- 1Department of Oral and Maxillofacial Surgery, West China Hospital of Stomatology, Sichuan University, Chengdu, China
- 2State Key Laboratory of Oral Diseases, West China College of Stomatology, Sichuan University, Chengdu, China
- 3Department of Prosthodontics, West China Hospital of Stomatology, Sichuan University, Chengdu, China
- 4State Key Laboratory of Oral Diseases, National Center of Stomatology, National Clinical Research Center for Oral Diseases, Frontier Innovation Center for Dental Medicine Plus, West China Hospital of Stomatology, Sichuan University, Chengdu, China
Tumor-associated macrophages (TAMs) play a critical role in supporting tumor growth and metastasis, taming host immunosurveillance, and augmenting therapeutic resistance. As the current treatment paradigms for cancers are generally insufficient to exterminate cancer cells, anti-cancer therapeutic strategies targeting TAMs have been developed. Since TAMs are highly heterogeneous and the pro-tumoral functions are mediated by phenotypes with canonical surface markers, TAM-associated materials exert anti-tumor functions by either inhibiting polarization to the pro-tumoral phenotype or decreasing the abundance of TAMs. Furthermore, TAMs in association with the immunosuppressive tumor microenvironment (TME) and tumor immunity have been extensively exploited in mounting evidence, and could act as carriers or accessory cells of anti-tumor biomaterials. Recently, a variety of TAM-based materials with the capacity to target and eliminate cancer cells have been increasingly developed for basic research and clinical practice. As various TAM-based biomaterials, including antibodies, nanoparticles, RNAs, etc., have been shown to have potential anti-tumor effects reversing the TME, in this review, we systematically summarize the current studies to fully interpret the specific properties and various effects of TAM-related biomaterials, highlighting the potential clinical applications of targeting the crosstalk among TAMs, tumor cells, and immune cells in anti-cancer therapy.
Introduction
It has been illustrated that tumor initiation and progression are not only related to the genomic changes of cancerous cells, but are also affected by the tumor microenvironment (TME) (1, 2). Tumor associated macrophages (TAMs), as a key regulator in TMEs, are made up of a mix of tissue resident and exudative macrophages in varying proportions based on the type, location, and stage of the tumor (3). Typically, TAMs can be designated as M1- and M2-polarized macrophages. M1 macrophages produce pro-inflammatory cytokines, including interleukin‐1β (IL‐1β), IL‐6, IL‐12, tumor necrosis factor‐α (TNF‐α), and interferon‐γ (IFN‐γ), etc., which activate host immune responses against microbes and viruses, subsequently leading to the suppression of tumor progression (4). While M2 macrophages secrete anti‐inflammatory cytokines, including IL‐10, IL‐13, and transforming growth factor‐β (TGF‐β), exerting the promotion of cancer occurrence and development (5). However, there are still some situations where M2 macrophages might be an inhibitor in tumor progression. For instance, Rakaee et al. provided evidence showing that high level of CD204+/CD68+ M2 macrophages would be an independent positive prognostic marker of prolonged survival in lung cancer (6), indicating the exact function of the TAMs highly depends on cellular phenotype. To support this notion, in our preliminary study, we found that oral cancer metastasis in clodronate-treated mice was not significantly reduced by M1/2 macrophage reduction (7), suggesting that TAMs with specific surface markers, instead of the board M1/2 macrophages, exert various cellular functions in cancers. Although the controversy of TAMs subtypes in tumor biological behaviors, TAMs are still extensively studied and regarded as a great potential target. Importantly, various TAMs-related materials have been created for anti-tumor therapy not only in the basic research but also in the pre-clinical settings.
Biological materials based on TAMs have recently been divided into three main aspects as follows: materials targeting TAMs directly, and those targeting TAMs indirectly through cancer cells and through immune cells (8, 9). An increasing number of studies of biological materials targeting TAMs have focused on experimental and pre-clinical anti-tumor approaches with encouraging signs; however, there are still a number of challenges to overcome before they can be employed in clinical practice. For instance, materials aiming at TAMs polarization might be oversimplified and problematic, as TAMs cannot be readily split into M1/M2 macrophages due to the existence of more nuanced phenotypes (10, 11). The cellular marker cluster of differentiation (CD68) has been widely used as a pan-macrophage marker in most studies; however, it has been reported that CD68 occasionally expressed in dendric cells, stromal cells, even cancer cells (12), indicating that any biological materials targeting CD68+ TAMs might be off-target. Furthermore, there are still some obstacles to the optimization of biocompatibility and efficacy in the application of nanomaterials targeting TAMs. Thus, to comprehensively understand the current progress of TAMs based on materials, in this review, we systematically summarized the design and application of materials based on TAMs from various routes and targets, providing new insights for anti-cancer therapeutic avenues.
Materials targeting tumor-associated macrophages directly
TAM phenotypic heterogeneity and its relationship with materials application
TAMs are highly heterogeneous stromal cells, and these distinct phenotypic characteristics are well established by techniques including immunohistochemistry, flow cytometry, single-cell sequencing, etc. Recently, it has been proposed that tissue macrophages arise from not only blood monocytes, but also the embryonic precursors deriving from the yolk sac and/or fetal liver (13, 14). The differentiated cell type’s chromatin landscape, among other epigenomic traits, represents the macrophage developmental origins (15), indicating that epigenetic modification and ontogeny can influence its identity development and thus dictate phenotypic heterogeneity. Furthermore, the TME in different cancers could also significantly alter macrophages phenotypes in distinct anatomical regions. For instance, TAMs are formed of a heterogeneous population of macrophages in hepatocellular carcinoma and breast cancer (16, 17). Interestingly, even in the same TME, the majority of the TAMs population differs in phenotype, which is related to the distances between cells, and systemic toxicity might result if all types of TAMs are targeted. To support this, using mass cytometry with extensive antibody panels, Chevrier et al. found that there were 17 unique macrophage phenotypes in the TME of human renal cell carcinoma, and even that the same type of macrophage not only expressed the CD169 (as an anti-tumorigenic marker), but also co-expressed with pro-tumorigenic markers including CD163, CD206, etc. (10), suggesting that the application of targeting materials should focus on, or be aware of, the most important subsets and paradoxical behaviors to optimize the therapeutic efficacy. Another potential hypothesis for the different phenotypic heterogeneity in the same type of tumor is that the environment caused by distinct sites might modulate macrophage phenotypes. For instance, in gastric cancer, Huang et al. provided data showing that CD68+IRF8+ macrophages dominated in the closest sites to the cancer cells, while the CD68+CD163+CD206+ macrophages dominated in the furthest sites (18), demonstrating the macrophages marker expression differences in tumor areas. Due to heterogeneity, TAMs release different mediators: either anti-tumor, including IL-6, IL-1β, chemokine (C-C motif) ligand 2 (CCL2), and TNF, or pro-tumor, including IL-10, TGF-β, vascular endothelial growth factor (VEGF), platelet-derived growth factor (PDGF), CCL17, CCL22, and CCL24 (19, 20). By using the monoclonal and specific antibodies for cellular markers, various cytokines and transcriptional profiles have been utilized for targeting TAMs (21). In summary, the phenotypic heterogeneity of TAMs depends on cellular origins, cancer type, and cellular distribution in the TME, etc., and with the discovery of more specific markers, we will be able to more accurately identify the subpopulations and develop the targeting materials for anti-cancer therapy.
TAM co-culture systems and their relationship with materials application
To monitor the biological behavior of TAMs, co-culture systems, which are necessary in recreating TMEs, could provide a promising human in vivo-like tissue model, including two-dimensional (2D) and three‐dimensional (3D) cell cultures (Figure 1A). In vitro 2D cell cultures, such as Transwell inserts with Matrigel, have been widely used to explore the polarization and pertinent signaling pathways of TAMs in vitro (22). However, limited by physical structure and components, existing 2D models might remodel cells and their internal cytoskeleton, and affect cell arrangements on a flat substrate (23), making the exploration of cell performance and the simulation of natural environments in vivo difficult. Thus, 3D co-culture systems have arisen to mimic the situation in vivo, in which the spatial organization of cells is more reliable for the physiological relevance of experiments. At present, the types of 3D co-culture models related to TAMs can mainly be classified into spheroids, scaffold-based models, and microfluidic-based 3D models (Figure 1A). The 3D multi-cellular spheroid model, using the ‘hanging drop’ approach, or aggregate cultures with a matrix construction including heterogeneous populations of cells could be devised with hypoxia and necrotic patches to imitate tumor features in vivo (24, 25), leading to better comprehension of TAM performance as influenced by the reactive oxygen species (ROS) and hypoxic region in the TME. Regarding scaffold-based models, a large number of either organic or inorganic matrices and scaffolds have been employed to mimic the extracellular matrix due to good biocompatibility. For instance, Matrigel is composed of a reconstituted basement membrane extract (BME) secreted by a mouse sarcoma, and the natural scaffolds consists of purified proteins such as type I collagen, while artificial scaffolds are made up of polyethylene glycol (PEG)-based hydrogels and synthetic alternatives to Matrigel (26). Additionally, microfluidics, with high spatial controllability, can support short-term culture, and are usually designed with a different number of chambers and lateral channels. For example, utilizing the adjacent gel channels in a microfluidic device to explore paracrine signals, Huang et al. found that the macrophages invaded the gels composed of type I collagen and Matrigel, and transformed into distinct phenotypes when cultured next to the cancer cells (27). Together, although the 3D in vitro models have been widely employed to mimic in vivo settings for more closely analyzing the cell-to-cell crosstalk and the organoid, etc., the inner cells in the sphere models might not be flourished by angiological system like them in human tumors. In the spheroids, cells locating in different zones exhibit different proliferation, and the tumor cells in the core exhibited quiescent due to the limited oxygen and nutrient delivery (28). Unlike this, the inner cells in human tumors could be flourished by vascular and lymphatic vessels, even the specific vasculogenic mimicry (VM) which was defined as a fluid conducting channel embedded in extracellular matrix to feed tumor cells (29). Of note, the majority of macrophages originate from monocytes in blood and affiliate in the perivascular lesions; thus, one of the challenges for 3D co-culture systems for macrophages is to create microcirculation in the 3D culture models and locate the macrophages beside extravascular sites.
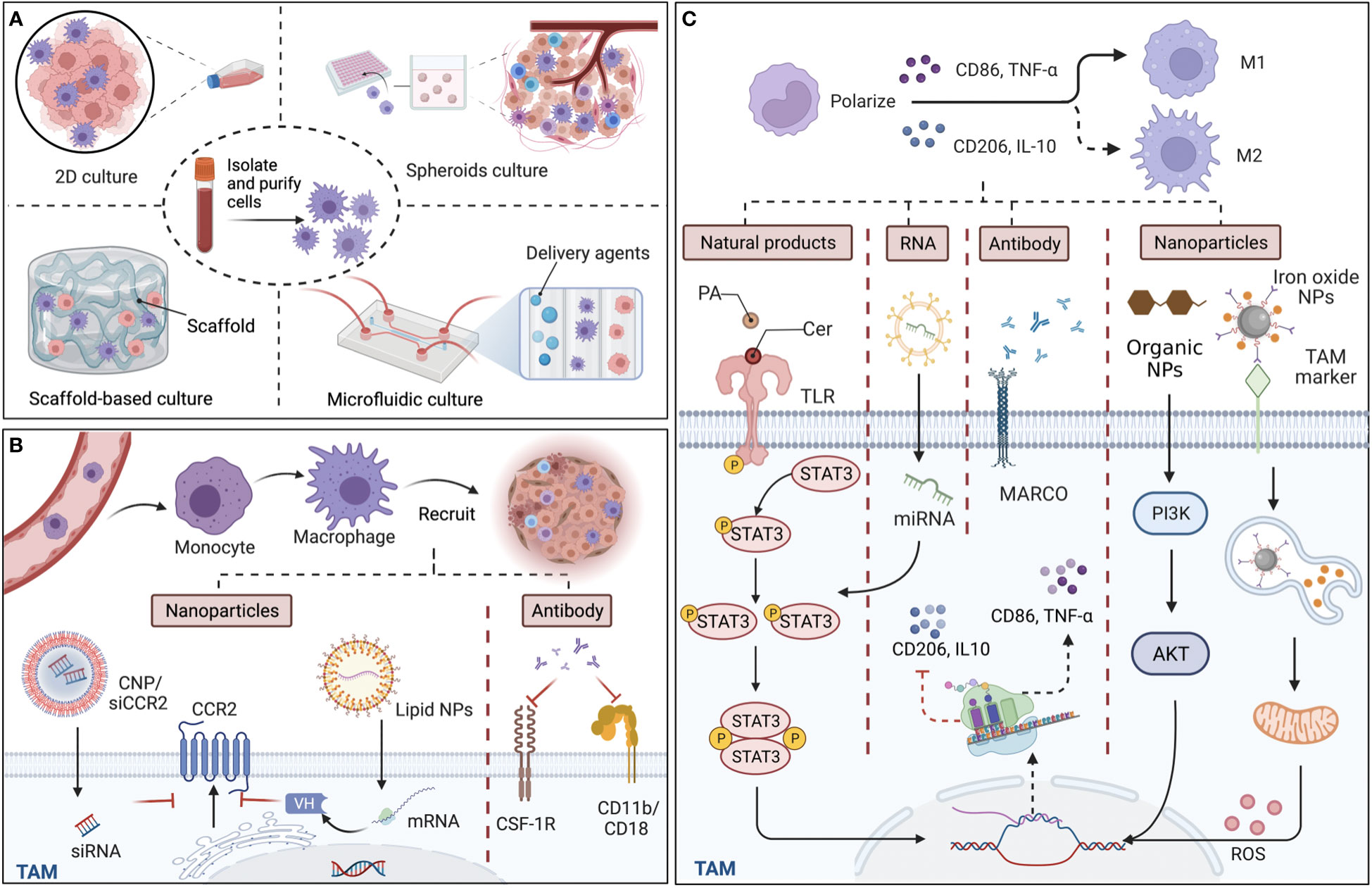
Figure 1 Culture models in vitro for tumor-associated macrophages (TAMs) and targeted approaches for TAM recruitment and polarization. (A) The structure of main TAM co-culture systems. Compared with two-dimensional (2D) cultures, which traditionally cultivate macrophages and cancer cells on a plane, 3D culture models including spheroids, scaffold-based models, and microfluidic-based models are more accurate in reproducing cell and tissue physiology. (B) TAMs derive from monocytes and recruit into tumor sites guided by various chemokines and cytokines. By targeting chemokine (C-C motif) receptor 2 (CCR2), colony-stimulating factor 1 receptor (CSF-1R), and CD11b/CD18, nanoparticles (NPs), including siRNA-coated NPs (CNP/siCCR2) and lipid NPs, and antibodies can effectively reduce TAM infiltration and reshape immunosuppressive tumor microenvironments. (C) Since M1 macrophages are typically identified by the secretion of surface markers CD86 and tumor necrosis factor-α (TNF-α), while M2 macrophages are identified by CD206 and interleukin‐10 (IL‐10), the main mechanism of the various materials that affect the polarization of TAMs is interference with the expression of the relevant markers. Some natural ingredients, such as ceramide (Cer) and palmitic acid (PA), could act as agonists of toll-like receptors (TLRs), efficiently modulating the STAT3 signaling pathways and polarizing TAMs to the M1 phenotype. Regarding artificial ingredients, exosomal miRNA, which affects the diverse signaling pathways for the expression of markers, monoclonal antibodies (mcAbs) against MARCO receptor, which is expressed by M2 macrophages can repolarize M2 back to TAMs, and NPs, modulate related signaling pathways and reactive oxygen species (ROS) release, exhibiting with efficacy in cancer therapy. Back arrows: promotion; Red “T” arrows: inhibition.
