCs are generally divided into three categories: classical (cDC1 and cDC2), plasmacytoid cell (pDCs), and monocyte or inflammatory DCs. Functionally, cDCs are more prone to present antigens, pDCs are key in type I interferon (IFN) secretion during viral infection, and the monocyte-derived or inflammatory DCs are common in areas of inflammation (27).
DCs demonstrate considerable functional heterogeneity, even in the same cell population. Villani et al. divided DCs into six groups based on single-cell RNA-seq data (28). Nevertheless, they also pointed out that single-cell sequencing alone was insufficient to support the new DC definition and classification, and it was difficult to distinguish whether DCs were a class of cells in different functional states under the same cell group or a bona fide new cell population based on differences in gene expression, which requires verification via in vivo and in vitro experiments.
It is believed that the cDCs are derived from common DC progenitors (CDP) and monocyte or inflammatory DCs originate from monocyte–macrophage DC progenitors, while the origin of pDCs remain a matter of debate. Previously, pDCs were thought to be of the myeloid lineage, whereas recent single-cell studies suggested that they mainly originate from the lymphoid lineage. It was believed that cell differentiation and development is a step-wise directed differentiation process, i.e., from HSC to common myeloid progenitors (CMP), granulocyte-macrophage progenitors (GMP), and monocyte-dendritic progenitor (MDP) to CDP, followed by migration to the peripheral organs to differentiate and mature into cDCs. However, recent research has challenged this view. Helft et al. reported that precursor cells with lymphoid commitment such as multi-lymphoid progenitors can also differentiate into DCs (29). Others have also suggested that the differentiation tendency of precursor cells with the same surface marker definition vary considerably. Therefore, unlike the previous directional development model of this tree-like structure (Figure 3), the development process in the human body might present multidirectional differentiation, including cells such as monocyte-dendritic progenitors and common DC progenitors, which maintain the directional differentiation of DCs while being able to differentiate into other cells when responding to external stimuli.
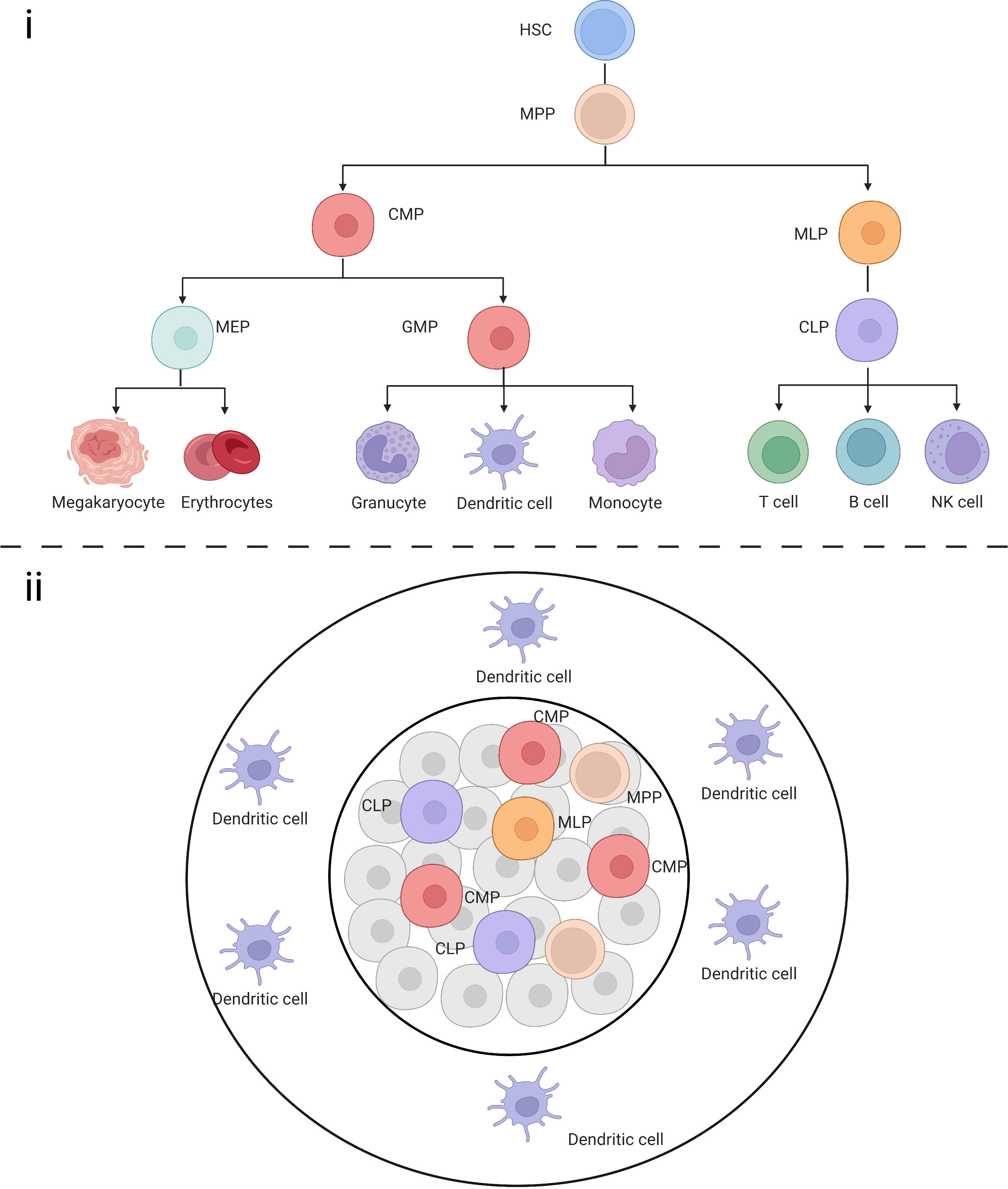
Figure 3 (i) Schematic representation of classical HSCs in a tree-like model. (ii) Multiple progenitor cells maintain the ability to differentiate into DCs.
Compared with lung cancer, PDAC contains significantly fewer DCs (especially cDC1), which are mainly located on the tumor periphery (30). The maturation and antigen presentation-ability of cDC1 are relatively weak. This deficiency in cDC1 abundance and maturation are unique to pancreatic tumors, occurring from the early stage of pancreatic intraepithelial neoplasia and declining systematically and progressively as the disease progresses, which is caused by IL-6-mediated apoptosis (31). In PDAC, cDC1 and pDC infiltration in the tumor and tumor stroma was associated with better prognosis (32). High DC infiltration polarizes CD4+ and CD8+ tumor-infiltrating lymphocytes, while relatively poor DC abundance and antigen-presenting function impair the T cell response, leading to tumor immune escape. Therefore, DCs are indispensable as a key link in activating adaptive immunity. In a genetically engineered PDAC tumor model constructed by Hegde et al., featuring the poor infiltration and activation of DCs in the tumor, the pathological adaptive immune responses initiated by the expression of the engineered model neoantigen even accelerated the occurrence of PDAC through pro-fibrotic inflammation. By mobilizing cDCs into pancreatic lesions and maintaining their activation and function, this pro-fibrotic inflammation was reversed and PDAC progression was controlled. Based on this premise, a variety of DC-based treatment methods have emerged, including DC vaccines (33) and CD40 agonists that promote DC maturity (34). CD40 agonists effectively promote cDC1 maturation, while the Flt3 ligand (Flt3L) helps to increase cDC1 abundance, and the synergistic effect of the two promotes superior T cell activation and enhances anti-tumor immunity (31). Some trials of DC vaccines have also shown clinical effectiveness. For example, in a Phase I clinical trial of patients with PDAC, 10 patients were injected with autologous tumor lysate-loaded autologous monocyte-derived DCs. During a median follow-up period of 25 months, 7 patients have not experienced tumor recurrence or progression and no vaccine-related serious adverse reactions occurred, indicating good feasibility, safety, and clinical efficacy (33). The combination of CD40 agonists and DC vaccines also demonstrated clinical effectiveness by combining the advantages of both (34).
