- 1Department of Physiology and Pharmacology, Laboratory affiliated to Pasteur Italy, University of Rome La Sapienza, Rome, Italy
- 2IRCCS Neuromed, Pozzilli (IS), Italy
Microglial cells play pleiotropic homeostatic activities in the brain, during development and in adulthood. Microglia regulate synaptic activity and maturation, and continuously patrol brain parenchyma monitoring for and reacting to eventual alterations or damages. In the last two decades microglia were given a central role as an indicator to monitor the inflammatory state of brain parenchyma. However, the recent introduction of single cell scRNA analyses in several studies on the functional role of microglia, revealed a not-negligible spatio-temporal heterogeneity of microglial cell populations in the brain, both during healthy and in pathological conditions. Furthermore, the recent advances in the knowledge of the mechanisms involved in the modulation of cerebral activity induced by gut microbe-derived molecules open new perspectives for deciphering the role of microglial cells as possible mediators of these interactions. The aim of this review is to summarize the most recent studies correlating gut-derived molecules and vagal stimulation, as well as dysbiotic events, to alteration of brain functioning, and the contribution of microglial cells.
Introduction
Microglia are multitasking cells that naturally respond to pathogens and maintain central nervous system (CNS) tissue integrity and functionality throughout life (1, 2). In the last decade, many studies deeper investigated on microglia phenotyping and functioning with new straightforward techniques in healthy and diseased brain (3, 4). In parallel, an increasing number of new data displayed the ability of gut microbiota in modulating microglia functions in healthy as well diseased conditions. Intrinsic and extrinsic factors such as host genetics, diet, lifestyle and drug use can significantly impact the gut microbiota, shaping both the microbial community and the pools of microbiota-derived metabolites (5–8). Here, we firstly summarize relevant studies on microglia identity and functions; then, we collected information on how microbiota-derived signals such as short chain fatty acids (SCFA), lipopolysaccharide (LPS), tryptophan derived molecules, vagal nerve stimulation and microbiota alteration affect the microglial phenotype and functions during steady-state and pathological conditions.
Microglia: The brain resident macrophages
Microglia control cerebral homeostasis
Microglia are the resident macrophages of the CNS that contribute to the innate immune surveillance of the brain and its homeostasis (9–11). Microglial cells derive from erythro-myeloid yolk sac precursors and before the maturation of the blood-brain barrier (BBB), they colonize the mouse brain at embryonic day 9.5 (12). Here, these cells proliferate and distribute throughout the CNS becoming the only myeloid cells in the healthy brain parenchyma (13); other myeloid cells, derived from the bone marrow, reside in the peri-parenchymal regions (perivascular and meningeal spaces) (14), or enter the brain only in pathological conditions (15). Microglia account for about 10% of brain cells, with some regional differences (16–18) and, together with astrocytes and oligodendrocytes, contribute to the non-neuronal part of the brain that supports the CNS environment (19). Microglial cells can be identified by specific markers, such as Transmembrane Protein 119 (TMEM119), Purinergic Receptor P2Y12 (P2RY12), and the Spalt-like transcription factor (SALL1) (20), however many of them are shared with macrophages (3). Alteration of certain markers such as the triggering receptor expressed on myeloid cells 2 (TREM2) and the Colony Stimulating Factor-1 receptor (CSF-1R) correlates with neurodegenerative diseases (21) and leukodystrophy (22) respectively indicating the important role played by these cells in the homeostatic control of CNS. Microglia continuously patrol cerebral parenchyma through their cellular processes and quickly react to pathological signals derived from acute and chronic injuries, neurodegenerative processes, or physiological aging (9, 11, 23). Microglial response to insults is often referred to as “activation” and includes migration or process extension to the damaged site, cell proliferation, phagocytosis, and production of soluble molecules (19); however, it must be mentioned that “activation” does not identify unique microglial phenotypes or functions. In particular, microglia may assume an ameboid-like or highly ramified shape upon different stimuli, increase or reduce its phagocytic activity and produce a number of cytokines, chemokines, and growth factors that may affect other glial or neuronal cell activities.
Microglia secrete trophic and repair factors
According to the homeostatic role of microglia, these cells are able to secrete molecules that participate in the immune surveillance of the brain and produce neurotrophic factors important to neuronal homeostasis. In particular, microglial brain-derived neurotrophic factor (BDNF), is one of the main factors that regulates synaptic plasticity and spines density (24–26). Other evidence supports the involvement of microglia in the maturation and functioning of other brain cells. For instance, microglia-conditioned media pursue the differentiation program of neural stem/precursor cells (NSPCs) and oligodendrocyte precursor cells (OPCs) into astrocytes (27) and mature oligodendrocyte (28), respectively. Among the roles played by microglial cells to maintain cerebral homeostasis, tissue repair is one of the most prominent. As mentioned above, microglia constantly monitor the cerebral parenchyma, controlling neurons and their activity, performing a rapid response against intruders, and eliminating debris present throughout the cerebral environment. However, after injury or neural damage, microglia are also involved in tissue repair, through the release of molecules such as BDNF, tumor necrosis factor-alfa (TNFα), and Arginase-1 (29) that promote recovery from damage. Myelination is one important aspect of the recovery processes that involve microglia; this process requires iron, an important co-factor for oligodendrocytes (30), and microglia are the principal suppliers of this metal. Microglia also affect the re-myelination of damaged neurons with the release of molecules that promote the proliferation and differentiation of oligodendrocyte progenitor cells (OPC) (31). Re-myelination is a regenerative process that occurs even upon diseases such as multiple sclerosis (MS) but often fails in the progressive phase of the pathology (32). Tissue repair is a process that occurs after a natural immune response; however, an excessive phagocytic activity could damage tissues (33). To understand the role of activated microglial cells in tissue repair after an injury, Cunha and colleagues performed experiments in zebrafish and mice deleted from myeloid differentiation primary response gene 88 (MyD88), an adapter protein involved in cell-mediated immune response. In particular, they highlighted the importance of the pro-inflammatory phenotype in microglial cells to induce degradation and clearance of phagocytic myelin and to increase oligodendrogenesis (34). It has been widely accepted that regenerative properties are linked to the activity of immune cells; this is due initially to the activation of the pro-inflammatory phenotype that increases the proliferation of OPCs and, later, during the late phase of the immune response, microglial cells acquire an anti-inflammatory phenotype that releases growth factors that promote OPC differentiation (35). These results show the functions of microglia, from development to adult life, indicating not only the immune activity performed by these cells but also their roles in the maintenance of cerebral homeostasis
Microglia promote neural circuits development and function
Another function performed by microglia in the CNS is to control the formation of neuronal circuits. In particular, brain circuits need microglia for proper development, functioning and maintenance of their plasticity. Microglia cells, similarly to macrophages, exhibit phagocytic activity, a receptor-mediated process that recognizes, engulfs and eliminates dead cells, bacteria or cellular debris. Among the receptors involved in this process are the toll-like receptors (TLR) that recognize microbial pathogens and TREM2, activated against apoptotic cells (36). During development, neuronal cells move to reach their final destination, but a high number of newborn cells will be eliminated before, during, and after the journey has been completed. This elimination mainly results from mechanisms of programmed cell death (PCD) (37). In vivo experiments indicated that microglia play an active role in this process and that the alteration of microglial activity deeply influences the elimination of neural precursor cells (NPCs) (38). It has been demonstrated that mice lacking the fractalkine receptor, C-X3-C Motif Chemokine Receptor 1 (CX3CR1), highly expressed on microglial cells, showed an increase in the number of apoptotic neurons in layer V of the cerebral cortex (39); this effect can be related to the activity of insulin-like growth factor 1 (IGF-1) a trophic factor implicated in NPC survival which is reduced in CX3CR1-deficient mice (40, 41). The connection between microglia and neural circuits, in addition to the normal building of the brain network, sees these cells also involved in the formation of memory. Synaptic plasticity is the neuronal event involved in long-term potentiation (LTP), one of the molecular mechanism that better explain the processes of learning and memory (42). Elimination of microglia cells with clodronate affects LTP in the hippocampal CA1 area, highlighting the role of these cells in the organization and preparation of circuits for memory formation (43). This key role is also observed during development, when mice lacking CX3CR1 show a reduction in both excitatory postsynaptic currents and glutamate release (44). Another important aspect of microglia in memory formation concerns the consolidation of the engrams (45). The role of microglia in the maintenance of memories is due to phagocytic activity and trogocytosis (46) that eliminates synapses (47). Eliminating the number of microglial cells with CSF-1R inhibitors, or reducing their phagocytic activity improves long-term effects on memory consolidation in mice (45). According to these data, microglia may play important roles in modulating neuronal elimination, The elimination of debris is important for correct neuronal networking: blockade of microglial CSF-1R affects their removal and neuronal connectivity (48). Moreover, the correct formation of the mouse visual system requires microglial activity for the pruning, which consists of the elimination of weaker or excessive synaptic connections, through the activation of the CR3 complement receptor in the retinogeniculate system (49). The pruning activity in this brain region is not mediated by CX3CR1 (50), which, however, contributes to the pruning of the barrel cortex and hippocampus (51, 52), indicating a spatial functional heterogeneity of microglia for the same activity. The application of high-throughput approaches for the study of single-cell RNA-seq led to the identification of microglial sensoma (53), common to a number of degenerative neurological conditions, but also to the discovery of spatially and temporally different microglial clusters that opens new perspectives for a more precise understanding of microglial roles in the brain (54).
