- 1Department of Neurosurgery, Universitair Ziekenhuis Brussel (UZ Brussels), Brussels, Belgium
- 2C4N - Center for Neurosciences, Vrije Universiteit Brussel, Brussels, Belgium
- 3Laboratory for Medical & Molecular Oncology (LMMO), Vrije Universiteit Brussel, Brussels, Belgium
- 4Laboratory for Molecular and Cellular Therapy (LMCT), Vrije Universiteit Brussel, Brussels, Belgium
- 5Department of Medical Oncology, Universitair Ziekenhuis Brussel (UZ Brussels), Brussels, Belgium
Despite relentless efforts to improve outcome, the prognosis of glioblastoma (GBM) remains poor. Standard therapy at first diagnosis consists of maximal safe surgical resection followed by radiochemotherapy, but treatment options at recurrence are scarce and have limited efficacy. Immunotherapy is a broad term that covers several treatment strategies, including immune checkpoint inhibition (ICI). The successes of systemically administered therapeutic monoclonal antibodies that block the Programmed death receptor or ligand (PD-(L)1) and Cytotoxic T-Lymphocyte associated protein (CTLA)-4 immune checkpoints in other cancer types could not be reproduced in glioblastoma. This is considered to be related to the intrinsic low immunogenicity and strong immunosuppressive tumor microenvironment of glioblastoma, in addition to the presence of a blood-glioma and blood-brain barrier that limits many systemically administered therapeutic agents from reaching their target. In this mini-review, we address the specific aspects of immune suppression in glioblastoma and discuss potential strategies that could help to overcome it. The potential advantages of incorporating surgical resection in clinical trials of immunotherapy for glioblastoma, including window-of-opportunity studies, are highlighted. Combination strategies that include surgical resection, as well as local administration of therapeutic agents in the brain are discussed as a potential strategy to achieve an effective immunological response against glioblastoma.
Introduction
Glioblastoma (GBM) is a deadly disease. Standard treatment consists of maximal safe resection followed by radiochemotherapy. This treatment prolongs survival but the tumor recurs in the vast majority of patients, at which point no effective standard therapy has been defined (1). If glioblastoma recurrence is amenable for surgery, surgical resection will be proposed to the patient, but it is generally accepted that this needs to be completed with systemic therapy. Tumor response rates of any salvage therapy (chemotherapy, targeted- or immunotherapy) are typically shown to be 5 to 10%, six-month progression-free survival (6mPFS) from recurrence onward around 15% (PFS of 2 to 4 months), and median overall survival (OS) around 25 weeks (2, 3). Despite disappointing success rates of clinical trials, most studies have shown that a small minority of the patients will have durable responses to certain study treatments before relapse occurs - not influencing median survival but forming the tail of the Kaplan-Meier survival curve. This implies that response to treatment can differ greatly between patients, and characterizing which specific features of the tumor predicts a better response to available treatment options could advance treatment success in selected glioblastoma patients significantly. Attempts at predicting response have been frustrating, however, and no predictive marker has been validated for clinical decision-making today. It is assumed that this would require more detailed knowledge of the different aspects of the tumor at the molecular level, and an understanding of how first-line treatment influences tumor evolution in space and time. Besides important intertumoral heterogeneity, glioblastoma also has significant intratumoral heterogeneity that pertains to the tumor cells as well as to the tumor microenvironment (TME). This heterogeneity is the result of differential selective pressures originating from cancer treatment as well as the anti-tumor immune response, which leads to resistant clones that drive tumor progression, contributing to the aggressive behavior and strong immunosuppressive nature of the disease (4). Immune therapy has proven successful in tumors with high mutational burden and an inflammatory TME such as melanoma and non-small cell lung carcinoma, but large trials of systemically administered immune checkpoint inhibitors (ICI) in glioblastoma have shown mainly negative results (5, 6). Even though there is no improved overall survival and response rates are below 10%, responses - when they occur - are characterized by dense immune-cell infiltration on histopathology and tend to be more durable than with other treatment types (7). Case reports of children with germline bi-allelic mismatch repair deficiency (MMRd)-related glioblastoma successfully treated with checkpoint inhibitors at least demonstrate that the immune system can be harnessed to be active against glioblastoma and that the main challenge lies in converting the strong immunosuppressive microenvironment from a “cold” into a ‘hot’ one (5, 7). In adult patients, however, high microsatellite unstable (MSI-H) glioblastoma,harbouring MMRd, was found to be refractory to treatment with pembrolizumab (8). Knowledge of the mechanism of immune evasion of glioblastoma is constantly increasing and provides insight into what could constitute an effective immunotherapeutic strategy to treat this tumor.