4. Mast cells
In mice, mast cells are classified as connective tissue mast cells (CTMCs) and mucosal mast cells (MMC). Li et al. used Runx1cre/EYFP and Csf1rcre/EYFP reporter mice to confirm that CTMCs in adipose tissue and the pleural cavity were mainly derived from early EMPs, while CTMCs in other organs were derived mostly from late EMPs. Therefore, mouse CTMCs are considered tissue-resident while mouse MMC are derived mainly from the bone marrow (35).
There are three mast cell populations in human: tryptase-positive mast cells (MCTs), chymase-positive mast cells (MCCs), and tryptase/chymase-positive mast cells (MCTCs) (Figure 4). Mast cells are important in infection (36), chronic inflammation (37), and metabolic diseases (38).
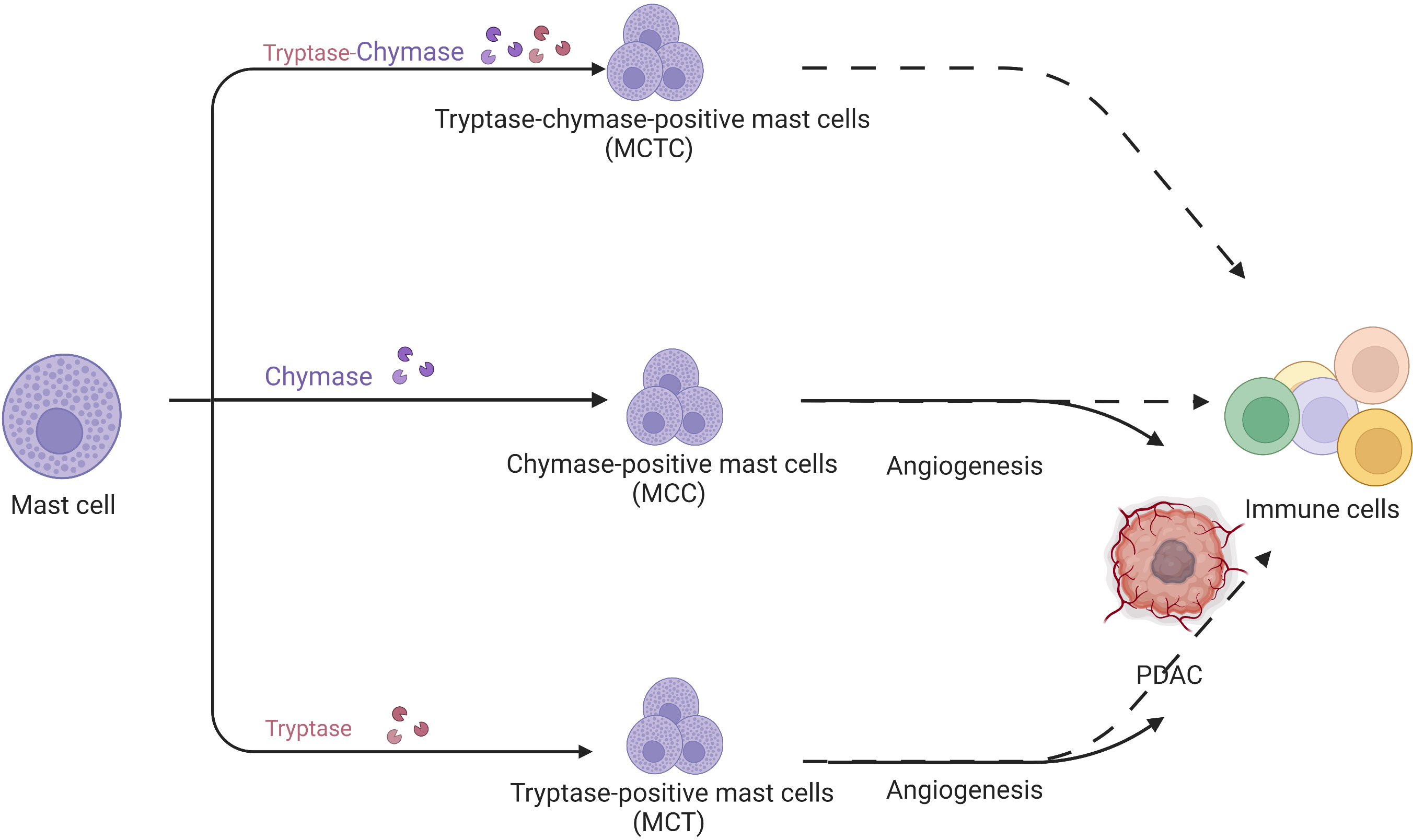
Figure 4 Mast cells are divided into tryptase/chymase-positive mast cells, MCCs, and MCTs according to their contents. MCCs and MCTs promote tumor development through their pro-angiogenic effects but their specific role in pancreatic cancer is unknown.
In human PDAC, Strouch et al. (39) firstly determined that MCTs were significantly increased in tumors and associated with poor prognosis. Guo (40) et al. and Ammendola et al. (41) demonstrated that MCTs were strongly associated with angiogenesis in PDAC. Ammendola et al. also confirmed that MCCs were positively associated with angiogenesis in pancreatic cancer (42). However, the above studies were based on the immunochemistry of PDAC specimens. Given the limitations of the method, immunochemistry cannot accurately assess the mast cell fraction and function in the PDAC microenvironment. Furthermore, most studies focused on tryptase-induced angiogenesis without the connection of mast cells and other immune cells in the tumor microenvironment. Some researchers believed that mast cell function cannot be reflected accurately by dividing mast cells according to their proteases. Therefore, referring to macrophage categorization, mast cells are divided into anti-tumor MC1 and pro-tumor MC2 based on TNF, granzyme B (GZMB), IL9, VEGF-A, and C-X-C motif chemokine ligand 8 (CXCL8) expression. Consequently, mast cell function remains ambiguous in PDAC.
