- 1Department of Stem Cell Transplantation and Cellular Therapy, The University of Texas MD Anderson Cancer Center, Houston, TX, United States
- 2Department of Medicine III, Hematology and Oncology, School of Medicine, Technical University of Munich, Munich, Germany
- 3Department of Radiation Oncology, The University of Texas MD Anderson Cancer Center, Houston, TX, United States
The advent of cellular immunotherapy in the clinic has entirely redrawn the treatment landscape for a growing number of human cancers. Genetically reprogrammed immune cells, including chimeric antigen receptor (CAR)-modified immune effector cells as well as T cell receptor (TCR) therapy, have demonstrated remarkable responses across different hard-to-treat patient populations. While these novel treatment options have had tremendous success in providing long-term remissions for a considerable fraction of treated patients, a number of challenges remain. Limited in vivo persistence and functional exhaustion of infused immune cells as well as tumor immune escape and on-target off-tumor toxicities are just some examples of the challenges which restrain the potency of today’s genetically engineered cell products. Multiple engineering strategies are being explored to tackle these challenges.The advent of multiplexed precision genome editing has in recent years provided a flexible and highly modular toolkit to specifically address some of these challenges by targeted genetic interventions. This class of next-generation cellular therapeutics aims to endow engineered immune cells with enhanced functionality and shield them from immunosuppressive cues arising from intrinsic immune checkpoints as well as the hostile tumor microenvironment (TME). Previous efforts to introduce additional genetic modifications into immune cells have in large parts focused on nuclease-based tools like the CRISPR/Cas9 system or TALEN. However, nuclease-inactive platforms including base and prime editors have recently emerged and promise a potentially safer route to rewriting genetic sequences and introducing large segments of transgenic DNA without inducing double-strand breaks (DSBs). In this review, we discuss how these two exciting and emerging fields—cellular immunotherapy and precision genome editing—have co-evolved to enable a dramatic expansion in the possibilities to engineer personalized anti-cancer treatments. We will lay out how various engineering strategies in addition to nuclease-dependent and nuclease-inactive precision genome editing toolkits are increasingly being applied to overcome today’s limitations to build more potent cellular therapeutics. We will reflect on how novel information-rich unbiased discovery approaches are continuously deepening our understanding of fundamental mechanisms governing tumor biology. We will conclude with a perspective of how multiplexed-engineered and gene edited cell products may upend today’s treatment paradigms as they evolve into the next generation of more potent cellular immunotherapies.
1 Introduction
Chimeric antigen receptor (CAR) engineering of immune effector cells (IEC), such as T cells or Natural Killer (NK) cells, is a promising frontier of personalized cancer therapy, manifesting sustained remissions in specific populations of patients with relapsed or refractory (R/R) lymphoid malignancies (1). This approach entails reprogramming immune effector cells with synthetic receptors equipped with multiprong features enabling antigen recognition, downstream signaling, and costimulatory activation, ultimately resulting in targeted elimination of antigen-expressing tumor cells. The promise of CAR-T cell therapy has primarily been realized through CD19-directed therapies against B cell malignancies (2–6), and more recently through BCMA-directed therapies against multiple myeloma (7, 8), for which there are several commercial products now available. This success has spurred extensive preclinical investigation of cellular immunotherapy against other malignancies with an expectant dynamic and exciting pace of new indications. However, the clinical efficacy of CAR-based therapies against other malignancies, particularly solid tumors, has not yet been matched in many settings due to issues relating to trafficking, infiltration, clonal heterogeneity, off-target activity or on-target off-tumor activity against normal tissues, and the hostile immunosuppressive TME (9). There are additional logistical and clinical limitations to CAR-T cell therapy. The cumbersome and lengthy production associated with manufacturing an autologous product renders high expenditure and restrictive use (10). Even if previously generated allogeneic T cell products are HLA-matched with the recipient, genetic modification is still required to reduce the risk of graft-versus-host disease (GvHD) mediated by the native αβ T-cell receptor (TCR). Furthermore, CAR-T cell therapy can cause toxicities, such as immune effector cell–associated neurotoxicity syndrome (ICANS) and cytokine release syndrome (CRS), which can increase hospitalization duration and treatment cost (10). NK cells are innate immune cells that serve as an attractive alternative platform for CAR engineering and offer several advantages to the use of T cells, including avoiding the risk of GvHD, scalability in an off-the-shelf allogeneic cellular product, and a superior safety profile (10).
Enduring challenges with CAR engineered effector cells that may yield suboptimal response and disease relapse include i) antigen negative recurrence from immunoediting and antigen escape in the tumor, or ii) limited in vivo persistence or functional exhaustion of the CAR-engineered cells, resulting in antigen positive relapse (11). Strategies to combat antigen negative relapse thematically involve targeting multiple antigens either simultaneously or in a sequential manner. Approaches to overcome effector exhaustion or to increase proliferation and potency are varied and include i) altering the choice of co-stimulation (12), ii) cytokine cargo coupling (e.g. IL-15, IL-12, IL-18) with awareness of the detriment from unconstrained tonic signaling (13), and iii) synergizing CAR adoptive cell therapy with checkpoint blockade (14), among other tactics. Such advancements are being supplanted with pioneering, multiplexed gene editing tools including Clustered Regularly Interspaced Short Palindromic Repeats (CRISPR) based editing to introduce deliberate genetic perturbations that enhance the potency of CAR effectors by, for example, reprograming immune cell metabolism, equipping cells with methods to resist exhaustion, disrupting pathways for immunosuppression, or improving survival (11). Such interventions and investigations show enormous promise in the marriage of sophisticated, hypothesis-driven modification of immune cells in a site-directed fashion to modulate cellular pathways that negatively impact the efficacy and fitness of CAR-modified effector cells.
In this review, through the prism of challenges the field has been facing with CAR-based immunotherapeutic strategies, we will discuss the evolution of innovative multiplex engineering strategies and precision gene editing tools that are designed to enhance the therapeutic scope and potency of this groundbreaking personalized therapy.
2. Multiplexed engineering as a tool to enhance cellular therapies
The expanding possibilities in personalized medicine coincide with the dramatic improvements in immune cell engineering, most notably the successful transition of CAR-T and CAR-NK cells as well as TCR gene therapy (15) into the clinic.
One of the most valuable features of cellular immunotherapy lies in the capacity of infused immune cells to expand and evolve in vivo. Indeed, some studies point towards peak CAR transcript levels as assessed in the patients’ peripheral blood to be a potential predictor of clinical response (16, 17). Contrary to antibody-based strategies, which often need to be administered continuously to mitigate rapid in vivo degradation, cell therapy products are ‘living drugs’ which exhibit striking plasticity with regard to their phenotypic, transcriptomic and epigenetic composition post-infusion. This dramatic evolution is shaped by the continuous selective pressures arising from the interaction with both the tumor cells as well as bystander host immune cells and may have profound effects on the persistence, memory formation and anti-tumor potency of infused immune effector cells (18–20). Seminal studies have in recent years investigated the single-cell trajectories of infused CAR-T cells and found key pre-infusion transcriptomic signatures which correlate with response and potential toxicity and may in the future serve as predictive biomarkers to help guide data-driven treatment decisions (21–24).
Novel high-dimensional assays with single-cell resolution have recently elucidated the complex epigenetic underpinnings of T cell exhaustion (25–30), and it is likely that other immune effector cells, including genetically engineered lymphocytes are subject to and governed by many of the same mechanisms although much is still to be learned in this regard.
Multiplexed gene engineering, ultimately, attempts to modulate these cellular signatures to enhance the safety and efficacy of cell therapies. By combining the effective delivery of synthetic receptors with additional cellular modifications brought about by the opportunities arising from precise gene editing, the next generation of multiplexed engineered cellular immunotherapies strives to enhance anti-tumor potency, increase in vivo persistence and overcome functional exhaustion by increasing cellular fitness and/or shielding engineered immune cells from the inhibitory cues received from endogenous checkpoints or immunosuppressive metabolites in the tumor microenvironment (TME) by introducing multiple genetic modifications (Figure 1).
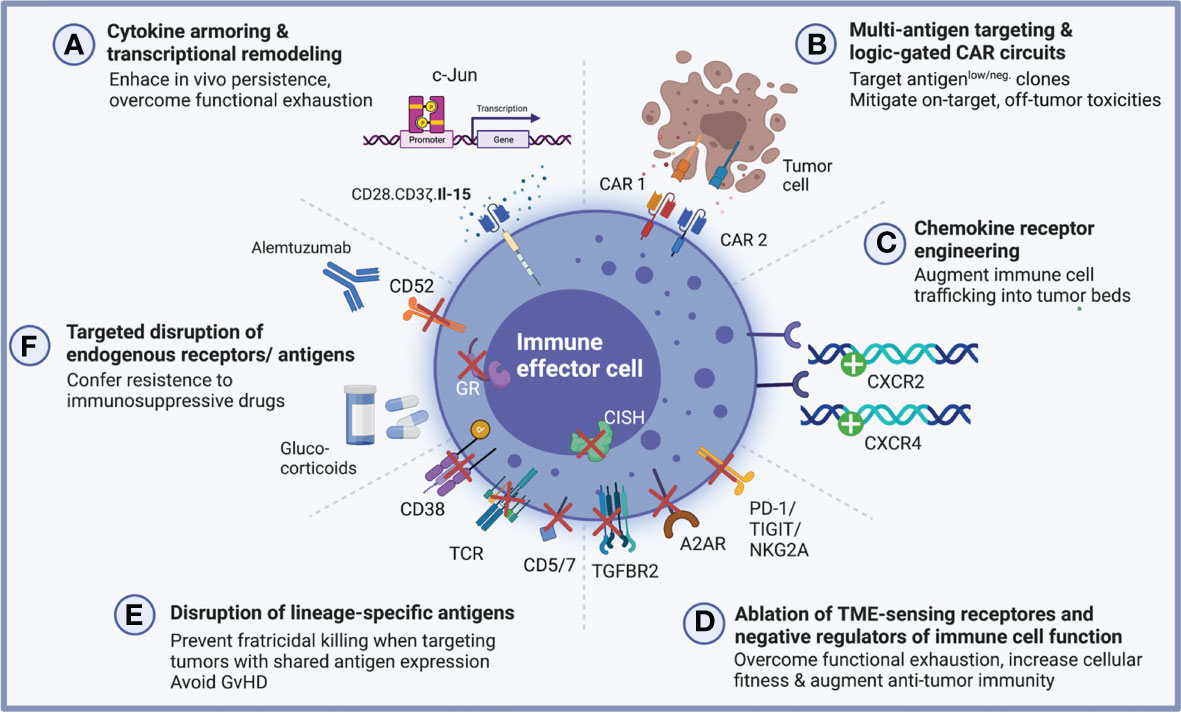
Figure 1 Multiplexed engineering and gene editing strategies to augment anti-tumor potency and cellular fitness. A growing number of multiplexed engineering approaches have been pursued to enhance the cellular fitness and augment anti-tumor potency of genetically engineered antigen-specific immune effector cells. (A) Cytokine-armored immune effector cells are reprogrammed using a multi-cistronic vector system which endows them with antigen specificity and furthermore encodes for a specific cytokine to provide autocrine growth support for enhanced functionality. In the setting of CAR-NK cells, this strategy has successfully been employed to augment in vivo persistence through ectopic IL-15 secretion (31). Epigenetic modulation by targeted insertion of transcription factors may confer exhaustion resistance as has been shown for c-Jun in CAR-T cells (32). (B) Combinatorial targeting strategies including dual CARs promise to increase IEC potency against antigen-low tumor clones by recognizing multiple relevant target antigens (33, 34). When combined with logic-gated circuits, these multi-receptor engineering approaches may overcome on-target, off-tumor toxicities which might arise from shared antigen expression between tumor and healthy tissue (35–37). (C) Through the targeted insertion of chemokine receptors, immune effector cells can be rewired to traffic more effectively into tumor beds, a critical lever to engineer cell products which are capable of combatting solid tumors (38, 39). (D) Building on the clinical success of immune checkpoint blockade, efforts are underway to genetically disrupt known negative regulators of immune cell function including, for instance, PD-1, TIGIT and NKG2A as well as TME-sensing receptors which bind immunosuppressive metabolites including adenosine and TGFβ (40–56). (E, F) Deletion of lineage-specific antigens or endogenous receptors may render engineered immune cells resistant to fratricidal killing, which is crucially important when targeting malignancies with an overlapping surfaceome (57, 58). For allogeneic CAR-T cells in particular, elimination of the endogenous T cell receptor (TCR) prevents the deleterious effects of alloreactivity and GvHD induction and site-directed engineering strategies now accomplish both simultaneously - deletion of the endogenous TRAC locus and CAR delivery (59, 60). Lastly, genetic deletion of endogenous antigens and receptors may prove advantageous when co-administrating lymphodepleting and immunosuppressive drugs (61, 62). Created with BioRender.com.
In the following section, we will outline the most pivotal examples of multiplexed engineered immune cells, a growing fraction of which have moved into clinical testing.
2.1. Synthetic transgenic payloads for enhanced functionality
2.1.1. Cytokine armoring
Cytokine armoring was one of the early examples for how multiplexed engineering can endow genetically modified immune cells with enhanced functionality (Figure 1A).
Bi- and multi-cistronic vector systems are now routinely deployed to deliver synthetic external receptors for tumor antigen recognition together with additional molecular payloads such as cytokines for autocrine growth support (31, 63–71). For NK cells in particular, IL-15 has long been known to be a critical determinant for prolonged in vivo persistence (71) and different strategies have been devised to endow NK cells with IL-15 mediated autocrine growth support. In a phase I/II trial led by our group, NK cells engineered to express a CD19-directed CAR molecule together with IL-15 demonstrated remarkable responses in heavily pre-treated patients with CD19 positive hematologic malignancies (16, 31). Similar strategies are being pursued by others, for instance by retaining IL-2 within the endoplasmic reticulum (72) or by equipping NK cells with a membrane-bound IL-15/IL-15 receptor fusion protein (73).
Building on the observation that exposure to certain cytokines can fundamentally remodel the transcriptomic signature of immune cells and enhance their potency and even induce formation of a memory-like phenotype (74–77), there has been a growing interest in exploring other cytokines beyond IL-15 to engineer more powerful immune cell immunotherapies for sustained clinical responses (13, 78). Interestingly, this transcriptional remodeling can give rise to novel, thus far unrecognized, immune cell signatures, as exemplified by a recent report on IL-12-transduced CAR-T cells which emulate an NK cell-like phenotype with the capacity for antigen-independent HLA-E-restricted killing – a property which might complement CAR-driven responses by preventing immune escape via antigen loss (78).
Other strategies employed to remodel the transcriptional landscape and tune CAR-modified immune cells towards higher anti-tumor potency rely on the direct ectopic expression of transcription factors as exemplified by work from Crystal Mackall’s group on c-Jun signaling to overcome CAR-T functional exhaustion (32).
2.1.2. Multi-targeting CARs & rational design of logic circuits
CAR-engineered cellular immunotherapies have shown remarkable paradigm-shifting successes particularly for liquid cancers. CD19-positive lymphoid malignancies are particularly well-suited to be targeted using CAR-engineered IECs as target antigen expression is relatively high and on-target off-tumor toxicities can be managed in many cases. Although antigen-low or antigen-negative relapses represent a major challenge (79) and efforts are underway to fine-tune CAR signal strength to address low antigen density (80), other human cancers have been disproportionally harder to target as they lack uniform expression of tumor-specific antigens or are characterized by a higher degree of clonal heterogeneity. Solid tumors in particular pose extraordinary challenges to engineered immune cells as they exhibit a set of unique challenges including shared antigen expression with healthy tissues, impaired trafficking, and homing into tumor beds, a hostile and immunosuppressive tumor microenvironment (TME) and a more heterogenous clonal architecture. Overcoming these challenges, hence, promises to target a broader range of human cancers and has driven a spur of innovation in recent years.
One strategy to address the relative paucity of tumor-specific antigens as well as tumor immune evasion due to clonal evolution and emergence of antigen-negative clones has been to engineer bispecific dual or tandem CAR-modified immune effector cells, some of which are governed by logic-gated circuits to specifically differentiate between shared targets expressed on cancerous cells versus healthy tissues (Figure 1B).
Recent work on bispecific CD19/CD22-directed CAR-T cells demonstrated the potential of dual targeting approaches to enhance anti-tumor activity and efforts are underway to further advance multi-specific CAR targeting both in the pre-clinical and clinical settings (33, 34, 79, 81, 82). Advancements in multi-receptor-based engineering approaches and in the ability to reprogram the intracellular signaling networks underpinning CAR activation has allowed for the design of logic-gated CAR-T cells which can effectively target tumor-associated antigens while avoiding on-target off-tumor toxicity and thus sparing healthy tissues (83, 84). Preclinical data reported by the Seattle group have demonstrated how CAR-T cells targeting tumor-associated ROR-1 can be prevented from inducing otherwise toxic abrogation of healthy bone marrow stromal tissue by endowing them with synthetic notch receptors (SynNotch) which specifically recognize EpCAM or B7-H3 leading to tumor regression while sparing healthy tissues (35). In NK cells, similar strategies have been conceived, for instance the recent report of FLT3 OR CD33 NOT EMCN CAR-NK cells which are activated by CD33+ or FLT3+ leukemic blasts but inhibited by concomitant recognition of Endomucin, which is expressed by the majority of healthy hematopoietic progenitor cells (HSCs) (36).