The anti-tumor immune response
The current canonical concept of the cancer-immunity cycle dictates that tumor-specific antigens that are released from the tumor cells upon cell death are presented by dendritic cells (DCs) to the T-cells, leading to their activation and clonal expansion (9). Immune checkpoint signaling is crucial to regulate immunological inhibitory feedback mechanisms and the activation of T-cells (10). The activated T-cells then proceed to attack tumor cells expressing these antigens, causing more immunogenic cell death (ICD) (9). If, however, the immune response is not successful in completely eliminating the threat, natural selection of immune-resistant tumor cells is promoted. This process is known as immuno-editing and may select for immunosuppressive escape mechanisms (11). Based on the quantification of CD3 and CD8-positive T lymphocytes both in the tumor center and the invasive margin (ie the Immunoscore), tumors can be classified as hot, immunosuppressed, T-cell excluded, or cold (12, 13). These subtypes typically also harbor differential expression of immune checkpoint molecules, immune signature in gene expression analysis, and tumor mutational burden. Tumors are considered hot when they harbor high T-cell infiltration, expression of Programmed death-ligand 1 (PD-L1), high tumor mutational burden (TMB), and increased Interferon-gamma signaling. Immunosuppressed tumors show an intermediate amount of infiltrating T cells but have a TME that limits activation and further recruitment of immune cells despite the absence of physical barriers. Tumors that are characterized by poor T cell infiltration, low TMB, low Major Histocompatibility Complex (MHC) expression, and low PD-L1 expression are considered cold. As a fourth subtype, in excluded tumors, T cells and other effector cells are unable to efficiently infiltrate into the tumor nests and instead accumulate at the invasive margins of the tumor. Excluded tumors typically display increased hypoxia and increased angiogenesis, hallmarks of glioblastoma.
Immune suppression in glioblastoma
The glioblastoma TME is characterized by a high concentration of several immunosuppressive molecules, such as Interleukin (IL)-6, IL-10, Transforming Growth Factor-beta (TGF-β), and prostaglandin E2 (PG-E2). These molecules are produced by the tumor cells and tumor-associated macrophages (TAMs). TAMs form the largest immune infiltrate in glioblastoma tumors and exhibit extensive heterogeneity, reflecting their intrinsic ability to respond to the various environmental cues that exist within the tumor microenvironment. TAMs were found to consist of microglia (Mg)- and monocyte (Mo)-derived populations, with both TAM subsets displaying varying, but mostly tumor-promoting activities (14–17). Inhibition of the anti-tumor immune response by TAMs and tumor cells occurs by downregulating MHC expression, suppressing T-cell activation and expansion, inducing T-cell anergy, activating suppressive regulatory T-cells (Treg) and myeloid-derived suppressor cells (MDSC), and driving TAMs to the immunosuppressive M2 phenotype (18). Due to the aggressive growth of glioblastoma, tissue hypoxia is a frequent phenomenon. The hypoxia induces activation of HIF-1alpha and eventually VEGF production which has immunosuppressive properties (19).
As a result of the low tumor mutational burden (TMB) combined with all these mechanisms of immune suppression resulting in a relative paucity of tumor-infiltrating lymphocytes and low expression of anti-PD1, GBM has typically been labeled as a cold tumor. Even though all GBMs generally harbor an immune suppressive TME, recent work has shown inter-tumor heterogeneity in the immune contexture of glioblastoma TME. By studying the IDH-wt GBM TME transcriptome, three distinct GBM subtypes have been revealed: TMEhigh, TMEmed, and TMElow (20). The TMEhigh tumors have significantly increased expression of genes specific to all immune populations (elevated lymphocyte, myeloid cell immune checkpoint, PD-1, and CTLA-4 transcripts), TMEmed tumors are enriched for endothelial cell gene expression profiles and show heterogeneous immune cell populations. TMElow tumors show low expression of all immune and endothelial cell markers and are thus considered an ‘immune desert’ group.
Even though the mesenchymal subtype relatively less often has TMElow immune composition (10% vs 35% and 55% for the proneural and classical subtype, respectively), all TME subtypes could be found in all tumor cell gene expression subtypes (21). No correlation was observed between the specific TME subtype and patient survival. Nonetheless, response to immune therapy seemed better in the TMEhigh group of patients (20). This study also showed that these novel TME subtypes are dynamic and tend to evolve across primary and recurrent GBMs, contributing to longitudinal heterogeneity. This could imply that there is the potential for a forced subtype switch in glioblastoma, making the tumor more responsive to immune therapy. Either way, each TME subtype most likely requires a different immune strategy in order to optimize response (22).
Overcoming the immune suppressive nature of glioblastoma
Systemic administration of ICI has not been successful in obtaining improved survival results for patients with glioblastoma. ( (5, 6) It has been suggested before that for tumors with a cold TME, which by definition have deployed a combination of immunosuppressive strategies, combinatorial therapeutic strategies need to be deployed to achieve tumor response. This includes combining immune therapies (vaccination therapy, adoptive cell transfer, oncolytic virus therapy, ICI, TAM reprogramming) but also looking at smart immunotherapy combinations with currently used treatments such as surgery and radiation therapy (23). Indeed, these commonly employed treatments not only lead to tumor mass reduction and depletion of immunosuppressive cells, but also to increased tumor antigen uptake and presentation by DCs, thus promoting anti-tumor immunity (22). The exact effect of temozolomide (TMZ)-chemotherapy on immunotherapy effectiveness has not been fully elucidated. Although it has been suggested that lymphopenia caused by TMZ could prove beneficial since eradication of immune suppressive cells takes place, recent studies rather show that TMZ exerts a negative effect on immune effector cells (24). The immunosuppressive effect of corticosteroids, which are frequently used to reduce peritumoral edema in GBM patients, has been well established, and it is generally advisable to attempt to withhold steroids from patients treated with immunotherapy (25).