Identity and spatial heterogeneity
Microglial cells are characterized by dynamic changes from the early development until adult life, transferring from the yolk sac and then migrating into the CNS (12), where they proliferate and distribute to different areas (55); This activity highlights the presence of intrinsic changes in microglia both at the transcriptomic and functional levels, which ensure rapid adaptation to the environment. These changes can not only be related to the state of rest or activated microglia but highlight the presence of time- and space-dependent molecular programs. Recently, the use of advanced tools to study the gene expression programs of cells allowed to highlight the differences induced by space and time on myeloid cells (56). Matcovitch-Natan and colleagues, using RNA sequencing (RNA-seq) studied microglia gene expression from the embryonic stage until the adult brain (57). They described three microglial stages: the first, where microglia express genes related to the cell cycle such as minichromosome maintenance complex component 5 (Mcm5), and Disabled-2 (Dab2), the second, of pre-microglia, typical of the last embryonal stage, characterized by genes related to neuronal development such as Csf1, C-X-C Motif Chemokine Receptor 2 (Cxcr2) and, the last adult stage where microglia increase the expression of genes such as Cluster of Differentiation 14 (Cd14), Prostate transmembrane protein, androgen induced 1 (Pmepa1) (57). Recent work further improved this RNA-seq analysis, grouping the transcriptomic subpopulation of microglial cells through an independent component analysis (58). With this type of analysis Hammond and colleagues identified nine different microglial clusters in mice, with some genes predominant at specific ages, others common to all stages (Complement C1q A chain, C1qa; FC receptor-like S, Fcrls; Trem2) and others only transiently expressed (59). During the first stages of development, they observed the greatest variety of gene expression, which is reduced with time, becoming similar in the juvenile and adult phases. A similar description of microglial gene expression at the single cell level was obtained by Masuda (54). The spatial heterogeneity of microglia in different brain regions is not new (60); however, in Masuda’s work, this type of investigation has been extended to microglia under both physiological and pathological conditions. The homeostatic microglia have been classified into ten clusters segregated into two main groups belonging to the embryonic (C1-C6) and postnatal (C7-C10) stages. In the embryonic stage, these microglia groups are segregated in the embryonic CNS, as well as after birth where clusters are divided both spatially and temporally. In particular, in young mice, the C10 cluster of microglia was present in both the cortical region and in the hippocampus, whereas the C7 cluster was predominantly present in the cerebellum and corpus callosum of the adult (54). These data acquire a particular interest because they describe the different subtypes of microglia not only during the developmental phase but also in adult life, where the spatial distribution plays an important role in the characterization of microglia subpopulations. Interestingly, after an injury, specific microglia subtypes were recruited after three (C11) and fourteen (C12-C13) days, again indicating the presence of time-dependent phenotypes (54). Although they found several transcriptional models of gene expression in microglia, it is difficult to say whether these changes are related to different clusters, or rather they could represent a microglial adaptation to the environment. Microglia clustering is not a prerogative of the brain, but it has been also described in the spinal cord. This was confirmed by Tansley and colleagues by single-cells RNA sequencing (scRNA-seq) in both male and female mice in a nerve injury model. Interestingly, they confirmed the existence of several subpopulations of microglia, mostly belonging to six clusters; however, the situation changes after nerve lesions in different sexes. Male mice were characterized by a large transcription of pro-inflammatory genes, but the same intensity was not found in females, indicating an intrinsic difference between the two sexes to painful reactions. However, despite the different initial pro-inflammatory intensity, the immune response, after the injury, followed the natural course towards an anti-inflammatory transcriptional program (61). In addition, to confirm a different transcription program between the two sexes, they found an activated immune cluster (C9) only in male mice and, in females, a less intense proliferation program (61). Collectively, these papers, summarized in Table 1, have highlighted the existence of different microglial subpopulations that share common transcriptional programs, but that can quickly change based on different temporal and spatial signals from the environment or by the host.
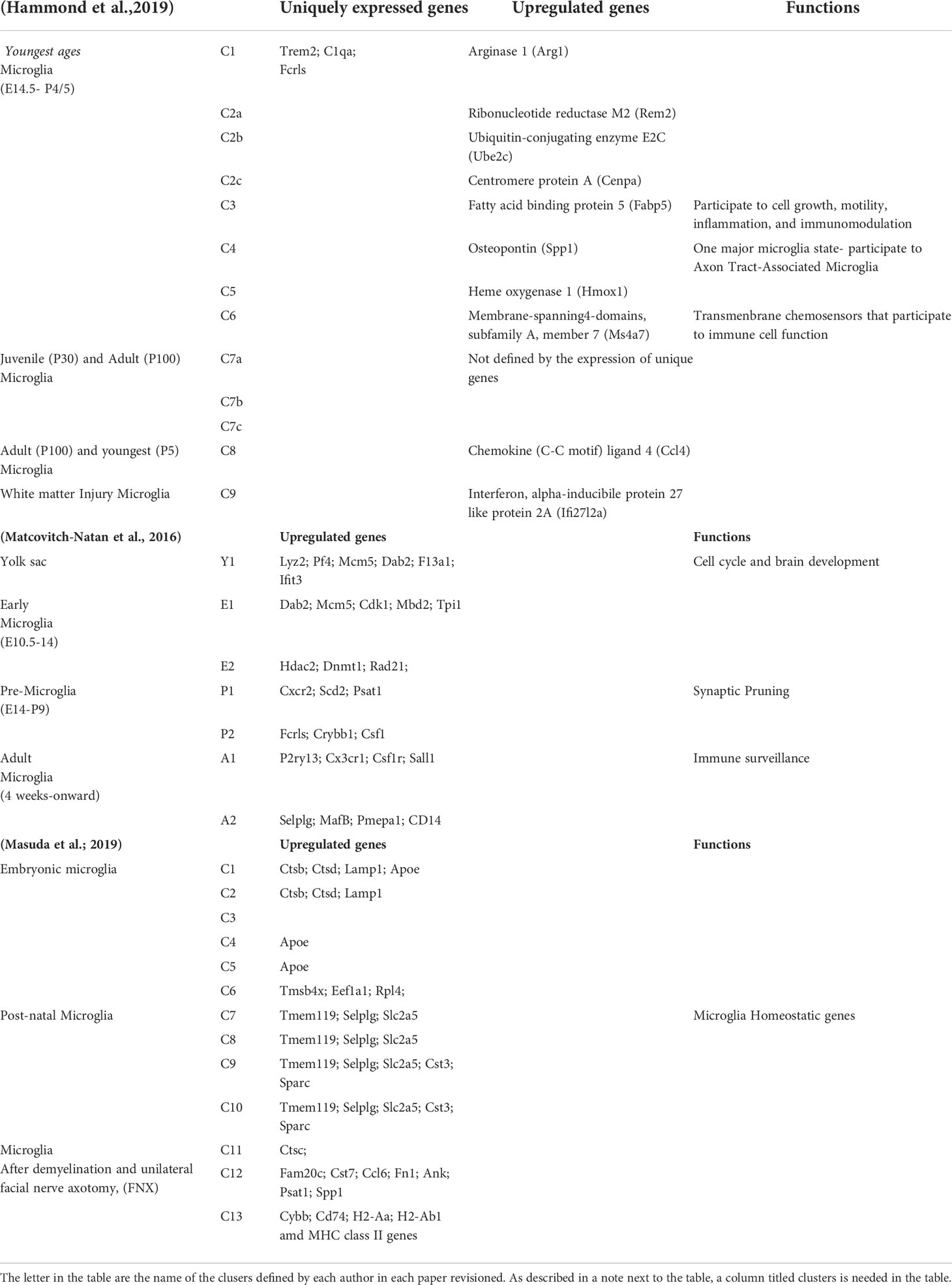
Table 1 Microglia identity and spatial heterogeneity: Recapitulation of expressed markers and functions of clustered microglia in different temporal and spatial conditions.