Similar to macrophages, mast cells undergo prenatal development in two distinct waves: the first is from the yolk sac and fetal liver and the second is from the aorta-gonad-mesonephros region (43). Salomonsson et al. reported a group of Lin−CD34hiCD117int/hiFcϵRI+ cells in the peripheral blood in humans (44) that could further differentiate into mast cells in in vitro culture and were therefore considered mast cell precursors. However, considering the high heterogeneity of mast cells in peripheral tissue, some researchers proposed a different lineage model. Similar to DCs, a pool of non-committed stem cells might give rise to different types of mast cells (43).
With the further development of new technical methods, including single-cell sequencing and spatial transcriptomes, the identification and functional conversion of mast cells at different times and locations in pancreatic cancer occurrence and development will be further explored.
5. Myeloid-derived suppressor cells
Myeloid-derived suppressor cells (MDSCs) comprise a heterogeneous population of immature bone marrow cells that originate from bone marrow HSCs, differentiate into CMP and immature myeloid cells (IMP), and then migrate to peripheral organs and develop into GMP, which in turn form granulocytes, macrophages, or DCs (45). However, under pathological conditions, especially in tumors, a large number of cytokines are produced, including granulocyte-macrophage colony-stimulating factor (GM-CSF), granulocyte colony-stimulating factor (G-CSF), and macrophage colony-stimulating factor (M-CSF), which promote the formation of MDSCs through mitogen-activated protein kinase (MAPK) or phosphatidylinositol-4,5-bisphosphate 3-kinase (PI3K) pathways (46–48).
Based on their phenotypic and cell surface markers, MDSCs can be classified into two main types: polymorphonuclear MDSCs (or granulocytic (G-MDSCs)) and mononuclear or monocytic MDSCs. Human G-MDSCs are labeled as HLA-DR-CD33+CD11b+CD15+CD14-, and mononuclear or monocytic MDSCs are labeled as HLA-DRlowCD11b+CD14+CD15- (49, 50). MDSCs share surface markers with normal myeloid cell subsets, such as CD14, CD15, and CD33, and exhibit intrinsic heterogeneity; therefore, it is imperative to combine phenotypic characterization with functional assays for their identification.
MDSCs have a significant immunosuppressive function in the tumor immune microenvironment, which is mainly achieved by inhibiting the function of effector T lymphocytes, and this immunosuppressive effect is antigen-nonspecific. They can secret indoleamine 2,3-dioxygenase (IDO), arginase-I (Arg-1), inducible nitric oxide synthase (iNOS), reactive oxygen species (ROS), and several inhibitory cytokines (IL-10, IL-13 and TGF-β) (51) (Figure 5).
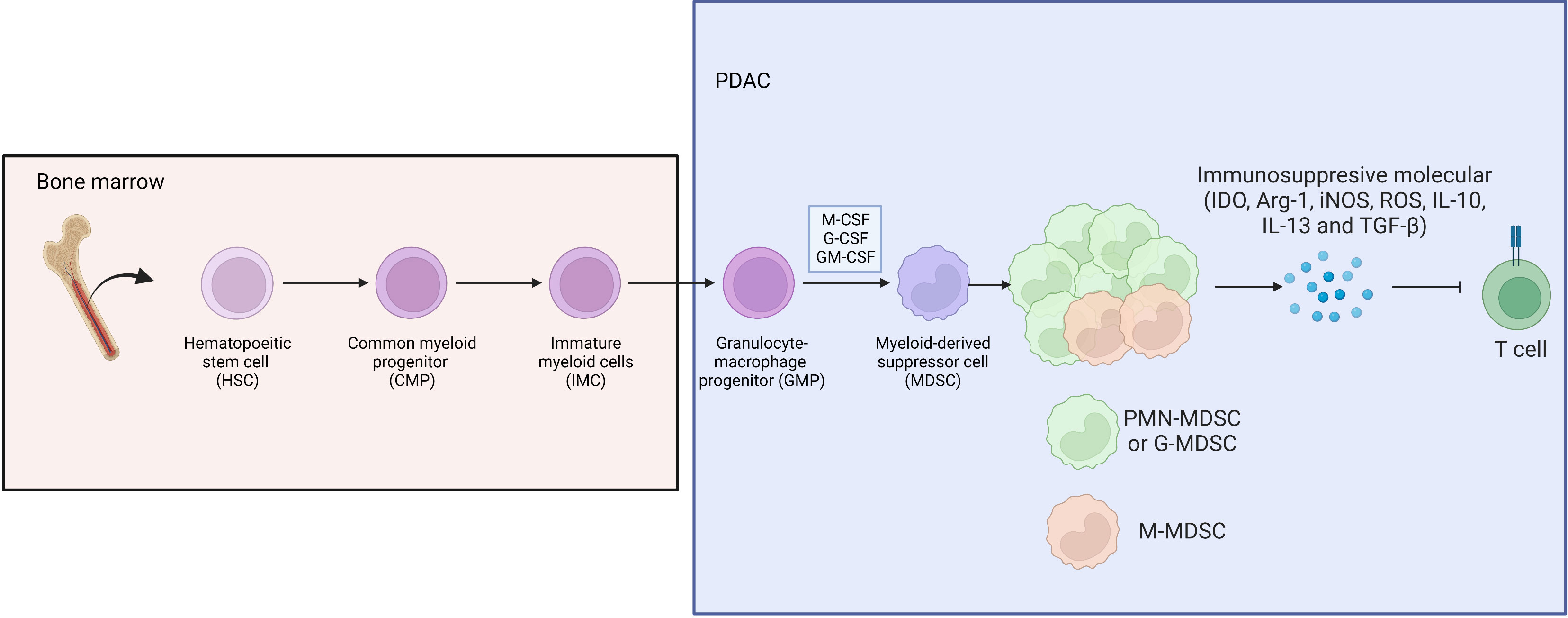
Figure 5 Bone marrow derived HSC differentiate to CMP, IMP and GMP, then develop into MDSC under stimulation of M-CSF, G-CSF and GM-CSF in PDAC, where G-MDSC occupied the majority of MDSC subpopulation.
Khaled et al. phenotyped MDSCs in pancreatic cancer and found that G-MDSCs represented the majority of the MDSC subpopulation in circulation and in tumors, not mononuclear or monocytic MDSCs. And they found that Arg was significantly highly expressed in circulating G-MDSCs, which could dramatically inhibit the proliferation and activation of T cells (52). Enrichment of G-MDSCs further led to tumor progression, accompanied by a decrease in monocyte MDSC frequency. And it is worth noting that even after tumor resection, the MDSC cells in the peripheral blood of patients are still high, which may be related to tumor micrometastasis (53). Takeuchi et al. reported that the production of GM-CSF was significantly enhanced in various PDAC cell lines or PDAC tumor tissues of patients after chemotherapy, thereby inducing monocyte differentiation into MDSCs (54). The high heterogeneity of MDSCs means that selective depletion of MDSCs with antibodies for therapeutic effect remains elusive. However, Choueiry et al. reported that CD200 expression might expand MDSCs, and targeting CD200 could enhance the activity of checkpoint immunotherapy (55). In addition, Karakhanova et al. demonstrated that sildenafil could reduce the amount of chronic inflammatory factors (IL-1β, VEGF, and GM-CSF) and suppress immunosuppressive MDSC function, thereby restoring T cell antitumor reactivity and significantly prolonging survival in treated mice. Furthermore, Porembka et al. reported that zoledronic acid reduces MDSC prevalence and tumor growth in PDAC (56). However, the immunotherapy targeting MDSCs still needs more investigation.