Vascular endothelial growth factor (VEGF) has been demonstrated to suppress antitumor immunity by stimulating the proliferation of regulatory T-cells (Treg) and inhibiting Dendritic Cell (DC) maturation (26, 27). Antiangiogenic agents that target VEGF or its receptor have been proposed as the ideal adjunct to immune therapy. This is not only because they could reinforce the antitumor immune response but also because they are effective against peritumoral edema and thus can limit the necessity of immunosuppressive corticosteroids. However, studies that combined the small molecule VEGF-R Tyrosin Kinase Inhibitor (TKI) axitinib with the anti-PD-L1 ICI avelumab or the monoclonal anti-VEGF antibody Bevacizumab with the anti-PD1 ICI pembrolizumab have not been able to demonstrate improved overall survival (28, 29). This might be due to the ineffectiveness of systemically administered ICI, and should not rule out antiangiogenic agents as a potential adjunct to effective immunotherapy for glioblastoma.
The use of ICI prior to surgery (neoadjuvant treatment) has been proposed as a promising strategy. In patients with recurrent glioblastoma, neoadjuvant anti-PD1 therapy (with nivolumab or pembrolizumab) resulted in higher immune cell infiltration, enhanced expression of interferon-γ-related genes, and enhanced clonal expansion of T cells (30, 31). Even though the impact of this neo-adjuvant therapy on survival is still not established, the principle that neo-adjuvant therapy with PD1 blockade induces a local immunomodulatory effect has been demonstrated.
Combination of surgery and immunotherapy
The role of the surgical intervention in the treatment of glioma of any type is currently being revalued in itself since it has been demonstrated that supratotal resection for low-grade glioma but also for glioblastoma improves survival compared to total resection (32, 33). This suggests that the resection of the invasive front of the tumor, which in glioblastoma is most likely located in the non-contrast enhancing area of the tumor, yields additional benefit.
The net immunological effect of the surgical resection is leaning toward immune suppression. Although the inevitable tissue and vascular trauma caused during surgery triggers the innate and, at a later stage, the adaptive immune system, regulation by immune suppression quickly follows. This immune suppression is characterized by the release of growth factors (VEGF, Platelet-derived growth factor (PDGF), TGF-β), clotting factors, stress hormones (glucocorticoids, catecholamines, prostaglandins), and cytokines into the extracellular space, and tends to last several weeks following the initial cancer surgery (34, 35). The postoperative period is thus marked by an immune suppressive state but there is mounting evidence that this state is reversible (34). Moreover, specifically in glioblastoma, the debulking of the hypoxic core and invasive margin of the tumor may achieve a reduction of immunosuppression as a net result. Consequently, the surgery and perioperative period provide a window of opportunity that not only can be exploited to obtain tissue for analysis but also to reduce immunosuppression and mount an effective anti-tumor immune response.
Local administration of immunotherapeutic agents
The search for effective systemic therapy for glioblastoma has not only been troubled by the intrinsic therapeutic resistance mechanisms and substantial spatial and longitudinal heterogeneity of these tumors, but also by the fact that they lie secluded within the confines of the Blood-Brain Barrier (BBB) (36). Since the BBB prevents most systemic agents from reaching their target within the central nervous system, local delivery strategies are increasingly being explored. Explored strategies for local application include drug-loaded biodegradable wafers, drug-loaded hydrogels, intrathecal delivery using an Ommaya reservoir, direct intraoperative injection into the brain tumor or resection cavity wall and convection-enhanced delivery through one or several implanted catheters (37–39). Each of these strategies has its specific advantages and disadvantages (40). Local administration of recombinant oncolytic virus (recombinant polio–rhinovirus chimera PVSRIPO, adenovirus DNX-2401, Vocimagene Amiretrorepvec TOCA-511, etc) has been performed previously (41–43) Direct local injection of the therapeutic agent has the advantage that it is straightforward, can be incorporated into the workflow of a brain tumor resection, and can be performed at multiple locations allowing coverage of the entire tissue lining of the resection cavity.
Glitipni trial
In order to obtain a sufficiently high local concentration of nivolumab and ipilimumab while still avoiding potential (systemic) side effects that have traditionally limited clinical trials with anti-cytotoxic T-lymphocyte associated protein-4 (CTLA-4), we hypothesized that local administration following resection of glioblastoma could improve the anti-tumor immune response and improve patient outcome (44). We thus set up a phase I adaptive clinical trial (the “Glitipni” trial) with intracerebral injection of nivolumab and ipilimumab where, after dose escalation for each product has been performed, additional methods of local administration as well as additional immunotherapy are to be added to new cohorts. Patients with recurrent glioblastoma that are candidates for resection of their recurrence, have good performance status, and are not taking steroids are eligible for participation. Twenty-four hours preoperatively, patients receive an intravenous administration of low-dose (10mg) nivolumab. Maximal safe resection is performed, and at the end of the surgery, the immune checkpoint inhibitors (ipilimumab (IPI) 10mg/2ml alone in cohort 1 and ipilimumab 5mg/1ml and nivolumab 10mg/1ml from cohort 2 onward) are injected in the wall of the resection cavity in 20 to 30 injections of approximately 0,1ml each. (Figure 1) Postoperatively, intravenous administration of low-dose nivolumab was pursued every two weeks. These methods were used in cohorts 1 and 2, the results of which have been published recently and are summarized hereunder (45).