In a similar vein, preliminary reports have laid out an IF-BETTER gated combinatorial CAR design which selectively targets AML bulk and leukemic stem cells using an ADGRE2-targeting CAR molecule combined with a CLECL12A-directed chimeric costimulatory receptor (CCR). By adding CLEC12A as a rationally selected combinatorial target, T cells engineered to express this logic-gated construct recognize both ADGRE2high/CLEC12Aneg and ADGRE2low/CLEC12Ahigh leukemic cells, preventing ADGRE2low immune escape while sparing ADGRE2low/CLEC12Aneg healthy hematopoiesis (37). Efforts to further advance logic circuits for precision targeting of tumor cells are underway and allow the construction of various logical conditionalities (e.g., AND, OR, NOT). Today, these synthetic circuits are not restricted to extracellular antigens but can also be applied to recognize MHC-bound intracellular tumor-associated peptides, for instance via scFv fragments targeting specific peptide-MHC complexes fused to synNotch receptors for conditional activation (85).
Besides widening the therapeutic window when targeting shared antigens, dual CAR strategies can also be harnessed to mitigate fratricidal killing of neighboring CAR-engineered IECs, which can occur following transfer of the targeted antigen from the tumor cells to the IECs through means of trogocytosis. As was recently illustrated for CD19-directed CAR-NK cells, integration of KIR-based inhibitory CAR receptors can shield NK cells from such effects and yield more potent tumor killing (86).
Collectively, these early examples foreshadow the enormous potential of rationally designed multi-targeted CAR constructs to precisely target and discriminate tumor cells from normal cells, addressing one of the most foundational challenges in cancer therapy.
2.1.3. Chemokine receptor engineering
Besides shared antigen expression, impaired trafficking of immune cells into tumors represents another formidable hurdle to overcome to engineer more effective cellular immunotherapies, particularly, when aiming to combat solid tumors. In the realm of CAR-T cells, many studies have evaluated multiplexed engineering strategies to equip CAR- and TCR-modified T cells with chemokine receptors for enhanced tumor infiltration (87–96). In NK cells, similarly, a number of studies have reported improved NK cell infiltration and anti-tumor potency by overexpressing chemokine receptors (38, 97, 98) or augmenting release of chemokines from tumor beds (99, 100). The first chemokine receptor-engineered CAR-NK cells to be reported leveraged ectopic CXCR4 expression for enhanced trafficking into glioblastoma tumor beds (39) (Figure 1C). Similar efforts are being pursued by others (101). Nevertheless, much is still to be learned to fully leverage the potential of augmented chemokine signaling as many challenges remain. Most noteworthy, the mechanisms of impaired tumor microcirculation resulting in inadequate chemotactic gradients as well as context-specific differences in the ultimate effects of chemokine receptor-ligand interactions need to be better understood for these tools to successfully transition into the clinic.
3. Multiplexed genome editing as a tool to enhance cellular therapies
While the previous section focused on engineering strategies aiming at introducing synthetic transgenic payloads to enhance immune cell function, the following section aims to portray precision genome editing tools being employed to disrupt and modulate endogenous immune cell pathways which ultimately restrain anti-tumor potency.
3.1. Evolution of gene editingtool kits
3.1.1. Nuclease-based genome editing
Over the past decades, a plethora of gene editing strategies have been devised to introduce targeted genetic modifications into host genomes of eukaryotic cells (Figure 2). Long before the advent of the CRISPR/Cas9 system, zinc-finger nucleases (ZFNs) made their debut in the 1980s and allowed for the first time for site-directed gene editing by recognizing specific genomic sequences, leading to notable successes including the generation of autologous CCR5-/- CD4 T cells for the treatment of HIV (102) and genetic ablation of the checkpoint molecule PD-1 in tumor infiltrating lymphocytes (40). Transcription activator-like effector nucleases (TALENs), which are structurally related to ZFNs, followed in 2009 and further increased target specificity by binding more than 30 base pairs. Within the realm of cell therapy, TALEN technology has been used to engineer allogeneic CAR-T cells which are devoid of their endogenous T cell receptor (TCR) to prevent harmful GvHD (61). Arguably the most prominent toolkit for genetic editing is provided by the CRISPR/Cas9 system, which allows for a high degree of customization with respect to site-specificity at comparably low cost.
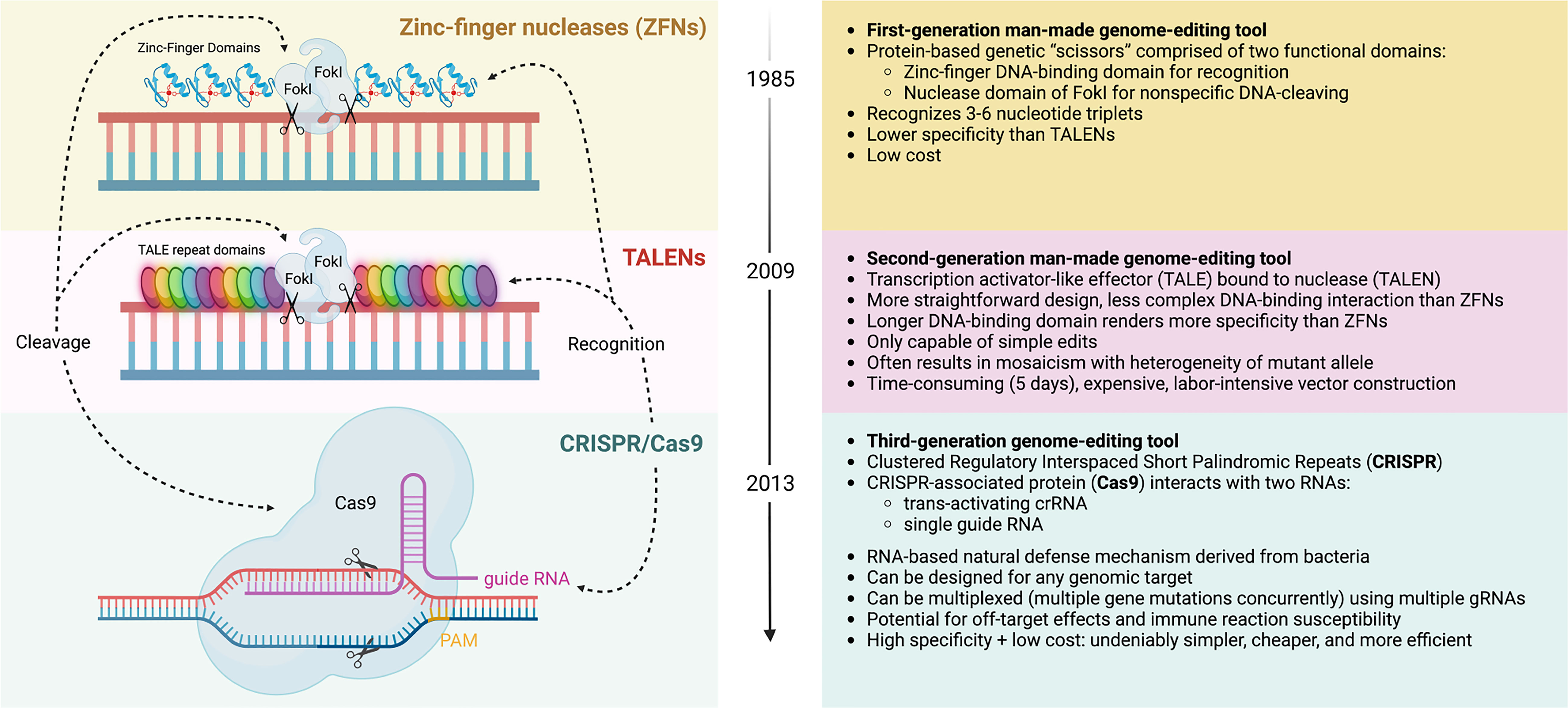
Figure 2 Evolution of gene editing toolkits. Zinc-finger nucleases (ZFNs) are a first-generation man-made gene-editing tool that consists of two domains: 1) a DNA-cleaving domain comprised of the non-specific nuclease domain of FokI to introduce DNA double-stranded breaks, and 2) two DNA-binding domain chains, called “finger” modules, each recognizing a unique hexamer (6 bp) sequence of DNA. The fusion of DNA-binding and DNA-cleaving domains forms a pair of “genomic scissors”, with high specificity but lower than that of Transcription activator-like effector nucleases (TALENs) or Clustered Regulatory Interspaced Short Palindromic Repeats (CRISPR) gene editing systems. TALENs are a second-generation gene-editing tool also based on chimeric nucleases like ZFN with the same non-specific DNA cleavage domain (FokI), but it comprises of longer sequence-specific DNA-binding TALE modules, each of which contacts a single DNA base pair. By fusing with nuclease (TALE-Nuclease or TALEN), this tool can be used to edit genes, but only one at a time, unlike CRISPR. The longer programmable DNA-binding domain comprises of a series of 33-35 amino acid repeat domains, which allows for improved specificity than ZFNs, but increases cost and is marked by a low-efficiency process of vector construction. TALEN editing may induce mosaicism, in which mutations are only present in some transfected cells. The advent of CRISPR/Cas9 gene editing more recently has allowed for the creation of a tool with low cost, high specificity, high efficiency, and much simpler construction. This RNA-based tool is derived from a natural bacterial defense mechanism. The nuclease consists of the CRISPR-associated protein (Cas9) protein classically, though other Cas proteins have been used for cleaving. The complex initially binds to a short sequence known as the protospacer adjacent motif (PAM). This nuclease interacts with two RNAs for recognition and specificity, a trans-activating crRNA and single guide RNA, sometimes combined for simplicity. Notably, CRISPR allows for multiplexed gene editing, where multiple gene mutations can be issued concurrently. Complications include the potential for off-target effects and potential immune reaction susceptibility. As indicated, all systems employ a recognition module and cleavage domain that can be manipulated independently. Created with BioRender.com.
Over the past several years, multiple studies have explored preclinical applications of CRISPR technology to optimize cellular therapeutics based on T cells (5–10) as well as NK cells (11–13). In 2020, this progress culminated in the first successful clinical application of CRISPR-engineered TCR-T cells (103). Common to all these Cas9-mediated editing strategies is the induction of double-strand breaks (DSBs) in DNA which are subsequently repaired by either non-homologous end joining (NHEJ) or homology-directed repair (HDR). Incomplete repair of these breakage points may give rise to indels leading to frameshift mutations and subsequent knock-out of one or more targeted genes. When combined with DNA donor template, the same toolkit allows the targeted integration of exogenous DNA inserts at defined genomic loci (104) (Figure 3). Incremental improvements have, over time, expanded the potential length of the genetic payloads which can be inserted and increased editing efficiency. Nevertheless, challenges to deliver template DNA to target cells persist and limited abundance of template DNA continues to represent a potential bottleneck for targeted genomic insertions (125–127).
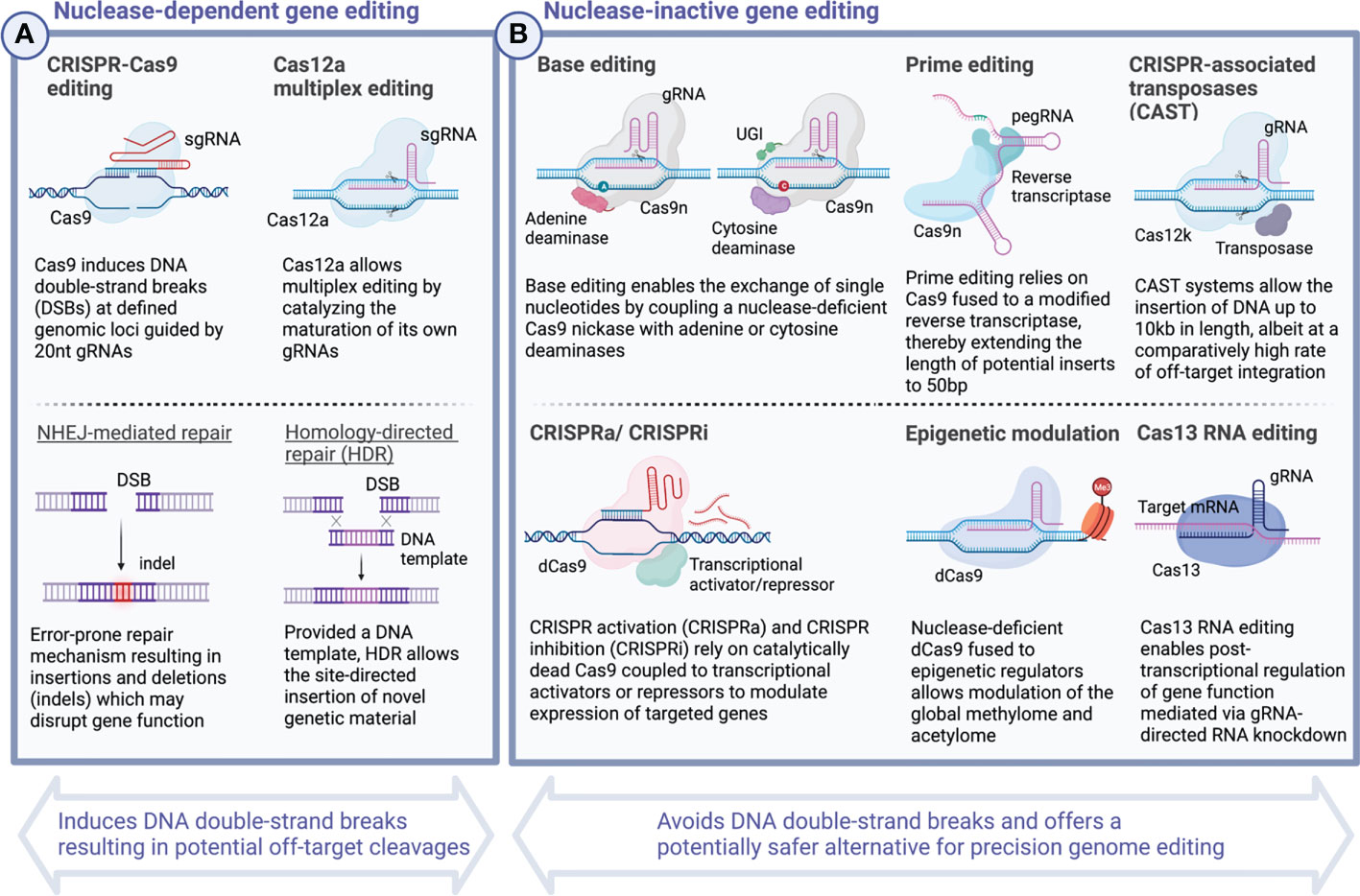
Figure 3 CRISPR - A versatile toolkit for the targeted deletion, rewriting and insertion of novel genetic information. Continuous iterations of technological innovation have considerably extended the scope and versatility of CRISPR-based gene editing strategies. Today, CRISPR genome editing encompasses a wide spectrum of diverse applications including the direct rewriting of genetic code, modulation of gene expression, and post-transcriptional RNA editing. While the most widely known CRISPR-Cas9 system and others rely on inducing DNA double-strand breaks (DSBs) and carry the potential risk of introducing deleterious effects arising from off-target cleavages (A), other CRISPR-based editing tools, including base editing (105, 106) and prime editing (107), allow to rewrite the genetic code without the requirement for DNA double-strand cleavages and, hence, present a potentially safer alternative (B). Over time, the possibilities to insert novel genetic material have expanded dramatically with the most recent advances including Twin prime editing (108) and CRISPR-associated transposase (CAST)-editing (109) now allowing large transgenic inserts in the range of up to 10-40 kb length. Besides editing the genetic code, powerful tools now exist which allow to tune transcriptional activation in a targeted manner. CRISPRa and CRISPRi rely on the site-directed delivery of transcriptional effector molecules to activate or repress gene expression (110–113). More profound epigenetic modulation can be achieved by tethering catalytically dead Cas9 to methyltransferases and acetyltransferases to alter the global methylome and edit histone modifications (114–124). Created with BioRender.com.
Retron-derived template DNA promise to overcome this technical limitation by drastically increasing the concentrations of available template DNA which in turn may increase precision genome-editing efficiency (128). Retrons serve a role in bacterial phage defense and possess the capacity for reverse transcription (RT) (128–132). Recently, their ability to generate designer RT-DNA in vivo was shown to be feasible for human genome-editing applications (128). Over time, it will be interesting to observe how the various platforms of precise-genome engineering will evolve and which ones will prove to be most suitable for clinical translation in the space of engineered immune cell therapeutics.
3.1.2. Nuclease-inactive precision genome editing
Despite their great promises, all previously outlined nuclease-based gene editing strategies hold the potential safety concern of introducing inadvertent off-target cleavages which may lead to potentially deleterious consequences (133–137).