TAM recruitment and its relationship with materials application
Monocytes and monocyte-related myeloid-derived suppressor cells (M-MDSCs) are the main precursors of TAMs, which are recruited to the tumor site under the guidance of a variety of chemical attractants and influence tumor progression; thus, various materials targeting TAM recruitment have been created for anti-cancer therapy (Figure 1B). Since the process is influenced by chemokines, such as CCL2, colony-stimulating factor 1 (CSF-1), chemokine (C-X-C motif) ligand 12 (CXCL12), etc., and cytokines, including IL-6, IL-8, IL-34, and members of the VEGF family, blocking the related signaling pathways with nanoparticles (NPs) or specific antibodies is an effective way to affect TAM recruitment from both an epigenetic and molecular level. Taking CCL2-CCR2 signaling pathways as an example, Shen et al. found that prepared siRNA-coated NPs (CNP/siCCR2) effectively inhibited CCR2 expression and blocked the recruitment of monocytes to the tumor site, leading to reduced tumor metastasis (30). Despite the encapsulation of direct effectors, lipid NPs could also load mRNA, which could translate a single-domain antibody (VH) as bispecific CCL2/CCL5 inhibitors (BisCCL2/5i), reversing the infiltration of TAMs in the TME (31). In another blocking approach, monoclonal antibodies (mcAbs) targeting signals in TAM recruitment have also been developed as anti-tumor therapy by binding to and neutralizing the surface markers, and then modulating TAM recruitment with the suppression of adhesion and migration to the vascular endothelium and chemotaxis, including anti-CSF-1R mcAbs (32) and neutralizing CD11b mcAbs, which could also act as adjuvants to radiotherapy (33). Therefore, targeting the specific signaling pathways of monocytes can effectively inhibit TAM recruitment, reduce TAM infiltration in solid tumors, and reshape the immunosuppressive TME, while the protection of monocytes that derived to macrophages in normal tissues is worth noting in the process. To support this, Mehta et al. provided data demonstrating that anti-CSF-1R antibodies influenced monocyte/macrophage migration in triplenegative breast cancer (34). In summary, compared with injecting antibodies themselves, mRNA-loaded NPs, which allow the generation of immunotherapeutic proteins in the TAM nucleus and cytoplasm, are equipped with a specific signaling peptide at the N-terminal end of BisCCL2/5i and secrete to the TME effectively, thus taking effect at lower doses and reducing possible systemic toxicity. Furthermore, to maximize the efficacy of agents, which are widely distributed, preferential aggregation in myeloid cells around bone marrow and peripheral blood is the potential area of inquiry taking advantage of the properties of materials.
TAM polarization and its relationship with materials application
Characterized by different surface markers, secretions, and functions, the polarization state of TAMs is not constant as a result of plasticity, which transforms towards the M1 phenotype for pro-inflammation and anti-tumor effects, while alternatively to M2 exerting anti-inflammation and pro-tumor functions. At present, the strategy of targeting TAM polarization is primarily accomplished by regulating signals with agonists and inhibitors, etc. (Table 1). With regard to agent type, biological materials are mainly composed of natural and artificial ingredients, including RNA, antibodies, NPs, etc. (Figure 1C). For instance, lipids in natural ceramide (Cer) and palmitic acid (PA) could bind to TLR (toll-like receptor) and cause M2 phenotype repolarization, subsequently inhibiting human colon cancer invasion (39). Since these natural lipids are non-toxic, highly concentrated in vivo, and easily isolated from plasma membrane, powerful properties might be further explored in blocking TAM polarization for anti-cancer therapy. Artificial materials such as exosomal miRNAs, which could influence the production of target mRNAs by binding to their 3′untranslated regions (3′UTRs), utilize transcription factors including PPARs, STAT3/6, etc., and induce TAM repolarization at the transcriptional level. For instance, Ying et al. showed that exosomal miR-222-3p promoted M2 macrophage polarization by the SOCS3/STAT3 pathway in ovarian cancer (50). Furthermore, mcAbs based on blocking TAM polarization could interact with related receptors, directly affecting tumor progression. For example, MARCO expressed by M2 macrophages and the injection of its specific antibody (ED31) decreased tumor growth and metastasis in vivo by polarizing TAMs to the M1 subtype (51). Importantly, organic NPs, RP6530 (C23H18FN5O2), a novel PI3Kδ/γ inhibitor with nanomolar inhibitory potency, suppressed tumor growth by downregulating the limiting glycolytic enzyme PKM2 and repolarizing M2 (44), indicating that kinases in signaling pathways related to macrophage phenotypic polarization can also be targeted. Different from influencing TAMs themselves, inorganic NPs, such as ferumoxytol and calcium carbonate NPs, can mediate the repolarization of TAMs by regulating the ROS and acidity of the TME. For instance, Zanganeh et al. found that, with the increase in M1, ferumoxytol significantly inhibited the growth of subcutaneous adenocarcinoma in mice (52). Notably, due to the limitations of inorganic materials’ own properties on the human body in that iron over-exposure might increase the incidence of cancer by increasing oxidative stress and DNA damage, the application needs to pay strict attention to the dosage and timing. On the whole, therapeutic strategies targeting TAM polarization processes mainly include the modulation of gene expression, surface receptors, and the TME, among which organic NPs targeting enzymes or signaling pathways might be more effective without reliance on FcR-like antibodies.
Materials based on tumor-associated macrophages for targeting cancer cells
The application of TAM-related antibodies for targeting cancer cells
Therapeutic antibodies can stimulate immune-mediated tumor cell death by engaging innate immune cell lineages or activating complement cascades once they have been bound to cell surface antigens. As immune effectors, macrophages can not only express antibody fragment crystallizable receptors (FcR), but also crosstalk with tumor cells through the efficiency of various antibodies as they could directly execute antibody-dependent cell-mediated cytotoxicity (ADCC) and antibody-dependent cellular phagocytosis (ADCP), as well as complement-dependent cytotoxicity (CDC). TAMs in the TME are crucial to increase the effectiveness of antibody-mediated cancer immunotherapy, and the functions of TAMs have been proven to contribute to mcAbs’ effectiveness in vitro, in vivo, and in clinical trials (Table 2). According to the structural and functional differences, antibodies can be divided into IgA, IgD, IgE, IgG, and IgM, among which IgG is the most commonly used mcAb, exerting therapeutic actions by attracting cells with FcγR family members, including FcγR I, FcγR II, and FcγR III, or blocking the molecular signal (73). The interaction between FcγR on macrophages and immune complexes leads to enhanced phagocytosis and the release of superoxide, TNF, tissue plasminogen activator, prostaglandins, and leukotrienes (74), which further modulates tumor development. To fully engage TAMs, improvements in binding efficacy with antibodies could be carried out by changing Fc fragments with genetic modification by splicing with overlap extension (75), and protein modification by modulating Fc regions to be fucosylation-free (76). Except for genetic and protein engineering, remodeled antibodies are applied in anti-cancer therapy, including antibody fragments, bispecific antibodies (bsAbs), and antibody-drug conjugates (ADCs) (Figure 2A).
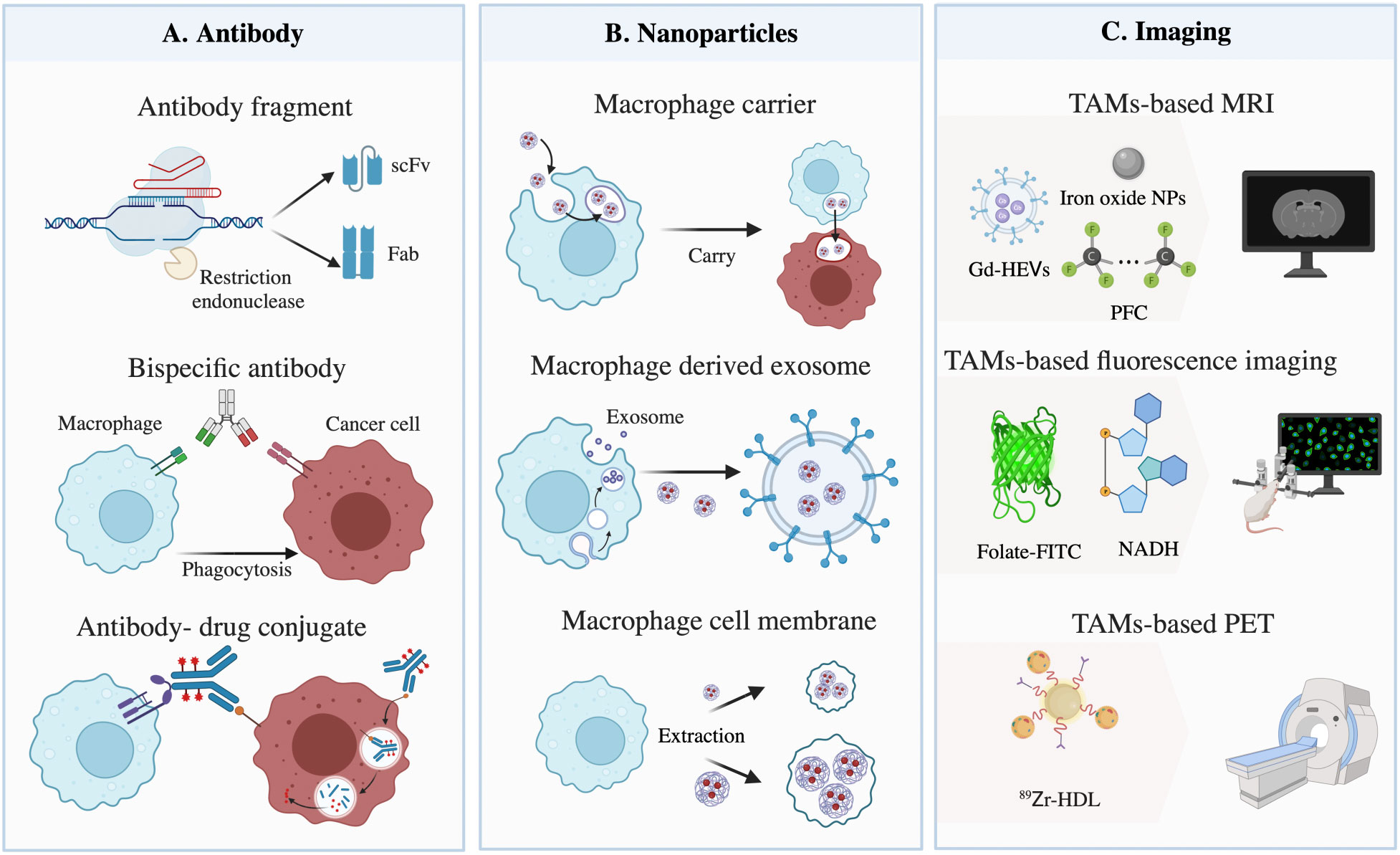
Figure 2 Materials based on tumor-associated macrophages (TAMs) for targeting cancer cells. (A) Illustration of restructured antibody targeting TAMs and cancer cells. The method mainly consists of three parts: antibody fragments, bispecific antibodies, and antibody-drug conjugates. (B) Macrophages, exosomes, and cell membranes act as nanoparticle carriers to target tumors and induce phagocytosis. (C) Materials used for TAM-based imaging, composed of gadolinium (Gd) with extracellular vesicles (Gd-HEVs), iron oxide nanoparticles (NPs), and perfluorocarbon compounds (PFCs) for magnetic resonance imaging (MRI), folate-conjugated fluorescein isothiocyanate (folate-FITC) and nicotinamide adenine dinucleotide (NADH) for fluorescence imaging, and 89Zr-high-density lipoprotein (HDL) for positron emission tomography (PET).