6. Innate lymphoid cells
Innate lymphoid cells (ILCs) can be classified into natural killer (NK) cells, ILC1s, ILC2s, and ILC3s. As classic innate immune lymphocytes, NK cells have a different origin from ILC1s, ILC2s, and ILC3s, mainly originating from the bone marrow and constantly migrating through the circulation to the peripheral tissues (57, 58). The extreme fibrotic feature of PDAC means that the proportion of NK cell infiltration in PDAC tissue is very low (< 0.5%) and NK cell function in patients with pancreatic cancer is significantly inhibited compared with that in healthy subjects (59). NK cells express natural cytotoxicity receptors (NCRs: NCR1/NKp46, NCR2/NKP44, NCR3/NKP30), killer inhibitory receptors (KIRs), and other receptors, such as NK cell receptor D (NKG2D) and DNAX accessory molecule-1 (DNAM-1). In PDAC, NK cells exhibit impaired cytotoxicity receptors and increased IL-10 expression (60). As a novel immune receptor-targeted drug in pancreatic cancer, NKG2D is receiving increasing attention (61).
The mouse pancreas rarely contains ILC1s and ILC3s, wherein ILC2s are prevalent. ILC2s exhibit pronounced heterogeneity and high plasticity. Xu et al. reported that a higher percentage of ILC2s predicted poor prognosis in patients with hepatocellular carcinoma (62). Heinrich et al. reported that ILC2s were associated with better prognosis of patients with hepatocellular carcinoma (63). The discrepancy between these two studies might be because Xu et al. focused on KLRG1- ILC2s, while Heinrich et al. concentrated on IL33 (alarmin)-activated KLRG1+ ILC2s.
Moral et al. demonstrated that IL33-activated ILC2s recruited CD103+ DCs to activate CD8+ T cells in PDAC, which predicted better survival (64). However, Alam et al. reported that the mycobiome upregulated IL33 then activated ILC2s in PDAC, which was associated with poor prognosis (65). Therefore, the function of ILC2 in pancreas is still on the debate. Our research confirmed that IL33-activated ILC2s shrank tumors in a mouse model. However, the tumor microenvironment is complicated, such that not only are molecules such as IL33 present, but so are extreme physical conditions, such as hypoxia. Furthermore, we detected no significant association between IL33 and overall survival or disease-free survival in the Cancer Genome Atlas PDAC data (1). Therefore, IL33 activation in ILC2 function in cancer might be overestimated. IL33-activated ILC2s have a well-established role in inflammation, especially in asthma (66). However, in the tumor microenvironment, the classical suppression of tumorigenicity 2 (ST2)–IL33 pathway might not be the dominant pathway. Our RNA-seq data demonstrated that hypoxia induced regulatory ILCs from an ILC2 population in PDAC, which differed from the Id3-expressing regulatory ILCs in the mouse intestines (67) and the retinoic acid-induced regulatory ILCs in patients with asthma (68) (Figure 6). Therefore, thorough investigation of the PDAC tumor microenvironment complexity to clearly demonstrate how ILC2s transition when encountering different signals is still required.
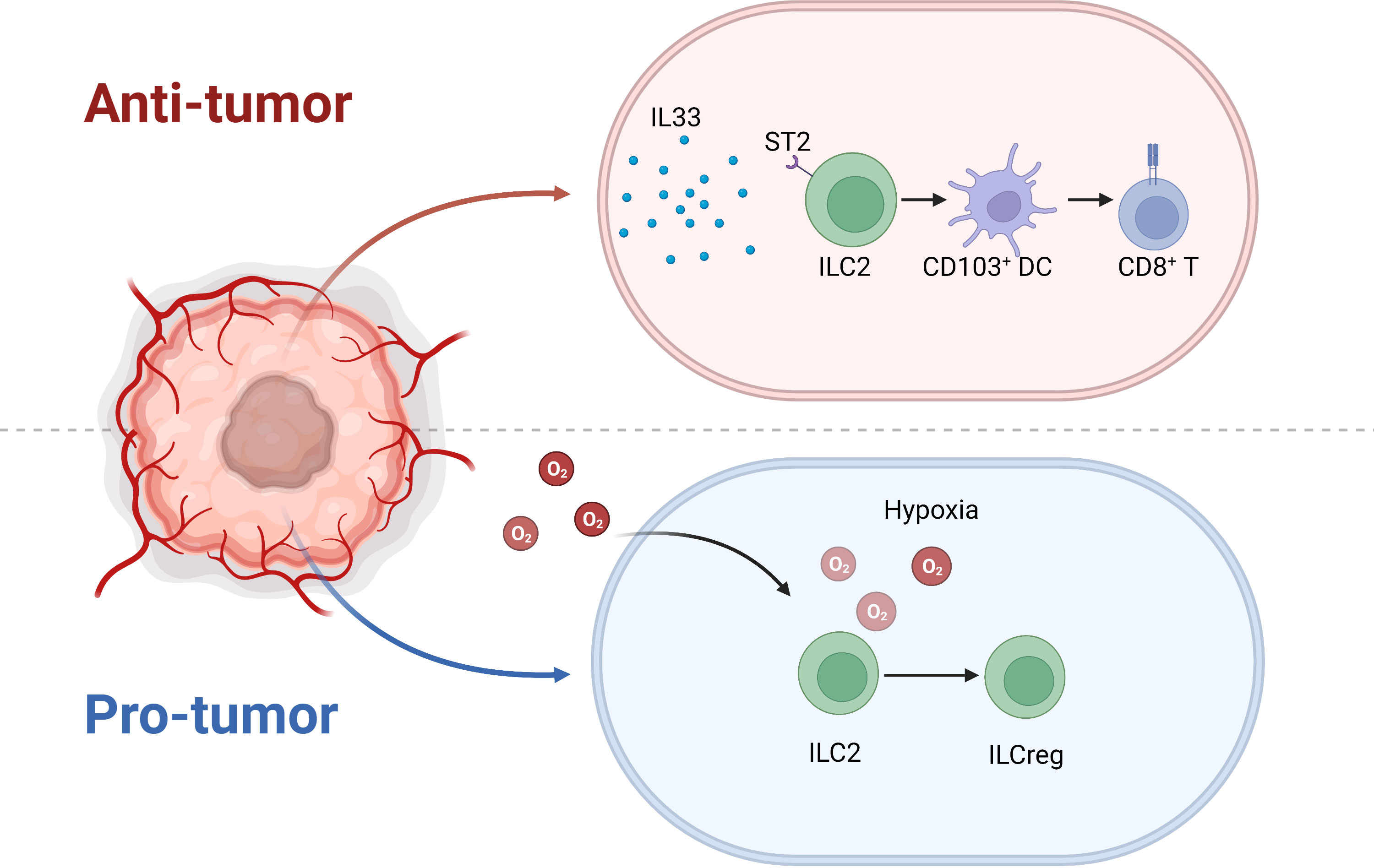
Figure 6 Under IL33 stimulation, ILC2 recruits CD103+ DCs and prime CD8+ T cells to inhibit tumor progression. Nonetheless, the extreme hypoxic environment of pancreatic cancer induces ILC2 conversion to ILCreg.