In 2016, researchers around David Liu devised a novel strategy which allows the introduction of genetic alterations without the requirement of inducing DSBs (Figure 3). Base editing traditionally refers to the sgRNA-directed exchange of single nucleotides mediated by modified forms of Cas9 protein, Cas9 nickases (nCas9), which lack the capacity to cleave DNA, but instead are fused to bacterial deaminases to substitute single nucleotides (105, 106, 138), although recently CRISPR-free all-protein base editors have been described (139). For nCas9-mediated base editing, single-strand DNA point mutations are subsequently resolved in the process of replication and are used to deliberately alter the codon sequence including insertion of premature STOP codons. Base editors allow correction of pathogenic allele variants offering novel treatment avenues for monogenetic disorders including some causes of sickle cell disease (140) and β-thalassemia (141, 142). The first base editor to have entered the clinic aims to silence PCSK9 in patients with heterozygous familial hypercholesterolemia and further candidates are already on the horizon (141, 143).
The last years have seen the first applications of base editing to cellular immunotherapy (144), for instance to engineer fratricide-resistant CAR-T cells by targeting the pan-T lineage antigens CD3 and CD7 (145). Another group recently reported the introduction of four simultaneous base edits to generate allogeneic CD7-directed CAR-T devoid of endogenous expression of TRAC, CD52, CD7 and PD-1 (146).
While base editing allows for transition mutations (purine-for-purine and pyrimidine-for-pyrimidine substitutions), it is limited in its ability to perform transversion mutations (exchanging purines for pyrimidines and vice versa), targeted deletions and insertions which would be required to correct for genetic disorders including Tay-Sachs disease and cystic fibrosis among others (107). Prime editing overcomes this limitation by linking nCas9 to a reverse transcriptase, both of which are guided to a desired genomic locus by a customizable prime editing guide RNA (pegRNA) (107) (Figure 3). After binding to the target region, prime editing allows for single base exchanges as well as insertions and deletions of synthetic DNA sequences of limited length (107). Efforts are ongoing to increase both prime editing efficiency (147) as well as the potential size of targeted inserts, the latter of which has recently been expanded to incorporate DNA sequences of up to 5kb length using a platform dubbed twin prime editing (TwinPE) (108).
In recent years, the field has seen continuous incremental improvements in editing efficiency, precision, and versatility. Novel genome engineering strategies have emerged which build on existing platforms to further expand their utility. Programmable Addition via Site-specific Targeting Elements (PASTE), for instance, uses prime editing technology to insert specific landing sites (AttB) into the host genome which allows subsequent introduction of large genomic sequences of up to 36kb length (148). Yet another strategy for large genomic insertions relies on CRISPR-associated transposases (CAST), which have been demonstrated to enable the insertion of templates of up to 10 kb, however, with a considerable fraction of 50% off-target integrations (109). Against this backdrop, it will be interesting to see how these approaches can eventually be applied to enhance the safety and potency of cell therapy products.
3.2. Disruption of immune checkpoints and TME-sensing receptors
Precision genome editing strategies including CRISPR/Cas9 and TALEN allow the disruption of inhibitory immune cell pathways, for instance, by ablation of immune checkpoint molecules or TME-sensing receptors (Figure 1D). To enhance CAR-T potency, genetic perturbations have targeted negative regulators of T cell function including PD-1 (41–46), CTLA-4 (43, 45), TIGIT (45), TIM-3 (45–47), LAG-3 (45, 46, 48). Knockdown of GM-CSF in CD19-directed CAR-T cells additionally led to mitigated IEC-mediated toxicities in one pre-clinical study (149). In NK cells, seminal work established the crucial role of the intracellular regulatory checkpoint CIS which acts to suppress cytokine signaling and can be targeted using the CRISPR/Cas9 editing for enhanced NK cellular fitness and potency in non-engineered or CAR engineered settings (49, 50, 150). NKG2A, another potent inhibitory molecule in NK cell, similarly leads to enhanced NK cell potency when genetically blocked, and it will be interesting to see whether this strategy can be translated to build NKG2A-disrupted CAR-NK cells that are resistant to tumors expressing its cognate ligand HLA-E (51).
Receptors sensing the immunosuppressive metabolites of the tumor microenvironment (TME), have likewise been targeted to render NK cells resistant towards these inhibitory cues. Transforming growth factor β1 (TGF-β) is a powerful immunosuppressive cytokine which can have profound negative effects on the anti-tumor immunity of NK cells. CRISPR-mediated targeted ablation of TGF-βR2 rescued NK cell effector functions from these deleterious effects (52, 53). Similarly, genetic disruption of the adenosine A2A receptor may contribute to enhanced CAR-T efficacy as it effectively shields them from the negative consequences of the immunosuppressive metabolite adenosine (54).
3.3. Disruption of lineage-specific antigens to mitigate fratricide and expand clinical utility
Other applications using genetic ablation have aimed to delete lineage-specific antigens which might otherwise result in fratricidal killing of engineered immune cells (Figure 1E). For CAR-T cells, this has been of particular importance when targeting T cell malignancies which share the same pan-T cell antigens including CD5 and CD7 (57). In NK cells, disruption of endogenous CD38 has enabled the design of cell products which, when combined with anti-CD38 monoclonal antibodies (mAbs), confer anti-myeloma specificity for antibody-dependent cellular cytotoxicity (ADCC) while shielding NK cells from CD38-mediated fratricide (151).
Other manipulations can expand the therapeutic breadth of immune effector cells, e.g., by knocking out CD52 (61, 152) or the glucocorticoid receptor (62) to promote resistance towards treatment-induced immunosuppression when co-administered with alemtuzumab or corticosteroids, respectively (Figure 1F).
Furthermore, engineered immune cells can be reprogrammed for more favorable interactions with the host immune system, e.g., by disrupting MHC-1 molecules to avoid host-mediated rejection of engineered T cells, or to prevent GvHD by deleting the endogenous TRAC locus, as is necessary for universal allogeneic CAR-T cell candidates (44, 59, 153).
3.4. Sequence-specific editing to enhance immune cell potency
Iterations of TRAC locus engineering now allow for the site-directed insertion of CAR molecules to replace the endogenous T cell receptor signaling complex and thereby reduce the propensity of engineered cells for tonic signaling which has been implicated in premature T cell exhaustion (59). Other strategies leverage sequence-specific editing to fine-tune and optimize antigen sensitivity of engineered T cells as was demonstrated by the generation of HLA-independent T cell receptors (HIT receptors), which exhibit drastically heightened antigen affinity for killing of tumor cells with low antigen density (60).
In conclusion, the high degree of modularity and flexibility for customization provided by the evolving genome engineering technologies has ushered in a new era of cell therapy products which are characterized by rational design approaches building on the ever deeper molecular understandings of tumor-immune cell interactions.
4 CRISPR-enabled forward genetic screening
Great strides have been made in recent years to endow immune cells not only with antigen specificity but also enhanced functionality and increased potency by multiplexed genetic engineering and gene editing strategies. Common to all these efforts is that they attempted to overcome previously established and recognized mechanisms of immune cell dysfunction and anergy. While the field is eagerly waiting to see the outcomes when these multi-engineered cell products eventually advance to the clinics, novel unbiased and large-scale discovery approaches have in recent years opened up new avenues to decode novel and undescribed mechanisms of immune cell regulation that might prove instrumental in designing the next generation of engineered immune effector cells.
Pooled loss-of-function CRISPR screens in cancer cell lines have become an invaluable tool to discern molecular mechanisms of therapeutic sensitivity and resistance. A large body of work across a number of different human cancer types has elucidated genetic dependencies that determine responses to anti-neoplastic treatments (154, 155). In recent years, combinatorial screens have further expanded these possibilities and allowed to identify systematically distinct genetic interactions, for instance, to discover synthetic lethal partners which might be exploited for pharmacological interventions. The unique molecular features of the DNA endonuclease Cas12a, which can simplify multiple simultaneous genetic edits, has greatly improved the editing efficiency compared to traditional Cas9 approaches and now permits successful identification of hits in combinatorial drop-out screens (156–159).
Whereas combinatorial screens can bring to light genetic interactions which may drive the onset, maintenance or progression of tumor cells, other platforms set out to investigate cancer-type dependent genetic dependencies. As is increasingly understood, CAR-T resistance mechanisms may vary dramatically across tumor types and explain some of the observed differential sensitivity for solid tumors versus hematologic malignancies (160). Novel platforms aim to investigate these distinct patterns of tumor-intrinsic resistance pathways in a massively parallel manner. For instance, CRISPR loss-of-function screening applied to a pool of hundreds of barcoded cancer cell lines (PRISM) in an orthogonal screening format recently helped elucidate shared cancer-intrinsic transcriptional signatures which correlate with NK cell-sensitivity (161). Noteworthy, this form of therapeutic sensitivity mapping may guide future biomarker-driven treatment algorithms.
4.1 Loss-of-function screens in primary immune cells
Beginning in 2018, CRISPR screening strategies, which sought to directly interrogate different subsets of primary immune cells, started to emerge (Figure 4). Previously, technical hurdles in delivering the high-molecular weight Cas9 protein had hampered the efforts of performing such studies in hard-to-transduce primary cells. For this reason, researchers have in many cases leveraged transgenic murine models with constitutive Cas9 expression, circumventing the need to deliver large bacterial endonucleases into primary human immune cells (169–175). Notably, these works have been able to decipher fundamental aspects of immune cell regulation in an unbiased and massively parallel large-scale fashion, complementing decades of research on immunoregulatory pathways governing T cell function.
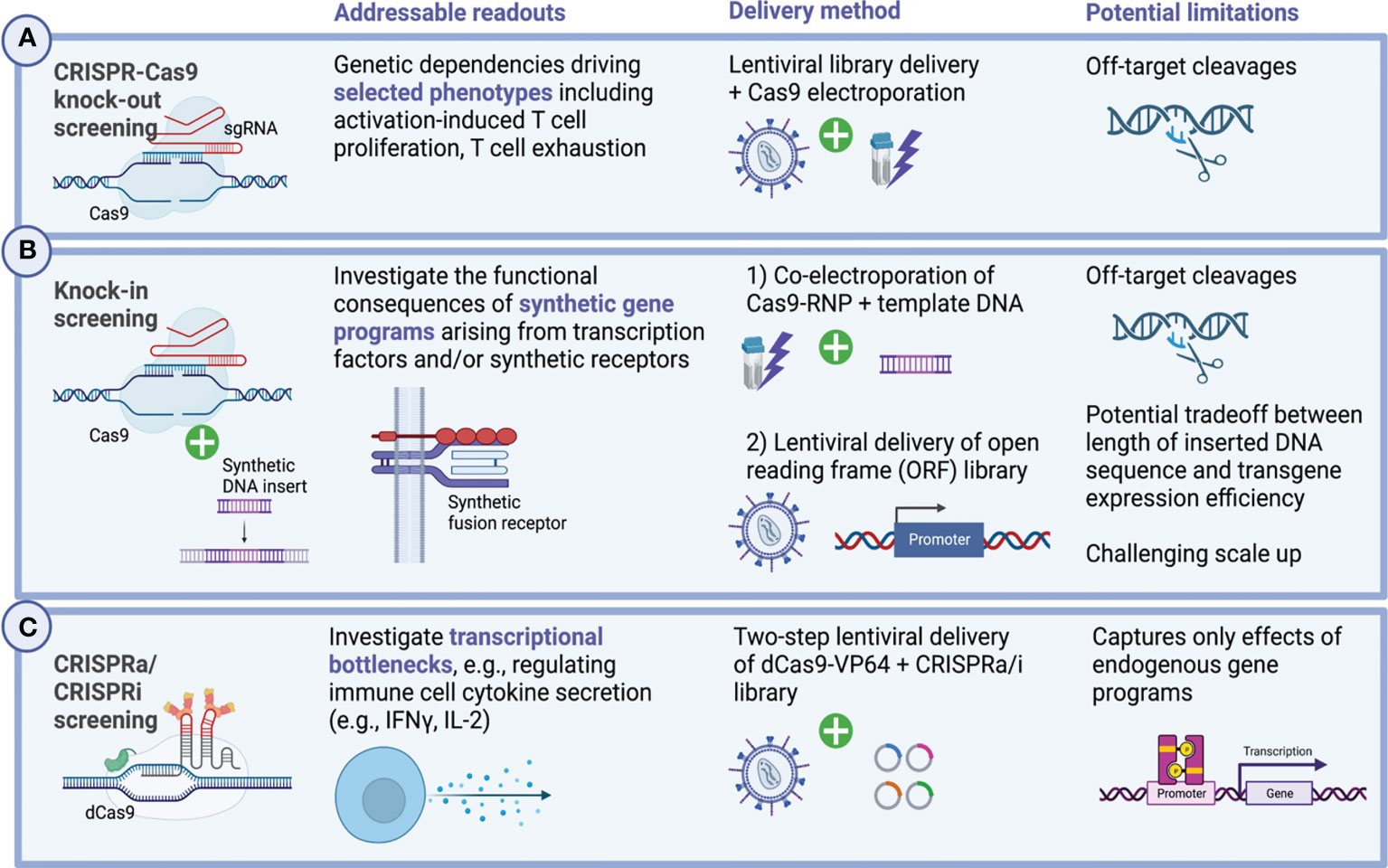
Figure 4 Genome-wide CRISPR screening in primary human immune cells. Forward genetic screening enables the unbiased discovery of genetic dependencies governing cellular functions. Applied to the context of primary immune cells, including genetically engineered immune effector cells, these large-scale discovery approaches have in recent years contributed greatly to develop a deeper understanding of the molecular mechanisms regulating activation-induced T cell proliferation, cytokine secretion and functional exhaustion. Technically, a set of different design approaches exist and enable to address distinct biological questions and confer relationships between genotype and phenotype. (A) Pooled CRISPR/Cas9 knock-out screens allow to interrogate in a massively parallel manner the functional consequences of genetic deletions at genome-scale. In primary human T cells as well as CAR-T cells, lentiviral gRNA library delivery is typically followed by transient Cas9 delivery via electroporation (162–165). Multiple different readouts have previously been investigated including activation-induced T cell proliferation and cytokine secretion as well as T cell persistence and exhaustion. More sophisticated assays combine these efforts with additional selective pressures, for instance, by challenging T cells with immunosuppressive metabolites such as adenosine to select for biologically relevant phenotypes which might inform the design of the next generation of cellular therapies (162–165). (B) Knock-in screens allow the unbiased investigation of novel synthetic gene programs and have lately been translated to primary human T cells in which context they were able to elucidate synthetic fusions receptors which render T cells resistant to immunosuppressive cues from the TME (55, 166, 167). Delivering gene inserts can be accomplished using different strategies, the most recent of which can deliver large transgenes up to multiple kb in size at scale (108, 109). (C) Transcriptional remodeling using CRISPRa and CRISPRi-mediated has successfully been applied to primary human T cells and helped elucidate genetic mechanisms orchestrating cytokine secretion of CD4+ and CD8+ T cells (168). Created with BioRender.com.
Genome-wide CRISPR knockout screens in primary human T cells were first reported in 2018, when two groups pioneered a hybrid gene editing approach combining lentiviral sgRNA delivery with transient Cas9 transfection via electroporation (162, 163) (Figure 4A). In these studies, the authors were able to identify known and unknown genetic mechanisms governing activation-induced proliferation of primary human CD4+ and CD8+ T cells (162) as well as hematopoietic stem and progenitor cell (HSPC) expansion (163). Building on the same platform, the researchers were furthermore able to assess the ramifications of a set of different immunosuppressive selective pressures on immune effector cells (including adenosine, TGF-β, cyclosporine, tacrolimus) to identify potential routes to eventually overcome them (162, 164).
4.1.1 High-content CRISPR readouts
Another aspect, which has greatly improved the biological relevance of these studies is driven by the advent of high-content CRISPR readouts. By relying on, for instance, marker-based sorting to differentiate distinct cellular phenotypes or by integrating other high-dimensional assays including single cell RNA sequencing, these next-generation CRISPR screens provide more depth compared to traditional assays which primarily addressed resistance or sensitivity to a certain external selective pressures (176).
One prominent example comes from work done by the group of Jeremy Rich, who investigated genetic determinants of exhaustion, as defined by surface expression of PD-1, in EGFRvIII-directed CAR-T cells when challenged with glioblastoma stem cells (GSCs) (165). In their seminal work, they used cell sorting to assess guide enrichment in PD-1+ CAR-T compartment and found crucial regulators of CAR-mediated glioblastoma killing. RNA sequencing of GSC-challenged CAR-T cells, which had been specifically edited based on previously identified hits, revealed distinct transcriptomic signatures characterized by upregulation of T cell activation markers and proinflammatory cytokines.
The integration of CRISPR screening and single cell RNA sequencing, hence, powerfully illustrates the potential of high-dimensional studies to capture the complex regulatory mechanisms which determine immune cell fates and anti-tumor immunity (162, 165). In light of the recent report of the first genome-scale mapping of transcriptional effects of genetic perturbations (Perturb-seq) (177), going forward, the relevance of these information-rich assays is likely going to further increase and will help inform the rational design of next-generation engineered cell products.