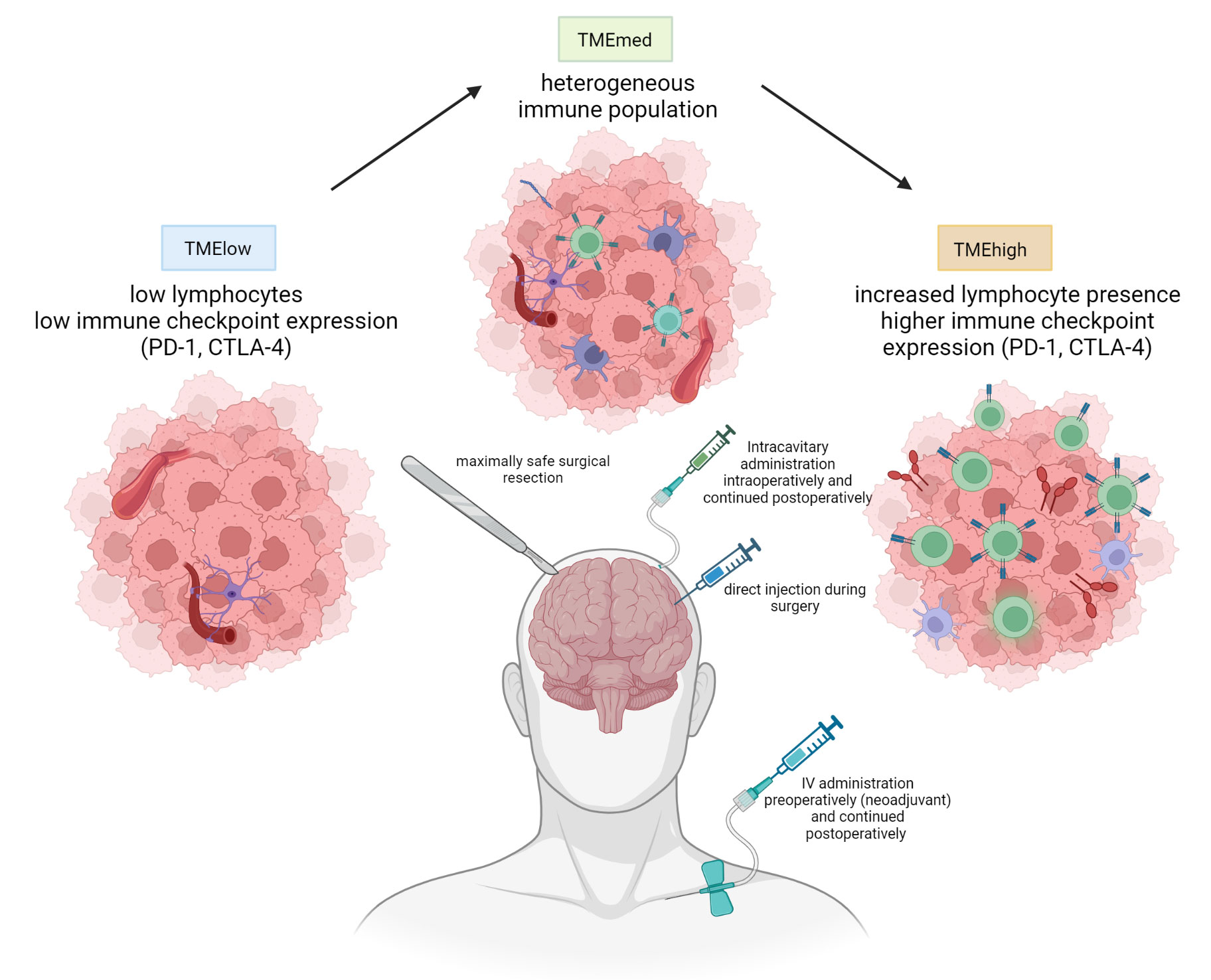
Figure 1 Current methods used in the Glitipni study. The aim of the strategy is to combine surgical treatment with a combination of local and systemic immunotherapy by decreasing immunosuppression and inducing immune response. Currently employed immunotherapeutic agents in our trials include local, locoregional, and systemic immune checkpoint inhibitors and local myeloid dendritic cells (created with BioRender.com).
Patient demographics
Twenty-seven patients in total were enrolled: three patients were included in cohort 1, and twenty-four in cohort 2. Most patients (81%) had a World Health Organisation (WHO) grade IV tumor at first diagnosis and 93% had an Isocitrate dehydrogenase (IDH)-wild-type tumor. All patients had surgical resection at first diagnosis, followed by standard radiochemotherapy in 85%. Thirty percent of patients had previously undergone resection for recurrence at inclusion, and the median number of prior systemic therapies was three. Summarizing, these two cohorts generally consisted of patients with good performance scores, under low-dose or no corticosteroids but with some history of treatment for recurrence.