Similar to tissue-resident macrophages, the maintenance and expansion of most ILCs in peripheral tissues is independent of the bone marrow. ILC precursor cells migrate to the peripheral tissues during the embryonic and postnatal developmental window and their proportions differ greatly in different peripheral organs and tissues (69). Huang et al. used the CD45.1 and CD45.2 mouse parabiosis model to prove that there was a considerable proportion of immune cells in the circulation from each mouse type; although ILCs were hardly exchanged with each other in the peripheral organs (70). However, because of the abundant proliferation of ILCs in infection, it is speculated that a group of ILC precursor cells is present in the peripheral organs. Zeis et al. reported on a population of IL18R1+ST2- ILC2 precursor cells in the lung that could differentiate into ILC2s in vitro and in vivo (71).
However, because of its relatively “clean” property, the non-cancerous pancreas contains almost no lymphocytes. Therefore, it is difficult to detect the possible ILC2 progenitor cells in the pancreas. In PDAC, 20–30% of CD45+ cells are CD103+. Therefore, circulatory ILC2s might exist in patients with PDAC (1). Lim et al. reported the presence of CD117+ multi-potent ILC progenitors in human peripheral blood, which gave rise to all ILC subsets (72). Moreover, Huang et al. reported the existence of inflammatory ILC2s in mice that could migrate from the intestinal lamina propria to other tissues under the induction of IL25 or helminth-induced inflammation and replenish natural ILC2s in various organs (70). Therefore, the function and proportion of tissue-resident and circulating ILC2s in PDAC still requires further investigation.
7. Conclusion
Pancreatic cancer is one of the most malignant tumors and its incidence increases year-by-year as lifestyles change. Nevertheless, the 5-year survival rate remains at approximately 10% and there has been no significant improvement compared with 10 years ago (73, 74). Therefore, there is an extremely urgent need to find new treatments. Unfortunately, unlike other digestive system tumors, immune checkpoint antibodies are not successful to treat pancreatic cancer. Nonetheless, innate immunity might be a promising target.
Accumulating research evidence demonstrates that most innate immune cells in the peripheral organs are more inclined to be tissue-resident rather than classically bone marrow-derived. The unsatisfactory efficacy of current immunotherapy regiments in PDAC prompted us to investigate the roles of innate immune cells in PDAC. A reasonable therapeutic schedule should focus on not only enhancing the function of adaptive immunity cells, but also on activating innate immunity in the tumor microenvironment. The application of DC vaccines or their combination with CD40 agonists have achieved promising results in early phase clinical studies. However, targeting the innate immune system still faces huge challenges and requires more detailed exploration to bring hope for a breakthrough in pancreatic cancer immunotherapy.
Author contributions
LY and SS contributed to the conception of the study and wrote the manuscript. WC reviewed the manuscript and provided suggestions for revision. All authors contributed to the article and approved the submitted version.
Funding
This study was jointly supported by the National Natural Science Foundation of China (82173091 to LY 81972693 to WC), Shanghai Pujiang Program (2021PJD014 to LY), Research Project of Shanghai Municipal Health Commission (20214Y0396 to LY), Zhejiang Provincial Nature Science Foundation of China (LR20H160001 to WC), Key R&D projects of Zhejiang Province (2021C03012 to WC), Young Qihuang Scholar of National Administration of Traditional Chinese Medicine (to WC), Zhejiang Provincial Ten Thousand Plan for Young Top Talents (2018, to WC), Training objects of health innovative talents of Zhejiang Health (2018, to WC). The funding agencies had no role in study design, data collection, and analysis, decision to publish, or preparation of the manuscript.
Conflict of interest
The authors declare that the research was conducted in the absence of any commercial or financial relationships that could be construed as a potential conflict of interest.
Publisher’s note
All claims expressed in this article are solely those of the authors and do not necessarily represent those of their affiliated organizations, or those of the publisher, the editors and the reviewers. Any product that may be evaluated in this article, or claim that may be made by its manufacturer, is not guaranteed or endorsed by the publisher.
Abbreviations
PDAC, pancreatic adenocarcinoma; HSChematopoietic stem cell; RNA-seq, RNA sequencing; EMP, erythroid-myeloid precursor; TAM, tumor-associated macrophageDC dendritic cell; DFS, disease-free survival; OS, overall survival; CDP, common DC progenitor; CMP, common myeloid progenitor; GMP, granulocyte-macrophage progenitor; MDP, monocyte-dendritic progenitor; MLP, multi-lymphoid progenitor; CTMC, connective tissue mast cell; MMC, mucosal mast cell; MC, tryptase-positive mast cell; MCC, chymase-positive mast cell; MCTC, ryptase/chymase-positive mast cell; ILC, innate lymphoid cell; NK cell, natural killer cell; ILCreg, regulatory ILC.