Microglia functions in the brain
Microglia in healthy brain
Microglia represent the resident myeloid cells that inhabit the cerebral parenchyma and contribute to its surveillance and maintenance. Among the homeostatic functions of microglial cells, their neuromodulatory properties have been deeply documented (62). These activities are made possible by the presence of several receptors on microglial cells, among them, purinergic receptors which recognize adenosine triphosphate (ATP) and their metabolites, signals commonly used by neurons to communicate with microglia (63). Bidirectional communication between microglial cells and neurons is also important to stimulate the movement of microglial cells towards neurons to modulate their activity (63). Recently, ATP-dependent microglia activation has been correlated to an inhibitory program to reduce excessive/dangerous neuronal activity (64). This stimulation of purinergic receptors increases the release of BDNF, which plays an important role in brain neuromodulation; in particular, this neurotrophic factor is implicated in both neuronal differentiation and synaptic plasticity (65); specific depletion of BDNF from microglia does not modify its brain levels but reduced the formation of new synapses in the cerebral motor cortex and the motor learning abilities in mice (66). Among the physiological activities of microglia, it has been recently shown that these cells participate in the regulation of sleep, in mice. It has been described that the accumulation of ATP during the sleep phase reduces the microglial expression of CX3CR1 and that microglial depletion increases the duration of NREM sleep during the wake phase, with a simultaneous reduction of the hippocampal excitatory neurotransmission in mice (67). Altogether, these data indicate that, under physiological conditions, microglia participate both in the homeostatic balance and synaptic activity of the brain microenvironment.
Microglia in brain diseases
Considering the fundamental role of microglial cells in the brain, it is not surprising that they also participate in brain disorders, as well as inflammatory diseases of the brain. Alzheimer’s disease (AD) is a progressive neurodegenerative disorder characterized by general cognitive impairment and with two distinctive pathological signs: extracellular plaques composed of amyloid β-peptide (Aβ) and intraneuronal tangles composed of hyperphosphorylated tau proteins. Both patients and animal models of this pathology are characterized by microglial cells with altered phagocytic activity around the amyloid plaques (68). In animal models of AD, extensive genome-wide association studies (GWAS) have identified many immune-related genes associated with high-risk factors for this pathology, which are highly expressed in microglial cells (48). Among these genes, mutated forms of TREM2 represent a pivotal risk factor for this neurodegenerative disease (69). TREM2 controls the activity of microglial in synapses, and the elimination or mutations on this receptor contribute to the deposition of amyloid plaques in mice brains (70) as well as to an energy imbalance that increases microglial cell dysfunction (71). These data, in agreement with others, attribute to microglial cells an active role in fighting AD since it is possible that wrapping the amyloid plaques helps to form a protective barrier that reduces the neurodegeneration process progression. In different neurodegenerative diseases, such as Parkinson’s disease characterized by motor impairment, microglia behave differently, contributing to neuroinflammation and speeding up neurodegeneration. For instance, mutations in leucine-rich repeat kinase 2 (LRRK2) are associated with different forms of PD (monogenic and sporadic) in an age-dependent manner (72). LRRK2 is a serine/threonine kinase (73), and mutations in this protein increase kinase activity and contribute to neurodegeneration in PD (74). LRRK2 increases the pro-inflammatory phenotype in α-synuclein-treated microglia (75), whereas in LRRK2-deficient rats the inflammatory phenotype mediated by this fibrillar aggregate is reduced (76). Even if the in vivo effects of this mutated protein on microglial cells are controversial, the relationship between inflammation and LRRK2 on resident brain macrophages is defined. In particular, exposure of microglia to LPS increases the inflammatory phenotype and the level of LRRK2, while inhibition of LRRK2 reduces the production of pro-inflammatory cytokines (77). In addition, LRRK2 appears to regulate microglial motility, reducing its migration (78) and favoring the local inflammation state. Together, these data strengthen the knowledge of the role of LRR2 in neurodegeneration and the effect of microglial cells as operators of the inflammatory state observed in PD. Among diseases affecting the brain, synchronized hyperactivation of neural cells causes epilepsy, a neuropsychiatric disorder that occurs when excitatory activation of neurons exceeds the inhibitory (79). Changes in microglia were also observed after seizures: in the hippocampus of mice treated with kainic acid, microglial cells showed an activation that persists even 24 hours after injections as a side effect of hyperactivity (80, 81). Other evidence support the contribution of microglial cells to brain hyperactivity due to inflammatory response. In particular, the release of cytokines stimulates neuronal activity, favoring epileptogenesis (82) The activation of TLRs, which promote microglial activity, affects seizures: mice lacking the TLR3 or mice treated with TLR4 antagonist show an attenuation of recurrent spontaneous seizures after injections of pilocarpine (83) and kainate (84), respectively. Neuroinflammation is a common condition in brain diseases, and since microglia are the main cells that direct inflammation in the brain, it is not surprising that they are involved in many neural disorders. Among mental illnesses, depression is a condition that affects people and causes emotional suffering; the events that can generate the depressive state are many, however, a common condition is the inflammatory state of the brain. Interferon-gamma (IFN-γ) is a cytokine that promotes microglial activation: Zhang and colleagues demonstrated that intracerebroventricular injection of IFN-γ impaired hippocampal neurogenesis and induced depressive-like behaviors in mice, effects reduced using minocycline to inhibit microglia (85).The works described until now, clearly demonstrated that microglia have distinct roles in a healthy brain, organizing the functions to maintain homeostatic balance; however, there are clear proofs supporting the contribution of microglial cells even in brain disease, when these cells can improve or worsen disease progression. Together, these evidence suggest that microglial cells could represent a promising therapeutic target for brain diseases.
Gut microbiota-derived molecules controlling microglia in healthy and diseased brain
Microbiota is the term used to describe all the microorganisms (bacteria, yeasts, archaea, protozoa and viruses) living on the epithelial barriers of the human body (nose, eye, skin, lungs, gastrointestinal, genital and urinary tract). The human gut is the natural habitat for more than 100 trillion of microorganisms. The intestinal microbiome, i.e. the totality of the genetic heritage owned by the intestinal microbiota, encodes over three millions of genes and produces thousands of molecules important for host health. These molecules exert both local and peripheral effects maintaining the homeostasis of intestine and distant organs. Small molecules, such as the short chain fatty acids (SCFAs), lipopolysaccharide (LPS) or tryptophan (trp) metabolites are produced in the colon and largely absorbed by the colonocytes; part of them reach the systemic circulation, playing key roles in microbiota-gut-brain cross-talk (Figure 1). A second route for gut-brain communication is represented by signal through the vagal nerve. It ensures bidirectional communication, using both neurotransmitters such as serotonin and glutamate and, gut hormones (Figure 1). Healthy gut microbiota is fundamental for maintaining normal host functioning; indeed, alteration of the gut microbiota and its metabolites, defined as gut dysbiosis, can induce harmful effects on the host. The imbalance in microbiota composition leads to a wide range of inflammatory and metabolic pathologies including brain diseases (86–89). In the following paragraphs the most studied gut microbiota-derived molecules, vagal nerve stimulation and their effects on microglia will be reviewed.