Adverse events
There were no surgical complications related to the intraoperative injections of ipilimumab (cohort-1), or ipilimumab plus nivolumab (cohort-2). Postoperative fever was seen in 14/27 patients and was grade 1 in 12 patients, which included all three patients from cohort-1, and grade 2 in 2 patients. There was a low incidence of suspected immune-related adverse events: pruritus (n=7), rash (n=4), hypothyroidism (n=2), a sarcoid-like reaction (n=1), and fatigue (n=17). Subacute postoperative neurological deterioration was seen in two patients. This was considered to be due to excessive edema as a result of the immune response, and full recovery was seen in these patients upon steroid treatment.
Efficacy
The median PFS for both cohorts combined was 11.7 weeks (95% CI: 10 to 12; range 2–152), while median OS was 38 weeks (95%CI: 27 to 49) with a one and two-year PFS of 40.7% (95% CI: 22 to 59) and 27% (95% CI: 9 to 44), respectively. Proof-of-concept of an effective immune response following study treatment was seen in the three patients that underwent repeat resection upon suspected recurrence. In one of these patients, the resected tissue consisted of glioblastoma tumor cells with focal lymphocytic infiltration. In the two other patients, the resected tissue consisted of infiltration by inflammatory cells mainly composed of lymphocytes without any evidence of glioblastoma tumor recurrence at all. In translational analyses, no correlation could be found between PD-L1 immunohistochemistry or gene expression profile and OS in univariate analysis. B7-H3 gene expression was the only factor that remained associated with significantly worse survival in multivariate Cox logistic regression (p>0.029).
Current status and future prospects for Glitipni
As illustrated in Table 1, adaptations to dosing, administered compounds, and methods of administration have been made following the first two cohorts. At the moment of writing, 102 patients have been treated in the context of the trial, spanning all cohorts. Results from grouped cohorts will be published in the following year.
Thus far, we performed experimental therapy with intracerebral and intracavitary injection of ICI in varying combinations and administration routes, and also combined ICI with intracerebral injection of autologous myeloid dendritic cells. In earlier work performed by our group, intratumoral injection of these cells was found to be able to obtain durable complete remissions in some patients with ICI refractory melanoma (46). For the planned upcoming trial, we will complement the established per- and postoperative immunotherapy with neoadjuvant administration of ipilimumab and nivolumab starting four weeks prior to the surgical intervention, at which point intracerebral and intracavitary injection of ICI will take place at the doses established in the previous cohorts. This will also allow us to sample Cerebrospinal fluid (CSF) and tissue to examine the concentration of study drugs. Extensive translational analysis of the tumor tissue and CSF, including bulk and single-cell tumor and TME genome profiling, as well as assessment of the T-cell repertoire, will also allow us to look into the impact of neoadjuvant therapy on the TME and help in developing predictive markers for the effectiveness of immunotherapy.
Conclusions
Many hurdles remain in finding an effective treatment for glioblastoma, but preclinical and clinical evidence points towards a potentially important role for immunotherapy. Difficulties remain determining the optimal method of administration, choosing the exact immunotherapy type, personalization of therapy to suit the patient’s tumor-specific characteristics, and judging the role of the combination of immunotherapy with currently employed treatment, including surgery, chemo- and radiotherapy, and perhaps even steroids. In immunologically cold tumors like glioblastoma, combinations of immunotherapeutic efforts are most likely to generate an effective anti-tumor immune response. Administering ICI in the neo-adjuvant setting prior to surgery seems to shift the tumor TME towards a more immunotherapy-receptive environment in a subset of cases. The surgical intervention following the neo-adjuvant treatment can also be leveraged to not only locally administer the immunotherapeutic agents but also to obtain tissue for pharmacokinetic studies. As a locally administered immunotherapeutic, the combination of ICI with myeloid dendritic cells currently seems most promising based on the preliminary results of our Glitipni clinical trial.
Author contributions
JD wrote the first draft of the manuscript. All authors contributed to manuscript revision, read, and approved the submitted version.
Funding
Johnny Duerinck has received a postdoctoral mandate from Stichting Tegen Kanker for his work in the Glitipni study. The Glitipni study is sponsored and funded by the Universitair Ziekenhuis and Kom op Tegen Kanker.
Acknowledgments
We would like to acknowledge the patients who consented to participate in the Glitipni study, their families, and professional healthcare providers. We would also like to thank the study nurses Katrien Van den Bossche, Maud Allard, Silke Knockaert, and Evelien Vandeurzen (UZ Brussel) for their help with the data collection.
Conflict of interest
BN reports personal financial compensation from Roche, Bristol-Myers Squibb, Merck Sharp & Dohme, Novartis, and AstraZeneca for public speaking, consultancy, and participation in advisory board meetings.
The remaining authors declare that the research was conducted in the absence of any commercial or financial relationships that could be construed as a potential conflict of interest.