References
1. Ye L, Jin K, Liao Z, Xiao Z, Xu H, Lin X, et al. Hypoxia-reprogrammed regulatory group 2 innate lymphoid cells promote immunosuppression in pancreatic cancer. EBioMedicine (2022) 79:104016. doi: 10.1016/j.ebiom.2022.104016
2. Steele NG, Carpenter ES, Kemp SB, Sirihorachai VR, The S, Delrosario L, et al. Multimodal mapping of the tumor and peripheral blood immune landscape in human pancreatic cancer. Nat Cancer (2020) 1:1097–112. doi: 10.1038/s43018-020-00121-4
3. Ginhoux F, Greter M, Leboeuf M, Nandi S, See P, Gokhan S, et al. Fate mapping analysis reveals that adult microglia derive from primitive macrophages. Science (2010) 330:841–5. doi: 10.1126/science.1194637
4. Merad M, Manz MG, Karsunky H, Wagers A, Peters W, Charo I, et al. Langerhans cells renew in the skin throughout life under steady-state conditions. Nat Immunol (2002) 3:1135–41. doi: 10.1038/ni852
5. Hashimoto D, Chow A, Noizat C, Teo P, Beasley MB, Leboeuf M, et al. Tissue-resident macrophages self-maintain locally throughout adult life with minimal contribution from circulating monocytes. Immunity (2013) 38:792–804. doi: 10.1016/j.immuni.2013.04.004
6. Yona S, Kim KW, Wolf Y, Mildner A, Varol D, Breker M, et al. Fate mapping reveals origins and dynamics of monocytes and tissue macrophages under homeostasis. Immunity (2013) 38:79–91. doi: 10.1016/j.immuni.2012.12.001
7. Bain CC, Bravo-Blas A, Scott CL, Perdiguero EG, Geissmann F, Henri S, et al. Constant replenishment from circulating monocytes maintains the macrophage pool in the intestine of adult mice. Nat Immunol (2014) 15:929–37. doi: 10.1038/ni.2967
8. Zhu Y, Herndon JM, Sojka DK, Kim KW, Knolhoff BL, Zuo C, et al. Tissue-resident macrophages in pancreatic ductal adenocarcinoma originate from embryonic hematopoiesis and promote tumor progression. Immunity (2017) 47:323–338 e326. doi: 10.1016/j.immuni.2017.07.014
9. Bockorny B, Semenisty V, Macarulla T, Borazanci E, Wolpin BM, Stemmer SM, et al. BL-8040, a CXCR4 antagonist, in combination with pembrolizumab and chemotherapy for pancreatic cancer: The COMBAT trial. Nat Med (2020) 26:878–85. doi: 10.1038/s41591-020-0880-x
10. Zhou Y, Yang D, Yang Q, Lv X, Huang W, Zhou Z, et al. Single-cell RNA landscape of intratumoral heterogeneity and immunosuppressive microenvironment in advanced osteosarcoma. Nat Commun (2020) 11:6322. doi: 10.1038/s41467-020-20059-6
11. Zhang L, Li Z, Skrzypczynska KM, Fang Q, Zhang W, O'Brien SA, et al. Single-cell analyses inform mechanisms of myeloid-targeted therapies in colon cancer. Cell (2020) 181:442–459 e429. doi: 10.1016/j.cell.2020.03.048
12. Sathe A, Grimes SM, Lau BT, Chen J, Suarez C, Huang RJ, et al. Single-cell genomic characterization reveals the cellular reprogramming of the gastric tumor microenvironment. Clin Cancer Res (2020) 26:2640–53. doi: 10.1158/1078-0432.CCR-19-3231
13. Yang S, Liu Q, Liao Q. Tumor-associated macrophages in pancreatic ductal adenocarcinoma: Origin. Polarization Function Reprogramming Front Cell Dev Biol (2020) 8:607209. doi: 10.3389/fcell.2020.607209
14. Corcoran RB, Contino G, Deshpande V, Tzatsos A, Conrad C, Benes CH, et al. STAT3 plays a critical role in KRAS-induced pancreatic tumorigenesis. Cancer Res (2011) 71:5020–9. doi: 10.1158/0008-5472.CAN-11-0908
15. Ray KC, Moss ME, Franklin JL, Weaver CJ, Higginbotham J, Song Y, et al. Heparin-binding epidermal growth factor-like growth factor eliminates constraints on activated kras to promote rapid onset of pancreatic neoplasia. Oncogene (2014) 33:823–31. doi: 10.1038/onc.2013.3
16. Zhang Y, Velez-Delgado A, Mathew E, Li D, Mendez FM, Flannagan K, et al. Myeloid cells are required for PD-1/PD-L1 checkpoint activation and the establishment of an immunosuppressive environment in pancreatic cancer. Gut (2017) 66:124–36. doi: 10.1136/gutjnl-2016-312078
17. Poh AR, Ernst M. Tumor-associated macrophages in pancreatic ductal adenocarcinoma: Therapeutic opportunities and clinical challenges. Cancers (Basel) (2021) 13(12):2860. doi: 10.3390/cancers13122860
18. Shi C, Washington MK, Chaturvedi R, Drosos Y, Revetta FL, Weaver CJ, et al. Fibrogenesis in pancreatic cancer is a dynamic process regulated by macrophage-stellate cell interaction. Lab Invest (2014) 94:409–21. doi: 10.1038/labinvest.2014.10
19. Griesmann H, Drexel C, Milosevic N, Sipos B, Rosendahl J, Gress TM, et al. Pharmacological macrophage inhibition decreases metastasis formation in a genetic model of pancreatic cancer. Gut (2017) 66:1278–85. doi: 10.1136/gutjnl-2015-310049
20. Atanasov G, Potner C, Aust G, Schierle K, Dietel C, Benzing C, et al. TIE2-expressing monocytes and M2-polarized macrophages impact survival and correlate with angiogenesis in adenocarcinoma of the pancreas. Oncotarget (2018) 9:29715–26. doi: 10.18632/oncotarget.25690
21. Ye H, Zhou Q, Zheng S, Li G, Lin Q, Wei L, et al. Tumor-associated macrophages promote progression and the warburg effect via CCL18/NF-kB/VCAM-1 pathway in pancreatic ductal adenocarcinoma. Cell Death Dis (2018) 9:453. doi: 10.1038/s41419-018-0486-0
22. Kimsey TF, Campbell AS, Albo D, Wilson M, Wang TN. Co-Localization of macrophage inflammatory protein-3alpha (Mip-3alpha) and its receptor, CCR6, promotes pancreatic cancer cell invasion. Cancer J (2004) 10:374–80. doi: 10.1097/00130404-200411000-00007
23. Zhang D, Li L, Jiang H, Li Q, Wang-Gillam A, Yu J, et al. Tumor-stroma IL1beta-IRAK4 feedforward circuitry drives tumor fibrosis, chemoresistance, and poor prognosis in pancreatic cancer. Cancer Res (2018) 78:1700–12. doi: 10.1158/0008-5472.CAN-17-1366
24. Xian G, Zhao J, Qin C, Zhang Z, Lin Y, Su Z. Simvastatin attenuates macrophage-mediated gemcitabine resistance of pancreatic ductal adenocarcinoma by regulating the TGF-beta1/Gfi-1 axis. Cancer Lett (2017) 385:65–74. doi: 10.1016/j.canlet.2016.11.006
25. D'Errico G, Alonso-Nocelo M, Vallespinos M, Hermann PC, Alcala S, Garcia CP, et al. Tumor-associated macrophage-secreted 14-3-3zeta signals via AXL to promote pancreatic cancer chemoresistance. Oncogene (2019) 38:5469–85. doi: 10.1038/s41388-019-0803-9