4.2 Knock-in screening
While targeted genetic ablation has the potential to disrupt pathways which restrain immune cell activation or contribute to exhaustion, knock-in of large gene cassettes, may hold even greater potential to reprogram immune cells for heightened effector functions or exhaustion resistance (32). As has recently been demonstrated, forward genetic screening can be deployed to systematically investigate the transcriptional and functional ramifications arising from the introduction of synthetic gene programs in pooled gain-of-function screens in primary human T cells (55, 166, 167) (Figure 4B). Two main technical platforms for gain-of-function screening have been described relying on either i) non-viral delivery of promoterless double-stranded DNA templates to a specific locus via co-electroporation with CRISPR/Cas9 ribonucleoproteins (RNPs) for subsequent HDR-mediated integration (55) or ii) lentiviral delivery of a barcoded open reading frame (ORF) library (166). Of note, these platforms allow to systematically interrogate the functional consequences of the integration of completely novel gene programs including synthetic receptors which physiologically might not be present in the targeted immune cells, a key difference compared to CRISPR activation (CRISPRa)-based screens, which focus on activation of endogenous genes (168, 178–180). For instance, insertion of lymphotoxin-β receptor (LTBR), typically expressed in myeloid cells, into unmodified as well as CAR-engineered T cells, led to exhaustion resistance and improved effector functions by formation of an autocrine positive feedback loop, as a recent genome-scale pooled knock-in screen selecting for highly-dividing primary T cells revealed (166). Similarly, an in vivo pooled knock-in screen which assessed the relative genotype abundance of 36 synthetic receptors, transcription factors and metabolic regulators among melanoma-infiltrating lymphocytes (TILs), found that introduction of a novel chimeric TGF-β-R2-41BB receptor endowed T cells with the capacity to resist immunosuppressive signaling from TGF-β leading to enhanced melanoma clearance in vivo (55). As the field evolves, both the breadth of screenable gene products has increased and the associated sequencing efforts have been streamlined using modular screening strategies (167). These modular platforms also enable combinatorial screening, for instance, to investigate the synergies from the combined integrations of different transcription factors (167).
Collectively, these examples demonstrate the power of CRISPR-based unbiased discovery tools to identify novel gene programs, which, when introduced into engineered lymphocytes might augment cellular fitness and anti-tumor potency.
4.3 Tuning transcriptional networks for enhanced immune cell function
Today, CRISPR-mediated genome editing allows for the targeted ablation, rewriting and introduction of entirely new segments of DNA (105–107, 110–113, 181). Specific modifications using a catalytically dead form of Cas9 (dCas9) fused with transcriptional activator (CRISPR activation – CRISPRa) or repressor molecules (CRISPR interference – CRISPRi) furthermore allow the targeted epigenetic modulation of gene expression (110–113, 123, 124) (Figure 3). Both CRISPRi and CRISPRa have been used to screen for transcriptional networks regulating cellular function at genome-scale (177, 180, 181) to decipher resistance mechanisms involved in dampening anti-tumor immunity (180). Recent efforts point to the potential application of multiplexed CRISPRa technology as a tumor vaccine by upregulating TAAs for enhanced T cell anti-tumor immunity (179).
In primary human T cells, reciprocal genome-wide CRISPRi/CRISPRa screening has been used to expose transcriptional bottlenecks which restrict T cell activation. Paired with scRNAseq (Perturb-seq), the study further enabled deep molecular characterization of the identified therapeutically relevant T cell states which may prove instrumental when designing novel T cell-based immunotherapies (168) (Figure 4C). In another recent study, CRISPRa gain-of-function screening was applied to CAR-modified murine T cells and was able to demarcate proline metabolism as a pivotal driver of CAR-T cellular fitness and function (178).
Of note, the CRISPRa toolkit is also being leveraged by adjacent research fields, for instance, to revisit fundamental questions of tumor biology, such as whether specific mutations implicated in tumor resistance have been acquired through clonal evolution or were pre-existing. Such questions are now possible to answer using CRISPRa-inducible reporters for live clonal retrieval (182).
4.3.1 Transcriptome editing using CRISPR-Cas13 proteins
Whereas CRISPRi and CRISPRa rely on the stable introduction of transcriptional activators and suppressors to modulate gene expression, Cas13 allows for direct transcriptome engineering via RNA editing without the requirement for permanent genetic manipulations (183–186) (Figure 3). CRISPR-Cas13-mediated transcriptome engineering now allows for both targeted RNA knockdown as well as the rewriting of RNA transcripts, e.g., to correct for pathogenic mutations (187). Of note, Cas13-based approaches may suffer from some of the same drawbacks and limitations as Cas9 including potential off-target editing (188) and host rejection due to pre-existing immunogenicity (189). Nevertheless, this emerging technology represents a promising toolkit for transcriptome engineering which is potentially safer than CRISPR-Cas9-mediated approaches as it can induce defined cellular states without introducing genomic alterations. Recent innovations further yielded chemically modified forms of Cas13, which were able to stably modulate gene expression in CD4 and CD8 T cells (190).
The potential implications of these next-generation CRISPR tools are vast and aim to i) better understand the basic biology of anti-tumor immunity and ii) to build better and more powerful cellular therapeutics. Moreover, while a number of potential candidate genes for augmented T cell immunotherapy have been proposed, it will be critical to see how robustly pre-clinical validation efforts of identified hits can predict the successful transition into clinical development programs.
5 In vivo gene editing and cell engineering strategies
In its latest iteration, precision genome engineering has been adapted for cell-type specific in vivo genetic editing to treat various human diseases including cancer. In a report from 2021, CRISPR/Cas9-mediated in vivo deletion of transthyretin (TTR), which is mutated in progressively fatal transthyretin amyloidosis, led to a striking decrease of circulating transthyretin protein levels in the peripheral blood of treated patients (191). Using an apolipoprotein-E (ApoE)-recruiting lipid nanoparticle (LNP) loaded with Cas9-encoding mRNA and a TTR-directed sgRNA, this platform allows to specifically correct this pathogenic variant in hepatocytes (192). Similarly, pre-clinical efforts have resulted in the in vivo generation of fibroblast activation protein (FAP)-directed CAR-T cells to treat cardiac injury, by selectively reprogramming T cells using a CD5-targeted LNP system (193). Besides LNP-mediated strategies, implantable bioinstructive scaffolds have been developed, which can generate CAR-T cells in vivo and, hence, circumvent the cost-intensive and lengthy vein-to-vein times required for ex vivo manufacturing of autologous CAR-T products (194).
Most recently, nuclease-inactive genome editing strategies have also transitioned to in vivo applications to correct pathogenic mutations in diseases like Hutchinson–Gilford progeria syndrome (195) or familial hypercholesterinemia using size-optimized adeno-associated viruses (AAV) as delivery vehicles (196).
These developments may have far-reaching implications, as they promise to dramatically reduce the time and cost of manufacturing cell products, which could ultimately increase patient access. Furthermore, the immense adaptability of precision genome editing strategies for designing and delivering DNA sequences to correct pathogenic allele variants make it likely that these powerful tools will play an increasingly important role in the treatment of many of the most devastating human diseases.
6 Ensuring patient safety and navigating regulatory challenges
Despite the well-founded optimism, safety concerns arising from various engineering and genome editing strategies remain a preeminent concern, particularly when novel multiplex modified cellular therapeutics transition into the clinic. Recently, temporary halts of ongoing clinical trials investigating gene-edited cell products illustrated the delicate regulatory trade-off to find the right balance between granting patients access to innovative treatments while ensuring utmost patient safety.
Safety concerns may arise both from the vector system employed to deliver genetic cargo or from the induced genetic alterations, themselves. While overall vector safety has greatly improved over the last decades, nuclease-based genome editing strategies continue to carry the risk of inadvertent off-target cleavages. Off-target toxicities of CRISPR/Cas9 editing include mutational events or chromosomal rearrangements that may appear despite the first 20 nucleotide sequence of the sgRNA directing the efficiency of Cas9. Inadvertent off-target cleavage may lead to the deleterious loss of tumor suppressor genes (197). One such example of off-target mutations is the integration of DNA mismatches in the PAM-distal portion of the sgRNA sequence (134, 135, 198–200). Multiple unbiased sequencing platforms exist today and allow to detect these undesired genomic edits (201–203) and assess potential associated risks to decide whether a particular cell product can be safely infused. Global and sensitive detection of DSBs introduced by CRISPR RNA-guided nucleases is enabled through Genome-Wide, Unbiased Identification of DSBs enabled by Sequencing (GUIDE-seq) (201), Circularization for in vitro Reporting of Cleavage Effects by Sequencing (CIRCLE-seq) (202), or RNase H-dependent PCR Amplification Sequencing (rhamp-Seq) (203), which all function as assays to assess off-target cleavage. Approaches to diminish or prevent off-target effects of CRISPR/Cas9 editing include (i) identifying unique target sites without homology to other genomic regions, (ii) modifying the sgRNA by distal truncation of the 3’ end (204), (iii) substitution of regular Cas9 with high fidelity Cas9 (HiFi) or alternative nucleases such as Cas12 (205), (iv) lowering the concentration and optimizing the ratio of Cas9-sgRNA given to the cells (206), or (v) fusing dCas9 with Fokl nuclease (fCas9) to allow for higher sequence targeting specificity (207, 208). Alternatively, nuclease-inactive genome engineering including base editing and PRIME editing present potentially safer alternatives as they avoid DNA double-strand breaks and may well evolve to play a more important role for genome-edited cell products for clinical use going forward.
Today, clinical development programs aiming to translate promising cellular candidates into the clinic are in ever closer contact with regulatory bodies to jointly sketch a safe path forward for highly innovative products to enter the clinic. As the field matures, the most relevant learnings have recently been formalized in Food and Drug Administration (FDA)-issued guidance documents. One important document is focused on Considerations for the Development of Chimeric Antigen Receptor (CAR) Cell Products (209) and another important document is geared towards Human Gene Therapy Products Incorporating Human Genome Editing (210).
Within the framework of these resources, the FDA has issued recommendations regarding the chemistry, manufacturing, and control (CMC), as well as toxicology, pharmacology, and clinical study design involving CAR engineered immune effector cell therapies (209). A prominent guidance involves the choice of vector for gene delivery. Vectors integrating into cellular DNA (e.g., retroviral-based vectors or transposons) may increase the risk of delayed adverse events, including potentially by insertional mutagenesis, and this may require long-term follow-up. Such occurrences would be unexpected in non-integrating vectors, and thus such long-term follow-up for these products may not be warranted. The FDA advises that the necessity of each additional functional domain be justified in application reports, with a description of how these elements may affect CAR specificity, functionality, safety, and immunogenicity. Batch-based manufacturing and process optimization are recommended. If viral vectors are utilized, transduction reliability with batch-to-batch consistent viral multiplicity of infection (MOI) is critical. Aseptic technique should be carried out under current good manufacturing process (CGMP) conditions. Guidance also covers the manufacture and delivery of CAR products, with allowance decreed to both fresh and cryopreserved products depending on the desired shelf-life and quality of the products. Cryopreserved cell products may be more practical for delivery of the cells to different clinical sites, provided that controlled thawing procedures are carefully outlined, potential transport plans are established, and assurance regarding the safety of cryoprotectants has been assessed. Regarding study design, sponsors are recommended to arrange a priori analyses for all subjects, as well as for patients stratified by the receipt of bridging therapy or not. A plan for stopping rules from dose-limiting toxicities, adverse effect monitoring and attribution, and follow-up must be described.
The FDA has outlined separate guidelines for human gene therapy products incorporating human genome editing, encompassing CRISPR-engineered cell products (210). They elaborate on different genome editing tools, delivery methods, and conditions (e.g., ex vivo, in vivo), with stipulated goals to stabilize components, uphold aseptic processing, and minimize off-target genome alterations. The IND should contain documentation regarding genome editing efficiency and specificity. With appropriate controls, orthogonal and redundant models are recommended that incorporate in silico, biochemical, and cellular-based assays, for unbiased genome-wide analysis to detect potential off-target sites. Safety analyses should include assessments of the immunogenicity of genome editing components, viability assessment, evaluation for any residual gene editing components, and testing for selective survival advantage of edited cells, including dysregulated growth or clonal proliferation. It is essential to evaluate genomic integrity, including deletions or insertions, chromosomal rearrangements, exogenous DNA integration, and potential insertional mutagenesis or oncogenicity.
7 Concluding remarks and future directions
The intersection of multiplexed engineering, precision genome editing, and cellular immunotherapy has brought about an unprecedented wave of innovations which has over the last couple of years dramatically expanded the available treatment modalities to combat cancer and other devastating diseases. Iterative cycles of innovation have extended the scope of genome editing strategies and novel delivery methods have helped enable the design of multiplexed-engineered cell products, redrawing the treatment landscape in oncology and beyond. Nevertheless, important challenges remain, and the field is actively exploring opportunities to overcome them.
First, there is an imperative to better understand the mechanisms of therapeutic resistance and relapse following cellular immunotherapy. Devising strategies to overcome tumor immune escape and prevent functional exhaustion of infused immune cells while mitigating inadvertent toxicities are just two examples of how improved cellular therapeutics may evolve to become more powerful with time. As outlined, forward genetic screening approaches paired with information-rich multi-omic analyses may critically inform these novel strategies by uncovering the biological mechanisms which orchestrate the complex interplay between immune cells and tumors. Multiplexed gene engineering may, in turn, build on these learnings to enable the design of more powerful cell products which can produce more durable and potent anti-tumor immune responses.
Sketching a path forward for cellular immunotherapy to effectively combat solid tumors indisputably represents one of the most formidable challenges, the field seeks to address, and numerous highly differentiated strategies are being pursued to accomplish this goal. Besides tackling the challenges of shared antigen expression (35–37, 211), downregulation of MHC molecules (212–214) and impaired trafficking (39), the field is continuously branching out, for instance, towards aiming to restore the immunogenicity of immunologically ‘cold’ tumors, via various approached including epigenetic modulation (215, 216), tumor vaccination strategies (179) or by incorporating bacterial virulence factors to attract bystander immune cells (217). Researchers have also investigated the possibility of delivering CAR targets to solid tumors via oncolytic viruses, in the hope to render the transformed cells targetable using established CAR-T cell products (218). Furthermore, recent work has also pointed to other immune cell subsets for CAR modification (1, 219–223), including CAR-modified macrophages (CAR-M), which may provide unique advantages to better penetrate solid tumor capsules (224). Following the recent report of in vivo edited hepatocytes (191), future studies may also aim to reprogram the tumor cells directly in vivo. This approach might be leveraged to restore treatment sensitivity by augmenting the release of immune cell-attracting chemokines (99, 225) or by forcing expression of TAAs for enhanced immunogenicity (179). Other strategies might aim to selectively induce cell death, for instance, by targeted disruption of oncogenes or hybrid pharmaco-genetic interventions to suppress synthetic lethal gene interactions.
Lastly, a growing number of stakeholders in the field are aiming to expand the scope of eligible patients who can benefit from the tremendous advancements of cellular immunotherapy and precision genome engineering. The transition towards allogeneic off-the-shelf cell products (16, 31, 73, 226, 227) and in vivo-manufactured cell products (193, 194) is already in full swing. Bi- and multi-specific engager molecules are being explored to endow immune cells with CAR-like anti-tumor specificity (228–237), bypassing the complexities associated with cellular engineering entirely. Combinations of both approaches in the form of BiTE-secreting CAR-T cells epitomize the highly modular nature of next generation cellular immunotherapy (238).
Going forward, we expect that these and other emerging technologies will further advance and deepen our understanding of cancer biology and help engineer better, more potent and safer immunotherapies to combat various human cancers.
Author contributions
AB and MD conceived the outline for this manuscript. AB, GM, and MD wrote the manuscript and created figures. All authors contributed to the article and approved the submitted version.
Funding
AB received support from the German Research Foundation as Walter Benjamin Postdoctoral Fellow (464778766). GM receives support from the Radiological Society of North America (RSNA) and the American Society for Radiation Oncology (ASTRO). The project described was supported by RSNA Research & Education Foundation, through the Canon Medical Systems, USA/RSNA Research Resident Grant. The content is solely the responsibility of the authors and does not necessarily represent the official views of the RSNA R&E Foundation. MD acknowledges support from the Society for Immunotherapy of Cancer (SITC), The Leukemia SPORE developmental research program award, the Andrew Sabin Family Foundation and the MD Anderson AML and B-cell lymphoma moonshot programs.
Conflict of interest
MD and The University of Texas MD Anderson Cancer Center have an institutional financial conflict of interest with Takeda Pharmaceutical for the licensing of the CAR-NK cell technology.
The remaining authors declare that the research was conducted in the absence of any commercial or financial relationships that could be construed as a potential conflict of interest.
Publisher’s note
All claims expressed in this article are solely those of the authors and do not necessarily represent those of their affiliated organizations, or those of the publisher, the editors and the reviewers. Any product that may be evaluated in this article, or claim that may be made by its manufacturer, is not guaranteed or endorsed by the publisher.