The molecular weight of the Fab fragment obtained by restriction endonuclease digestion is equivalent to 1/3 of the intact antibody. The molecular weight of the single-chain variable fragment (scFv) or single domain antibody (VH, VL nanobody) prepared by genetic engineering is even smaller, composed of approximately 120 amino acids and equivalent to 1/6 or 1/12 of the intact antibody, respectively, thus improving its penetration of solid tumors (77). For instance, chimeric antigen receptor (CAR) macrophage therapy could target cancer cells based on CD3ζ, which is homologous to the FcϵRI-γ, a canonical signaling molecule for ADCP, and repolarize M2 macrophages, therefore promoting antigen-specific phagocytosis and tumor clearance in vitro (78). Notably, while designing, appropriate miniaturization should be carried out according to its pharmacokinetic characteristics, to avoid weakening the affinity between antibody and target antigens, and reducing the clinical effects owing to the decrease in half-life.
BsAbs are roughly classified into fragment-based bsAbs, composed of two scFvs, and Fc-based bsAbs, with Fc domains linked to Fab or scFv, which could target tumor antigens and conjunct with macrophages or other types of antigenic epitopes at the same time, enhancing binding efficacy and anti-tumor specificity. For instance, Chen et al. provided data demonstrating that bsAbs targeted FcγR and stimulated innate and adaptive responses in the TME, leading to the polarization and phagocytosis of TAMs (79). In addition, compared with Fc-based bsAbs, the former has a lower molecular weight, indicating better penetration in solid tumors and faster clearance. Moreover, catumaxomab, a trifunctional antibody (trAb), can bind three different cell types: tumor cells, T-cells, and accessory cells (80), broadening our horizons in the design of antibodies. Although this method offers great potentials, the affinity and stability of bsAbs are influenced by the properties of additional linkers, which need to be taken into consideration.
Acting as another class of highly promising antibody-based therapeutics, ADCs are composed of an antibody targeting cancer cell-specific antigens, a cytotoxic drug, and a chemical linker that connects the drug and the antibody. Cancer targets for approved ADCs vary, such as CD33 for acute myeloid leukemia (81), and HER2 for lung adenocarcinoma (82). When mentioned drugs, pyrrolobenzodiazepine (PBD) dimers, a type of anti-tumor/antibiotic natural chemical generated by actinomycetes, are the most potent class of cytotoxic drugs, followed by maytansinoids, auristatins, and calicheamicin, all of which have similar activity (83). Specifically, in order to improve macrophage phagocytosis, materials conjugated to the linkers could also be replaced by immunostimulatory drugs, including TLR agonists, scavenger receptor ligands, proinflammatory cytokines, chemokines, etc. For instance, Dela Cruz et al. showed that, compared with the unmodified antibody, an anti-HER2 antibody fused with granulocyte-macrophage colony-stimulating factor (GM-CSF), a cytokine associated with an increased expression of major histocompatibility complex class II (MHC-II) on monocytes, was more stable in the blood and more effective in activating TAMs, leading to an enhanced anti-tumor response (84). Of note, before the internalization of ADCs, extracellular enzymes released by surrounding cancer cells and TAMs could cleave diffusible drugs, resulting in surrounding ‘bystander’ cell death. Thus, antibody specificity, payload drug cytotoxicity, linker stability, and cleavage should be fully considered when designing ADCs.
The application of nanoparticles based on TAM carriers for targeting cancer cells
NPs with a small size and diverse surface characteristics are proper materials for penetrating poorly vascularized and fibrotic tumors. At present, NPs targeting cancer cells, in mainstream design, are based on enhanced permeation and retention (EPR), due to the leaky vasculature and poor drainage of solid tumors. Recent research has revealed that most NPs enter the tumor site through endothelial cells actively instead of inter-endothelial gaps passively (85). Thus, taking advantage of preferential aggregation within macrophages after systemic delivery and the strong infiltration potential of myeloid cells, the loading of NPs into monocytes/macrophages in the blood circulation system can be employed to facilitate drug release in the tumor’s bulk. Furthermore, TAMs can cross the blood-brain barrier (BBB) and infiltrate further to the hypoxic TME, driven by oxygen gradients and signal pathways without reliance on the EPR effect (86). Studies on NPs drug delivery systems use a variety of materials, including biological carriers, viral particles, carbon nanotubes, albumin NPs, etc. (Table 3), among which liposomes, polymeric NPs, and iron oxide NPs are the most commonly used. Moreover, as a component of Live Cell-mediated Drug Delivery Systems (LCDDS), M1 macrophages, which can target tumors and promote inflammation in the TME, act as drug carriers with either the complete or chosen essential components, including exosomes and external cellular membranes (105) (Figure 2B).
Anti-cancer drugs engulfed in macrophages-loaded NPs can reduce toxicity and increase the loading ratio. Notably, the phagosomes of macrophages might cause degradation of the drugs and affect the functions of TAMs, thus there are accumulating studies aimed at addressing and solving these defects. For instance, in a mouse model of pulmonary metastases, Kim et al. developed the lipid–NPs conjugates and incubated them into the macrophage membrane to encourage the hydrophobic compound to conjugate with TAMs effectively and stably for anti-tumor therapy (106). Recently, genetic engineering, surface modification, and cellular backpacks have been able to modulate the function of macrophages, enhancing the infiltration of solid tumors. For example, as CRISPR-Cas9 gene-editing technology could influence the expression of surface markers, macrophages showed a four-fold increase in the elimination of cancer cells with arginine NPs (107). Through cell surface modification, hybridizing macrophages with NPs that provide numerous new sites for anti-cancer drug loading could decrease the toxic effect on macrophage carriers (108). Furthermore, polymer patches, as phagocytosis-resistant backpacks, conjugated to the surface of macrophages and crossed the BBB without changing the TAMs functions, including targeting and phagocytosis ability, and the properties of the loaded particles (109). In summary, these data suggest that modulating the contact area and enhancing bonding strength could further ensure stability when penetrating the solid tumor.
With a diameter of 40-160 nm and a specific content of RNA, proteins, and other compounds, macrophage-derived exosomes are derived from the invagination of the cellular membrane and processed into mature multivesicular bodies (MVBs) in the cytoplasm (110, 111). Exosomes, especially M1 macrophage-derived exosomes (M1-exos), inherit similar surface membrane properties from macrophages and can be employed to deliver various anti-tumor components via crosstalk between TAMs and cancer cells. For instance, Harney et al. found that in nude mice with triple negative breast cancer (TNBC) cells, M1-exos loaded with paclitaxel (PTX) or doxorubicin (DOX) could target cancer cells and enhance the anti-tumor effects (112). Since exosomes are a highly heterogeneous population of membrane vesicles and no specific biomarkers have yet been confirmed, the isolation and purification of M1-exos are challenging, and can be resolved by developing exosome-mimetic vesicles or proper genetic engineering. It is worth noting that THP-1-derived exosomes are sensitive to extracellular stimulation, including lipopolysaccharide (LPS) (113) and IL-4 (114), by modulating inherent exosomal contents, and have a relatively fixed size, which could influence the stability and selection of loading NPs.
Unlike exosomes, membrane-coated NPs without other contents are flexible in size and are unacted upon by the surrounding status, leading to an effective and stable therapeutic effect with a sufficient drug-loading capacity and nanosize. Purified macrophage membranes, obtained from disrupted cells by centrifugation, can coat NPs by a direct extrusion method, and have favorable biocompatibility and tumor-homing ability in systemic circulation. For instance, Cao et al. showed that using macrophage membranes to coat liposomes loaded with cytotoxic anti-cancer drugs could increase the cellular uptake of NPs and effectively inhibit lung metastasis, owing to the interaction between integrins on membranes and VCAM-1 on cancer cells, as well as CCR2 and CCL2 (115). However, since membrane vesicles without an intact signaling axis need to be engulfed by cancer cells for drug delivery, the membrane at this time inhibits NP release when accumulating in the TME. To support this, Zhang et al. found that modulating NP properties for effective membrane escape was necessary with pH-sensitive polymer, taking advantage of the pH difference between the TME and vesicles to influence the water influx, expansion, and eruption of membrane coating (116).
Materials based on TAMs for tumor imaging
At present, TAM-based imaging has been performed in magnetic resonance imaging (MRI), fluorescent imaging, and positron emission tomography (PET) for research investigation in cancers (Figure 2C). Since the high-pressure properties of hypoxia TME restrict the effective aggregation and tissue penetration of materials targeting cancer cells directly (117), TAMs, which have high phagocytic ability and a wide distribution in tumor sites, can guarantee the relevant ingredients enrichment. In this case, by observing the difference in the number and phenotype of TAMs, TAM-based imaging can not only be useful for tumor diagnosis and prognosis, but also for addressing the edge of tumors for surgery. For instance, macrophage-specific radiotracers or probes can be designed as antibodies with TAM-specific biomarkers, folate (M1) and mannose receptor (M2), coupled to signal probes of magnetic resonance agents and radionuclides, or TAM-related NPs with signal-giving moieties (118), suggesting that using TAM-based materials to transmit signal compositions of imaging is a promising strategy.
MRI contrast agents (Cas), including gadolinium (Gd), iron oxide NPs, and fluorine 19 (19F), are critical in obtaining accurate contrast-enhanced anatomical images. Due to the rapid elimination of Gd through renal metabolism, Rayamajhi et al. found that macrophages could act as Gd carriers with cell-derived hybrid extracellular vesicles (Gd-HEVs) to prolong the retention time (119). As Gd exerts nephrogenic systemic fibrosis (NSF) toxicity, interestingly, intravenously injected iron oxide NPs are preferentially phagocyted by macrophages to decrease toxicity. For instance, ferumoxytol, a type of iron oxide NPs for macrophages, caused tumor enhancement on postcontrast scans by MRI image due to the existence of CD68 and CD163 TAMs (120). However, since iron oxide NPs can also be phagocyted by neutrophils and other phagocytic cells, the accuracy and specificity of these imaging techniques need to be improved. Notably, 19F, as perfluorocarbon compounds (PFCs), can be delivered intravenously in the same way as iron oxide NPs, and can image macrophages better due to its low background. To support this notion, Khurana et al. showed a strong association between the quantity of 19F atoms and the number of macrophages after PFC injection (121), and 19F accuracy is more reliable than ferumoxytol (122).
Fluorescence imaging can be used for cancer imaging by detecting TAMs. Using knock-in mice whose macrophages were marked with fluorescent dextran, various populations of myeloid-derived cells showed different biological behaviors and were distributed in different parts of the tumor (123), indicating that monitoring macrophages using fluorescence imaging is feasible. On account of the unique overexpression of folate receptor (FR)-β+ in TAMs, folate-conjugated fluorescein isothiocyanate (folate-FITC) could be utilized for the precise optical detection of tumors by targeting M1 macrophages (124). More experiments are needed for conventional exogenous fluorophores before clinical applications. In addition, endogenous fluorescence metabolites, including nicotinamide adenine dinucleotide (NADH) and flavin adenine dinucleotide (FAD), involved in glycolysis and cellular respiration are sources of autofluorescence signals and can also be used to quantify TAMs. For instance, Szulczewski et al. discovered that macrophages accounted for almost 75% of the cells with elevated FAD inside a mammary cancer mouse model (125). In summary, since fluorescence imaging reports changes in TME longitudinally over time and reflects the immediate and divergent therapy effects, the half-time of materials influenced by the renal route of excretion should be fully considered.
Although PET can monitor a high enrichment of nanotracers non-invasively, limited data have supported that TAM-related NPs are good choices for cancer PET imaging. Different PET tracers might be valuable for monitoring TAM immunology by appropriately integrating with NPs. For instance, Pérez-Medina et al. performed an analysis of 89Zr-PL-high-density lipoprotein (HDL) and 89Zr-AI-HDL, which can be contained by TAMs and act as delivery cargo, to assess the burden of TAMs in vivo using PET imaging (126). However, HDL also targets other immune cells (127), indicating that the accuracy of TAM-related NPs in PET imaging needs to be further explored. Taken together, the evidence suggests that TAM-based tracers in MRI, fluorescence imaging, and PET highlight potential avenues for cancer diagnosis and therapy. Despite focusing on TAMs themselves, designing materials to detect TAM-derived secretions might be a potential orientation of imaging. In an alternative approach, combining MRI with fluorescence imaging or PET for particular macrophage polarizations might increase the precision of MRI based on TAMs.