Publisher’s note
All claims expressed in this article are solely those of the authors and do not necessarily represent those of their affiliated organizations, or those of the publisher, the editors and the reviewers. Any product that may be evaluated in this article, or claim that may be made by its manufacturer, is not guaranteed or endorsed by the publisher.
References
1. Stupp R, Hegi ME, Mason WP, van den Bent MJ, Taphoorn MJB, Janzer RC, et al. Effects of radiotherapy with concomitant and adjuvant temozolomide versus radiotherapy alone on survival in glioblastoma in a randomised phase III study: 5-year analysis of the EORTC-NCIC trial. Lancet Oncol (2009) 10:459–66. doi: 10.1016/S1470-2045(09)70025-7
2. Wong ET, Hess KR, Gleason MJ, Jaeckle KA, Kyritsis AP, Prados MD, et al. Outcomes and prognostic factors in recurrent glioma patients enrolled onto phase II clinical trials. J Clin Oncol (1999) 17:2572–8. doi: 10.1200/JCO.1999.17.8.2572
3. Lamborn KR, Yung WKA, Chang SM, Wen PY, Cloughesy TF, DeAngelis LM, et al. Progression-free survival: an important end point in evaluating therapy for recurrent high-grade gliomas. Neuro Oncol (2008) 10:162–70. doi: 10.1215/15228517-2007-062
4. Kim EL, Sorokin M, Kantelhardt SR, Kalasauskas D, Sprang B, Fauss J, et al. Intratumoral heterogeneity and longitudinal changes in gene expression predict differential drug sensitivity in newly diagnosed and recurrent glioblastoma. Cancers (2020) 12(2):520. doi: 10.3390/cancers12020520
5. Sampson JH, Omuro AMP, Preusser M, Lim M, Butowski NA, Cloughesy TF, et al. A randomized, phase 3, open-label study of nivolumab versus temozolomide (TMZ) in combination with radiotherapy (RT) in adult patients (pts) with newly diagnosed, O-6-methylguanine DNA methyltransferase (MGMT)-unmethylated glioblastoma (GBM): CheckMate-498. J Clin Orthod (2016) 34:TPS2079–TPS2079. doi: 10.1200/JCO.2016.34.15_suppl.TPS2079
6. Reardon DA, Omuro A, Brandes AA, Rieger J, Wick A, Sepulveda J, et al. (2017). Randomized phase 3 study evaluating the efficacy and safety of nivolumab vs bevacizumab in patients with recurrent glioblastoma: checkmate 143. Neuro-Oncol 19 (suppl_3):iii21. doi: 10.1093/neuonc/nox036.071
7. Bouffet E, Campbell BB, Merico D, Borja RD, Aronson M, Durno C, et al. Immune checkpoint inhibition for hypermutant glioblastoma multiforme resulting from germline biallelic mismatch repair deficiency. J Clin Oncol (2016) 34:2206–11. doi: 10.1200/JCO.2016.66.6552
8. Marabelle A, Le DT, Ascierto PA, Di Giacomo AM, De Jesus-Acosta A, Delord J-P, et al. Efficacy of pembrolizumab in patients with noncolorectal high microsatellite Instability/Mismatch repair-deficient cancer: results from the phase II KEYNOTE-158 study. J Clin Oncol (2020) 38:1–10. doi: 10.1200/JCO.19.02105
9. Chen DS, Mellman I. Oncology meets immunology: the cancer-immunity cycle. Immunity (2013) 39:1–10. doi: 10.1016/j.immuni.2013.07.012
10. Bagchi S, Yuan R, Engleman EG. Immune checkpoint inhibitors for the treatment of cancer: clinical impact and mechanisms of response and resistance. Annu Rev Pathol (2021) 16:223–49. doi: 10.1146/annurev-pathol-042020-042741
11. O’Donnell JS, Teng MWL, Smyth MJ. Cancer immunoediting and resistance to T cell-based immunotherapy. Nat Rev Clin Oncol (2019) 16:151–67. doi: 10.1038/s41571-018-0142-8
12. Galon J, Bruni D. Approaches to treat immune hot, altered and cold tumours with combination immunotherapies. Nat Rev Drug Discovery (2019) 18:197–218. doi: 10.1038/s41573-018-0007-y
13. Angell H, Galon J. From the immune contexture to the immunoscore: the role of prognostic and predictive immune markers in cancer. Curr Opin Immunol (2013) 25:261–7. doi: 10.1016/j.coi.2013.03.004
14. Friebel E, Kapolou K, Unger S, Núñez NG, Utz S, Rushing EJ, et al. Single-cell mapping of human brain cancer reveals tumor-specific instruction of tissue-invading leukocytes. Cell (2020) 181:1626–1642.e20. doi: 10.1016/j.cell.2020.04.055
15. Klemm F, Maas RR, Bowman RL, Kornete M, Soukup K, Nassiri S, et al. Interrogation of the microenvironmental landscape in brain tumors reveals disease-specific alterations of immune cells. Cell (2020) 181:1643–1660.e17. doi: 10.1016/j.cell.2020.05.007