References
1. Daher M, Rezvani K. Outlook for new car-based therapies with a focus on car nk cells: What lies beyond car-engineered T cells in the race against cancer. Cancer Discov (2021) 11(1):45–58. doi: 10.1158/2159-8290.Cd-20-0556
2. Maude SL, Laetsch TW, Buechner J, Rives S, Boyer M, Bittencourt H, et al. Tisagenlecleucel in children and young adults with b-cell lymphoblastic leukemia. N Engl J Med (2018) 378(5):439–48. doi: 10.1056/NEJMoa1709866
3. Schuster SJ, Bishop MR, Tam CS, Waller EK, Borchmann P, McGuirk JP, et al. Tisagenlecleucel in adult relapsed or refractory diffuse Large b-cell lymphoma. N Engl J Med (2019) 380(1):45–56. doi: 10.1056/NEJMoa1804980
4. Park JH, Riviere I, Gonen M, Wang X, Senechal B, Curran KJ, et al. Long-term follow-up of Cd19 car therapy in acute lymphoblastic leukemia. N Engl J Med (2018) 378(5):449–59. doi: 10.1056/NEJMoa1709919
5. June CH, O’Connor RS, Kawalekar OU, Ghassemi S, Milone MC. Car T cell immunotherapy for human cancer. Science (2018) 359(6382):1361–5. doi: 10.1126/science.aar6711
6. Wang M, Munoz J, Goy A, Locke FL, Jacobson CA, Hill BT, et al. Kte-X19 car T-cell therapy in relapsed or refractory mantle-cell lymphoma. N Engl J Med (2020) 382(14):1331–42. doi: 10.1056/NEJMoa1914347
7. Munshi NC, Anderson LD, Shah N, Madduri D, Berdeja J, Lonial S, et al. Idecabtagene vicleucel in relapsed and refractory multiple myeloma. N Engl J Med (2021) 384(8):705–16. doi: 10.1056/NEJMoa2024850
8. Raje N, Berdeja J, Lin Y, Siegel D, Jagannath S, Madduri D, et al. Anti-bcma car T-cell therapy Bb2121 in relapsed or refractory multiple myeloma. N Engl J Med (2019) 380(18):1726–37. doi: 10.1056/NEJMoa1817226
9. Marofi F, Motavalli R, Safonov VA, Thangavelu L, Yumashev AV, Alexander M, et al. Car T cells in solid tumors: Challenges and opportunities. Stem Cell Res Ther (2021) 12(1):81. doi: 10.1186/s13287-020-02128-1
10. Rafei H, Daher M, Rezvani K. Chimeric antigen receptor (Car) natural killer (Nk)-cell therapy: Leveraging the power of innate immunity. Br J Haematol (2021) 193(2):216–30. doi: 10.1111/bjh.17186
11. Biederstadt A, Rezvani K. Engineering the next generation of car-nk immunotherapies. Int J Hematol (2021) 114(5):554–71. doi: 10.1007/s12185-021-03209-4
12. Weinkove R, George P, Dasyam N, McLellan AD. Selecting costimulatory domains for chimeric antigen receptors: Functional and clinical considerations. Clin Transl Immunol (2019) 8(5):e1049. doi: 10.1002/cti2.1049
13. Bell M, Gottschalk S. Engineered cytokine signaling to improve car T cell effector function. Front Immunol (2021) 12:684642. doi: 10.3389/fimmu.2021.684642
14. Grosser R, Cherkassky L, Chintala N, Adusumilli PS. Combination immunotherapy with car T cells and checkpoint blockade for the treatment of solid tumors. Cancer Cell (2019) 36(5):471–82. doi: 10.1016/j.ccell.2019.09.006
15. Chapuis AG, Egan DN, Bar M, Schmitt TM, McAfee MS, Paulson KG, et al. T Cell receptor gene therapy targeting Wt1 prevents acute myeloid leukemia relapse post-transplant. Nat Med (2019) 25(7):1064–72. doi: 10.1038/s41591-019-0472-9
16. Liu E, Marin D, Banerjee P, Macapinlac HA, Thompson P, Basar R, et al. Use of car-transduced natural killer cells in Cd19-positive lymphoid tumors. New Engl J Med (2020) 382(6):545–53. doi: 10.1056/NEJMoa1910607
17. Neelapu SS, Locke FL, Bartlett NL, Lekakis LJ, Miklos DB, Jacobson CA, et al. Axicabtagene ciloleucel car T-cell therapy in refractory Large b-cell lymphoma. N Engl J Med (2017) 377(26):2531–44. doi: 10.1056/NEJMoa1707447
18. Upadhyay R, Boiarsky JA, Pantsulaia G, Svensson-Arvelund J, Lin MJ, Wroblewska A, et al. A critical role for fas-mediated off-target tumor killing in T-cell immunotherapy. Cancer Discov (2021) 11(3):599–613. doi: 10.1158/2159-8290.Cd-20-0756
19. Singh N, Lee YG, Shestova O, Ravikumar P, Hayer KE, Hong SJ, et al. Impaired death receptor signaling in leukemia causes antigen-independent resistance by inducing car T-cell dysfunction. Cancer Discov (2020) 10(4):552–67. doi: 10.1158/2159-8290.Cd-19-0813
20. Boulch M, Cazaux M, Loe-Mie Y, Thibaut R, Corre B, Lemaître F, et al. A cross-talk between car T cell subsets and the tumor microenvironment is essential for sustained cytotoxic activity. Sci Immunol (2021) 6(57):eabd4344. doi: 10.1126/sciimmunol.abd4344
21. Sheih A, Voillet V, Hanafi L-A, DeBerg HA, Yajima M, Hawkins R, et al. Clonal kinetics and single-cell transcriptional profiling of car-T cells in patients undergoing Cd19 car-T immunotherapy. Nat Commun (2020) 11(1):219. doi: 10.1038/s41467-019-13880-1
22. Melenhorst JJ, Chen GM, Wang M, Porter DL, Chen C, Collins MA, et al. Decade-long leukaemia remissions with persistence of Cd4+ car T cells. Nature (2022) 602(7897):503–9. doi: 10.1038/s41586-021-04390-6
23. Bai Z, Woodhouse S, Zhao Z, Arya R, Govek K, Kim D, et al. Single-cell antigen-specific landscape of car T infusion product identifies determinants of Cd19-positive relapse in patients with all. Sci Adv (2022) 8(23):eabj2820. doi: 10.1126/sciadv.abj2820
24. Bai Z, Lundh S, Kim D, Woodhouse S, Barrett DM, Myers RM, et al. Single-cell multiomics dissection of basal and antigen-specific activation states of Cd19-targeted car T cells. J ImmunoTher Cancer (2021) 9(5):e002328. doi: 10.1136/jitc-2020-002328
26. Abdel-Hakeem MS, Manne S, Beltra J-C, Stelekati E, Chen Z, Nzingha K, et al. Epigenetic scarring of exhausted T cells hinders memory differentiation upon eliminating chronic antigenic stimulation. Nat Immunol (2021) 22(8):1008–19. doi: 10.1038/s41590-021-00975-5
27. Wherry EJ, Kurachi M. Molecular and cellular insights into T cell exhaustion. Nat Rev Immunol (2015) 15(8):486–99. doi: 10.1038/nri3862
28. Belk JA, Daniel B, Satpathy AT. Epigenetic regulation of T cell exhaustion. Nat Immunol (2022) 23(6):848–60. doi: 10.1038/s41590-022-01224-z
29. Sen DR, Kaminski J, Barnitz RA, Kurachi M, Gerdemann U, Yates KB, et al. The epigenetic landscape of T cell exhaustion. Science (2016) 354(6316):1165–9. doi: 10.1126/science.aae0491
30. Yates KB, Tonnerre P, Martin GE, Gerdemann U, Al Abosy R, Comstock DE, et al. Epigenetic scars of Cd8+ T cell exhaustion persist after cure of chronic infection in humans. Nat Immunol (2021) 22(8):1020–9. doi: 10.1038/s41590-021-00979-1
31. Liu E, Tong Y, Dotti G, Shaim H, Savoldo B, Mukherjee M, et al. Cord blood nk cells engineered to express il-15 and a Cd19-targeted car show long-term persistence and potent antitumor activity. Leukemia (2018) 32(2):520–31. doi: 10.1038/leu.2017.226
32. Lynn RC, Weber EW, Sotillo E, Gennert D, Xu P, Good Z, et al. C-jun overexpression in car T cells induces exhaustion resistance. Nature (2019) 576(7786):293–300. doi: 10.1038/s41586-019-1805-z
33. Science AM, Technology Co. L, Hospital BC. Study of anti-Cd19/Cd22 car nk cells in relapsed and refractory b cell lymphoma. (2019).
34. Shalabi H, Qin H, Su A, Yates B, Wolters PL, Steinberg SM, et al. Cd19/22 car T cells in children and young adults with b-all: Phase 1 results and development of a novel bicistronic car. Blood (2022) 140(5):451–63. doi: 10.1182/blood.2022015795
35. Srivastava S, Salter AI, Liggitt D, Yechan-Gunja S, Sarvothama M, Cooper K, et al. Logic-gated Ror1 chimeric antigen receptor expression rescues T cell-mediated toxicity to normal tissues and enables selective tumor targeting. Cancer Cell (2019) 35(3):489–503 e8. doi: 10.1016/j.ccell.2019.02.003
36. Gonzalez A, Roguev A, Frankel NW, Garrison BS, Lee D, Gainer M, et al. Abstract Lb028: Development of logic-gated car-nk cells to reduce target-mediated healthy tissue toxicities. Cancer Res (2021) 81(13 Supplement):LB028. doi: 10.1158/1538-7445.AM2021-LB028
37. Haubner S, Mansilla-Soto J, Nataraj S, He X, Park JH, Wang X, et al. “If-better” gating: Combinatorial targeting and synergistic signaling for enhanced car T cell efficacy. Blood (2021) 138(Supplement 1):2774–. doi: 10.1182/blood-2021-149263
38. Kremer V, Ligtenberg MA, Zendehdel R, Seitz C, Duivenvoorden A, Wennerberg E, et al. Genetic engineering of human nk cells to express Cxcr2 improves migration to renal cell carcinoma. J ImmunoTher Cancer (2017) 5(1):73. doi: 10.1186/s40425-017-0275-9
39. Müller N, Michen S, Tietze S, Töpfer K, Schulte A, Lamszus K, et al. Engineering nk cells modified with an egfrviii-specific chimeric antigen receptor to overexpress Cxcr4 improves immunotherapy of Cxcl12/Sdf-1α-Secreting glioblastoma. J Immunother (Hagerstown Md: 1997) (2015) 38(5):197. doi: 10.1097/CJI.0000000000000082
40. Beane JD, Lee G, Zheng Z, Mendel M, Abate-Daga D, Bharathan M, et al. Clinical scale zinc finger nuclease-mediated gene editing of pd-1 in tumor infiltrating lymphocytes for the treatment of metastatic melanoma. Mol Ther (2015) 23(8):1380–90. doi: 10.1038/mt.2015.71
41. Wang Z, Li N, Feng K, Chen M, Zhang Y, Liu Y, et al. Phase I study of car-T cells with pd-1 and tcr disruption in mesothelin-positive solid tumors. Cell Mol Immunol (2021) 18(9):2188–98. doi: 10.1038/s41423-021-00749-x
42. Rafiq S, Yeku OO, Jackson HJ, Purdon TJ, van Leeuwen DG, Drakes DJ, et al. Targeted delivery of a pd-1-Blocking scfv by car-T cells enhances anti-tumor efficacy in vivo. Nat Biotechnol (2018) 36(9):847–56. doi: 10.1038/nbt.4195
43. Ren J, Zhang X, Liu X, Fang C, Jiang S, June CH, et al. A versatile system for rapid multiplex genome-edited car T cell generation. Oncotarget (2017) 8(10):17002–11. doi: 10.18632/oncotarget.15218
44. Ren J, Liu X, Fang C, Jiang S, June CH, Zhao Y. Multiplex genome editing to generate universal car T cells resistant to Pd1 inhibition. Clin Cancer Res (2017) 23(9):2255–66. doi: 10.1158/1078-0432.CCR-16-1300
45. Lee YH, Lee HJ, Kim HC, Lee Y, Nam SK, Hupperetz C, et al. Pd-1 and tigit downregulation distinctly affect the effector and early memory phenotypes of Cd19-targeting car T cells. Mol Ther (2022) 30(2):579–92. doi: 10.1016/j.ymthe.2021.10.004
46. Zou F, Lu L, Liu J, Xia B, Zhang W, Hu Q, et al. Engineered triple inhibitory receptor resistance improves anti-tumor car-T cell performance Via Cd56. Nat Commun (2019) 10(1):4109. doi: 10.1038/s41467-019-11893-4
47. Jafarzadeh L, Masoumi E, Mirzaei HR, Alishah K, Fallah-Mehrjardi K, Khakpoor-Koosheh M, et al. Targeted knockdown of Tim3 by short hairpin rnas improves the function of anti-mesothelin car T cells. Mol Immunol (2021) 139:1–9. doi: 10.1016/j.molimm.2021.06.007
48. Zhang Y, Zhang X, Cheng C, Mu W, Liu X, Li N, et al. Crispr-Cas9 mediated lag-3 disruption in car-T cells. Front Med (2017) 11(4):554–62. doi: 10.1007/s11684-017-0543-6
49. Delconte RB, Kolesnik TB, Dagley LF, Rautela J, Shi W, Putz EM, et al. Cis is a potent checkpoint in nk cell–mediated tumor immunity. Nat Immunol (2016) 17(7):816–24. doi: 10.1038/ni.3470
50. Daher M, Basar R, Gokdemir E, Baran N, Uprety N, Nunez Cortes AK, et al. Targeting a cytokine checkpoint enhances the fitness of armored cord blood car-nk cells. Blood (2021) 137(5):624–36. doi: 10.1182/blood.2020007748
51. Kamiya T, Seow SV, Wong D, Robinson M, Campana D. Blocking expression of inhibitory receptor Nkg2a overcomes tumor resistance to nk cells. J Clin Invest (2019) 129(5):2094–106. doi: 10.1172/JCI123955
52. Shaim H, Shanley M, Basar R, Daher M, Gumin J, Zamler DB, et al. Targeting the alphav Integrin/Tgf-beta axis improves natural killer cell function against glioblastoma stem cells. J Clin Invest (2021) 131(14):e142116. doi: 10.1172/JCI142116
53. Daher M, Basar R, Shaim H, Gokdemir E, Uprety N, Kontoyiannis A, et al. The tgf-β/Smad signaling pathway as a mediator of nk cell dysfunction and immune evasion in myelodysplastic syndrome. Blood (2017) 130(Supplement 1):53–. doi: 10.1182/blood.V130.Suppl_1.53.53
54. Giuffrida L, Sek K, Henderson MA, Lai J, Chen AXY, Meyran D, et al. Crispr/Cas9 mediated deletion of the adenosine A2a receptor enhances car T cell efficacy. Nat Commun (2021) 12(1):3236. doi: 10.1038/s41467-021-23331-5
55. Roth TL, Li PJ, Blaeschke F, Nies JF, Apathy R, Mowery C, et al. Pooled knockin targeting for genome engineering of cellular immunotherapies. Cell (2020) 181(3):728–44 e21. doi: 10.1016/j.cell.2020.03.039
56. Slattery K, Woods E, Zaiatz-Bittencourt V, Marks S, Chew S, Conroy M, et al. Tgfβ drives nk cell metabolic dysfunction in human metastatic breast cancer. J Immunother Cancer (2021) 9(2):e002044. doi: 10.1136/jitc-2020-002044
57. Dai Z, Mu W, Zhao Y, Cheng J, Lin H, Ouyang K, et al. T Cells expressing Cd5/Cd7 bispecific chimeric antigen receptors with fully human heavy-Chain-Only domains mitigate tumor antigen escape. Signal Transduction Targeted Ther (2022) 7(1):85. doi: 10.1038/s41392-022-00898-z
58. Goodridge JP, Bjordahl R, Mahmood S, Reiser J, Gaidarova S, Blum R, et al. Abstract 1550: Ft576 path to first-of-Kind clinical trial: Translation of a versatile multi-antigen specific off-the-Shelf nk cell for treatment of multiple myeloma. Cancer Res (2021) 81(13 Supplement):1550. doi: 10.1158/1538-7445.AM2021-1550
59. Eyquem J, Mansilla-Soto J, Giavridis T, van der Stegen SJC, Hamieh M, Cunanan KM, et al. Targeting a car to the trac locus with Crispr/Cas9 enhances tumour rejection. Nature (2017) 543(7643):113–7. doi: 10.1038/nature21405
60. Mansilla-Soto J, Eyquem J, Haubner S, Hamieh M, Feucht J, Paillon N, et al. Hla-independent T cell receptors for targeting tumors with low antigen density. Nat Med (2022) 28(2):345–52. doi: 10.1038/s41591-021-01621-1
61. Qasim W, Zhan H, Samarasinghe S, Adams S, Amrolia P, Stafford S, et al. Molecular remission of infant b-all after infusion of universal talen gene-edited car T cells. Sci Trans Med (2017) 9(374):eaaj2013. doi: 10.1126/scitranslmed.aaj2013
62. Basar R, Uprety N, Ensley E, Daher M, Klein K, Martinez F, et al. Generation of glucocorticoid resistant sars-Cov-2 T-cells for adoptive cell therapy. Cell Rep (2021) 36(3):109432. doi: 10.1016/j.celrep.2021.109432
63. Yeku OO, Purdon TJ, Koneru M, Spriggs D, Brentjens RJ. Armored car T cells enhance antitumor efficacy and overcome the tumor microenvironment. Sci Rep (2017) 7(1):10541. doi: 10.1038/s41598-017-10940-8