Materials based on tumor-associated macrophages for targeting immune cells
Except for cancer cells and TAMs, the TME is composed of fibroblasts, endothelial cells, various types of immune cells, etc. In addition, adaptive immune cells (T cells and B cells) and innate immune cells, including macrophages, neutrophils, dendritic cells (DC), and natural killer (NK) cells, also influence the occurrence and development of tumors. Participating in innate and adaptive immunity, macrophages, as mediators, play an important role in regulating the immune response to tumors (Figure 3).
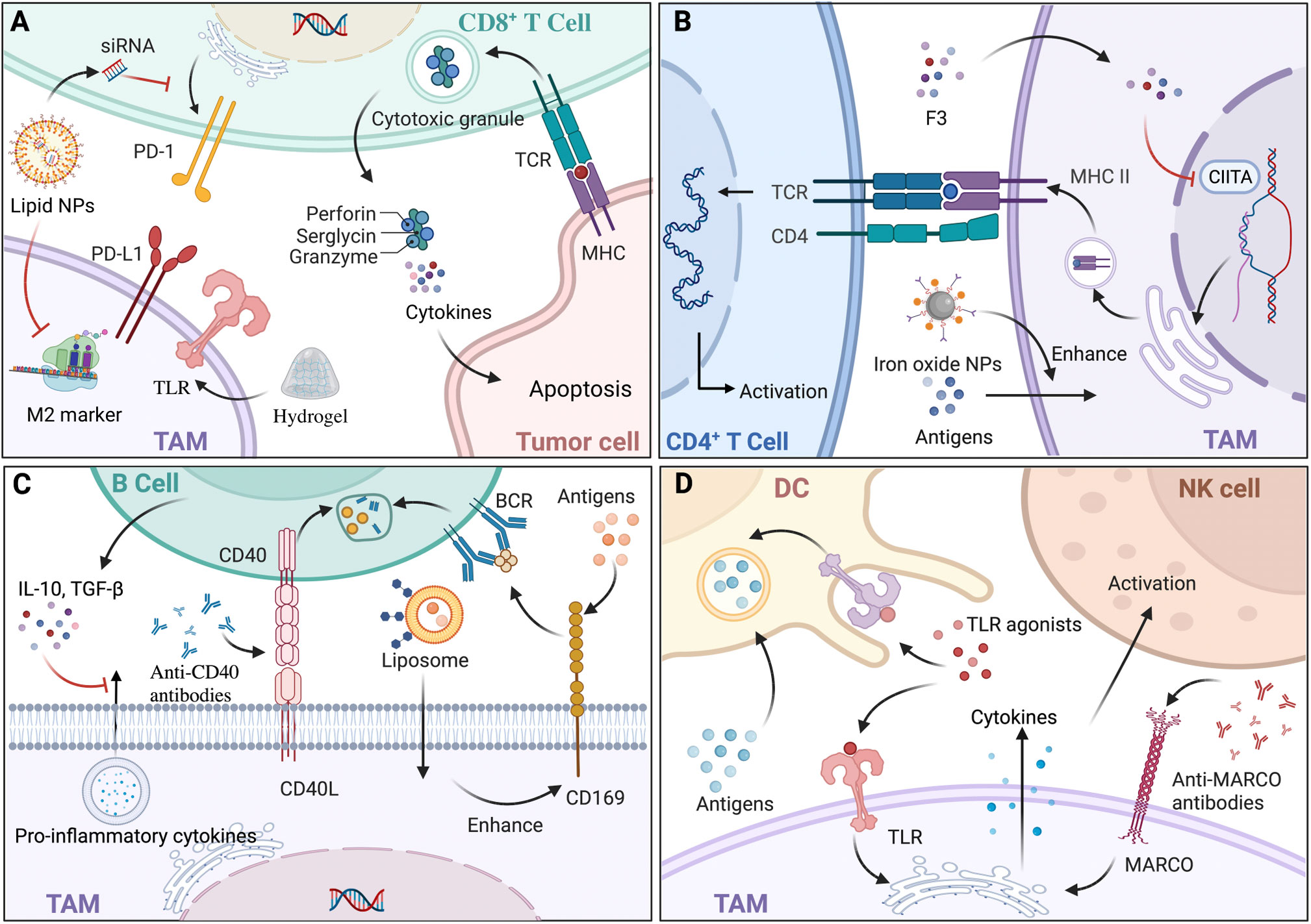
Figure 3 The interaction and materials based on tumor-associated macrophages (TAMs) to target immune cells. (A) CD8+ T cells induce tumor cell apoptosis mainly through the release of cytokines and the exocytosis of granules containing perforin and granzyme. Hydrogel, a type of nanoparticle (NP), loaded with antigens can activate CD8+ T cells indirectly with the help of macrophages expressing toll-like receptors (TLRs). In addition, lipid NPs loaded with siRNA could specifically block the M2-marker expression of TAMs and lead to decreased expression of PD-1. (B) TAMs expressing major histocompatibility complex class II (MHC-II) present tumor antigens and activate CD4+ T cells with T cell receptor (TCR). There are two ways to fully boost CD4+ T cells, one of which is modulating MHC-II expression by epigenetic silencing with chromatin modifiers, including S. crispus leaves (F3), that can inhibit CIITA transcription; the other is enhancing antigens presentation and MHC-II pathway by iron oxide NPs. (C) Regulatory B (Breg) cells secrete interleukin‐10 (IL‐10) and transforming growth factor‐β (TGF‐β), decreasing pro-inflammatory cytokine secretion of TAMs, which modulate the biological behavior of tumors. Since CD169+ macrophages capture antigens and present to B cell receptor (BCR) on B cells, liposomal NPs with glycan ligands were preferentially phagocytized to modulate B cells indirectly. Anti-CD40 antibodies acted on CD40-CD40L pathways with a greater upregulation of anti-tumor activity. (D) TAMs transfer antigens to dendritic cells (DCs) by CD169 for immunotherapy. Since TLRs exist on both DCs and TAMs, TLR agonists could enhance antigens presentation of DCs and pro-inflammation cytokines’ secretion of TAMs, leading to the immunostimulatory tumor microenvironment (TME). Via the increase of cytokines, such as IL-15, anti-MARCO antibodies activate natural killer (NK) cells. Back arrows: promotion; Red “T” arrows: inhibition.
CD8+ T cells and their application in TAM-related materials
The crosstalk between CD8+ T cells and macrophages undoubtedly exacerbates the immunological escape of cancer cells and has a negative effect on cancer immunotherapy. CD8+ T cells differentiate into cytotoxic T lymphocytes (CTLs) and CTLs exert anti-tumor functions in three ways: physical contact with cancer cells via intracellular signal activation, the release of cytokines, and exocytosis of granules containing perforin and granzyme (128). By stimulation of TNF‐α and IFN‐γ secreted from M1 macrophages, CD8+ T cells transformed into a cytotoxic phenotype with upregulated PD-1 expression (129). In contrast, influenced by IL-10 and TGF‐β secreted by M2 macrophages, CD8+ T cells were kept away from cancer cells and their immune activity was attenuated, resulting in the immunological escape of cancer cells (130). Thus, TAM-related materials including microparticles (MPs), NPs, and RNAs could be equipped with the ability to impact the infiltration of CD8+ T cells to affect anti-tumor immunity (Figure 3A). Mannose-modified macrophage-derived MPs equipped with metformin (Met@Man-MPs) can polarize TAMs to M1 phenotype and remodel the TME by recruiting CTLs through the degradation of collagen fiber (131). While MPs with a particle size of 100-1000 nm limited tissue biodistribution, it is suggested that developing MPs with a narrower size or nanosize would be ideal for the delivery of drugs. To support this, Muraoka et al. found that NPs could activate CD8+ T cells indirectly through the TLRs expressed by macrophages (132). In another study, conjugated with recombinant ovalbumin (OVA), gold NPs with a diameter of 10-22 nm, which were engulfed by macrophages, exhibited greater anti-tumor efficacy with the further infiltration of CD8+ T cells (133), indicating that the immune response of NPs would also be mediated by particle size. Additionally, TAMs targeting NPs with RNAs would decrease T cells apoptosis and enhance CTL activation by either influencing the IL-6 and IL-10 secretion of TAMs (134) or modulating the amount of diverse TAM phenotypes causing CTL infiltration (46). Notably, challenges still exist regarding NPs with RNAs for delivery blockade in terms of digestion by various enzymes, unreachability to target cells, and endocytosis before proper degradation. To sum up, since CD8+ T cells exert tumor elimination by a relatively direct method, TAM-related materials as drug adjuvants or carriers for the further infiltration of CD8+ T cells have great potential to ensure essential contact with cancer cells.
CD4+ T cells and their application in TAM-related materials
CD4+ T cells differentiate into various subtypes to participate in immunity, among which T-helper 1 (Th1) and Th2 account for the majority. Considered as the most essential helper cell type for cancer immunity, Th1 cells eliminate cancer cells indirectly with the help of MHC-II positive macrophages (135), and Th2 cells exert anti-tumor functions by inducing inflammation in TME with the participation of M2 macrophages (136). Given that TAMs in TME act as APCs expressing MHC-II to present tumor antigens, TAM-related materials are promising in activating CD4+ T cells (Figure 3B). For instance, Rong et al. developed iron-chelated melanin-like NPs (Fe@PDA-PEG) to promote TAM polarization and the recruitment of Th cells by high efficacy in capture, phago-endocytosis, processing, and the presentation of tumor-associated antigens (TAAs), subsequently leading to suppressed tumor progression (137). As MHC-II expression can be influenced by its transactivator (CIITA) (138), it indicates that materials targeting CIITA transcriptional activity is potential by affecting promoter selection, mRNA stability, and post-translational modification. For example, Yankuzo et al. discovered a bioactivator of S. crispus leaves (F3) by facilitating IFN-γ production in the tumor site, which could induce CD4+ T cell infiltration and increase CIITA and MHC-II expression of cancer cells with the attenuation of CD68+ TAMs (139). Notably, without the interaction between CD4+ T cells and TAMs, IFN-γ itself could not modulate TAMs for tumor elimination (140), indicating that anti-tumor materials based on cytokines should fully consider the crosstalk among immune cells and can be integrated with specific agents to mimic the essential signaling pathways. In summary, the evidence suggests that TAM-related materials could be conducted by epigenetic modulations targeting MHC-II and accessory genes to guarantee antigen presentation to CD4+ T cells efficiently and to increase the abundance of CD4+ T cells for sufficient interaction with macrophages.
B cells and their application in TAM-related materials
Tumor-infiltrating B lymphocytes (TIBs) with a strong capacity for immune responses are crucial in the TME for activating T cell responses and causing cancer cell death, while regulatory B (Breg) cells decrease the pro-inflammatory cytokine secretion of macrophages and promote tumor progression (141) (Figure 3C). In inflammatory tumor tissue, CD169+ macrophages, which capture intact antigens and preserve on their surface for a long time, cross-present TAAs to TIBs, and in turn, B cells secrete neurotransmitter GABA (γ-aminobutyric acid), which could increase the ratio of anti-inflammatory macrophages (142). For the sake of convenience in further exploring the interactions between TAMs and B cells, peritoneal cavity (PerC) cell culture serves as an ideal in vitro system, as it concludes most B cell subtypes (143). At present, materials that modulate B cells mainly act on either macrophage, which could affect TIB indirectly or the signaling pathways between them (Figure 3C). For instance, Chen et al. showed that newly designed liposomal NPs with glycan ligands, which were affiliative to CD169, could effectively deliver antigens to CD169+ macrophages (144), modulating B cell biological behavior indirectly. While it is remarkable that using glycan is lacking in specificity, developing ligands targeting an exclusive receptor might enhance anti-tumor therapy. Regarding signaling pathways, in preclinical studies, anti-CD40 IgG1 mcAbs exerted strong anti-tumor activity with a greater upregulation of activation markers on B cells by modulating CD40-CD40L interaction between macrophages and B cells, leading to higher efficacy of antigen presentation and immune response (145). Similarly, Bruhns et al. found that IgG1 was more appropriate for immunostimulatory activities compared with the other IgG antibodies (146). Further, since FcγRIIB is the only FcγR on B cells, upregulation of FcγRIIB might improve the efficacy of the specific mcAbs. Thus, materials aiming at gene modification have potential by influencing receptor expression or lipid rafts incorporation for the essential distribution of FcγRIIB.