26. Steinman RM, Hawiger D, Liu K, Bonifaz L, Bonnyay D, Mahnke K, et al. Dendritic cell function in vivo during the steady state: a role in peripheral tolerance. Ann N Y Acad Sci (2003) 987:15–25. doi: 10.1111/j.1749-6632.2003.tb06029.x
27. Chen B, Zhu L, Yang S, Su W. Unraveling the heterogeneity and ontogeny of dendritic cells using single-cell RNA sequencing. Front Immunol (2021) 12:711329. doi: 10.3389/fimmu.2021.711329
28. Villani AC, Satija R, Reynolds G, Sarkizova S, Shekhar K, Fletcher J, et al. Single-cell RNA-seq reveals new types of human blood dendritic cells, monocytes, and progenitors. Science (2017) 356(6335):eaah4573. doi: 10.1126/science.aah4573
29. Helft J, Anjos-Afonso F, Veen AGvd, Chakravarty P, Bonnet D, Reis e Sousa C. Dendritic cell lineage potential in human early hematopoietic progenitors. Cell Rep (2017) 20:529–37. doi: 10.1016/j.celrep.2017.06.075
30. Hegde S, Krisnawan VE, Herzog BH, Zuo C, Breden MA, Knolhoff BL, et al. Dendritic cell paucity leads to dysfunctional immune surveillance in pancreatic cancer. Cancer Cell (2020) 37:289–307 e289. doi: 10.1016/j.ccell.2020.02.008
31. Lin JH, Huffman AP, Wattenberg MM, Walter DM, Carpenter EL, Feldser DM, et al. Type 1 conventional dendritic cells are systemically dysregulated early in pancreatic carcinogenesis 217. J Exp Med. (2020) 217(8):e20190673. doi: 10.1084/jem.20190673
32. Plesca I, Benesova I, Beer C, Sommer U, Muller L, Wehner R, et al. Clinical significance of tumor-infiltrating conventional and plasmacytoid dendritic cells in pancreatic ductal adenocarcinoma. Cancers (Basel) (2022) 14(5):1216. doi: 10.3390/cancers14051216
33. Lau SP, Klaase L, Vink M, Dumas J, Bezemer K, van Krimpen A, et al. Autologous dendritic cells pulsed with allogeneic tumour cell lysate induce tumour-reactive T-cell responses in patients with pancreatic cancer: A phase I study. Eur J Cancer (2022) 169:20–31. doi: 10.1016/j.ejca.2022.03.015
34. Lau SP, van 't Land FR, van der Burg SH, Homs MYV, Lolkema MP, Aerts J, et al. Safety and tumour-specific immunological responses of combined dendritic cell vaccination and anti-CD40 agonistic antibody treatment for patients with metastatic pancreatic cancer: Protocol for a phase I, open-label, single-arm, dose-escalation study (REACtiVe-2 trial). BMJ Open (2022) 12:e060431. doi: 10.1136/bmjopen-2021-060431
35. Li Z, Liu S, Xu J, Zhang X, Han D, Liu J, et al. Adult connective tissue-resident mast cells originate from late erythro-myeloid progenitors. Immunity (2018) 49:640–653 e645. doi: 10.1016/j.immuni.2018.09.023
36. Tang Y, Wu H, Huo C, Zou S, Hu Y, Yang H. Transcriptomic profiling of mouse mast cells upon pathogenic avian H5N1 and pandemic H1N1 influenza a virus infection. Viruses (2022) 14(2):292. doi: 10.3390/v14020292
37. Rigo R, Chelbi R, Agopian J, Letard S, Griffon A, Ghamlouch H, et al. TET2 regulates immune tolerance in chronically activated mast cells. JCI Insight (2022) 7(7):e154191. doi: 10.1172/jci.insight.154191
38. He Y, Qu J, Yang Q, Wu Z, Liu M, Tso P. Effect of l-glutamine on chylomicron formation and fat-induced activation of intestinal mucosal mast cells in sprague-dawley rats. Nutrients (2022) 14(9):1777. doi: 10.3390/nu14091777
39. Strouch MJ, Cheon EC, Salabat MR, Krantz SB, Gounaris E, Melstrom LG, et al. Crosstalk between mast cells and pancreatic cancer cells contributes to pancreatic tumor progression. Clin Cancer Res (2010) 16:2257–65. doi: 10.1158/1078-0432.CCR-09-1230
40. Guo X, Zhai L, Xue R, Shi J, Zeng Q, Gao C. Mast cell tryptase contributes to pancreatic cancer growth through promoting angiogenesis via activation of angiopoietin-1. Int J Mol Sci (2016) 17(6):834. doi: 10.3390/ijms17060834
41. Ammendola M, Curro G, Laface C, Zuccala V, Memeo R, Luposella F, et al. Mast cells positive for c-kit receptor and tryptase correlate with angiogenesis in cancerous and adjacent normal pancreatic tissue. Clin Exp Allergy (2021) 49(6):874–82. doi: 10.3390/cells10020444
42. Laface C, Laforgia M, Zito AF, Loisi D, Zizzo N, Tamma R, et al. Chymase-positive mast cells correlate with tumor angiogenesis: First report in pancreatic cancer patients. Eur Rev Med Pharmacol Sci (2021) 25:6862–73. doi: 10.26355/eurrev_202111_27234
43. Dahlin JS, Maurer M, Metcalfe DD, Pejler G, Sagi-Eisenberg R, Nilsson G. The ingenious mast cell: Contemporary insights into mast cell behavior and function. Allergy (2022) 77:83–99. doi: 10.1111/all.14881
44. Salomonsson M, Malinovschi A, Kalm-Stephens P, Dahlin JS, Janson C, Alving K, et al. Circulating mast cell progenitors correlate with reduced lung function in allergic asthma. Clin Exp Allergy (2019) 49:874–82. doi: 10.1111/cea.13388
45. Thyagarajan A, Alshehri MSA, Miller KLR, Sherwin CM, Travers JB, Sahu RP. Myeloid-derived suppressor cells and pancreatic cancer: Implications in novel therapeutic approaches. Cancers (Basel) (2019) 11(11):1627. doi: 10.3390/cancers11111627
46. Solito S, Marigo I, Pinton L, Damuzzo V, Mandruzzato S, Bronte V. Myeloid-derived suppressor cell heterogeneity in human cancers. Ann N Y Acad Sci (2014) 1319:47–65. doi: 10.1111/nyas.12469
47. Pylayeva-Gupta Y, Lee KE, Hajdu CH, Miller G, Bar-Sagi D. Oncogenic kras-induced GM-CSF production promotes the development of pancreatic neoplasia. Cancer Cell (2012) 21:836–47. doi: 10.1016/j.ccr.2012.04.024
48. Groth C, Hu X, Weber R, Fleming V, Altevogt P, Utikal J, et al. Immunosuppression mediated by myeloid-derived suppressor cells (MDSCs) during tumour progression. Br J Cancer (2019) 120:16–25. doi: 10.1038/s41416-018-0333-1
49. Stromnes IM, Brockenbrough JS, Izeradjene K, Carlson MA, Cuevas C, Simmons RM, et al. Targeted depletion of an MDSC subset unmasks pancreatic ductal adenocarcinoma to adaptive immunity. Gut (2014) 63:1769–81. doi: 10.1136/gutjnl-2013-306271
50. Bronte V, Brandau S, Chen SH, Colombo MP, Frey AB, Greten TF, et al. Recommendations for myeloid-derived suppressor cell nomenclature and characterization standards. Nat Commun (2016) 7:12150. doi: 10.1038/ncomms12150