64. Pegram H, Purdon T, Van Leeuwen D, Curran K, Giralt S, Barker J, et al. Il-12-Secreting Cd19-targeted cord blood-derived T cells for the immunotherapy of b-cell acute lymphoblastic leukemia. Leukemia (2015) 29(2):415–22. doi: 10.1038/leu.2014.215
65. Kueberuwa G, Kalaitsidou M, Cheadle E, Hawkins RE, Gilham DE. Cd19 car T cells expressing il-12 eradicate lymphoma in fully lymphoreplete mice through induction of host immunity. Mol Therapy-Oncol (2018) 8:41–51. doi: 10.1016/j.omto.2017.12.003
66. Koneru M, Purdon TJ, Spriggs D, Koneru S, Brentjens RJ. Il-12 secreting tumor-targeted chimeric antigen receptor T cells eradicate ovarian tumors in vivo. Oncoimmunology (2015) 4(3):e994446. doi: 10.4161/2162402X.2014.994446
67. Liu Y, Di S, Shi B, Zhang H, Wang Y, Wu X, et al. Armored inducible expression of il-12 enhances antitumor activity of Glypican-3–targeted chimeric antigen receptor–engineered T cells in hepatocellular carcinoma. J Immunol (2019) 203(1):198–207. doi: 10.4049/jimmunol.1800033
68. Chmielewski M, Abken H. Car T cells releasing il-18 convert to T-bethigh Foxo1low effectors that exhibit augmented activity against advanced solid tumors. Cell Rep (2017) 21(11):3205–19. doi: 10.1016/j.celrep.2017.11.063
69. Kunert A, Chmielewski M, Wijers R, Berrevoets C, Abken H, Debets R. Intra-tumoral production of Il18, but not Il12, by tcr-engineered T cells is non-toxic and counteracts immune evasion of solid tumors. Oncoimmunology (2018) 7(1):e1378842. doi: 10.1080/2162402X.2017.1378842
70. Chen Y, Sun C, Landoni E, Metelitsa L, Dotti G, Savoldo B. Eradication of neuroblastoma by T cells redirected with an optimized Gd2-specific chimeric antigen receptor and interleukin-15gd2. car-Ts-Expressing Il15 eradicate neuroblastoma. Clin Cancer Res (2019) 25(9):2915–24. doi: 10.1158/1078-0432.CCR-18-1811
71. Hurton LV, Singh H, Najjar AM, Switzer KC, Mi T, Maiti S, et al. Tethered il-15 augments antitumor activity and promotes a stem-cell memory subset in tumor-specific T cells. Proc Natl Acad Sci (2016) 113(48):E7788–E97. doi: 10.1073/pnas.1610544113
72. Jochems C, Hodge JW, Fantini M, Fujii R, Morillon YM 2nd, Greiner JW, et al. An nk cell line (Hank) expressing high levels of granzyme and engineered to express the high affinity Cd16 allele. Oncotarget (2016) 7(52):86359–73. doi: 10.18632/oncotarget.13411
73. Cichocki F, Goodridge JP, Bjordahl R, Gaidarova S, Mahmood S, Abujarour R, et al. Off-the-Shelf, multiplexed-engineered ipsc-derived nk cells mediate potent multi-antigen targeting of b-cell malignancies with reduced cytotoxicity against healthy b cells. Blood (2021) 138(Supplement 1):407–. doi: 10.1182/blood-2021-148654
74. Romee R, Schneider SE, Leong JW, Chase JM, Keppel CR, Sullivan RP, et al. Cytokine activation induces human memory-like nk cells. Blood (2012) 120(24):4751–60. doi: 10.1182/blood-2012-04-419283
75. Romee R, Rosario M, Berrien-Elliott MM, Wagner JA, Jewell BA, Schappe T, et al. Cytokine-induced memory-like natural killer cells exhibit enhanced responses against myeloid leukemia. Sci Trans Med (2016) 8(357):357ra123. doi: 10.1126/scitranslmed.aaf2341
76. Berrien-Elliott MM, Cashen AF, Cubitt CC, Neal CC, Wong P, Wagner JA, et al. Multidimensional analyses of donor memory-like nk cells reveal new associations with response after adoptive immunotherapy for leukemia. Cancer Discovery (2020) 10(12):1854–71. doi: 10.1158/2159-8290.Cd-20-0312
77. Shapiro RM, Birch GC, Hu G, Vergara Cadavid J, Nikiforow S, Baginska J, et al. Expansion, persistence, and efficacy of donor memory-like nk cells infused for post-transplant relapse. J Clin Invest (2022) 132:e154334. doi: 10.1172/JCI154334
78. Hombach A, Barden M, Hannappel L, Chmielewski M, Rappl G, Sachinidis A, et al. Il12 integrated into the car exodomain converts Cd8+ T cells to poly-functional nk-like cells with superior killing of antigen-loss tumors. Mol Ther (2022) 30(2):593–605. doi: 10.1016/j.ymthe.2021.10.011
79. Spiegel JY, Patel S, Muffly L, Hossain NM, Oak J, Baird JH, et al. Car T cells with dual targeting of Cd19 and Cd22 in adult patients with recurrent or refractory b cell malignancies: A phase 1 trial. Nat Med (2021) 27:1419–31. doi: 10.1038/s41591-021-01436-0
80. Majzner RG, Rietberg SP, Sotillo E, Dong R, Vachharajani VT, Labanieh L, et al. Tuning the antigen density requirement for car T-cell activity. Cancer Discovery (2020) 10(5):702–23. doi: 10.1158/2159-8290.Cd-19-0945
81. Cronk RJ, Zurko J, Shah NN. Bispecific chimeric antigen receptor T cell therapy for b cell malignancies and multiple myeloma. Cancers (2020) 12(9):2523. doi: 10.3390/cancers12092523
82. Hirabayashi K, Du H, Xu Y, Shou P, Zhou X, Fucá G, et al. Dual-targeting car-T cells with optimal Co-stimulation and metabolic fitness enhance antitumor activity and prevent escape in solid tumors. Nat Cancer (2021) 2(9):904–18. doi: 10.1038/s43018-021-00244-2
83. Kloss CC, Condomines M, Cartellieri M, Bachmann M, Sadelain M. Combinatorial antigen recognition with balanced signaling promotes selective tumor eradication by engineered T cells. Nat Biotechnol (2013) 31(1):71–5. doi: 10.1038/nbt.2459
84. Choe JH, Watchmaker PB, Simic MS, Gilbert RD, Li AW, Krasnow NA, et al. Synnotch-car T cells overcome challenges of specificity, heterogeneity, and persistence in treating glioblastoma. Sci Trans Med (2021) 13(591):eabe7378. doi: 10.1126/scitranslmed.abe7378
85. Williams JZ, Allen GM, Shah D, Sterin IS, Kim KH, Garcia VP, et al. Precise T cell recognition programs designed by transcriptionally linking multiple receptors. Science (2020) 370(6520):1099–104. doi: 10.1126/science.abc6270
86. Li Y, Basar R, Wang G, Liu E, Moyes JS, Li L, et al. Kir-based inhibitory cars overcome car-nk cell trogocytosis-mediated fratricide and tumor escape. Nat Med (2022) 28:2133–44. doi: 10.1038/s41591-022-02003-x
87. Adachi K, Kano Y, Nagai T, Okuyama N, Sakoda Y, Tamada K. Il-7 and Ccl19 expression in car-T cells improves immune cell infiltration and car-T cell survival in the tumor. Nat Biotechnol (2018) 36(4):346–51. doi: 10.1038/nbt.4086
88. Jin L, Tao H, Karachi A, Long Y, Hou AY, Na M, et al. Cxcr1-or Cxcr2-modified car T cells Co-opt il-8 for maximal antitumor efficacy in solid tumors. Nat Commun (2019) 10(1):1–13. doi: 10.1038/s41467-019-11869-4
89. Luo H, Su J, Sun R, Sun Y, Wang Y, Dong Y, et al. Coexpression of Il7 and Ccl21 increases efficacy of car-T cells in solid tumors without requiring preconditioned lymphodepletion. Clin Cancer Res (2020) 26(20):5494–505. doi: 10.1158/1078-0432.CCR-20-0777
90. Cadilha BL, Benmebarek M-R, Dorman K, Oner A, Lorenzini T, Obeck H, et al. Combined tumor-directed recruitment and protection from immune suppression enable car T cell efficacy in solid tumors. Sci Adv (2021) 7(24):eabi5781. doi: 10.1126/sciadv.abi5781
91. Craddock JA, Lu A, Bear A, Pule M, Brenner MK, Rooney CM, et al. Enhanced tumor trafficking of Gd2 chimeric antigen receptor T cells by expression of the chemokine receptor Ccr2b. J Immunother (Hagerstown Md: 1997) (2010) 33(8):780. doi: 10.1097/CJI.0b013e3181ee6675
92. Lesch S, Blumenberg V, Stoiber S, Gottschlich A, Ogonek J, Cadilha BL, et al. T Cells armed with c-X-C chemokine receptor type 6 enhance adoptive cell therapy for pancreatic tumours. Nat Biomed Eng (2021) 5(11):1246–60. doi: 10.1038/s41551-021-00737-6
93. Itoh-Nakadai A, Saito Y, Murasawa-Tomizawa M, Kajita H, Matsumoto T, Matsuda M, et al. Cxcr4-expressing anti-Cd25 car T-cells effectively eliminate human aml cells in vivo. Blood (2020) 136:35–6. doi: 10.1182/blood-2020-142228
94. Whilding LM, Halim L, Draper B, Parente-Pereira AC, Zabinski T, Davies DM, et al. Car T-cells targeting the integrin αvβ6 and Co-expressing the chemokine receptor Cxcr2 demonstrate enhanced homing and efficacy against several solid malignancies. Cancers (2019) 11(5):674. doi: 10.3390/cancers11050674
95. Liu G, Rui W, Zheng H, Huang D, Yu F, Zhang Y, et al. Cxcr2-modified car-T cells have enhanced trafficking ability that improves treatment of hepatocellular carcinoma. Eur J Immunol (2020) 50(5):712–24. doi: 10.1002/eji.201948457
96. Peng W, Ye Y, Rabinovich BA, Liu C, Lou Y, Zhang M, et al. Transduction of tumor-specific T cells with Cxcr2 chemokine receptor improves migration to tumor and antitumor immune responses. Clin Cancer Res (2010) 16(22):5458–68. doi: 10.1158/1078-0432.Ccr-10-0712
97. Somanchi SS, Somanchi A, Cooper LJN, Lee DA. Engineering lymph node homing of ex vivo–expanded human natural killer cells Via trogocytosis of the chemokine receptor Ccr7. Blood (2012) 119(22):5164–72. doi: 10.1182/blood-2011-11-389924
98. Carlsten M, Levy E, Karambelkar A, Li L, Reger R, Berg M, et al. Efficient mrna-based genetic engineering of human nk cells with high-affinity Cd16 and Ccr7 augments rituximab-induced adcc against lymphoma and targets nk cell migration toward the lymph node-associated chemokine Ccl19. Front Immunol (2016) 7:105. doi: 10.3389/fimmu.2016.00105
99. Lee J, Kang TH, Yoo W, Choi H, Jo S, Kong K, et al. An antibody designed to improve adoptive nk-cell therapy inhibits pancreatic cancer progression in a murine model. Cancer Immunol Res (2019) 7(2):219. doi: 10.1158/2326-6066.CIR-18-0317
100. Walle T, Kraske JA, Liao B, Lenoir B, Timke C, Halbach E, et al. Radiotherapy orchestrates natural killer cell dependent antitumor immune responses through Cxcl8. Sci Adv (2022) 8(12):eabh4050. doi: 10.1126/sciadv.abh4050
101. Ng YY, Tay JC, Wang S. Cxcr1 expression to improve anti-cancer efficacy of intravenously injected car-nk cells in mice with peritoneal xenografts. Mol Therapy-Oncol (2020) 16:75–85. doi: 10.1016/j.omto.2019.12.006
102. Tebas P, Stein D, Tang WW, Frank I, Wang SQ, Lee G, et al. Gene editing of Ccr5 in autologous Cd4 T cells of persons infected with hiv. N Engl J Med (2014) 370(10):901–10. doi: 10.1056/NEJMoa1300662
103. Stadtmauer EA, Fraietta JA, Davis MM, Cohen AD, Weber KL, Lancaster E, et al. Crispr-engineered T cells in patients with refractory cancer. Science (2020) 367(6481):eaba7365. doi: 10.1126/science.aba7365
104. Yang H, Wang H, Shivalila Chikdu S, Cheng Albert W, Shi L, Jaenisch R. One-step generation of mice carrying reporter and conditional alleles by Crispr/Cas-mediated genome engineering. Cell (2013) 154(6):1370–9. doi: 10.1016/j.cell.2013.08.022
105. Gaudelli NM, Komor AC, Rees HA, Packer MS, Badran AH, Bryson DI, et al. Programmable base editing of a•T to G•C in genomic DNA without DNA cleavage. Nature (2017) 551(7681):464–71. doi: 10.1038/nature24644
106. Komor AC, Kim YB, Packer MS, Zuris JA, Liu DR. Programmable editing of a target base in genomic DNA without double-stranded DNA cleavage. Nature (2016) 533(7603):420–4. doi: 10.1038/nature17946
107. Anzalone AV, Randolph PB, Davis JR, Sousa AA, Koblan LW, Levy JM, et al. Search-and-Replace genome editing without double-strand breaks or donor DNA. Nature (2019) 576(7785):149–57. doi: 10.1038/s41586-019-1711-4
108. Anzalone AV, Gao XD, Podracky CJ, Nelson AT, Koblan LW, Raguram A, et al. Programmable deletion, replacement, integration and inversion of Large DNA sequences with twin prime editing. Nat Biotechnol (2022) 40(5):731–40. doi: 10.1038/s41587-021-01133-w
109. Strecker J, Ladha A, Gardner Z, Schmid-Burgk JL, Makarova KS, Koonin EV, et al. Rna-guided DNA insertion with crispr-associated transposases. Science (2019) 365(6448):48–53. doi: 10.1126/science.aax9181
110. Gilbert LA, Larson MH, Morsut L, Liu Z, Brar GA, Torres SE, et al. Crispr-mediated modular rna-guided regulation of transcription in eukaryotes. Cell (2013) 154(2):442–51. doi: 10.1016/j.cell.2013.06.044
111. Larson MH, Gilbert LA, Wang X, Lim WA, Weissman JS, Qi LS. Crispr interference (Crispri) for sequence-specific control of gene expression. Nat Protoc (2013) 8(11):2180–96. doi: 10.1038/nprot.2013.132
112. Qi LS, Larson MH, Gilbert LA, Doudna JA, Weissman JS, Arkin AP, et al. Repurposing crispr as an rna-guided platform for sequence-specific control of gene expression. Cell (2013) 152(5):1173–83. doi: 10.1016/j.cell.2013.02.022
113. Sander JD, Joung JK. Crispr-cas systems for editing, regulating and targeting genomes. Nat Biotechnol (2014) 32(4):347–55. doi: 10.1038/nbt.2842
114. Nuñez JK, Chen J, Pommier GC, Cogan JZ, Replogle JM, Adriaens C, et al. Genome-wide programmable transcriptional memory by crispr-based epigenome editing. Cell (2021) 184(9):2503–19. doi: 10.1016/j.cell.2021.03.025
115. Cano-Rodriguez D, Gjaltema RAF, Jilderda LJ, Jellema P, Dokter-Fokkens J, Ruiters MHJ, et al. Writing of H3k4me3 overcomes epigenetic silencing in a sustained but context-dependent manner. Nat Commun (2016) 7(1):1–11. doi: 10.1038/ncomms12284
116. Hilton IB, D’ippolito AM, Vockley CM, Thakore PI, Crawford GE, Reddy TE, et al. Epigenome editing by a crispr-Cas9-Based acetyltransferase activates genes from promoters and enhancers. Nat Biotechnol (2015) 33(5):510–7. doi: 10.1038/nbt.3199
117. Kearns NA, Pham H, Tabak B, Genga RM, Silverstein NJ, Garber M, et al. Functional annotation of native enhancers with a Cas9–histone demethylase fusion. Nat Methods (2015) 12(5):401–3. doi: 10.1038/nmeth.3325
118. Choudhury SR, Cui Y, Lubecka K, Stefanska B, Irudayaraj J. Crispr-Dcas9 mediated Tet1 targeting for selective DNA demethylation at Brca1 promoter. Oncotarget (2016) 7(29):46545. doi: 10.18632/oncotarget.10234
119. Xu X, Tao Y, Gao X, Zhang L, Li X, Zou W, et al. A crispr-based approach for targeted DNA demethylation. Cell Discov (2016) 2(1):1–12. doi: 10.1038/celldisc.2016.9
120. Bennett-Baker PE, Mueller JL. Crispr-mediated isolation of specific megabase segments of genomic DNA. Nucleic Acids Res (2017) 45:e165. doi: 10.1093/nar/gkx749
121. Lei Y, Zhang X, Su J, Jeong M, Gundry MC, Huang Y-H, et al. Targeted DNA methylation in vivo using an engineered Dcas9-Mq1 fusion protein. Nat Commun (2017) 8(1):1–10. doi: 10.1038/ncomms16026