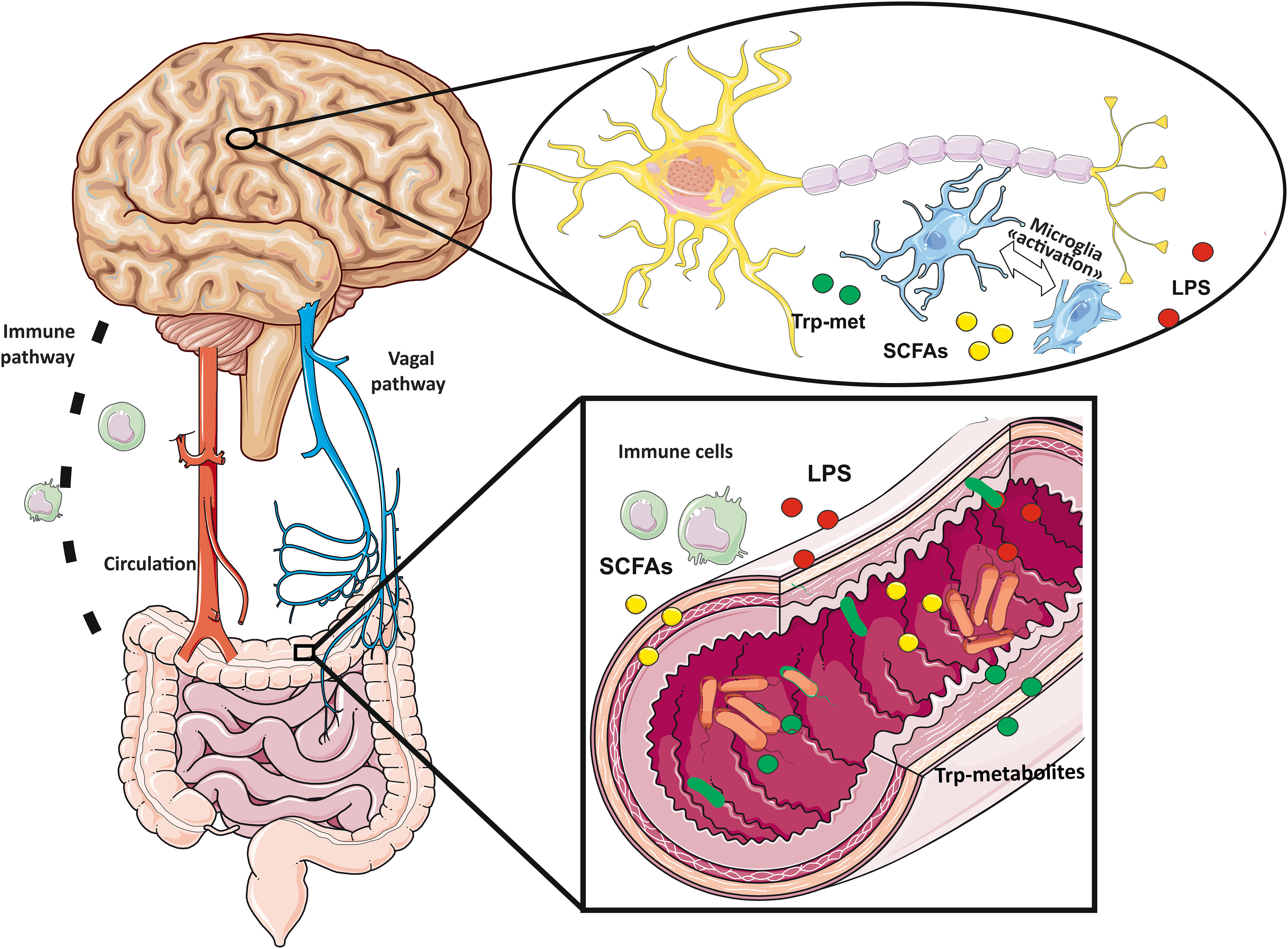
Figure 1 Gut-brain axis follows bidirectional communication routes: vagal nerve, immune system pathways and circulating small molecules produced by bacteria (tryptophan derived metabolites, short chain fatty acids and lipopolysaccharides) reaching the brain through the circulation.
SCFAs and microglia
The fermentation of fibers contained in the food by colon microbes produces bioactive molecules. Among them, the short-chain fatty acids (SCFAs; e.g., formate, valerate, acetate, propionate, and butyrate) which are small molecules ranging from 1 to 4 atoms of carbon. It has been shown that SCFAs can act through either G-protein-coupled receptors (GPCRs) or histone deacetylases (HDACs) (90, 91).
One of the first evidence showing the role of microbiota-derived molecules on microglia was obtained in germ-free (GF) mice by Erny and colleagues in 2015. The authors showed that GF mice displayed global defects in microglia, with altered cell shape and immature phenotype, leading to impaired innate immune responses. Classical SCFAs supplementation to GF mice reverted microglial phenotype and lipopolysaccharide response (92).
Later, the same authors identified microbiota-derived acetate as critical driver of microglia maturation and regulator of the homeostatic metabolic state in germ free mice. Further, they also show the ability of acetate to modulate microglial phagocytosis and disease progression in one murine model of Alzheimer’s disease (93). Recently, it has been observed that SCFAs can reverse the age-related pro inflammatory state of microglia. In aged mice, the supplementation of inulin, a prebiotic fermentable fiber, increased the endogenous levels of SCFAs and reduced the expression of many inflammatory genes, as demonstrated by scRNA-seq on microglial cells. In addition, microglia from aged mice spontaneously secreted more TNF-α than microglia from adults and this effect was reduced in microglia of aged mice fed with inulin (94). On the other hand, a fiber-deprived diet altered gut microbiome, reducing SCFAs and inducing cognitive impairment in mice. In particular, dietary fiber deficiency for 15 weeks correlated with microglia-mediated synaptic loss in the hippocampus, suggesting that fiber intake could represent a nutritional preventive strategy to reduce the risk of cognitive decline and neurodegenerative disease (95). In mouse models of Alzheimer’s disease, the expression of the synapse-associated proteins (Postsynaptic Density protein- 95-PSD-95; Synaptophisyn-SYP; N-methyl-D-aspartate receptor 2 B -NR2B) are reduced and the pro-inflammatory cytokines (TNF-α, IL-6, IL-1β) are increased. These alterations could be reversed treating mice daily, for 2 weeks, at early stage of disease, with sodium butyrate by intraperitoneal injection. In particular, SCFA treatment suppressed the over-activation of microglia and the accumulation of Aβ in AD mice (96). It has been shown by Sadler and colleagues that stroke alters the gut microbiota composition, inducing dysbiosis and a plasma decrease of SCFAs. The authors showed that classical SCFAs supplementation in drinking water for 4 weeks improved the behavioral outcome after stroke, reducing brain microgliosis and the expression of the phagocytosis marker CD68 in the ipsilateral hemispheric cortex (97). Housing rodents in an enriched environment (EE), a condition characterized by abundant social interactions, and cognitive, sensory, and motor stimulations could improve their learning and memory abilities (98). Recently, it has been shown that housing mice in an EE affects their gut microbiota and metabolome composition, elevating the concentration of specific SCFAs, with effects on synaptic plasticity processes (99). In the same year, Lupori and colleagues showed that classical SCFAs (acetate, propionate and butyrate) treatment changed microglia morphology in the visual cortex toward a hyper-ramified condition characteristic of high plasticity, similar to EE, favoring ocular dominance plasticity process (100). On the other hand, in a model of neuropathic pain induced by chronic constriction injury (CCI), Zhou and colleagues observed signs of mechanical and thermal pain, and an increased expressions of microglia (Iba1, CD11b) and pro-inflammatory markers (CD68, IL-1β, and TNF-α) both in the hippocampus and the spinal cord. These phenomena were accompanied by increased level of SCFAs in the gut. Antibiotic treatment reversed microglia activation but its action was blocked by SCFA administration, suggesting these molecules as key player in the pathogenesis of neuropathic pain (101). Recently, in α-synuclein overexpression and MPTP (1-methyl-4-phenyl-1,2,3,6-tetrahydropyridine) models of Parkinson’s disease (PD), the oral supplementation with SCFAs (mixture of acetate, propionate and butyrate or butyrate alone) worsened the disease progression, increasing microglia activation and TNF-α release (102, 103). Further researches are needed to clearly define the role of SCFAs, considering both the beneficial and detrimental effects described above on microglia and neuroinflammatory diseases.
As reviewed above, authors mainly described the effect of SCFAs mixture in vivo. In contrast, Wenzel and colleagues described the effect of single or combined treatment on human microglia cell line THP-1 with acetate, propionate, butyrate, formate, and valerate at an approximate physiological concentration ratio. They found that the SCFA mixture, as well as single SCFAs (at the highest concentrations used in the mixture, i.e. from 15 to 236 μM), decreased the secretion of IL-1β, MCP-1, TNF-α, and cytotoxins induced by LPS stimulation. In addition, formate and valerate reduced the phagocytic activity of LPS-stimulated THP-1 cells. Formate, but not valerate, also inhibited the N-formylmethionine-leucyl-phenylalanine (fMLP)-induced respiratory burst of HL-60 cells, reducing the production of reactive oxygen species (ROS) (104).
Altogether, these researches identify SCFAs as the key soluble links along the gut-brain axis and propose them as potential therapeutic tools to target microglia-related brain diseases.