DCs and NK cells and their application in TAM-related materials
DCs exert anti-tumor effects via TAA uptake, processing, and presentation, which display an extensive dysfunctional status under the influence of TAMs secreting immunosuppressive factors (147). To solve the numerical and functional DC deficiencies, TAM-related materials, which can promote DC activation and reverse the immunosuppressive TME, are potential treatment modalities (Figure 3D). For instance, TLR agonists could act as therapeutic materials, since TLR can not only stimulate DC maturation, antigen presentation, and cytotoxicity towards tumor cells (148), but also activate M1 macrophages to secrete pro-inflammation cytokines and create a potent immunostimulatory microenvironment (149). To date, three TLR agonists have been developed for tumor therapy in a clinical setting: imiquimod as a TLR7 agonist for the treatment of superficial basal cell carcinoma, Bacillus Calmette-Guérin (BCG) as a TLR2, TLR3 and TLR9 agonist for non-invasive transitional cell carcinoma of bladder therapy, and monophosphoryl lipid A (MPLA) as a TLR4 agonist for the human papillomavirus (HPV) vaccine to prevent cervical cancer (150). Of note, since DCs would rather phagocytize particles with a diameter of 20–200 nm (151), the drug size should be taken into consideration for the design of materials. Additionally, NK cells exhibiting a quick response to infections and malignancies are crucial in innate immunity, and can be activated by M1 macrophages secreting IL-12, and suppressed by M2 subsets secreting TGF-β (152). Similar to DCs, TAM-based materials act on NK cells by modulating related cytokines to mediate immunosuppression in the TME. For instance, Eisinger et al. found that performing an anti-MARCO antibody on TAMs could activate NK cells to inhibit tumor growth by modulating the IL-15 secretion of macrophages, a cytokine known to support NK cell proliferation, infiltration, and cytotoxic capacity (153). In addition, as macrophage subtypes have their own distinct effects on NK cells, materials targeting TAM polarization could indirectly modulate the biological behaviors of NK cells and cytokines in the TME (154). Together, compared with the materials acting on DCs and NK cells directly which might induce possible excessive immune activation because of targeting DCs and NK cells without specificity of distribution (155), and lead to off-target and normal tissue injury (156), TAM-related biomaterials would reverse the immunosuppressive TME and restore normal immunity to exert more effective anti-tumor functions.
Conclusions
In this review, we conclude the specific properties and various effects of TAM-based materials from bench to clinic. Targeting diverse crosstalk among TAMs, cancer cells, and immune cells, a variety of biomaterials with TAMs as accessory cells or carriers could modulate TAMs biological behaviors, exert tumor elimination and imaging, and engage adaptive and innate immunity for anti-tumor therapy. However, there are some limitations of these materials that need to be overcome.
Firstly, highly heterogeneous macrophages exhibit various immune responses to TAM-related materials of deficient specificity, which can easily lead to off-target and unstable anti-tumor effects. Hence, it is necessary to further investigate TAMs subtypes differentiation, design specific targeting molecules and develop more efficient tumor diagnostic and therapeutic modalities.
Secondly, since most research on biomaterials effects is conducted in vivo, data from mouse models require expanded studies to verify accuracy and biocompatibility for clinical application. It would be feasible to define a ‘TAM atlas’ of human solid tumors and compare it with mice data using emerging technologies, including tissue mass spectrometry, single-cell RNA sequencing (scRNA-Seq), and multiplex immunofluorescence, to predict immunotherapy treatment prognosis (157).
Thirdly, therapeutic strategies targeting macrophages might have potential adverse reactions. For instance, CSF-1R inhibitors can reduce the number of TAMs in vivo, but unexpectedly recruit a large number of immunosuppressive granulocytes (158). Furthermore, depletion of CD169+ macrophages can inhibit tumor growth and increase the infiltration of CD8+ T cells in the TME, unfortunately doing harm to bone homeostasis and bone marrow erythropoiesis (159). Thus, TAM-targeted therapy needs to further explore adverse reactions and the mechanisms of the drugs, possibly combining with other agents to reduce systemic toxicity.
Author contributions
QG and FW conceived and designed the study. FJ drafted the manuscript. FJ, XL, XC and FW searched and reviewed the literatures, and made the figures and tables. All of the authors critically reviewed and revised the manuscript. All authors have read and agreed to the published version of the manuscript.
Funding
This research was funded by the National Natural Science Foundation of China (No. 82002884), the National Key Research and Development Program of China (No. 2020YFA0714001), Sichuan Science and Technology Program (No. 2015JY0046), Science and Technology Program of Chengdu City (No. 2021-YF05-02031-SN) and the Undergraduate Innovation and Entrepreneurship Training Program of Sichuan University (No. 2022120939).
Acknowledgments
A part of the figures were created with BioRender.com. Due to space limitations, we apologize for not being able to cite and discuss all relevant work from different laboratories published to date.
Conflict of interest
The authors declare that the research was conducted in the absence of any commercial or financial relationships that could be construed as a potential conflict of interest.
Publisher’s note
All claims expressed in this article are solely those of the authors and do not necessarily represent those of their affiliated organizations, or those of the publisher, the editors and the reviewers. Any product that may be evaluated in this article, or claim that may be made by its manufacturer, is not guaranteed or endorsed by the publisher.
References
1. Ovais M, Mukherjee S, Pramanik A, Das D, Mukherjee A, Raza A, et al. Designing stimuli-responsive upconversion nanoparticles that exploit the tumor microenvironment. Adv Mater (Deerfield Beach Fla) (2020) 32(22):e2000055. doi: 10.1002/adma.202000055
2. Thakkar S, Sharma D, Kalia K, Tekade RK. Tumor microenvironment targeted nanotherapeutics for cancer therapy and diagnosis: A review. Acta Biomater (2020) 101:43–68. doi: 10.1016/j.actbio.2019.09.009
3. Inagaki K, Kunisho S, Takigawa H, Yuge R, Oka S, Tanaka S, et al. Role of tumor-associated macrophages at the invasive front in human colorectal cancer progression. Cancer Sci (2021) 112(7):2692–704. doi: 10.1111/cas.14940
4. Chen Y, Jin H, Song Y, Huang T, Cao J, Tang Q, et al. Targeting tumor-associated macrophages: A potential treatment for solid tumors. J Cell Physiol (2021) 236(5):3445–65. doi: 10.1002/jcp.30139
5. Zeng Z, Liu Y, Wen Q, Li Y, Yu J, Xu Q, et al. Experimental study on preparation and anti-tumor efficiency of nanoparticles targeting M2 macrophages. Drug Deliv (2021) 28(1):943–56. doi: 10.1080/10717544.2021.1921076
6. Rakaee M, Busund LR, Jamaly S, Paulsen EE, Richardsen E, Andersen S, et al. Prognostic value of macrophage phenotypes in resectable non-small cell lung cancer assessed by multiplex immunohistochemistry. Neoplasia (New York NY) (2019) 21(3):282–93. doi: 10.1016/j.neo.2019.01.005
7. Wu FL, Nolan K, Strait AA, Bian L, Nguyen KA, Wang JH, et al. Macrophages promote growth of squamous cancer independent of T cells. J Dental Res (2019) 98(8):896–903. doi: 10.1177/0022034519854734
8. Jahchan NS, Mujal AM, Pollack JL, Binnewies M, Sriram V, Reyno L, et al. Tuning the tumor myeloid microenvironment to fight cancer. Front Immunol (2019) 10:1611. doi: 10.3389/fimmu.2019.01611
9. Yang M, McKay D, Pollard JW, Lewis CE. Diverse functions of macrophages in different tumor microenvironments. Cancer Res (2018) 78(19):5492–503. doi: 10.1158/0008-5472.can-18-1367
10. Chevrier S, Levine JH, Zanotelli VRT, Silina K, Schulz D, Bacac M, et al. An immune atlas of clear cell renal cell carcinoma. Cell (2017) 169(4):736–49.e18. doi: 10.1016/j.cell.2017.04.016
11. Xue J, Schmidt SV, Sander J, Draffehn A, Krebs W, Quester I, et al. Transcriptome-based network analysis reveals a spectrum model of human macrophage activation. Immunity (2014) 40(2):274–88. doi: 10.1016/j.immuni.2014.01.006
12. Vakkila J, Lotze MT, Riga C, Jaffe R. A basis for distinguishing cultured dendritic cells and macrophages in cytospins and fixed sections. Pediatr Dev Pathol (2005) 8(1):43–51. doi: 10.1007/s10024-004-5045-2
13. Lahmar Q, Keirsse J, Laoui D, Movahedi K, Van Overmeire E, Van Ginderachter JA. Tissue-resident versus monocyte-derived macrophages in the tumor microenvironment. Biochim Biophys Acta (2016) 1865(1):23–34. doi: 10.1016/j.bbcan.2015.06.009
14. Wynn TA, Chawla A, Pollard JW. Macrophage biology in development, homeostasis and disease. Nature (2013) 496(7446):445–55. doi: 10.1038/nature12034
15. Lavin Y, Winter D, Blecher-Gonen R, David E, Keren-Shaul H, Merad M, et al. Tissue-resident macrophage enhancer landscapes are shaped by the local microenvironment. Cell (2014) 159(6):1312–26. doi: 10.1016/j.cell.2014.11.018
16. Zhang Q, He Y, Luo N, Patel SJ, Han Y, Gao R, et al. Landscape and dynamics of single immune cells in hepatocellular carcinoma. Cell (2019) 179(4):829–45.e20. doi: 10.1016/j.cell.2019.10.003
17. Wagner J, Rapsomaniki MA, Chevrier S, Anzeneder T, Langwieder C, Dykgers A, et al. A single-cell atlas of the tumor and immune ecosystem of human breast cancer. Cell (2019) 177(5):1330–45.e18. doi: 10.1016/j.cell.2019.03.005
18. Huang YK, Wang M, Sun Y, Di Costanzo N, Mitchell C, Achuthan A, et al. Macrophage spatial heterogeneity in gastric cancer defined by multiplex immunohistochemistry. Nat Commun (2019) 10(1):3928. doi: 10.1038/s41467-019-11788-4
19. Wan S, Kuo N, Kryczek I, Zou W, Welling TH. Myeloid cells in hepatocellular carcinoma. Hepatol (Baltimore Md) (2015) 62(4):1304–12. doi: 10.1002/hep.27867
20. Capece D, Fischietti M, Verzella D, Gaggiano A, Cicciarelli G, Tessitore A, et al. The inflammatory microenvironment in hepatocellular carcinoma: a pivotal role for tumor-associated macrophages. BioMed Res Int (2013) 2013:187204. doi: 10.1155/2013/187204
21. Gordon S, Taylor PR. Monocyte and macrophage heterogeneity. Nat Rev Immunol (2005) 5(12):953–64. doi: 10.1038/nri1733
22. Maolake A, Izumi K, Shigehara K, Natsagdorj A, Iwamoto H, Kadomoto S, et al. Tumor-associated macrophages promote prostate cancer migration through activation of the CCL22-CCR4 axis. Oncotarget (2017) 8(6):9739–51. doi: 10.18632/oncotarget.14185
23. Knight E, Przyborski S. Advances in 3D cell culture technologies enabling tissue-like structures to be created in vitro. J Anat (2015) 227(6):746–56. doi: 10.1111/joa.12257
24. Costa EC, Moreira AF, de Melo-Diogo D, Gaspar VM, Carvalho MP, Correia IJ. 3D tumor spheroids: an overview on the tools and techniques used for their analysis. Biotechnol Adv (2016) 34(8):1427–41. doi: 10.1016/j.biotechadv.2016.11.002