122. Vojta A, Dobrinić P, Tadić V, Bočkor L, Korać P, Julg B, et al. Repurposing the crispr-Cas9 system for targeted DNA methylation. Nucleic Acids Res (2016) 44(12):5615–28. doi: 10.1093/nar/gkw159
123. Amabile A, Migliara A, Capasso P, Biffi M, Cittaro D, Naldini L, et al. Inheritable silencing of endogenous genes by hit-and-Run targeted epigenetic editing. Cell (2016) 167(1):219–32. doi: 10.1016/j.cell.2016.09.006
124. Liu XS, Wu H, Ji X, Stelzer Y, Wu X, Czauderna S, et al. Editing DNA methylation in the mammalian genome. Cell (2016) 167(1):233–47.e17. doi: 10.1016/j.cell.2016.08.056
125. He X, Tan C, Wang F, Wang Y, Zhou R, Cui D, et al. Knock-in of Large reporter genes in human cells Via Crispr/Cas9-induced homology-dependent and independent DNA repair. Nucleic Acids Res (2016) 44(9):e85–e. doi: 10.1093/nar/gkw064
126. Tasca F, Brescia M, Wang Q, Liu J, Janssen JM, Szuhai K, et al. Large-Scale genome editing based on high-capacity adenovectors and crispr-Cas9 nucleases rescues full-length dystrophin synthesis in dmd muscle cells. Nucleic Acids Res (2022) 50(13):7761–82. doi: 10.1093/nar/gkac567
127. Ling X, Xie B, Gao X, Chang L, Zheng W, Chen H, et al. Improving the efficiency of precise genome editing with site-specific Cas9-oligonucleotide conjugates. Sci Adv (2020) 6(15):eaaz0051. doi: 10.1126/sciadv.aaz0051
128. Lopez SC, Crawford KD, Lear SK, Bhattarai-Kline S, Shipman SL. Precise genome editing across kingdoms of life using retron-derived DNA. Nat Chem Biol (2022) 18(2):199–206. doi: 10.1038/s41589-021-00927-y
129. Farzadfard F, Lu TK. Genomically encoded analog memory with precise in vivo DNA writing in living cell populations. Science (2014) 346(6211):1256272. doi: 10.1126/science.1256272
130. Sharon E, Chen S-AA, Khosla NM, Smith JD, Pritchard JK, Fraser HB. Functional genetic variants revealed by massively parallel precise genome editing. Cell (2018) 175(2):544–57. doi: 10.1016/j.cell.2018.08.057
131. Schubert MG, Goodman DB, Wannier TM, Kaur D, Farzadfard F, Lu TK, et al. High-throughput functional variant screens Via in vivo production of single-stranded DNA. Proc Natl Acad Sci (2021) 118(18):e2018181118. doi: 10.1073/pnas.2018181118
132. Mirochnitchenko O, Inouye S, Inouye M. Production of single-stranded DNA in mammalian cells by means of a bacterial retron. J Biol Chem (1994) 269(4):2380–3. doi: 10.1016/S0021-9258(17)41956-9
133. Zhang XH, Tee LY, Wang XG, Huang QS, Yang SH. Off-target effects in Crispr/Cas9-mediated genome engineering. Mol Ther Nucleic Acids (2015) 4(11):e264. doi: 10.1038/mtna.2015.37
134. Pattanayak V, Lin S, Guilinger JP, Ma E, Doudna JA, Liu DR. High-throughput profiling of off-target DNA cleavage reveals rna-programmed Cas9 nuclease specificity. Nat Biotechnol (2013) 31(9):839–43. doi: 10.1038/nbt.2673
135. Fu Y, Foden JA, Khayter C, Maeder ML, Reyon D, Joung JK, et al. High-frequency off-target mutagenesis induced by crispr-cas nucleases in human cells. Nat Biotechnol (2013) 31(9):822–6. doi: 10.1038/nbt.2623
136. Cho SW, Kim S, Kim Y, Kweon J, Kim HS, Bae S, et al. Analysis of off-target effects of Crispr/Cas-derived rna-guided endonucleases and nickases. Genome Res (2014) 24(1):132–41. doi: 10.1101/gr.162339.113
137. Corrigan-Curay J, O’reilly M, Kohn DB, Cannon PM, Bao G, Bushman FD, et al. Genome editing technologies: Defining a path to clinic: Genomic editing: Establishing preclinical toxicology standards, Bethesda, Maryland 10 June 2014. Mol Ther (2015) 23(5):796–806. doi: 10.1038/mt.2015.54
138. Nishida K, Arazoe T, Yachie N, Banno S, Kakimoto M, Tabata M, et al. Targeted nucleotide editing using hybrid prokaryotic and vertebrate adaptive immune systems. Science (2016) 353(6305):aaf8729. doi: 10.1126/science.aaf8729
139. Mok BY, Kotrys AV, Raguram A, Huang TP, Mootha VK, Liu DR. Crispr-free base editors with enhanced activity and expanded targeting scope in mitochondrial and nuclear DNA. Nat Biotechnol (2022) 40:1378–87. doi: 10.1038/s41587-022-01256-8
140. Newby GA, Yen JS, Woodard KJ, Mayuranathan T, Lazzarotto CR, Li Y, et al. Base editing of haematopoietic stem cells rescues sickle cell disease in mice. Nature (2021) 595(7866):295–302. doi: 10.1038/s41586-021-03609-w
141. Kingwell K. Base editors hit the clinic. Nat Rev Drug Discov (2022) 21:545–7. doi: 10.1038/d41573-022-00124-z
142. Zeng J, Wu Y, Ren C, Bonanno J, Shen AH, Shea D, et al. Therapeutic base editing of human hematopoietic stem cells. Nat Med (2020) 26(4):535–41. doi: 10.1038/s41591-020-0790-y
143. Musunuru K, Chadwick AC, Mizoguchi T, Garcia SP, DeNizio JE, Reiss CW, et al. In vivo crispr base editing of Pcsk9 durably lowers cholesterol in primates. Nature (2021) 593(7859):429–34. doi: 10.1038/s41586-021-03534-y
144. Webber BR, Lonetree C-l, Kluesner MG, Johnson MJ, Pomeroy EJ, Diers MD, et al. Highly efficient multiplex human T cell engineering without double-strand breaks using Cas9 base editors. Nat Commun (2019) 10(1):5222. doi: 10.1038/s41467-019-13007-6
145. Georgiadis C, Rasaiyaah J, Gkazi SA, Preece R, Etuk A, Christi A, et al. Base-edited car T cells for combinational therapy against T cell malignancies. Leukemia (2021) 35(12):3466–81. doi: 10.1038/s41375-021-01282-6
146. Diorio C, Murray R, Naniong M, Barrera LA, Camblin AJ, Chukinas JA, et al. Cytosine base editing enables quadruple-edited allogeneic car-T cells for T-all. Blood (2022) 140:619–29. doi: 10.1182/blood.2022015825
147. Nelson JW, Randolph PB, Shen SP, Everette KA, Chen PJ, Anzalone AV, et al. Engineered pegrnas improve prime editing efficiency. Nat Biotechnol (2022) 40(3):402–10. doi: 10.1038/s41587-021-01039-7
148. Ioannidi EI, Yarnall MTN, Schmitt-Ulms C, Krajeski RN, Lim J, Villiger L, et al. Drag-and-Drop genome insertion without DNA cleavage with crispr-directed integrases. bioRxiv (2021). doi: 10.1101/2021.11.01.466786
149. Sterner RM, Sakemura R, Cox MJ, Yang N, Khadka RH, Forsman CL, et al. Gm-csf inhibition reduces cytokine release syndrome and neuroinflammation but enhances car-T cell function in xenografts. Blood (2019) 133(7):697–709. doi: 10.1182/blood-2018-10-881722
150. Zhu H, Blum RH, Bernareggi D, Ask EH, Wu Z, Hoel HJ, et al. Metabolic reprograming via deletion of cish in human ipsc-derived nk cells promotes in vivo persistence and enhances anti-tumor activity. Cell Stem Cell (2020) 27:224–37.e6. doi: 10.1016/j.stem.2020.05.008
151. Goodridge JP, Bjordahl R, Mahmood S, Reiser J, Gaidarova S, Blum R, et al. Ft576: Multi-specific off-the-Shelf car-nk cell therapy engineered for enhanced persistence, avoidance of self-fratricide and optimized mab combination therapy to prevent antigenic escape and elicit a deep and durable response in multiple myeloma. Blood (2020) 136(Supplement 1):4–5. doi: 10.1182/blood-2020-142750
152. Poirot L, Philip B, Schiffer-Mannioui C, Le Clerre D, Chion-Sotinel I, Derniame S, et al. Multiplex genome-edited T-cell manufacturing platform for “Off-the-Shelf” adoptive T-cell immunotherapiesgenome editing for allogenic adoptive T-cell immunotherapy. Cancer Res (2015) 75(18):3853–64. doi: 10.1158/0008-5472.CAN-14-3321
153. Jo S, Das S, Williams A, Chretien A-S, Pagliardini T, Le Roy A, et al. Endowing universal car T-cell with immune-evasive properties using talen-gene editing. Nat Commun (2022) 13(1):3453. doi: 10.1038/s41467-022-30896-2
154. Wang T, Wei JJ, Sabatini DM, Lander ES. Genetic screens in human cells using the crispr-Cas9 system. Science (2014) 343(6166):80–4. doi: 10.1126/science.1246981
155. Shalem O, Sanjana NE, Hartenian E, Shi X, Scott DA, Mikkelsen TS, et al. Genome-scale crispr-Cas9 knockout screening in human cells. Science (2014) 343(6166):84–7. doi: 10.1126/science.1247005
156. Parrish PCR, Thomas JD, Gabel AM, Kamlapurkar S, Bradley RK, Berger AH. Discovery of synthetic lethal and tumor suppressor paralog pairs in the human genome. Cell Rep (2021) 36(9):109597. doi: 10.1016/j.celrep.2021.109597
157. Zetsche B, Heidenreich M, Mohanraju P, Fedorova I, Kneppers J, DeGennaro EM, et al. Multiplex gene editing by crispr–Cpf1 using a single crrna array. Nat Biotechnol (2017) 35(1):31–4. doi: 10.1038/nbt.3737
158. Chow RD, Wang G, Ye L, Codina A, Kim HR, Shen L, et al. In vivo profiling of metastatic double knockouts through crispr–Cpf1 screens. Nat Methods (2019) 16(5):405–8. doi: 10.1038/s41592-019-0371-5
159. Gier RA, Budinich KA, Evitt NH, Cao Z, Freilich ES, Chen Q, et al. High-performance crispr-Cas12a genome editing for combinatorial genetic screening. Nat Commun (2020) 11(1):3455. doi: 10.1038/s41467-020-17209-1
160. Larson RC, Kann MC, Bailey SR, Haradhvala NJ, Llopis PM, Bouffard AA, et al. Car T cell killing requires the ifnγr pathway in solid but not liquid tumours. Nature (2022) 604(7906):563–70. doi: 10.1038/s41586-022-04585-5
161. Sheffer M, Lowry E, Beelen N, Borah M, Amara SN, Mader CC, et al. Genome-scale screens identify factors regulating tumor cell responses to natural killer cells. Nat Genet (2021) 53(8):1196–206. doi: 10.1038/s41588-021-00889-w
162. Shifrut E, Carnevale J, Tobin V, Roth TL, Woo JM, Bui CT, et al. Genome-wide crispr screens in primary human T cells reveal key regulators of immune function. Cell (2018) 175(7):1958–71 e15. doi: 10.1016/j.cell.2018.10.024
163. Ting PY, Parker AE, Lee JS, Trussell C, Sharif O, Luna F, et al. Guide swap enables genome-scale pooled crispr-Cas9 screening in human primary cells. Nat Methods (2018) 15(11):941–6. doi: 10.1038/s41592-018-0149-1
164. Carnevale J, Shifrut E, Kale N, Nyberg WA, Blaeschke F, Chen YY, et al. Rasa2 ablation in T cells boosts antigen sensitivity and long-term function. Nature (2022) 609(7925):174–82. doi: 10.1038/s41586-022-05126-w
165. Wang D, Prager BC, Gimple RC, Aguilar B, Alizadeh D, Tang H, et al. Crispr screening of car T cells and cancer stem cells reveals critical dependencies for cell-based therapies. Cancer Discov (2021) 11(5):1192–211. doi: 10.1158/2159-8290.CD-20-1243
166. Legut M, Gajic Z, Guarino M, Daniloski Z, Rahman JA, Xue X, et al. A genome-scale screen for synthetic drivers of T cell proliferation. Nature (2022) 603(7902):728–35. doi: 10.1038/s41586-022-04494-7
167. Blaeschke F, Chen YY, Apathy R, Li Z, Mowery CT, Nyberg WA, et al. Modular pooled discovery of synthetic knockin sequences to program durable cell therapies. bioRxiv (2022). doi: 10.1101/2022.07.27.501186
168. Schmidt R, Steinhart Z, Layeghi M, Freimer JW, Bueno R, Nguyen VQ, et al. Crispr activation and interference screens decode stimulation responses in primary human T cells. Science (2022) 375(6580):eabj4008. doi: 10.1126/science.abj4008
169. Henriksson J, Chen X, Gomes T, Ullah U, Meyer KB, Miragaia R, et al. Genome-wide crispr screens in T helper cells reveal pervasive crosstalk between activation and differentiation. Cell (2019) 176(4):882–96.e18. doi: 10.1016/j.cell.2018.11.044
170. Parnas O, Jovanovic M, Eisenhaure Thomas M, Herbst Rebecca H, Dixit A, Ye Chun J, et al. A genome-wide crispr screen in primary immune cells to dissect regulatory networks. Cell (2015) 162(3):675–86. doi: 10.1016/j.cell.2015.06.059
171. LaFleur MW, Nguyen TH, Coxe MA, Yates KB, Trombley JD, Weiss SA, et al. A crispr-Cas9 delivery system for in vivo screening of genes in the immune system. Nat Commun (2019) 10(1):1668. doi: 10.1038/s41467-019-09656-2
172. Dong MB, Wang G, Chow RD, Ye L, Zhu L, Dai X, et al. Systematic immunotherapy target discovery using genome-scale in vivo crispr screens in Cd8 t cells. Cell (2019) 178(5):1189–204.e23. doi: 10.1016/j.cell.2019.07.044
173. Ye L, Park JJ, Dong MB, Yang Q, Chow RD, Peng L, et al. In vivo crispr screening in Cd8 T cells with aav–sleeping beauty hybrid vectors identifies membrane targets for improving immunotherapy for glioblastoma. Nat Biotechnol (2019) 37(11):1302–13. doi: 10.1038/s41587-019-0246-4
174. Wei J, Long L, Zheng W, Dhungana Y, Lim SA, Guy C, et al. Targeting regnase-1 programs long-lived effector T cells for cancer therapy. Nature (2019) 576(7787):471–6. doi: 10.1038/s41586-019-1821-z
175. Belk JA, Yao W, Ly N, Freitas KA, Chen Y-T, Shi Q, et al. Genome-wide crispr screens of T cell exhaustion identify chromatin remodeling factors that limit T cell persistence. Cancer Cell (2022) 40(7):768–86.e7. doi: 10.1016/j.ccell.2022.06.001
176. Bock C, Datlinger P, Chardon F, Coelho MA, Dong MB, Lawson KA, et al. High-content crispr screening. Nat Rev Methods Primers (2022) 2(1):8. doi: 10.1038/s43586-021-00093-4
177. Replogle JM, Saunders RA, Pogson AN, Hussmann JA, Lenail A, Guna A, et al. Mapping information-rich genotype-phenotype landscapes with genome-scale perturb-seq. Cell (2022) 185(14):2559–75.e28. doi: 10.1016/j.cell.2022.05.013
178. Ye L, Park JJ, Peng L, Yang Q, Chow RD, Dong MB, et al. A genome-scale gain-of-Function crispr screen in Cd8 T cells identifies proline metabolism as a means to enhance car-T therapy. Cell Metab (2022) 34(4):595–614.e14. doi: 10.1016/j.cmet.2022.02.009
179. Wang G, Chow RD, Bai Z, Zhu L, Errami Y, Dai X, et al. Multiplexed activation of endogenous genes by crispra elicits potent antitumor immunity. Nat Immunol (2019) 20(11):1494–505. doi: 10.1038/s41590-019-0500-4
180. Joung J, Kirchgatterer PC, Singh A, Cho JH, Nety SP, Larson RC, et al. Crispr activation screen identifies bcl-2 proteins and B3gnt2 as drivers of cancer resistance to T cell-mediated cytotoxicity. Nat Commun (2022) 13(1):1606. doi: 10.1038/s41467-022-29205-8
181. Gilbert LA, Horlbeck MA, Adamson B, Villalta JE, Chen Y, Whitehead EH, et al. Genome-scale crispr-mediated control of gene repression and activation. Cell (2014) 159(3):647–61. doi: 10.1016/j.cell.2014.09.029
182. Umkehrer C, Holstein F, Formenti L, Jude J, Froussios K, Neumann T, et al. Isolating live cell clones from barcoded populations using crispra-inducible reporters. Nat Biotechnol (2021) 39(2):174–8. doi: 10.1038/s41587-020-0614-0