LPS and microglia
Changes in the gut microbiota leading to the expansion of gram-negative bacteria increase the release of microbial LPS, a bacteria cell wall component. LPS activates the TLRs, membrane-spanning receptors expressed in microglial cells, which recognize common damage- or pathogen-associated molecular-patterns (DAMPS, PAMPs) (105). LPS can stimulate host immunity and the immunological response depends on the origin of the microbial species. Indeed, the lipid A portion of LPS is variable and contains the endotoxic component, thus contributing to the structural and functional diversity of LPSs among microbial species (106). In this regard, an in vitro study showed that exposure of rat neonatal microglia to LPS from Cyanobacterium Oscillatoria sp. resulted in a concomitant release of proinflammatory and anti-inflammatory mediators (107). In contrast, a study that treated rat microglia with LPS from Cyanobacterium Microcystis aeruginosa described the induction of a classical (or M1-like) microglia state and the release of pro-inflammatory mediators (108).
It has been shown that stress decreases the level of Lactobacillus and Bifidobacterium and increases the gram-negative bacteria, with an increase of LPS (109, 110). Chronic LPS release activates systemic inflammation and neuroinflammation, both effects being crucial for the pathogenesis of neurodegenerative and psychiatric disorders (111). Indeed, elevated levels of LPS are observed in amyotrophic lateral sclerosis (112), AD (113) and severe autism (114).
Neuroinflammation, indeed, mainly comprises the over activation of microglia cells that can became harmful for brain parenchyma.
It has been shown that intraperitoneal injection of LPS increases the number of Iba-1 positive cells in different brain region, in a dose-dependent manner in conventional mice, but not in GF mice (115, 116) underlying the key role of microbiota in driving LPS-mediated effects.
As reviewed, several factors can alter the abundance of LPS in the host; however, the actual role of microbiota derived -LPS in activating microglia remains to be investigated.
Tryptophan-derived ligands of aryl hydrocarbon receptor and microglia
In addition to SCFAs, it has been recently demonstrated that aryl hydrocarbon receptor (AhR) ligands secreted by gut bacteria can influence microglia. Tryptophanase-expressing bacteria catalyze the conversion of dietary tryptophan to indole, the precursor for the synthesis of AhR agonists (indoxyl-3-sulfate and indole-3-propionic acid). Rothhammer and colleagues showed that AhR activation by dietary tryptophan (Trp) metabolites suppressed the microglial expression of NF-κB dependent transcripts such as Tnfa and regulated microglial expression of Tgfa and Vegfb in a mouse model of multiple sclerosis, the experimental autoimmune encephalomyelitis (EAE) (117, 118). The same effects were shown in human microglia, activating AhR by the tryptophan metabolite indoxyl-3-sulfate (I3S). AhR activation suppressed the expression of pro-inflammatory and neurotoxic genes (TNFA, IL6, IL12A, NOS2) and boosted the expression of anti-inflammatory IL10; AhR activation also induced TGFA and reduced VEGFB expression in human microglia (118). The dietary metabolites and other AhR ligands, such as indirubin-3′-oxime, were also shown to inhibit the inflammatory phenotype of microglial cells in rat brain (119). On the other hand, in a model of mouse middle cerebral artery occlusion (MCAO), Tanaka and colleagues showed that AhR expression increased in microglia during ischemia. Pre-treatment with an AhR antagonist, CH223191, significantly reduced the expression of TNFα, IL-1β and cyclooxygenase-2 (COX-2) in the cortex and striatum of MCAO mice (120). Altogether, these data suggest that microbial tryptophan and other AhR ligands can modulate microglia, adding new therapeutic targets for brain diseases with inflammatory components.
Quorum sensing peptides and microglia
Among the bacterial derived molecules able to modulate microglia, the quorum sensing peptides (QSPs) have been recently described. QSPs are oligopeptides produced by Gram-positive bacteria to communicate with their peers in a cell-density dependent manner. In particular, Janssens and colleagues described the ability of an active heptapeptide (SDLPFEH, named PapRIV) originating from members of Bacillus cereus group. The authors showed that PapRIV is present in mouse plasma and that can reach the brain, crossing the brain blood barrier (121). In addition, they described that PaPRIV has pro-inflammatory effects on the microglial BV-2 cells, inducing the expression of IL-6 and TNF-α, along with an increase of intracellular ROS. This is the first evidence for a possible role of this bacterial quorum sensing peptide in gut-to-brain signaling (121).
Vagal nerve stimulation and microglia
Vagal afferent neurons located in the nodose ganglion (NG) innervate the gut and terminate in the nucleus of solitary tract (NST) of the brainstem. Vagal afferent terminals are located below the gut epithelium, where they sense signals derived from the gut microbiota, with effects on host behavior. The NST is a target for gastrointestinal signals modulating satiety, and alterations in the gut-brain vagal pathway may promote overeating and obesity. In rats treated with high fat diet (HFD), the alteration of microbiota composition triggered the reorganization of vagal afferents and the activation of microglial cells in the NST (122). Similar data were obtained in rats fed with low fat/high sugar diet (123). Kim and colleagues demonstrated that HFD-induced dysbiosis, per se, decreased vagal innervation and increased Iba1 positive cells in the NG of GF rats which received fecal transplantation from HFD- (45 or 60% fat) or low-fat diet- (LFD, 13% fat) treated rats (124). These studies suggested that HFD-triggered shift in gut microbiome may affect the vagal gut-brain communication resulting in microglia activation and increased body fat accumulation.
It has been reported that the electrical stimulation of the vagus nerve, in the presence of a peripheral immune challenge may affect microglial cells, up-regulating anti-inflammatory pathways in the brain (125, 126). Vagus nerve stimulation, combined with LPS challenge, decreases microglial production of the pro-inflammatory cytokines IL-6, IL-1β, and TNFα, an effect abolished upon vagotomy (127). These data suggested that vagus nerve activity reduces neuroinflammation. In line with this observation, in a rat model of transient ischemia, berberine, an alkaloid with weak antibiotic properties, decreased CD86-positive and increased CD163-positive microglial cells in the cerebral cortex, in a microbiota-dependent manner. The authors show that the hydrogen sulfide (H2S) derived from microbiota metabolization of berberine, stimulates the vagus nerve through the transient receptor potential vanilloid 1 (TRPV1) and that a cocktail of antibiotics blocked vagal activation, confirming the necessity of a functional metabolizing microbiota (128).
Recently, Yunpeng and colleagues showed that Lactobacillus rhamnosus (JB-1) has anxiolytic effects and decreases Iba1+ microglial cells in mouse hippocampus. The authors also demonstrated that the loss of vagal integrity, obtained by subdiaphragmatic vagotomy, inhibited these effects, further pointing to the important role of vagus nerve in the signaling between the gut microbiota and the brain (129). Although different studies performed in different models reported that microglial cells are modulated by vagal activation, the relationships between the vagus nerve and the immune signaling, in microbiota-gut-brain communication, need further investigation.
Gut microbiota modulation by antibiotics in healthy and disease: The effects on microglia
The use of antibiotics (ABX) to eliminate or unbalance the gut microbiota is a useful tool to study the relationship of specific bacterial cohorts and different brain functions in adult animal models. At difference with the GF mice, which permit to study the role of the gut microbiota during development, ABX, especially the non-absorbable ones, open to the opportunity to study the role of gut microbes and their associated molecules in healthy mice or murine brain disease models.
In adult healthy mice, two-weeks of ABX treatment influence hippocampal microglial density, reducing their basal patrolling activity, and impairing process rearrangement in response to damages (130). In addition, ABX treatment induced a reduction of spontaneous postsynaptic glutamatergic currents and in general, of synaptic connectivity (110).
Interestingly, in adult healthy mice, it has been demonstrated (by RNA-seq of FACS-purified microglia) that one-week of ABX treatment triggered transcriptomic changes in microglia, in a sex dependent manner. In particular, Thion and colleagues showed that ABX treatment induced different expression of 92 microglial genes in male mice, and of only 40 genes in female mice, suggesting a sexually dimorphic impact of microbiota on microglial transcriptomes (131).
A more prolonged (4 weeks) ABX treatment, aimed to fully eradicate the gut microbiota, led to microglia activation, with an increase of CD40 and MHC II expression, IL-6 and TNF-α production, and more Iba1+ cells in the hippocampal CA3 and CA1 regions (112). Theis treatment also induced a reduction in the synaptic transmission measured as hippocampal cholinergic gamma power oscillations in the CA3, after ABX treatment (132).