25. Däster S, Amatruda N, Calabrese D, Ivanek R, Turrini E, Droeser RA, et al. Induction of hypoxia and necrosis in multicellular tumor spheroids is associated with resistance to chemotherapy treatment. Oncotarget (2017) 8(1):1725–36. doi: 10.18632/oncotarget.13857
26. Aisenbrey EA, Murphy WL. Synthetic alternatives to matrigel. Nat Rev Mater (2020) 5(7):539–51. doi: 10.1038/s41578-020-0199-8
27. Huang CP, Lu J, Seon H, Lee AP, Flanagan LA, Kim HY, et al. Engineering microscale cellular niches for three-dimensional multicellular co-cultures. Lab Chip (2009) 9(12):1740–8. doi: 10.1039/b818401a
28. Edmondson R, Broglie JJ, Adcock AF, Yang L. Three-dimensional cell culture systems and their applications in drug discovery and cell-based biosensors. Assay Drug Dev Technol (2014) 12(4):207–18. doi: 10.1089/adt.2014.573
29. Mao JM, Liu J, Guo G, Mao XG, Li CX. Glioblastoma vasculogenic mimicry: signaling pathways progression and potential anti-angiogenesis targets. biomark Res (2015) 3:8. doi: 10.1186/s40364-015-0034-3
30. Shen S, Zhang Y, Chen KG, Luo YL, Wang J. Cationic polymeric nanoparticle delivering CCR2 siRNA to inflammatory monocytes for tumor microenvironment modification and cancer therapy. Mol Pharmaceut (2018) 15(9):3642–53. doi: 10.1021/acs.molpharmaceut.7b00997
31. Wang Y, Tiruthani K, Li S, Hu M, Zhong G, Tang Y, et al. mRNA delivery of a bispecific single-domain antibody to polarize tumor-associated macrophages and synergize immunotherapy against liver malignancies. Adv Mater (Deerfield Beach Fla) (2021) 33(23):e2007603. doi: 10.1002/adma.202007603
32. Dowlati A, Harvey RD, Carvajal RD, Hamid O, Klempner SJ, Kauh JSW, et al. LY3022855, an anti-colony stimulating factor-1 receptor (CSF-1R) monoclonal antibody, in patients with advanced solid tumors refractory to standard therapy: phase 1 dose-escalation trial. Investigational New Drugs (2021) 39(4):1057–71. doi: 10.1007/s10637-021-01084-8
33. Ahn GO, Tseng D, Liao CH, Dorie MJ, Czechowicz A, Brown JM. Inhibition of mac-1 (CD11b/CD18) enhances tumor response to radiation by reducing myeloid cell recruitment. Proc Natl Acad Sci United States America (2010) 107(18):8363–8. doi: 10.1073/pnas.0911378107
34. Mehta AK, Cheney EM, Hartl CA, Pantelidou C, Oliwa M, Castrillon JA, et al. Targeting immunosuppressive macrophages overcomes PARP inhibitor resistance in BRCA1-associated triple-negative breast cancer. Nat Cancer (2021) 2(1):66–82. doi: 10.1038/s43018-020-00148-7
35. Cavalcante RS, Ishikawa U, Silva ES, Silva-Júnior AA, Araújo AA, Cruz LJ, et al. STAT3/NF-κB signalling disruption in M2 tumour-associated macrophages is a major target of PLGA nanocarriers/PD-L1 antibody immunomodulatory therapy in breast cancer. Br J Pharmacol (2021) 178(11):2284–304. doi: 10.1111/bph.15373
36. Wanderley CW, Colón DF, Luiz JPM, Oliveira FF, Viacava PR, Leite CA, et al. Paclitaxel reduces tumor growth by reprogramming tumor-associated macrophages to an M1 profile in a TLR4-dependent manner. Cancer Res (2018) 78(20):5891–900. doi: 10.1158/0008-5472.can-17-3480
37. Wang D, Jiang W, Zhu F, Mao X, Agrawal S. Modulation of the tumor microenvironment by intratumoral administration of IMO-2125, a novel TLR9 agonist, for cancer immunotherapy. Int J Oncol (2018) 53(3):1193–203. doi: 10.3892/ijo.2018.4456
38. Wiehagen KR, Girgis NM, Yamada DH, Smith AA, Chan SR, Grewal IS, et al. Combination of CD40 agonism and CSF-1R blockade reconditions tumor-associated macrophages and drives potent antitumor immunity. Cancer Immunol Res (2017) 5(12):1109–21. doi: 10.1158/2326-6066.cir-17-0258
39. de Araujo Junior RF, Eich C, Jorquera C, Schomann T, Baldazzi F, Chan AB, et al. Ceramide and palmitic acid inhibit macrophage-mediated epithelial-mesenchymal transition in colorectal cancer. Mol Cell Biochem (2020) 468(1-2):153–68. doi: 10.1007/s11010-020-03719-5
40. Lian G, Chen S, Ouyang M, Li F, Chen L, Yang J. Colon cancer cell secretes EGF to promote M2 polarization of TAM through EGFR/PI3K/AKT/mTOR pathway. Technol Cancer Res Treat (2019) 18:1533033819849068. doi: 10.1177/1533033819849068
41. Zhang F, Parayath NN, Ene CI, Stephan SB, Koehne AL, Coon ME, et al. Genetic programming of macrophages to perform anti-tumor functions using targeted mRNA nanocarriers. Nat Commun (2019) 10(1):3974. doi: 10.1038/s41467-019-11911-5
42. Zhao J, Li H, Zhao S, Wang E, Zhu J, Feng D, et al. Epigenetic silencing of miR-144/451a cluster contributes to HCC progression via paracrine HGF/MIF-mediated TAM remodeling. Mol Cancer (2021) 20(1):46. doi: 10.1186/s12943-021-01343-5
43. Tan HY, Wang N, Man K, Tsao SW, Che CM, Feng Y. Autophagy-induced RelB/p52 activation mediates tumour-associated macrophage repolarisation and suppression of hepatocellular carcinoma by natural compound baicalin. Cell Death Dis (2015) 6(10):e1942. doi: 10.1038/cddis.2015.271
44. Locatelli SL, Careddu G, Serio S, Consonni FM, Maeda A, Viswanadha S, et al. Targeting cancer cells and tumor microenvironment in preclinical and clinical models of Hodgkin lymphoma using the dual PI3Kδ/γ inhibitor RP6530. Clin Cancer Res (2019) 25(3):1098–112. doi: 10.1158/1078-0432.ccr-18-1133
45. Sato-Kaneko F, Yao S, Ahmadi A, Zhang SS, Hosoya T, Kaneda MM, et al. Combination immunotherapy with TLR agonists and checkpoint inhibitors suppresses head and neck cancer. JCI Insight (2017) 2(18):e93397. doi: 10.1172/jci.insight.93397
46. Qian Y, Qiao S, Dai Y, Xu G, Dai B, Lu L, et al. Molecular-targeted immunotherapeutic strategy for melanoma via dual-targeting nanoparticles delivering small interfering RNA to tumor-associated macrophages. ACS Nano (2017) 11(9):9536–49. doi: 10.1021/acsnano.7b05465
47. Tsagozis P, Eriksson F, Pisa P. Zoledronic acid modulates antitumoral responses of prostate cancer-tumor associated macrophages. Cancer Immunol Immunother CII (2008) 57(10):1451–9. doi: 10.1007/s00262-008-0482-9
48. Di Caro G, Cortese N, Castino GF, Grizzi F, Gavazzi F, Ridolfi C, et al. Dual prognostic significance of tumour-associated macrophages in human pancreatic adenocarcinoma treated or untreated with chemotherapy. Gut (2016) 65(10):1710–20. doi: 10.1136/gutjnl-2015-309193
49. Huang Z, Yang Y, Jiang Y, Shao J, Sun X, Chen J, et al. Anti-tumor immune responses of tumor-associated macrophages via toll-like receptor 4 triggered by cationic polymers. Biomaterials (2013) 34(3):746–55. doi: 10.1016/j.biomaterials.2012.09.062
50. Ying X, Wu Q, Wu X, Zhu Q, Wang X, Jiang L, et al. Epithelial ovarian cancer-secreted exosomal miR-222-3p induces polarization of tumor-associated macrophages. Oncotarget (2016) 7(28):43076–87. doi: 10.18632/oncotarget.9246
51. Georgoudaki AM, Prokopec KE, Boura VF, Hellqvist E, Sohn S, Östling J, et al. Reprogramming tumor-associated macrophages by antibody targeting inhibits cancer progression and metastasis. Cell Rep (2016) 15(9):2000–11. doi: 10.1016/j.celrep.2016.04.084
52. Zanganeh S, Hutter G, Spitler R, Lenkov O, Mahmoudi M, Shaw A, et al. Iron oxide nanoparticles inhibit tumour growth by inducing pro-inflammatory macrophage polarization in tumour tissues. Nat Nanotechnol (2016) 11(11):986–94. doi: 10.1038/nnano.2016.168
53. Leidi M, Gotti E, Bologna L, Miranda E, Rimoldi M, Sica A, et al. M2 macrophages phagocytose rituximab-opsonized leukemic targets more efficiently than m1 cells in vitro. J Immunol (Baltimore Md 1950) (2009) 182(7):4415–22. doi: 10.4049/jimmunol.0713732
54. Tsao LC, Crosby EJ, Trotter TN, Agarwal P, Hwang BJ, Acharya C, et al. CD47 blockade augmentation of trastuzumab antitumor efficacy dependent on antibody-dependent cellular phagocytosis. JCI Insight (2019) 4(24):e131882. doi: 10.1172/jci.insight.131882
55. Ring NG, Herndler-Brandstetter D, Weiskopf K, Shan L, Volkmer JP, George BM, et al. Anti-SIRPα antibody immunotherapy enhances neutrophil and macrophage antitumor activity. Proc Natl Acad Sci United States America (2017) 114(49):E10578–e85. doi: 10.1073/pnas.1710877114
56. Li H, Somiya M, Kuroda S. Enhancing antibody-dependent cellular phagocytosis by re-education of tumor-associated macrophages with resiquimod-encapsulated liposomes. Biomaterials (2021) 268:120601. doi: 10.1016/j.biomaterials.2020.120601
57. Ye X, Li Y, Stawicki S, Couto S, Eastham-Anderson J, Kallop D, et al. An anti-axl monoclonal antibody attenuates xenograft tumor growth and enhances the effect of multiple anticancer therapies. Oncogene (2010) 29(38):5254–64. doi: 10.1038/onc.2010.268
58. Liu J, Xavy S, Mihardja S, Chen S, Sompalli K, Feng D, et al. Targeting macrophage checkpoint inhibitor SIRPα for anticancer therapy. JCI Insight (2020) 5(12):e134728. doi: 10.1172/jci.insight.134728
59. Kato Y, Tabata K, Kimura T, Yachie-Kinoshita A, Ozawa Y, Yamada K, et al. Lenvatinib plus anti-PD-1 antibody combination treatment activates CD8+ T cells through reduction of tumor-associated macrophage and activation of the interferon pathway. PloS One (2019) 14(2):e0212513. doi: 10.1371/journal.pone.0212513
60. Pienta KJ, Machiels JP, Schrijvers D, Alekseev B, Shkolnik M, Crabb SJ, et al. Phase 2 study of carlumab (CNTO 888), a human monoclonal antibody against CC-chemokine ligand 2 (CCL2), in metastatic castration-resistant prostate cancer. Investigational New Drugs (2013) 31(3):760–8. doi: 10.1007/s10637-012-9869-8
61. Ries CH, Cannarile MA, Hoves S, Benz J, Wartha K, Runza V, et al. Targeting tumor-associated macrophages with anti-CSF-1R antibody reveals a strategy for cancer therapy. Cancer Cell (2014) 25(6):846–59. doi: 10.1016/j.ccr.2014.05.016
62. Oflazoglu E, Stone IJ, Gordon KA, Grewal IS, van Rooijen N, Law CL, et al. Macrophages contribute to the antitumor activity of the anti-CD30 antibody SGN-30. Blood (2007) 110(13):4370–2. doi: 10.1182/blood-2007-06-097014
63. McEarchern JA, Oflazoglu E, Francisco L, McDonagh CF, Gordon KA, Stone I, et al. Engineered anti-CD70 antibody with multiple effector functions exhibits in vitro and in vivo antitumor activities. Blood (2007) 109(3):1185–92. doi: 10.1182/blood-2006-07-034017
64. Overdijk MB, Verploegen S, Bögels M, van Egmond M, Lammerts van Bueren JJ, Mutis T, et al. Antibody-mediated phagocytosis contributes to the anti-tumor activity of the therapeutic antibody daratumumab in lymphoma and multiple myeloma. mAbs (2015) 7(2):311–21. doi: 10.1080/19420862.2015.1007813
65. Kurdi AT, Glavey SV, Bezman NA, Jhatakia A, Guerriero JL, Manier S, et al. Antibody-dependent cellular phagocytosis by macrophages is a novel mechanism of action of elotuzumab. Mol Cancer Ther (2018) 17(7):1454–63. doi: 10.1158/1535-7163.mct-17-0998
66. Tai YT, Mayes PA, Acharya C, Zhong MY, Cea M, Cagnetta A, et al. Novel anti-b-cell maturation antigen antibody-drug conjugate (GSK2857916) selectively induces killing of multiple myeloma. Blood (2014) 123(20):3128–38. doi: 10.1182/blood-2013-10-535088
67. Tai YT, Horton HM, Kong SY, Pong E, Chen H, Cemerski S, et al. Potent in vitro and in vivo activity of an fc-engineered humanized anti-HM1.24 antibody against multiple myeloma via augmented effector function. Blood (2012) 119(9):2074–82. doi: 10.1182/blood-2011-06-364521
68. Advani R, Flinn I, Popplewell L, Forero A, Bartlett NL, Ghosh N, et al. CD47 blockade by Hu5F9-G4 and rituximab in non-hodgkin's lymphoma. New Engl J Med (2018) 379(18):1711–21. doi: 10.1056/NEJMoa1807315