51. Xu L, Zhou C, Liang Y, Fan T, Zhang F, Chen X, et al. Epigenetic modifications in the accumulation and function of myeloid-derived suppressor cells. Front Immunol (2022) 13:1016870. doi: 10.3389/fimmu.2022.1016870
52. Khaled YS, Ammori BJ, Elkord E. Increased levels of granulocytic myeloid-derived suppressor cells in peripheral blood and tumour tissue of pancreatic cancer patients. J Immunol Res (2014) 2014:879897. doi: 10.1155/2014/879897
53. Karakhanova S, Link J, Heinrich M, Shevchenko I, Yang Y, Hassenpflug M, et al. Characterization of myeloid leukocytes and soluble mediators in pancreatic cancer: importance of myeloid-derived suppressor cells. Oncoimmunology (2015) 4:e998519. doi: 10.1080/2162402X.2014.998519
54. Takeuchi S, Baghdadi M, Tsuchikawa T, Wada H, Nakamura T, Abe H, et al. Chemotherapy-derived inflammatory responses accelerate the formation of immunosuppressive myeloid cells in the tissue microenvironment of human pancreatic cancer. Cancer Res (2015) 75:2629–40. doi: 10.1158/0008-5472.CAN-14-2921
55. Choueiry F, Torok M, Shakya R, Agrawal K, Deems A, Benner B, et al. 3rd, T.A. mace, CD200 promotes immunosuppression in the pancreatic tumor microenvironment. J Immunother Cancer (2020) 8(1):e000189. doi: 10.1136/jitc-2019-000189
56. Porembka MR, Mitchem JB, Belt BA, Hsieh CS, Lee HM, Herndon J, et al. Pancreatic adenocarcinoma induces bone marrow mobilization of myeloid-derived suppressor cells which promote primary tumor growth. Cancer Immunol Immunother (2012) 61:1373–85. doi: 10.1007/s00262-011-1178-0
57. Yokoyama WM, Kim S, French AR. The dynamic life of natural killer cells. Annu Rev Immunol (2004) 22:405–29. doi: 10.1146/annurev.immunol.22.012703.104711
58. Geiger TL, Sun JC. Development and maturation of natural killer cells. Curr Opin Immunol (2016) 39:82–9. doi: 10.1016/j.coi.2016.01.007
59. Lim SA, Kim J, Jeon S, Shin MH, Kwon J, Kim TJ, et al. Defective localization with impaired tumor cytotoxicity contributes to the immune escape of NK cells in pancreatic cancer patients. Front Immunol (2019) 10:496. doi: 10.3389/fimmu.2019.00496
60. Marcon F, Zuo J, Pearce H, Nicol S, Margielewska-Davies S, Farhat M, et al. NK cells in pancreatic cancer demonstrate impaired cytotoxicity and a regulatory IL-10 phenotype. Oncoimmunology (2020) 9:1845424. doi: 10.1080/2162402X.2020.1845424
61. Liu H, Wang S, Xin J, Wang J, Yao C, Zhang Z. Role of NKG2D and its ligands in cancer immunotherapy. Am J Cancer Res (2019) 9:2064–78.
62. Xu X, Ye L, Zhang Q, Shen H, Li S, Zhang X, et al. Group-2 innate lymphoid cells promote HCC progression through CXCL2-Neutrophil-Induced immunosuppression. Hepatology (2021) 74:2526–43. doi: 10.1002/hep.31855
63. Heinrich B, Gertz EM, Schaffer AA, Craig A, Ruf B, Subramanyam V, et al. The tumour microenvironment shapes innate lymphoid cells in patients with hepatocellular carcinoma. Gut (2022) 71:1161–75. doi: 10.1136/gutjnl-2021-325288
64. Moral JA, Leung J, Rojas LA, Ruan J, Zhao J, Sethna Z, et al. ILC2s amplify PD-1 blockade by activating tissue-specific cancer immunity. Nature (2020) 579:130–5. doi: 10.1038/s41586-020-2015-4
65. Alam A, Levanduski E, Denz P, Villavicencio HS, Bhatta M, Alhorebi L, et al. Fungal mycobiome drives IL-33 secretion and type 2 immunity in pancreatic cancer. Cancer Cell (2022) 40:153–167 e111. doi: 10.1016/j.ccell.2022.01.003
66. Ye L, Pan J, Liang M, Pasha MA, Shen X, D'Souza SS, et al. A critical role for c-myc in group 2 innate lymphoid cell activation. Allergy (2020) 75:841–52. doi: 10.1111/all.14149
67. Wang S, Xia P, Chen Y, Qu Y, Xiong Z, Ye B, et al. Regulatory innate lymphoid cells control innate intestinal inflammation. Cell (2017) 171:201–216 e218. doi: 10.1016/j.cell.2017.07.027
68. Morita H, Kubo T, Ruckert B, Ravindran A, Soyka MB, Rinaldi AO, et al. Induction of human regulatory innate lymphoid cells from group 2 innate lymphoid cells by retinoic acid. J Allergy Clin Immunol (2019) 143:2190–2201 e2199. doi: 10.1016/j.jaci.2018.12.1018
69. Schneider C, Lee J, Koga S, Ricardo-Gonzalez RR, Nussbaum JC, Smith LK, et al. Tissue-resident group 2 innate lymphoid cells differentiate by layered ontogeny and In situ perinatal priming. Immunity (2019) 50:1425–1438 e1425. doi: 10.1016/j.immuni.2019.04.019
70. Huang Y, Mao K, Chen X, Sun MA, Kawabe T, Li W, et al. S1P-dependent interorgan trafficking of group 2 innate lymphoid cells supports host defense. Science (2018) 359:114–9. doi: 10.1126/science.aam5809
71. Zeis P, Lian M, Fan X, Herman JS, Hernandez DC, Gentek R, et al. In situ maturation and tissue adaptation of type 2 innate lymphoid cell progenitors. Immunity (2020) 53:775–792 e779. doi: 10.1016/j.immuni.2020.09.002
72. Lim AI, Li Y, Lopez-Lastra S, Stadhouders R, Paul F, Casrouge A, et al. Systemic human ILC precursors provide a substrate for tissue ILC differentiation. Cell (2017) 168:1086–1100 e1010. doi: 10.1016/j.cell.2017.02.021
73. Siegel RL, Miller KD, Fuchs HE, Jemal A. Cancer statistics. CA Cancer J Clin (2022) 72:7–33. doi: 10.3322/caac.21708
Keywords: pancreatic cancer, tumor immune microenvironment, innate immune lymphocyte, lineage tracing, innate immunity
Citation: Ye L, Shi S and Chen W (2023) Innate immunity in pancreatic cancer: Lineage tracing and function. Front. Immunol. 13:1081919. doi: 10.3389/fimmu.2022.1081919
Received: 27 October 2022; Accepted: 28 December 2022;
Published: 16 January 2023.
Edited by:
Tahseen H. Nasti, Emory University, United StatesReviewed by:
Rajesh Valanparambil, Emory University, United StatesSakshi M., Emory University, United States
Copyright © 2023 Ye, Shi and Chen. This is an open-access article distributed under the terms of the Creative Commons Attribution License (CC BY). The use, distribution or reproduction in other forums is permitted, provided the original author(s) and the copyright owner(s) are credited and that the original publication in this journal is cited, in accordance with accepted academic practice. No use, distribution or reproduction is permitted which does not comply with these terms.
*Correspondence: Wei Chen, dmlvZ3JvQDE2My5jb20=
†These authors have contributed equally to this work