16. Pombo Antunes AR, Scheyltjens I, Lodi F, Messiaen J, Antoranz A, Duerinck J, et al. Single-cell profiling of myeloid cells in glioblastoma across species and disease stage reveals macrophage competition and specialization. Nat Neurosci (2021) 24:595–610. doi: 10.1038/s41593-020-00789-y
17. Mundt S, Greter M, Becher B. The CNS mononuclear phagocyte system in health and disease. Neuron (2022) 110:3497–512. doi: 10.1016/j.neuron.2022.10.005
18. Razavi S-M, Lee KE, Jin BE, Aujla PS, Gholamin S, Li G. Immune evasion strategies of glioblastoma. Front Surg (2016) 3:11. doi: 10.3389/fsurg.2016.00011
19. Wei J, Wu A, Kong L-Y, Wang Y, Fuller G, Fokt I, et al. Hypoxia potentiates glioma-mediated immunosuppression. PloS One (2011) 6:e16195. doi: 10.1371/journal.pone.0016195
20. White K, Connor K, Meylan M, Bougoüin A, Salvucci M, Bielle F, et al. Identification, validation and biological characterization of novel glioblastoma tumour microenvironment subtypes: implications for precision immunotherapy. Ann Oncol (2023) 34:300–14. doi: 10.1016/j.annonc.2022.11.008
21. Wang Q, Hu B, Hu X, Kim H, Squatrito M, Scarpace L, et al. Tumor evolution of glioma-intrinsic gene expression subtypes associates with immunological changes in the microenvironment. Cancer Cell (2017) 32:42–56.e6. doi: 10.1016/j.ccell.2017.06.003
22. Kirchhammer N, Trefny MP, Auf der Maur P, Läubli H, Zippelius A. Combination cancer immunotherapies: emerging treatment strategies adapted to the tumor microenvironment. Sci Transl Med (2022) 14:eabo3605. doi: 10.1126/scitranslmed.abo3605
23. Bejarano L, Jordāo MJC, Joyce JA. Therapeutic targeting of the tumor microenvironment. Cancer Discovery (2021) 11:933–59. doi: 10.1158/2159-8290.CD-20-1808
24. Di Ianni N, Maffezzini M, Eoli M, Pellegatta S. Revisiting the immunological aspects of temozolomide considering the genetic landscape and the immune microenvironment composition of glioblastoma. Front Oncol (2021) 11:747690. doi: 10.3389/fonc.2021.747690
25. Kelly WJ, Gilbert MR. Glucocorticoids and immune checkpoint inhibitors in glioblastoma. J Neurooncol (2021) 151:13–20. doi: 10.1007/s11060-020-03439-2
26. Ohm JE, Gabrilovich DI, Sempowski GD, Kisseleva E, Parman KS, Nadaf S, et al. VEGF inhibits T-cell development and may contribute to tumor-induced immune suppression. Blood (2003) 101:4878–86. doi: 10.1182/blood-2002-07-1956
27. Gabrilovich DI, Chen HL, Girgis KR, Cunningham HT, Meny GM, Nadaf S, et al. Production of vascular endothelial growth factor by human tumors inhibits the functional maturation of dendritic cells. Nat Med (1996) 2:1096–103. doi: 10.1038/nm1096-1096
28. Awada G, Salama LB, Cremer JD, Schwarze JK, Fischbuch L, Seynaeve L, et al. Axitinib plus avelumab in the treatment of recurrent glioblastoma: a stratified, open-label, single-center phase 2 clinical trial (GliAvAx). J ImmunoTher Cancer (2020) 8:e001146. doi: 10.1136/jitc-2020-001146
29. Nayak L, Molinaro AM, Peters K, Clarke JL, Jordan JT, de Groot J, et al. Randomized phase II and biomarker study of pembrolizumab plus bevacizumab versus pembrolizumab alone for patients with recurrent glioblastoma. Clin Cancer Res (2021) 27:1048–57. doi: 10.1158/1078-0432.CCR-20-2500
30. Cloughesy T, Wen PY, Prins RM. Neoadjuvant anti-PD-1 immunotherapy promotes a survival benefit with intratumoral and systemic immune responses in recurrent glioblastoma. Nat Med (2019) 25:477–86. doi: 10.1038/s41591-018-0337-7
31. Schalper KA, Rodriguez-Ruiz ME, Diez-Valle R, López-Janeiro A, Porciuncula A, Idoate MA, et al. Neoadjuvant nivolumab modifies the tumor immune microenvironment in resectable glioblastoma. Nat Med (2019) 25:470–6. doi: 10.1038/s41591-018-0339-5
32. Hervey-Jumper SL, Zhang Y, Phillips JJ, Morshed RA, Young JS, McCoy L, et al. Interactive effects of molecular, therapeutic, and patient factors on outcome of diffuse low-grade glioma. J Clin Oncol (2023) 41:2029–42. doi: 10.1200/JCO.21.02929
33. Karschnia P, Young JS, Dono A, Häni L, Sciortino T, Bruno F, et al. Prognostic validation of a new classification system for extent of resection in glioblastoma: A report of the RANO resect group. Neuro Oncol (2023) 25(5):940–54. doi: 10.1093/neuonc/noac193/6664041