69. Beatty GL, Chiorean EG, Fishman MP, Saboury B, Teitelbaum UR, Sun W, et al. CD40 agonists alter tumor stroma and show efficacy against pancreatic carcinoma in mice and humans. Sci (New York NY) (2011) 331(6024):1612–6. doi: 10.1126/science.1198443
70. Papadopoulos KP, Gluck L, Martin LP, Olszanski AJ, Tolcher AW, Ngarmchamnanrith G, et al. First-in-Human study of AMG 820, a monoclonal anti-Colony-Stimulating factor 1 receptor antibody, in patients with advanced solid tumors. Clin Cancer Res (2017) 23(19):5703–10. doi: 10.1158/1078-0432.ccr-16-3261
71. Vijayaraghavan S, Lipfert L, Chevalier K, Bushey BS, Henley B, Lenhart R, et al. Amivantamab (JNJ-61186372), an fc enhanced EGFR/cMet bispecific antibody, induces receptor downmodulation and antitumor activity by Monocyte/Macrophage trogocytosis. Mol Cancer Ther (2020) 19(10):2044–56. doi: 10.1158/1535-7163.mct-20-0071
72. Brana I, Calles A, LoRusso PM, Yee LK, Puchalski TA, Seetharam S, et al. Carlumab, an anti-C-C chemokine ligand 2 monoclonal antibody, in combination with four chemotherapy regimens for the treatment of patients with solid tumors: an open-label, multicenter phase 1b study. Targeted Oncol (2015) 10(1):111–23. doi: 10.1007/s11523-014-0320-2
73. Karagiannis SN, Josephs DH, Bax HJ, Spicer JF. Therapeutic IgE antibodies: Harnessing a macrophage-mediated immune surveillance mechanism against cancer. Cancer Res (2017) 77(11):2779–83. doi: 10.1158/0008-5472.can-17-0428
74. Baxter EW, Graham AE, Re NA, Carr IM, Robinson JI, Mackie SL, et al. Standardized protocols for differentiation of THP-1 cells to macrophages with distinct M(IFNγ+LPS), M(IL-4) and M(IL-10) phenotypes. J Immunol Methods (2020) 478:112721. doi: 10.1016/j.jim.2019.112721
75. Velmurugan R, Challa DK, Ram S, Ober RJ, Ward ES. Macrophage-mediated trogocytosis leads to death of antibody-opsonized tumor cells. Mol Cancer Ther (2016) 15(8):1879–89. doi: 10.1158/1535-7163.mct-15-0335
76. Grugan KD, Dorn K, Jarantow SW, Bushey BS, Pardinas JR, Laquerre S, et al. Fc-mediated activity of EGFR x c-met bispecific antibody JNJ-61186372 enhanced killing of lung cancer cells. mAbs (2017) 9(1):114–26. doi: 10.1080/19420862.2016.1249079
77. Khodabakhsh F, Behdani M, Rami A, Kazemi-Lomedasht F. Single-domain antibodies or nanobodies: A class of next-generation antibodies. Int Rev Immunol (2018) 37(6):316–22. doi: 10.1080/08830185.2018.1526932
78. Klichinsky M, Ruella M, Shestova O, Lu XM, Best A, Zeeman M, et al. Human chimeric antigen receptor macrophages for cancer immunotherapy. Nat Biotechnol (2020) 38(8):947–53. doi: 10.1038/s41587-020-0462-y
79. Chen SH, Dominik PK, Stanfield J, Ding S, Yang W, Kurd N, et al. Dual checkpoint blockade of CD47 and PD-L1 using an affinity-tuned bispecific antibody maximizes antitumor immunity. J Immunother Cancer (2021) 9(10):e003464. doi: 10.1136/jitc-2021-003464
80. Shim H. Bispecific antibodies and antibody-drug conjugates for cancer therapy: Technological considerations. Biomolecules (2020) 10(3):360. doi: 10.3390/biom10030360
81. Rajala HLM, Anttila VJ, Haapio M, Keränen MAI, Wartiovaara-Kautto U, Räty R. Gemtuzumab-Ozogamicin-Related impaired hemoglobin-haptoglobin scavenging as on-Target/Off-Tumor toxicity of anti-CD33 AML therapy: A report of two cases. Case Rep Hematol (2021) 2021:6641349. doi: 10.1155/2021/6641349
82. Han H, Li S, Chen T, Fitzgerald M, Liu S, Peng C, et al. Targeting HER2 exon 20 insertion-mutant lung adenocarcinoma with a novel tyrosine kinase inhibitor mobocertinib. Cancer Res (2021) 81(20):5311–24. doi: 10.1158/0008-5472.can-21-1526
83. Nakada T, Sugihara K, Jikoh T, Abe Y, Agatsuma T. The latest research and development into the antibody-drug conjugate, [fam-] trastuzumab deruxtecan (DS-8201a), for HER2 cancer therapy. Chem Pharm Bull (2019) 67(3):173–85. doi: 10.1248/cpb.c18-00744
84. Dela Cruz JS, Trinh KR, Morrison SL, Penichet ML. Recombinant anti-human HER2/neu IgG3-(GM-CSF) fusion protein retains antigen specificity and cytokine function and demonstrates antitumor activity. J Immunol (Baltimore Md 1950) (2000) 165(9):5112–21. doi: 10.4049/jimmunol.165.9.5112
85. Sindhwani S, Syed AM, Ngai J, Kingston BR, Maiorino L, Rothschild J, et al. The entry of nanoparticles into solid tumours. Nat Mater (2020) 19(5):566–75. doi: 10.1038/s41563-019-0566-2
86. Chen XJ, Deng YR, Wang ZC, Wei WF, Zhou CF, Zhang YM, et al. Hypoxia-induced ZEB1 promotes cervical cancer progression via CCL8-dependent tumour-associated macrophage recruitment. Cell Death Dis (2019) 10(7):508. doi: 10.1038/s41419-019-1748-1
87. Ernsting MJ, Hoang B, Lohse I, Undzys E, Cao P, Do T, et al. Targeting of metastasis-promoting tumor-associated fibroblasts and modulation of pancreatic tumor-associated stroma with a carboxymethylcellulose-docetaxel nanoparticle. J Controlled Release (2015) 206:122–30. doi: 10.1016/j.jconrel.2015.03.023
88. Zhao P, Wang Y, Kang X, Wu A, Yin W, Tang Y, et al. Dual-targeting biomimetic delivery for anti-glioma activity via remodeling the tumor microenvironment and directing macrophage-mediated immunotherapy. Chem Sci (2018) 9(10):2674–89. doi: 10.1039/c7sc04853j
89. An L, Wang Y, Lin J, Tian Q, Xie Y, Hu J, et al. Macrophages-mediated delivery of small gold nanorods for tumor hypoxia photoacoustic imaging and enhanced photothermal therapy. ACS Appl Mater Interfaces (2019) 11(17):15251–61. doi: 10.1021/acsami.9b00495
90. Chen Q, Wang C, Zhang X, Chen G, Hu Q, Li H, et al. In situ sprayed bioresponsive immunotherapeutic gel for post-surgical cancer treatment. Nat Nanotechnol (2019) 14(1):89–97. doi: 10.1038/s41565-018-0319-4
91. Zhang X, Detering L, Sultan D, Luehmann H, Li L, Heo GS, et al. CC chemokine receptor 2-targeting copper nanoparticles for positron emission tomography-guided delivery of gemcitabine for pancreatic ductal adenocarcinoma. ACS Nano (2021) 15(1):1186–98. doi: 10.1021/acsnano.0c08185
92. Wen AM, Lee KL, Cao P, Pangilinan K, Carpenter BL, Lam P, et al. Utilizing viral Nanoparticle/Dendron hybrid conjugates in photodynamic therapy for dual delivery to macrophages and cancer cells. Bioconjugate Chem (2016) 27(5):1227–35. doi: 10.1021/acs.bioconjchem.6b00075
93. Nie W, Wu G, Zhang J, Huang LL, Ding J, Jiang A, et al. Responsive exosome nano-bioconjugates for synergistic cancer therapy. Angewandte Chem (International English) (2020) 59(5):2018–22. doi: 10.1002/anie.201912524
94. Mason CA, Kossatz S, Carter LM, Pirovano G, Brand C, Guru N, et al. An (89)Zr-HDL PET tracer monitors response to a CSF1R inhibitor. J Nucl Med (2020) 61(3):433–6. doi: 10.2967/jnumed.119.230466
95. Gao F, Tang Y, Liu WL, Zou MZ, Huang C, Liu CJ, et al. Intra/Extracellular lactic acid exhaustion for synergistic metabolic therapy and immunotherapy of tumors. Adv Mater (Deerfield Beach Fla) (2019) 31(51):e1904639. doi: 10.1002/adma.201904639
96. Li M, Yang Y, Xu C, Wei J, Liu Y, Cun X, et al. Tumor-targeted chemoimmunotherapy with immune-checkpoint blockade for enhanced anti-melanoma efficacy. AAPS J (2019) 21(2):18. doi: 10.1208/s12248-018-0289-3
97. Shmeeda H, Amitay Y, Gorin J, Tzemach D, Mak L, Stern ST, et al. Coencapsulation of alendronate and doxorubicin in pegylated liposomes: a novel formulation for chemoimmunotherapy of cancer. J Drug Targeting (2016) 24(9):878–89. doi: 10.1080/1061186x.2016.1191081
98. Tanei T, Leonard F, Liu X, Alexander JF, Saito Y, Ferrari M, et al. Redirecting transport of nanoparticle albumin-bound paclitaxel to macrophages enhances therapeutic efficacy against liver metastases. Cancer Res (2016) 76(2):429–39. doi: 10.1158/0008-5472.can-15-1576
99. Cullis J, Siolas D, Avanzi A, Barui S, Maitra A, Bar-Sagi D. Macropinocytosis of nab-paclitaxel drives macrophage activation in pancreatic cancer. Cancer Immunol Res (2017) 5(3):182–90. doi: 10.1158/2326-6066.cir-16-0125
100. Deng G, Sun Z, Li S, Peng X, Li W, Zhou L, et al. Cell-membrane immunotherapy based on natural killer cell membrane coated nanoparticles for the effective inhibition of primary and abscopal tumor growth. ACS Nano (2018) 12(12):12096–108. doi: 10.1021/acsnano.8b05292
101. Zhang F, Mastorakos P, Mishra MK, Mangraviti A, Hwang L, Zhou J, et al. Uniform brain tumor distribution and tumor associated macrophage targeting of systemically administered dendrimers. Biomaterials (2015) 52:507–16. doi: 10.1016/j.biomaterials.2015.02.053
102. Sabatino R, Battistelli S, Magnani M, Rossi L. Preclinical evaluation of an innovative anti-TAM approach based on zoledronate-loaded erythrocytes. Drug Deliv Trans Res (2018) 8(5):1355–64. doi: 10.1007/s13346-018-0560-2
103. Shen S, Li HJ, Chen KG, Wang YC, Yang XZ, Lian ZX, et al. Spatial targeting of tumor-associated macrophages and tumor cells with a pH-sensitive cluster nanocarrier for cancer chemoimmunotherapy. Nano Lett (2017) 17(6):3822–9. doi: 10.1021/acs.nanolett.7b01193
104. Alizadeh D, White EE, Sanchez TC, Liu S, Zhang L, Badie B, et al. Immunostimulatory CpG on carbon nanotubes selectively inhibits migration of brain tumor cells. Bioconjugate Chem (2018) 29(5):1659–68. doi: 10.1021/acs.bioconjchem.8b00146
105. Guerra AD, Yeung OWH, Qi X, Kao WJ, Man K. The anti-tumor effects of M1 macrophage-loaded poly (ethylene glycol) and gelatin-based hydrogels on hepatocellular carcinoma. Theranostics (2017) 7(15):3732–44. doi: 10.7150/thno.20251
106. Kim MS, Haney MJ, Zhao Y, Yuan D, Deygen I, Klyachko NL, et al. Engineering macrophage-derived exosomes for targeted paclitaxel delivery to pulmonary metastases: in vitro and in vivo evaluations. Nanomed Nanotechnol Biol Med (2018) 14(1):195–204. doi: 10.1016/j.nano.2017.09.011
107. Ray M, Lee YW, Hardie J, Mout R, Yeşilbag Tonga G, Farkas ME, et al. CRISPRed macrophages for cell-based cancer immunotherapy. Bioconjugate Chem (2018) 29(2):445–50. doi: 10.1021/acs.bioconjchem.7b00768
108. Holden CA, Yuan Q, Yeudall WA, Lebman DA, Yang H. Surface engineering of macrophages with nanoparticles to generate a cell-nanoparticle hybrid vehicle for hypoxia-targeted drug delivery. Int J Nanomed (2010) 5:25–36. doi: 10.2147/IJN.S8339
109. Klyachko NL, Polak R, Haney MJ, Zhao Y, Gomes Neto RJ, Hill MC, et al. Macrophages with cellular backpacks for targeted drug delivery to the brain. Biomaterials (2017) 140:79–87. doi: 10.1016/j.biomaterials.2017.06.017
110. Kalluri R, LeBleu VS. The biology, function, and biomedical applications of exosomes. Sci (New York NY) (2020) 367(6478):eaau6977. doi: 10.1126/science.aau6977
111. Liu J, Wu F, Zhou H. Macrophage-derived exosomes in cancers: Biogenesis, functions and therapeutic applications. Immunol Lett (2020) 227:102–8. doi: 10.1016/j.imlet.2020.08.003
112. Haney MJ, Zhao Y, Jin YS, Li SM, Bago JR, Klyachko NL, et al. Macrophage-derived extracellular vesicles as drug delivery systems for triple negative breast cancer (TNBC) therapy. J Neuroimmune Pharmacol (2020) 15(3):487–500. doi: 10.1007/s11481-019-09884-9
113. Bai G, Matsuba T, Niki T, Hattori T. Stimulation of THP-1 macrophages with LPS increased the production of osteopontin-encapsulating exosome. Int J Mol Sci (2020) 21(22):8490. doi: 10.3390/ijms21228490
114. Zhu X, Shen H, Yin X, Yang M, Wei H, Chen Q, et al. Macrophages derived exosomes deliver miR-223 to epithelial ovarian cancer cells to elicit a chemoresistant phenotype. J Exp Clin Cancer Res (2019) 38(1):81. doi: 10.1186/s13046-019-1095-1