Recently, many reports showed that the intestinal microbiota also influences the neurodevelopment, the behavior, and contributes to brain disorders. On the other hand, gut microbiota dysbiosis is observed in a number of brain disorders characterized by microglia dysfunction such as autism spectrum disorders (ASD), schizophrenia, AD, major depressive disorder, and PD (133–144)
In a murine model of Herpes simplex encephalitis (HSE), a complication of herpes simplex virus type I infection, Li and colleagues observed alterations in gut microbiota composition. In these mice, the use of oral ABX aggravates the virus-induced pathology, triggering the pro inflammatory activation of microglia. The administration of another microbial derived product, nicotinamide n-oxide, significantly diminished inflammation in ABX-treated or untreated HSE mice (145).
In a murine model of familial ALS (hSOD1G93A mice), it has been shown that a combination of 8 antibiotics (146) worsened the motor functions and reduced survival, exacerbating the pro-inflammatory phenotype of microglial cells in the spinal cord. The authors found that ABX treatment reduced the relative abundance of Akkermansia and of butyrate-producing bacteria such as Clostridium g24, Ruminococcus, and genera in the Lachnospiraceae family, that are triggers of anti-inflammatory responses (147, 148). These data suggest that these microbes could play a beneficial role at least in the familial form of ALS (149). Similarly, in a murine model of AD (the APPS1 model) the administration of Akkermansia muciniphila by oral gavage, every day for 6 months resulted in a delay of the pathological changes in the brain and ameliorated the spatial learning and memory tests (150).
On the other hand, it has been shown that, in two independent transgenic mouse models of AD (APPSWE/PS1ΔE9 and APPPS1-21(APPSWE/PS1L166P mice), only males showed reduced Aβ amyloidosis and altered phenotypes of plaque-associated microglia following administration of an ABX cocktail. In particular, ABX treatment altered the levels of selected microglial transcripts for homeostatic proteins in male but not in female mice (151, 152). More recently, the same authors showed that CSF-1R inhibitor-mediated depletion of microglia in ABX-treated male mice failed to reduce cerebral Aβ amyloidosis. Thus, they demonstrated the key role of microglia in bridging gut microbiota-mediated modulation of cerebral Aβ deposition (153). In a different mouse model of AD (5 x Familial AD, 5xFAD), it has been recently shown that GF or ABX treated mice differentially control the microglial mechanisms of Aβ clearance, preventing both neurodegeneration and cognitive deficits. While both conditions attenuated hippocampal pathological signs, only GF 5xFAD mice enhanced microglial Aβ uptake at early stages compared to ABX-treated 5xFAD mice. These observations were supported by RNA-sequencing of hippocampal microglia from control, GF and ABX-treated 5xFAD mice, that showed distinct profiles of microbiota-dependent gene expression associated with phagocytosis and altered microglial activation states (154). Different genetics and ABX treatments lead to different outcomes in microglia activation in the context of the same pathology, suggesting a plastic role of gut microbiota-microglia axis in AD.
In the last decade, the importance of gut microbiota in multiple sclerosis (MS) has been highlighted: many studies reported the presence of gut microbiota dysbiosis in patients (155–157).
In a murine model of progressive MS triggered by intracranial infection with Theiler’s murine encephalomyelitis virus (TMEV), Mestre and colleagues treated mice with oral ABX in the pre-symptomatic and symptomatic phases of the disease. The cocktail of ABX prevented motor dysfunction and limited axon damage in mice. In the spinal cord of TMEV mice treated with ABX, microglia assume a round amoeboid morphology associated to an anti-inflammatory gene profile (increase of IL-4 and IL-10) (158). Similar results were obtained in a different murine model of MS where a pretreatment of either three or seven days with oral ABX cocktail (Ampicillin, Metronidazole, Neomycin Sulfate, and Vancomycin) protected mice from signs of EAE. In addition, RT-PCR data on spinal cord tissue showed an upregulation of Arg1, a gene associated with anti-inflammatory microglia/macrophages and a downregulation of the pro-inflammatory genes iNos and TNF-α (159).
ABX treatment resulted beneficial also in one murine model of PD with α-synuclein (αSyn) overexpression, showing motor dysfunction and gastrointestinal (GI) constipation. In these mice, Sampson and colleagues reported that ABX treatment resulted in milder αSyn-dependent motor dysfunction and an increased GI function. Microglia showed morphological changes that indicated an arrest in maturation and/or a reduced activation state, suggesting that postnatal signaling between the gut and the brain modulates the disease (102).
Recently, the effect of gut microbiota alteration was studied also in brain tumor. Treating mice with two non-absorbable ABX (gentamicin and vancomycin) for two weeks prior to glioma transplantation in the brain reduced the cytotoxic NK cell subsets and altered the expression of inflammatory and homeostatic proteins in microglia. All these effects could contribute to the increased growth of intracranial glioma observed in ABX-treated mice (160).
In one rat model of major depression, where animals show high anxiety-like behavior, lower microglial numbers in prefrontal cortex and altered gut microbiota composition, Schmidtner and colleagues demonstrated that three-week treatment with oral minocycline, a broad-spectrum tetracycline antibiotic, alleviated the depressive-like phenotype. In addition, this treatment further reduced prefrontal microglial density, exclusively in male rats, and reduced the plasma concentrations of pro-inflammatory cytokines. These results support the microbiome-gut-brain axis as potential target in the treatment of depression (161).
The modulation of gut microbiota by antibiotics revealed several unexpected gut-brain links in many pathologies, worth to be further elucidated. However, ABX treatment could be also considered a new therapeutic strategy to be used not only for infectious diseases, but also for brain disorders associated with gut microbiota dysbiosis. Finally, it must be considered that there are many other ways to modulate the microbiota and therefore the microglia, not discussed here. These include, for example, fecal microbiota transplantation, administration of pro/prebiotics (e.g. sodium oligomannate) and/or live biotherapeutics.
Closing remarks
A number of recent studies demonstrated that the gut microbiota is a powerful modulator of microglial phenotype and functioning, in healthy and disease conditions. However, the molecular mechanisms used by the gut microbiota and the metabolome to impact host neuroimmune cells need further investigations. The availability of new DNA sequencing strategies, such as shotgun metagenomic sequencing and metabolomics will allow the analysis of gut microbiome composition at the level of species and will permit the identification of specific microbe cohorts and their cross-correlation with microbial metabolites in the different conditions. Therefore, we propose to investigate how the modulation of specific bacterial species or cohorts (by specifically depleting or enriching them) might result in microglia alteration in the brain of healthy and diseased murine models. Further, manipulation of microbiota composition by pre-biotics, environmental stimuli, dietary habits, and fecal transplantation might represent new therapeutic strategies to for the treatment of brain disorders involving microglia.
Author contributions
CL, GD and FM contributed to the conception, design, and writing of the manuscript. FM and GD designed the figure. All authors have read and agreed to the published version of the manuscript. All authors contributed to the article and approved the submitted version.
Funding
This work was supported by PRIN 2017, AIRC 2019 -IG 23010, RF-2018-12366215 to CL and by the European Union’s Horizon 2020 Research and Innovation Program under grant agreement No. 952455; EpiEpiNet (CL); GR-2016-02363254 to GD.
Conflict of interest
The authors declare that the research was conducted in the absence of any commercial or financial relationships that could be construed as a potential conflict of interest.
Publisher’s note
All claims expressed in this article are solely those of the authors and do not necessarily represent those of their affiliated organizations, or those of the publisher, the editors and the reviewers. Any product that may be evaluated in this article, or claim that may be made by its manufacturer, is not guaranteed or endorsed by the publisher.