34. Coffey JC, Wang JH, Smith MJF, Bouchier-Hayes D, Cotter TG, Redmond HP. Excisional surgery for cancer cure: therapy at a cost. Lancet Oncol (2003) 4:760–8. doi: 10.1016/S1470-2045(03)01282-8
35. Bakos O, Lawson C, Rouleau S, Tai L-H. Combining surgery and immunotherapy: turning an immunosuppressive effect into a therapeutic opportunity. J Immunother Cancer (2018) 6:86. doi: 10.1186/s40425-018-0398-7
36. Alexander BM, Cloughesy TF. Adult glioblastoma. J Clin Oncol (2017) 35:2402–9. doi: 10.1200/JCO.2017.73.0119
37. Parkins CC, McAbee JH, Ruff L, Wendler A, Mair R, Gilbertson RJ, et al. Mechanically matching the rheological properties of brain tissue for drug-delivery in human glioblastoma models. Biomaterials (2021) 276:120919. doi: 10.1016/j.biomaterials.2021.120919
38. Wait SD, Prabhu RS, Burri SH, Atkins TG, Asher AL. Polymeric drug delivery for the treatment of glioblastoma. Neuro Oncol (2015) 17:ii9–ii23. doi: 10.1093/neuonc/nou360
39. D’Amico RS, Aghi MK, Vogelbaum MA, Bruce JN. Convection-enhanced drug delivery for glioblastoma: a review. J Neurooncol (2021) 151:415–27. doi: 10.1007/s11060-020-03408-9
40. Vogelbaum MA. Personalized therapeutic delivery in the neurosurgical operating room. Proc Natl Acad Sci U S A (2018) 115:8846–8. doi: 10.1073/pnas.1812559115
41. Desjardins A, Gromeier M, Herndon JE 2nd, Beaubier N, Bolognesi DP, Friedman AH, et al. Recurrent glioblastoma treated with recombinant poliovirus. N Engl J Med (2018) 379:150–61. doi: 10.1056/NEJMoa1716435
42. Lang FF, Conrad C, Gomez-Manzano C, Yung WKA, Sawaya R, Weinberg JS, et al. Phase I study of DNX-2401 (Delta-24-RGD) oncolytic adenovirus: replication and immunotherapeutic effects in recurrent malignant glioma. J Clin Oncol (2018) 36:1419–27. doi: 10.1200/JCO.2017.75.8219
43. Cloughesy TF, Petrecca K, Walbert T, Butowski N, Salacz M, Perry J, et al. Effect of vocimagene amiretrorepvec in combination with flucytosine vs standard of care on survival following tumor resection in patients with recurrent high-grade glioma: a randomized clinical trial. JAMA Oncol (2020) 6:1939–46. doi: 10.1001/jamaoncol.2020.3161
44. Omuro A, Vlahovic G, Lim M, Sahebjam S, Baehring J, Cloughesy T, et al. Nivolumab with or without ipilimumab in patients with recurrent glioblastoma: results from exploratory phase 1 cohorts of CheckMate 143. Neuro Oncol (2017) 20:674–86. doi: 10.1093/neuonc/nox208
45. Duerinck J, Schwarze JK, Awada G, Tijtgat J, Vaeyens F, Bertels C, et al. Intracerebral administration of CTLA-4 and PD-1 immune checkpoint blocking monoclonal antibodies in patients with recurrent glioblastoma: a phase I clinical trial. J Immunother Cancer (2021) 9(6):e002296. doi: 10.1136/jitc-2020-002296
46. Schwarze JK, Tijtgat J, Awada G, Cras L, Vasaturo A, Bagnall C, et al. Intratumoral administration of CD1c (BDCA-1)+ and CD141 (BDCA-3)+ myeloid dendritic cells in combination with talimogene laherparepvec in immune checkpoint blockade refractory advanced melanoma patients: a phase I clinical trial. J Immunother Cancer (2022) 10(9):e005141. doi: 10.1136/jitc-2022-005141
Keywords: glioblastoma, immune therapy, immune checkpoint inhibition, local administration, surgery
Citation: Duerinck J, Tuyaerts S, Movahedi K and Neyns B (2023) Overcoming the immune suppressive nature of glioblastoma by leveraging the surgical intervention - current status and future perspectives. Front. Immunol. 14:1183641. doi: 10.3389/fimmu.2023.1183641
Received: 10 March 2023; Accepted: 08 May 2023;
Published: 19 May 2023.
Edited by:
Christine E. Engeland, Witten/Herdecke University, GermanyReviewed by:
Peng Zhang, Northwestern University, United StatesCopyright © 2023 Duerinck, Tuyaerts, Movahedi and Neyns. This is an open-access article distributed under the terms of the Creative Commons Attribution License (CC BY). The use, distribution or reproduction in other forums is permitted, provided the original author(s) and the copyright owner(s) are credited and that the original publication in this journal is cited, in accordance with accepted academic practice. No use, distribution or reproduction is permitted which does not comply with these terms.
*Correspondence: Johnny Duerinck, am9obm55LmR1ZXJpbmNrQHV6YnJ1c3NlbC5iZQ==