115. Cao H, Dan Z, He X, Zhang Z, Yu H, Yin Q, et al. Liposomes coated with isolated macrophage membrane can target lung metastasis of breast cancer. ACS Nano (2016) 10(8):7738–48. doi: 10.1021/acsnano.6b03148
116. Zhang Y, Cai K, Li C, Guo Q, Chen Q, He X, et al. Macrophage-Membrane-Coated nanoparticles for tumor-targeted chemotherapy. Nano Lett (2018) 18(3):1908–15. doi: 10.1021/acs.nanolett.7b05263
117. Song M, Liu T, Shi C, Zhang X, Chen X. Bioconjugated manganese dioxide nanoparticles enhance chemotherapy response by priming tumor-associated macrophages toward M1-like phenotype and attenuating tumor hypoxia. ACS Nano (2016) 10(1):633–47. doi: 10.1021/acsnano.5b06779
118. Baker DW, Zhou J, Tsai YT, Patty KM, Weng H, Tang EN, et al. Development of optical probes for in vivo imaging of polarized macrophages during foreign body reactions. Acta Biomater (2014) 10(7):2945–55. doi: 10.1016/j.actbio.2014.04.001
119. Rayamajhi S, Marasini R, Nguyen TDT, Plattner BL, Biller D, Aryal S. Strategic reconstruction of macrophage-derived extracellular vesicles as a magnetic resonance imaging contrast agent. Biomater Sci (2020) 8(10):2887–904. doi: 10.1039/d0bm00128g
120. Aghighi M, Theruvath AJ, Pareek A, Pisani LL, Alford R, Muehe AM, et al. Magnetic resonance imaging of tumor-associated macrophages: Clinical translation. Clin Cancer Res (2018) 24(17):4110–8. doi: 10.1158/1078-0432.ccr-18-0673
121. Khurana A, Chapelin F, Xu H, Acevedo JR, Molinolo A, Nguyen Q, et al. Visualization of macrophage recruitment in head and neck carcinoma model using fluorine-19 magnetic resonance imaging. Magnetic Resonance Med (2018) 79(4):1972–80. doi: 10.1002/mrm.26854
122. Makela AV, Gaudet JM, Foster PJ. Quantifying tumor associated macrophages in breast cancer: a comparison of iron and fluorine-based MRI cell tracking. Sci Rep (2017) 7:42109. doi: 10.1038/srep42109
123. Egeblad M, Ewald AJ, Askautrud HA, Truitt ML, Welm BE, Bainbridge E, et al. Visualizing stromal cell dynamics in different tumor microenvironments by spinning disk confocal microscopy. Dis Models Mechanisms (2008) 1(2-3):155–67. doi: 10.1242/dmm.000596
124. Sun JY, Shen J, Thibodeaux J, Huang G, Wang Y, Gao J, et al. In vivo optical imaging of folate receptor-β in head and neck squamous cell carcinoma. Laryngoscope (2014) 124(8):E312–9. doi: 10.1002/lary.24606
125. Szulczewski JM, Inman DR, Entenberg D, Ponik SM, Aguirre-Ghiso J, Castracane J, et al. In vivo visualization of stromal macrophages via label-free FLIM-based metabolite imaging. Sci Rep (2016) 6:25086. doi: 10.1038/srep25086
126. Pérez-Medina C, Tang J, Abdel-Atti D, Hogstad B, Merad M, Fisher EA, et al. PET imaging of tumor-associated macrophages with 89Zr-labeled high-density lipoprotein nanoparticles. J Nucl Med (2015) 56(8):1272–7. doi: 10.2967/jnumed.115.158956
127. Wang J, Meng J, Ran W, Lee RJ, Teng L, Zhang P, et al. Hepatocellular carcinoma growth retardation and PD-1 blockade therapy potentiation with synthetic high-density lipoprotein. Nano Lett (2019) 19(8):5266–76. doi: 10.1021/acs.nanolett.9b01717
128. Dranoff G. Cytokines in cancer pathogenesis and cancer therapy. Nat Rev Cancer (2004) 4(1):11–22. doi: 10.1038/nrc1252
129. Pushalkar S, Hundeyin M, Daley D, Zambirinis CP, Kurz E, Mishra A, et al. The pancreatic cancer microbiome promotes oncogenesis by induction of innate and adaptive immune suppression. Cancer Discov (2018) 8(4):403–16. doi: 10.1158/2159-8290.Cd-17-1134
130. Predina J, Eruslanov E, Judy B, Kapoor V, Cheng G, Wang LC, et al. Changes in the local tumor microenvironment in recurrent cancers may explain the failure of vaccines after surgery. Proc Natl Acad Sci United States America (2013) 110(5):E415–24. doi: 10.1073/pnas.1211850110
131. Wei Z, Zhang X, Yong T, Bie N, Zhan G, Li X, et al. Boosting anti-PD-1 therapy with metformin-loaded macrophage-derived microparticles. Nat Commun (2021) 12(1):440. doi: 10.1038/s41467-020-20723-x
132. Muraoka D, Harada N, Hayashi T, Tahara Y, Momose F, Sawada S, et al. Nanogel-based immunologically stealth vaccine targets macrophages in the medulla of lymph node and induces potent antitumor immunity. ACS Nano (2014) 8(9):9209–18. doi: 10.1021/nn502975r
133. Kang S, Ahn S, Lee J, Kim JY, Choi M, Gujrati V, et al. Effects of gold nanoparticle-based vaccine size on lymph node delivery and cytotoxic T-lymphocyte responses. J Controlled Release (2017) 256:56–67. doi: 10.1016/j.jconrel.2017.04.024
134. Noman MZ, Desantis G, Janji B, Hasmim M, Karray S, Dessen P, et al. PD-L1 is a novel direct target of HIF-1α, and its blockade under hypoxia enhanced MDSC-mediated T cell activation. J Exp Med (2014) 211(5):781–90. doi: 10.1084/jem.20131916
135. Shklovskaya E, Terry AM, Guy TV, Buckley A, Bolton HA, Zhu E, et al. Tumour-specific CD4 T cells eradicate melanoma via indirect recognition of tumour-derived antigen. Immunol Cell Biol (2016) 94(6):593–603. doi: 10.1038/icb.2016.14
136. Lorvik KB, Hammarström C, Fauskanger M, Haabeth OA, Zangani M, Haraldsen G, et al. Adoptive transfer of tumor-specific Th2 cells eradicates tumors by triggering an In situ inflammatory immune response. Cancer Res (2016) 76(23):6864–76. doi: 10.1158/0008-5472.can-16-1219
137. Rong L, Zhang Y, Li WS, Su Z, Fadhil JI, Zhang C. Iron chelated melanin-like nanoparticles for tumor-associated macrophage repolarization and cancer therapy. Biomaterials (2019) 225:119515. doi: 10.1016/j.biomaterials.2019.119515
138. Thakker S, Purushothaman P, Gupta N, Challa S, Cai Q, Verma SC. Kaposi's sarcoma-associated herpesvirus latency-associated nuclear antigen inhibits major histocompatibility complex class II expression by disrupting enhanceosome assembly through binding with the regulatory factor X complex. J Virol (2015) 89(10):5536–56. doi: 10.1128/jvi.03713-14
139. Yankuzo HM, Baraya YS, Mustapha Z, Wong KK, Yaacob NS. Immunomodulatory effects of a bioactive fraction of strobilanthes crispus in NMU-induced rat mammary tumor model. J Ethnopharmacol (2018) 213:31–7. doi: 10.1016/j.jep.2017.10.024
140. Perez-Diez A, Liu X, Matzinger P. Neoantigen presentation and IFNγ signaling on the same tumor-associated macrophage are necessary for CD4 T cell-mediated antitumor activity in mice. Cancer Res Commun (2022) 2(5):316–29. doi: 10.1158/2767-9764.crc-22-0052
141. Catalán D, Mansilla MA, Ferrier A, Soto L, Oleinika K, Aguillón JC, et al. Immunosuppressive mechanisms of regulatory b cells. Front Immunol (2021) 12:611795. doi: 10.3389/fimmu.2021.611795
142. Zhang B, Vogelzang A, Miyajima M, Sugiura Y, Wu Y, Chamoto K, et al. B cell-derived GABA elicits IL-10(+) macrophages to limit anti-tumour immunity. Nature (2021) 599(7885):471–6. doi: 10.1038/s41586-021-04082-1
143. Goldman N, Valiuskyte K, Londregan J, Swider A, Somerville J, Riggs JE. Macrophage regulation of b cell proliferation. Cell Immunol (2017) 314:54–62. doi: 10.1016/j.cellimm.2017.02.002
144. Chen WC, Kawasaki N, Nycholat CM, Han S, Pilotte J, Crocker PR, et al. Antigen delivery to macrophages using liposomal nanoparticles targeting sialoadhesin/CD169. PloS One (2012) 7(6):e39039. doi: 10.1371/journal.pone.0039039
145. White AL, Chan HT, Roghanian A, French RR, Mockridge CI, Tutt AL, et al. Interaction with FcγRIIB is critical for the agonistic activity of anti-CD40 monoclonal antibody. J Immunol (Baltimore Md 1950) (2011) 187(4):1754–63. doi: 10.4049/jimmunol.1101135
146. Bruhns P, Iannascoli B, England P, Mancardi DA, Fernandez N, Jorieux S, et al. Specificity and affinity of human fcgamma receptors and their polymorphic variants for human IgG subclasses. Blood (2009) 113(16):3716–25. doi: 10.1182/blood-2008-09-179754
147. Maruggi M, Layng FI, Lemos R Jr., Garcia G, James BP, Sevilla M, et al. Absence of HIF1A leads to glycogen accumulation and an inflammatory response that enables pancreatic tumor growth. Cancer Res (2019) 79(22):5839–48. doi: 10.1158/0008-5472.Can-18-2994
148. Stary G, Bangert C, Tauber M, Strohal R, Kopp T, Stingl G. Tumoricidal activity of TLR7/8-activated inflammatory dendritic cells. J Exp Med (2007) 204(6):1441–51. doi: 10.1084/jem.20070021
149. Cao M, Yan H, Han X, Weng L, Wei Q, Sun X, et al. Ginseng-derived nanoparticles alter macrophage polarization to inhibit melanoma growth. J Immunother Cancer (2019) 7(1):326. doi: 10.1186/s40425-019-0817-4
150. Smith M, García-Martínez E, Pitter MR, Fucikova J, Spisek R, Zitvogel L, et al. Trial watch: Toll-like receptor agonists in cancer immunotherapy. Oncoimmunology (2018) 7(12):e1526250. doi: 10.1080/2162402x.2018.1526250
151. Benne N, van Duijn J, Kuiper J, Jiskoot W, Slütter B. Orchestrating immune responses: How size, shape and rigidity affect the immunogenicity of particulate vaccines. J Controlled Release (2016) 234:124–34. doi: 10.1016/j.jconrel.2016.05.033
152. Gabrilovich DI, Ostrand-Rosenberg S, Bronte V. Coordinated regulation of myeloid cells by tumours. Nat Rev Immunol (2012) 12(4):253–68. doi: 10.1038/nri3175
153. Eisinger S, Sarhan D, Boura VF, Ibarlucea-Benitez I, Tyystjärvi S, Oliynyk G, et al. Targeting a scavenger receptor on tumor-associated macrophages activates tumor cell killing by natural killer cells. Proc Natl Acad Sci United States America (2020) 117(50):32005–16. doi: 10.1073/pnas.2015343117
154. La Fleur L, Botling J, He F, Pelicano C, Zhou C, He C, et al. Targeting MARCO and IL37R on immunosuppressive macrophages in lung cancer blocks regulatory T cells and supports cytotoxic lymphocyte function. Cancer Res (2021) 81(4):956–67. doi: 10.1158/0008-5472.Can-20-1885
155. Terrén I, Orrantia A, Vitallé J, Zenarruzabeitia O, Borrego F. NK cell metabolism and tumor microenvironment. Front Immunol (2019) 10:2278. doi: 10.3389/fimmu.2019.02278
156. Gerhard GM, Bill R, Messemaker M, Klein AM, Pittet MJ. Tumor-infiltrating dendritic cell states are conserved across solid human cancers. J Exp Med (2021) 218(1):e20200264. doi: 10.1084/jem.20200264
157. Cassetta L, Pollard JW. Tumor-associated macrophages. Curr Biol CB (2020) 30(6):R246–r8. doi: 10.1016/j.cub.2020.01.031
158. Kumar V, Donthireddy L, Marvel D, Condamine T, Wang F, Lavilla-Alonso S, et al. Cancer-associated fibroblasts neutralize the anti-tumor effect of CSF1 receptor blockade by inducing PMN-MDSC infiltration of tumors. Cancer Cell (2017) 32(5):654–68.e5. doi: 10.1016/j.ccell.2017.10.005
Keywords: tumor-associated macrophages, targeted therapy, cancer, crosstalk, biomaterials
Citation: Jing F, Liu X, Chen X, Wu F and Gao Q (2022) Tailoring biomaterials and applications targeting tumor-associated macrophages in cancers. Front. Immunol. 13:1049164. doi: 10.3389/fimmu.2022.1049164
Received: 20 September 2022; Accepted: 25 October 2022;
Published: 11 November 2022.
Edited by:
Xuyu Gu, Southeast University, ChinaCopyright © 2022 Jing, Liu, Chen, Wu and Gao. This is an open-access article distributed under the terms of the Creative Commons Attribution License (CC BY). The use, distribution or reproduction in other forums is permitted, provided the original author(s) and the copyright owner(s) are credited and that the original publication in this journal is cited, in accordance with accepted academic practice. No use, distribution or reproduction is permitted which does not comply with these terms.
*Correspondence: Fanglong Wu, d3VmYW5nbG9uZ0BzY3UuZWR1LmNu; Qinghong Gao, cWhnYW9Ac2N1LmVkdS5jbg==