References
1. Prinz M, Jung S, Priller J. Microglia biology: One century of evolving concepts. Cell (2019) 179:292–311. doi: 10.1016/J.CELL.2019.08.053
2. Sierra A, Paolicelli RC, Kettenmann H. Cien años de microglía: Milestones in a century of microglial research. Trends Neurosci (2019) 42:778–92. doi: 10.1016/J.TINS.2019.09.004
3. Crotti A, Ransohoff ,RM. Microglial physiology and pathophysiology: Insights from genome-wide transcriptional profiling. Immunity (2016) 44:505–15. doi: 10.1016/J.IMMUNI.2016.02.013
4. Kato D, Ikegami A, Horiuchi H, Moorhouse AJ, Nabekura J, Wake H. In vivo two-photon imaging of microglial synapse contacts. Methods Mol Biol (Humana Press Inc.) (2019) 2034:281–6. doi: 10.1007/978-1-4939-9658-2_20
5. David LA, Maurice CF, Carmody RN, Gootenberg DB, Button JE, Wolfe BE, et al. Diet rapidly and reproducibly alters the human gut microbiome. Nature (2014) 505:559–63. doi: 10.1038/nature12820
6. de Angelis M, Garruti G, Minervini F, Bonfrate L, Portincasa P, Gobbetti M. The food-gut human axis: The effects of diet on gut microbiota and metabolome. Curr Medicinal Chem (2017) 26:3567–83. doi: 10.2174/0929867324666170428103848
7. Singh RK, Chang H-W, Yan D, Lee KM, Ucmak D, Wong K, et al. Influence of diet on the gut microbiome and implications for human health. J Transl Med (2017) 15:73. doi: 10.1186/s12967-017-1175-y
8. Zmora N, Suez J, Elinav E. You are what you eat: diet, health and the gut microbiota. Nat Rev Gastroenterol Hepatol (2019) 16:35–56. doi: 10.1038/s41575-018-0061-2
9. Davalos D, Grutzendler J, Yang G, Kim J, Zuo Y, Jung S, et al. ATP mediates rapid microglial response to local brain injury in vivo. Nat Neurosci (2005) 8:752–8. doi: 10.1038/nn1472
10. Sierra A, de Castro F, del Rıo-Hortega JR, Rafael Iglesias-Rozas J, Garrosa M, Kettenmann H. The “Big-bang” for modern glial biology: Translation and comments on p ıo del rıo-hortega 1919 series of papers on microglia. (2016) 64(11):1801–40. doi: 10.1002/glia.23046
11. Nimmerjahn A, Kirchhoff F, Helmchen F. Neuroscience: Resting microglial cells are highly dynamic surveillants of brain parenchyma in vivo. Sci (1979) (2005) 308(5726):1314–8. doi: 10.1126/science.1110647
12. Ginhoux F, Greter M, Leboeuf M, Nandi S, See P, Gokhan S, et al. Fate mapping analysis reveals that adult microglia derive from primitive macrophages. Sci (1979) (2010) 330(6005):841–5. doi: 10.1126/science.1194637
13. Bruttger J, Karram K, Wörtge S, Regen T, Marini F, Hoppmann N, et al. Genetic cell ablation reveals clusters of local self-renewing microglia in the mammalian central nervous system. Immunity (2015) 43:92–106. doi: 10.1016/J.IMMUNI.2015.06.012
14. Cugurra A, Mamuladze T, Rustenhoven J, Dykstra T, Beroshvili G, Greenberg ZJ, et al. Skull and vertebral bone marrow are myeloid cell reservoirs for the meninges and CNS parenchyma. Sci (1979) (2021) 373(6553):eabf7844. doi: 10.1126/science.abf7844
15. Shechter R, London A, Schwartz M. Orchestrated leukocyte recruitment to immune-privileged sites: Absolute barriers versus educational gates. Nat Rev Immunol (2013) 13(3):206–18. doi: 10.1038/nri3391
16. van Hove H, Martens L, Scheyltjens I, de Vlaminck K, Pombo Antunes AR, de Prijck S, et al. A single-cell atlas of mouse brain macrophages reveals unique transcriptional identities shaped by ontogeny and tissue environment. Nat Neurosci (2019) 22:1021–35. doi: 10.1038/s41593-019-0393-4
17. Jordão MJC, Sankowski R, Brendecke SM, Sagar, Locatelli G, Tai YH, et al. Neuroimmunology: Single-cell profiling identifies myeloid cell subsets with distinct fates during neuroinflammation. Sci (1979) (2019) 363(6425):eaat7554. doi: 10.1126/science.aat7554
18. Mrdjen D, Pavlovic A, Hartmann FJ, Schreiner B, Utz SG, Leung BP, et al. High-dimensional single-cell mapping of central nervous system immune cells reveals distinct myeloid subsets in health, aging, and disease. Immunity (2018) 48:380–395.e6. doi: 10.1016/j.immuni.2018.01.011
19. Hammond TR, Robinton D, Stevens B. Microglia and the brain: Complementary partners in development and disease. Annu Rev Cell Dev Biol (2018) 34:523–44. doi: 10.1146/annurev-cellbio-100616-060509
20. Li Q, Barres BA. Microglia and macrophages in brain homeostasis and disease. Nat Rev Immunol (2018) 18(4):225–42. doi: 10.1038/nri.2017.125
21. Paloneva J, Kestilä M, Wu J, Salminen A, Böhling T, Ruotsalainen V, et al. Loss-of-function mutations in TYROBP (DAP12) result in a presenile dementia with bone cysts. Nat Genet (2000) 25(3):357–61. doi: 10.1038/77153
22. Rademakers R, Baker M, Nicholson AM, Rutherford NJ, Finch N, Soto-Ortolaza A, et al. Mutations in the colony stimulating factor 1 receptor (CSF1R) gene cause hereditary diffuse leukoencephalopathy with spheroids. Nat Publishing Group (2011) 44(2):200–5. doi: 10.1038/ng.1027
23. Salter MW, Stevens B. Microglia emerge as central players in brain disease. Nat Med (2017) 23:1018–27. doi: 10.1038/nm.4397
24. Huangi L, Jin J, Chen K, You S, Zhang H, Sideris A, et al. BDNF produced by cerebral microglia promotes cortical plasticity and pain hypersensitivity after peripheral nerve injury. PloS Biol (2021) 19(7):e300133. doi: 10.1371/journal.pbio.3001337
25. Kellner Y, Gödecke N, Dierkes T, Thieme N, Zagrebelsky M, Korte M. The BDNF effects on dendritic spines of mature hippocampal neurons depend on neuronal activity. Front Synaptic Neurosci (2014) 6:5. doi: 10.3389/fnsyn.2014.00005
26. Zhou LJ, Peng J, Xu YN, Zeng WJ, Zhang J, Wei X, et al. Microglia are indispensable for synaptic plasticity in the spinal dorsal horn and chronic pain. Cell Rep (2019) 27(13):3844–3859.e6. doi: 10.1016/j.celrep.2019.05.087
27. Nakanishi M, Niidome T, Matsuda S, Akaike A, Kihara T, Sugimoto H. Microglia-derived interleukin-6 and leukaemia inhibitory factor promote astrocytic differentiation of neural stem/progenitor cells. Eur J Neurosci (2007) 25(3):649–58. doi: 10.1111/j.1460-9568.2007.05309.x
28. Shigemoto-Mogami Y, Hoshikawa K, Goldman JE, Sekino Y, Sato K. Microglia enhance neurogenesis and oligodendrogenesis in the early postnatal subventricular zone. J Neurosci (2014) 34(6):2231–43. doi: 10.1523/JNEUROSCI.1619-13.2014
29. Louveau A, Nerrière-Daguin V, Vanhove B, Naveilhan P, Neunlist M, Nicot A, et al. Targeting the CD80/CD86 costimulatory pathway with CTLA4-ig directs microglia toward a repair phenotype and promotes axonal outgrowth. Glia (2015) 3(12):2298–312. doi: 10.1002/glia.22894
30. Clemente D, Ortega MC, Melero-Jerez C, de Castro F. The effect of glia-glia interactions on oligodendrocyte precursor cell biology during development and in demyelinating diseases. Front Cell Neurosci (2013) 7:268. doi: 10.3389/fncel.2013.00268
31. Franklin RJM, Ffrench-Constant C. Regenerating CNS myelin - from mechanisms to experimental medicines. Nat Rev Neurosci (2017) 18(12):753–69. doi: 10.1038/nrn.2017.136
32. Reich DS, Lucchinetti CF, Calabresi PA. Multiple sclerosis. N Engl J Med (2018) 378(2):169–80. doi: 10.1056/NEJMra1401483
33. Lloyd AF, Miron VE. The pro-remyelination properties of microglia in the central nervous system. Nat Rev Neurol (2019) 15(8):447–58. doi: 10.1038/s41582-019-0184-2
34. Cunha MI, Su M, Cantuti-Castelvetri L, Müller SA, Schifferer M, Djannatian M, et al. Pro-inflammatory activation following demyelination is required for myelin clearance and oligodendrogenesis. J Exp Med (2020) 217(5):e20191390. doi: 10.1084/jem.20191390