183. Cox DBT, Gootenberg JS, Abudayyeh OO, Franklin B, Kellner MJ, Joung J, et al. Rna editing with crispr-Cas13. Science (2017) 358(6366):1019–27. doi: 10.1126/science.aaq0180
184. Abudayyeh OO, Gootenberg JS, Konermann S, Joung J, Slaymaker IM, Cox DB, et al. C2c2 is a single-component programmable rna-guided rna-targeting crispr effector. Science (2016) 353(6299):aaf5573. doi: 10.1126/science.aaf5573
185. Shmakov S, Abudayyeh OO, Makarova KS, Wolf YI, Gootenberg JS, Semenova E, et al. Discovery and functional characterization of diverse class 2 crispr-cas systems. Mol Cell (2015) 60(3):385–97. doi: 10.1016/j.molcel.2015.10.008
186. Smargon AA, Cox DB, Pyzocha NK, Zheng K, Slaymaker IM, Gootenberg JS, et al. Cas13b is a type vi-b crispr-associated rna-guided rnase differentially regulated by accessory proteins Csx27 and Csx28. Mol Cell (2017) 65(4):618–30. doi: 10.1016/j.molcel.2016.12.023
187. Granados-Riveron JT, Aquino-Jarquin G. Crispr–Cas13 precision transcriptome engineering in cancer. Cancer Res (2018) 78(15):4107–13. doi: 10.1158/0008-5472.Can-18-0785
188. Ai Y, Liang D, Wilusz JE. Crispr/Cas13 effectors have differing extents of off-target effects that limit their utility in eukaryotic cells. Nucleic Acids Res (2022) 50(11):e65. doi: 10.1093/nar/gkac159
189. Tang X-ZE, Tan SX, Hoon S, Yeo GW. Pre-existing adaptive immunity to the rna-editing enzyme Cas13d in humans. Nat Med (2022) 28(7):1372–6. doi: 10.1038/s41591-022-01848-6
190. Méndez-Mancilla A, Wessels H-H, Legut M, Kadina A, Mabuchi M, Walker J, et al. Chemically modified guide rnas enhance crispr-Cas13 knockdown in human cells. Cell Chem Biol (2022) 29(2):321–7.e4. doi: 10.1016/j.chembiol.2021.07.011
191. Gillmore JD, Gane E, Taubel J, Kao J, Fontana M, Maitland ML, et al. Crispr-Cas9 in vivo gene editing for transthyretin amyloidosis. N Engl J Med (2021) 385(6):493–502. doi: 10.1056/NEJMoa2107454
192. Akinc A, Maier MA, Manoharan M, Fitzgerald K, Jayaraman M, Barros S, et al. The onpattro story and the clinical translation of nanomedicines containing nucleic acid-based drugs. Nat Nanotechnol (2019) 14(12):1084–7. doi: 10.1038/s41565-019-0591-y
193. Rurik JG, Tombácz I, Yadegari A, Fernández POM, Shewale SV, Li L, et al. Car T cells produced in vivo to treat cardiac injury. Science (2022) 375(6576):91–6. doi: 10.1126/science.abm0594
194. Agarwalla P, Ogunnaike EA, Ahn S, Froehlich KA, Jansson A, Ligler FS, et al. Bioinstructive implantable scaffolds for rapid in vivo manufacture and release of car-T cells. Nat Biotechnol (2022) 40:1250–8. doi: 10.1038/s41587-022-01245-x
195. Koblan LW, Erdos MR, Wilson C, Cabral WA, Levy JM, Xiong Z-M, et al. In vivo base editing rescues Hutchinson–gilford progeria syndrome in mice. Nature (2021) 589(7843):608–14. doi: 10.1038/s41586-020-03086-7
196. Davis JR, Wang X, Witte IP, Huang TP, Levy JM, Raguram A, et al. Efficient in vivo base editing via single adeno-associated viruses with size-optimized genomes encoding compact adenine base editors. Nat Biomed Eng (2022). doi: 10.1038/s41551-022-00911-4
197. Hoijer I, Emmanouilidou A, Ostlund R, van Schendel R, Bozorgpana S, Tijsterman M, et al. Crispr-Cas9 induces Large structural variants at on-target and off-target sites in vivo that segregate across generations. Nat Commun (2022) 13(1):627. doi: 10.1038/s41467-022-28244-5
198. Cong L, Ran FA, Cox D, Lin S, Barretto R, Habib N, et al. Multiplex genome engineering using Crispr/Cas systems. Science (2013) 339(6121):819–23. doi: 10.1126/science.1231143
199. Mali P, Yang L, Esvelt KM, Aach J, Guell M, DiCarlo JE, et al. Rna-guided human genome engineering Via Cas9. Science (2013) 339(6121):823–6. doi: 10.1126/science.1232033
200. Hsu PD, Scott DA, Weinstein JA, Ran FA, Konermann S, Agarwala V, et al. DNA Targeting specificity of rna-guided Cas9 nucleases. Nat Biotechnol (2013) 31(9):827–32. doi: 10.1038/nbt.2647
201. Tsai SQ, Zheng Z, Nguyen NT, Liebers M, Topkar VV, Thapar V, et al. Guide-seq enables genome-wide profiling of off-target cleavage by crispr-cas nucleases. Nat Biotechnol (2015) 33(2):187–97. doi: 10.1038/nbt.3117
202. Tsai SQ, Nguyen NT, Malagon-Lopez J, Topkar VV, Aryee MJ, Joung JK. Circle-seq: A highly sensitive in vitro screen for genome-wide crispr-Cas9 nuclease off-targets. Nat Methods (2017) 14(6):607–14. doi: 10.1038/nmeth.4278
203. Dobosy JR, Rose SD, Beltz KR, Rupp SM, Powers KM, Behlke MA, et al. Rnase h-dependent pcr (Rhpcr): Improved specificity and single nucleotide polymorphism detection using blocked cleavable primers. BMC Biotechnol (2011) 11:80. doi: 10.1186/1472-6750-11-80
204. Rose JC, Popp NA, Richardson CD, Stephany JJ, Mathieu J, Wei CT, et al. Suppression of unwanted crispr-Cas9 editing by Co-administration of catalytically inactivating truncated guide rnas. Nat Commun (2020) 11(1):2697. doi: 10.1038/s41467-020-16542-9
205. Kleinstiver BP, Sousa AA, Walton RT, Tak YE, Hsu JY, Clement K, et al. Engineered crispr-Cas12a variants with increased activities and improved targeting ranges for gene, epigenetic and base editing. Nat Biotechnol (2019) 37(3):276–82. doi: 10.1038/s41587-018-0011-0
206. Tycko J, Myer VE, Hsu PD. Methods for optimizing crispr-Cas9 genome editing specificity. Mol Cell (2016) 63(3):355–70. doi: 10.1016/j.molcel.2016.07.004
207. Naeem M, Majeed S, Hoque MZ, Ahmad I. Latest developed strategies to minimize the off-target effects in crispr-Cas-Mediated genome editing. Cells (2020) 9(7):1608. doi: 10.3390/cells9071608
208. Guilinger JP, Thompson DB, Liu DR. Fusion of catalytically inactive Cas9 to foki nuclease improves the specificity of genome modification. Nat Biotechnol (2014) 32(6):577–82. doi: 10.1038/nbt.2909
209. Center for Biologics Evaluation and Research UFaDA. Guidance document: Considerations for the development of chimeric antigen receptor (Car) T cell products. US Food Drug Administration (2022). Silver Spring, MD.
210. Administration UFaD. Human gene therapy products incorporating human genome editing. Vaccines Blood Biol US Food Drug Administration (2022). Silver Spring, MD.
211. Cho JH, Okuma A, Sofjan K, Lee S, Collins JJ, Wong WW. Engineering advanced logic and distributed computing in human car immune cells. Nat Commun (2021) 12(1):792. doi: 10.1038/s41467-021-21078-7
212. Christopher MJ, Petti AA, Rettig MP, Miller CA, Chendamarai E, Duncavage EJ, et al. Immune escape of relapsed aml cells after allogeneic transplantation. N Engl J Med (2018) 379(24):2330–41. doi: 10.1056/NEJMoa1808777
213. Garrido F, Aptsiauri N, Doorduijn EM, Garcia Lora AM, van Hall T. The urgent need to recover mhc class I in cancers for effective immunotherapy. Curr Opin Immunol (2016) 39:44–51. doi: 10.1016/j.coi.2015.12.007
214. Taylor BC, Balko JM. Mechanisms of mhc-I downregulation and role in immunotherapy response. Front Immunol (2022) 13:844866. doi: 10.3389/fimmu.2022.844866
215. Nahas MR, Stroopinsky D, Rosenblatt J, Cole L, Pyzer AR, Anastasiadou E, et al. Hypomethylating agent alters the immune microenvironment in acute myeloid leukaemia (Aml) and enhances the immunogenicity of a dendritic Cell/Aml vaccine. Br J Haematol (2019) 185(4):679–90. doi: 10.1111/bjh.15818
216. Hicks KC, Fantini M, Donahue RN, Schwab A, Knudson KM, Tritsch SR, et al. Epigenetic priming of both tumor and nk cells augments antibody-dependent cellular cytotoxicity elicited by the anti-Pd-L1 antibody avelumab against multiple carcinoma cell types. OncoImmunology (2018) 7(11):e1466018. doi: 10.1080/2162402X.2018.1466018
217. Jin C, Ma J, Ramachandran M, Yu D, Essand M. Car T cells expressing a bacterial virulence factor trigger potent bystander antitumour responses in solid cancers. Nat Biomed Eng (2022) 6(7):830–41. doi: 10.1038/s41551-022-00875-5
218. Park AK, Fong Y, Kim S-I, Yang J, Murad JP, Lu J, et al. Effective combination immunotherapy using oncolytic viruses to deliver car targets to solid tumors. Sci Trans Med (2020) 12(559):eaaz1863. doi: 10.1126/scitranslmed.aaz1863
219. Rotolo A, Caputo VS, Holubova M, Baxan N, Dubois O, Chaudhry MS, et al. Enhanced anti-lymphoma activity of Car19-inkt cells underpinned by dual Cd19 and Cd1d targeting. Cancer Cell (2018) 34(4):596–610.e11. doi: 10.1016/j.ccell.2018.08.017
220. Heczey A, Liu D, Tian G, Courtney AN, Wei J, Marinova E, et al. Invariant nkt cells with chimeric antigen receptor provide a novel platform for safe and effective cancer immunotherapy. Blood J Am Soc Hematol (2014) 124(18):2824–33. doi: 10.1182/blood-2013-11-541235
221. Fisher J, Anderson J. Engineering approaches in human gamma delta T cells for cancer immunotherapy. Front Immunol (2018) 9:1409. doi: 10.3389/fimmu.2018.01409
222. Rischer M, Pscherer S, Duwe S, Vormoor J, Jürgens H, Rossig C. Human Γδ T cells as mediators of chimaeric-receptor redirected anti-tumour immunity. Br J Haematol (2004) 126(4):583–92. doi: 10.1111/j.1365-2141.2004.05077.x
223. Suh HC, Pohl K, Javier APL, Slamon DJ, Chute JP. Effect of dendritic cells (Dc) transduced with chimeric antigen receptor (Car) on car T-cell cytotoxicity. Am Soc Clin Oncol (2017) 35:144. doi: 10.1200/JCO.2017.35.7_suppl.144
224. Klichinsky M, Ruella M, Shestova O, Lu XM, Best A, Zeeman M, et al. Human chimeric antigen receptor macrophages for cancer immunotherapy. Nat Biotechnol (2020) 38(8):947–53. doi: 10.1038/s41587-020-0462-y
225. Wennerberg E, Kremer V, Childs R, Lundqvist A. Cxcl10-induced migration of adoptively transferred human natural killer cells toward solid tumors causes regression of tumor growth in vivo. Cancer Immunol Immunother (2015) 64(2):225–35. doi: 10.1007/s00262-014-1629-5
226. Sheridan C. Off-the-Shelf, gene-edited car-T cells forge ahead, despite safety scare. Nat Biotechnol (2021) 40:5–8. doi: 10.1038/d41587-021-00027-1
227. van der Stegen SJC, Lindenbergh PL, Petrovic RM, Xie H, Diop MP, Alexeeva V, et al. Generation of T-Cell-Receptor-Negative Cd8αβ-positive car T cells from T-Cell-Derived induced pluripotent stem cells. Nat Biomed Eng (2022). doi: 10.1038/s41551-022-00915-0
228. Kerbauy LN, Marin ND, Kaplan M, Banerjee PP, Berrien-Elliott MM, Becker-Hapak M, et al. Combining Afm13, a bispecific Cd30/Cd16 antibody, with cytokine-activated blood and cord blood-derived nk cells facilitates car-like responses against Cd30(+) malignancies. Clin Cancer Res (2021) 27(13):3744–56. doi: 10.1158/1078-0432.Ccr-21-0164
229. Gauthier L, Morel A, Anceriz N, Rossi B, Blanchard-Alvarez A, Grondin G, et al. Multifunctional natural killer cell engagers targeting Nkp46 trigger protective tumor immunity. Cell (2019) 177(7):1701–13.e16. doi: 10.1016/j.cell.2019.04.041
230. Arvindam US, van Hauten PMM, Schirm D, Schaap N, Hobo W, Blazar BR, et al. A trispecific killer engager molecule against Clec12a effectively induces nk-cell mediated killing of aml cells. Leukemia (2021) 35(6):1586–96. doi: 10.1038/s41375-020-01065-5
231. Vallera DA, Zhang B, Gleason MK, Oh S, Weiner LM, Kaufman DS, et al. Heterodimeric bispecific single-chain variable-fragment antibodies against epcam and Cd16 induce effective antibody-dependent cellular cytotoxicity against human carcinoma cells. Cancer Biother Radiopharmaceut (2013) 28(4):274–82. doi: 10.1089/cbr.2012.1329
232. Vallera DA, Felices M, McElmurry R, McCullar V, Zhou X, Schmohl JU, et al. Il15 trispecific killer engagers (Trike) make natural killer cells specific to Cd33+ targets while also inducing persistence, in vivo expansion, and enhanced function. Clin Cancer Res (2016) 22(14):3440–50. doi: 10.1158/1078-0432.CCR-15-2710
233. Schmohl JU, Felices M, Todhunter D, Taras E, Miller JS, Vallera DA. Tetraspecific scfv construct provides nk cell mediated adcc and self-sustaining stimuli Via insertion of il-15 as a cross-linker. Oncotarget (2016) 7(45):73830–44. doi: 10.18632/oncotarget.12073
234. Schmohl JU, Felices M, Oh F, Lenvik AJ, Lebeau AM, Panyam J, et al. Engineering of anti-Cd133 trispecific molecule capable of inducing nk expansion and driving antibody-dependent cell-mediated cytotoxicity. Cancer Res Treatment: Off J Korean Cancer Assoc (2017) 49(4):1140. doi: 10.4143/crt.2016.491
235. Schmohl J, Gleason M, Dougherty P, Miller JS, Vallera DA. Heterodimeric bispecific single chain variable fragments (Scfv) killer engagers (Bikes) enhance nk-cell activity against Cd133+ colorectal cancer cells. Targeted Oncol (2016) 11(3):353–61. doi: 10.1007/s11523-015-0391-8
236. Gleason MK, Ross JA, Warlick ED, Lund TC, Verneris MR, Wiernik A, et al. Cd16xcd33 bispecific killer cell engager (Bike) activates nk cells against primary mds and mdsc Cd33+ targets. Blood (2014) 123(19):3016–26. doi: 10.1182/blood-2013-10-533398
237. Wiernik A, Foley B, Zhang B, Verneris MR, Warlick E, Gleason MK, et al. Targeting natural killer cells to acute myeloid leukemia in vitro with a Cd16× 33 bispecific killer cell engager and Adam17 inhibition. Clin Cancer Res (2013) 19(14):3844–55. doi: 10.1158/1078-0432.CCR-13-0505
Keywords: cell engineering, gene editing, immune effector cell, cell therapy, CAR (chimeric antigen receptor), CRISPR screening
Citation: Biederstädt A, Manzar GS and Daher M (2022) Multiplexed engineering and precision gene editing in cellular immunotherapy. Front. Immunol. 13:1063303. doi: 10.3389/fimmu.2022.1063303
Received: 06 October 2022; Accepted: 31 October 2022;
Published: 22 November 2022.
Edited by:
Kevin Van der Jeught, University of Miami, United StatesReviewed by:
Alex Michael Abel, LEAH Labs, Inc., United StatesSema Kurtulus, 2Seventy Bio, United States
Copyright © 2022 Biederstädt, Manzar and Daher. This is an open-access article distributed under the terms of the Creative Commons Attribution License (CC BY). The use, distribution or reproduction in other forums is permitted, provided the original author(s) and the copyright owner(s) are credited and that the original publication in this journal is cited, in accordance with accepted academic practice. No use, distribution or reproduction is permitted which does not comply with these terms.
*Correspondence: May Daher, mdaher@mdanderson.org