35. Lloyd AF, Davies CL, Holloway RK, Labrak Y, Ireland G, Carradori. D, et al. Central nervous system regeneration is driven by microglia necroptosis and repopulation. Nat Neurosci (2019) 22(7):1046–52. doi: 10.1038/s41593-019-0418-z
36. Fu R, Shen Q, Xu P, Luo JJ, Tang Y. Phagocytosis of microglia in the central nervous system diseases. Mol Neurobiol (2014) 49(3):1422–34. doi: 10.1007/s12035-013-8620-6
37. Vaux DL, Korsmeyer ,SJ. Cell death in development. Cell (1999) 96(2):245–54. doi: 10.1016/S0092-8674(00)80564-4
38. Cunningham CL, Martínez-Cerdeño V, Noctor ,SC. Microglia regulate the number of neural precursor cells in the developing cerebral cortex. J Neurosci (2013) 33(10):4216–33. doi: 10.1523/JNEUROSCI.3441-12.2013
39. Ueno M, Fujita Y, Tanaka T, Nakamura Y, Kikuta J, Ishii M, et al. Layer V cortical neurons require microglial support for survival during postnatal development. Nature neuroscience (2013) 16(5):543–51. doi: 10.1038/nn.3358
40. Mizutani M, Pino PA, Saederup N, Charo IF, Ransohoff RM, Cardona AE. The fractalkine receptor but not CCR2 is present on microglia from embryonic development throughout adulthood. J Immunol (2012) 188(1):29–36. doi: 10.4049/jimmunol.1100421
41. Wolf Y, Yona S, Kim K-W, Jung S, Sierra A. Microglia, seen from the CX 3 CR1 angle. Frontiers in cellular neuroscience (2013) 7:26. doi: 10.3389/fncel.2013.00026
42. Lisman J, Cooper K, Sehgal M, Silva AJ. Memory formation depends on both synapse-specific modifications of synaptic strength and cell-specific increases in excitability. Nat Neurosci (2018) 21:309–14. doi: 10.1038/s41593-018-0076-6
43. Raghuraman R, Karthikeyan A, Wei WL, Dheen ST, Sajikumar S. Activation of microglia in acute hippocampal slices affects activity-dependent long-term potentiation and synaptic tagging and capture in area CA1. Neurobiol Learn Mem (2019) 163:107039. doi: 10.1016/j.nlm.2019.107039
44. Basilico B, Pagani F, Grimaldi A, Cortese B, Di Angelantonio S, Weinhard L, et al. Microglia shape presynaptic properties at developing glutamatergic synapses. Glia (2019) 67:53–67. doi: 10.1002/glia.23508
45. Wang C, Yue H, Hu Z, Shen Y, Ma J, Li J, et al. Microglia mediate forgetting via complement-dependent synaptic elimination. Science (2020) 367:688. doi: 10.1126/science.aaz2288
46. Weinhard L, di Bartolomei G, Bolasco G, Machado P, Schieber NL, Neniskyte U. Microglia remodel synapses by presynaptic trogocytosis and spine head filopodia induction. Nat Commun (2018) 9(1):1228. doi: 10.1038/s41467-018-03566-5
47. Lehrman EK, Wilton DK, Litvina EY, Welsh CA, Chang ST, Frouin A, et al. CD47 protects synapses from excess microglia-mediated pruning during development. Neuron (2018) 100(1):120–134.e6. doi: 10.1016/j.neuron.2018.09.017
48. Ransohoff RM, Schafer D, Vincent A, Blachère NE, Bar-Or A. Neuroinflammation: Ways in which the immune system affects the brain. Neurotherapeutics (2015). doi: 10.1007/s13311-015-0385-3
49. Schafer DP, Lehrman EK, Kautzman AG, Koyama R, Mardinly AR, Yamasaki R, et al. Microglia sculpt postnatal neural circuits in an activity and complement-dependent manner. Neuron (2012) 74(4):691–705. doi: 10.1016/j.neuron.2012.03.026
50. Lowery RL, Tremblay ME, Hopkins BE, Majewska ,AK. The microglial fractalkine receptor is not required for activity-dependent plasticity in the mouse visual system. GLIA (2017) 65:1744–61. doi: 10.1002/glia.23192
51. Hoshiko M, Arnoux I, Avignone E, Yamamoto N, Audinat E. Deficiency of the microglial receptor CX3CR1 impairs postnatal functional development of thalamocortical synapses in the barrel cortex. J Neurosci (2012) 32:15106–11. doi: 10.1523/JNEUROSCI.1167-12.2012
52. Paolicelli RC, Bolasco G, Pagani F, Maggi L, Scianni M, Panzanelli P, et al. Synaptic pruning by microglia is necessary for normal brain development. Sci (1979) (2011) 333:1456–8. doi: 10.1126/science.1202529
53. Hickman SE, Kingery ND, Ohsumi TK, Borowsky ML. The microglial sensome revealed by direct RNA sequencing. Nat Neurosci (2013) 16(12):1896–905. doi: 10.1038/nn.3554
54. Masuda T, Sankowski R, Staszewski O, Böttcher C. Spatial and temporal heterogeneity of mouse and human microglia at single-cell resolution. Nature (2019) 566(7744):388–92. doi: 10.1038/s41586-019-0924-x
55. Squarzoni P, Oller G, Hoeffel G, Pont-Lezica L. Microglia modulate wiring of the embryonic forebrain. Cell Rep (2014) 8(5):1271–9. doi: 10.1016/j.celrep.2014.07.042
56. Gosselin D, Link VM, Romanoski CE, Fonseca GJ. Environment drives selection and function of enhancers controlling tissue-specific macrophage identities. Cell (2014) 159(6):1327–40. doi: 10.1016/j.cell.2014.11.023
57. Matcovitch-Natan O, Winter DR, Giladi A, Vargas Aguilar S. Microglia development follows a stepwise program to regulate brain homeostasis. Science (2016) .353(6301):aad8670. doi: 10.1126/science.aad8670
58. Saunders A, Macosko EZ, Wysoker A, Goldman M. Molecular diversity and specializations among the cells of the adult mouse brain. Cell (2018) 174(4):1015–1030.e16. doi: 10.1016/j.cell.2018.07.028
59. Hammond TR, Marsh SE, Stevens B. Immune signaling in neurodegeneration. Immunity (2019) 50(4):955–74. doi: 10.1016/j.immuni.2019.03.016
60. Grabert K, Michoel T, Karavolos MH, Clohisey S. Microglial brain region-dependent diversity and selective regional sensitivities to aging. Nat Neurosci (2016) 19(3):504–16. doi: 10.1038/nn.4222
61. Tansley S, Uttam S, Ureña Guzmán A, Yaqubi M. Single-cell RNA sequencing reveals time- and sex-specific responses of mouse spinal cord microglia to peripheral nerve injury and links ApoE to chronic pain. Nat Commun (2022) 13(1):843. doi: 10.1038/s41467-022-28473-8
62. Gomez-Nicola D, Perry VH. Microglial dynamics and role in the healthy and diseased brain: a paradigm of functional plasticity. Neuroscientist (2015) 21(2):169–84. doi: 10.1177/1073858414530512
63. Li Y, Du XF, Liu CS, Wen ZL, Du JL. Reciprocal regulation between resting microglial dynamics and neuronal activity in vivo. Dev Cell (2012) 11;23(6)::1189–202. doi: 10.1016/j.devcel.2012.10.027
64. Badimon A, Strasburger HJ, Ayata P, Chen X. Negative feedback control of neuronal activity by microglia. Nature (2020) 586(7829):417–23. doi: 10.1038/s41586-020-2777-8
65. Colucci-D’amato L, Speranza L, Volpicelli F. Neurotrophic factor bdnf, physiological functions and therapeutic potential in depression, neurodegeneration and brain cancer. Int J Mol Sci (2020) 21:1–29. doi: 10.3390/ijms21207777
66. Parkhurst CN, Yang G, Ninan I, Savas JN, Yates JR, Lafaille JJ, et al. Microglia promote learning-dependent synapse formation through brain-derived neurotrophic factor. Cell (2013) 155:1596–609. doi: 10.1016/j.cell.2013.11.030
67. Corsi G, Picard K, di Castro MA, Garofalo S, Tucci F, Chece G, et al. Microglia modulate hippocampal synaptic transmission and sleep duration along the light/dark cycle. GLIA (2022) 70:89–105. doi: 10.1002/glia.24090
68. Hong S, Dissing-Olesen L, Stevens B. New insights on the role of microglia in synaptic pruning in health and disease. Curr Opin Neurobiol (2016) 36:128–34. doi: 10.1016/j.conb.2015.12.004
69. Filipello F, Morini R, Corradini I, Zerbi V. The microglial innate immune receptor TREM2 is required for synapse elimination and normal brain connectivity. Immunity (2018) 48(5)::979–991.e8. doi: 10.1016/j.immuni.2018.04.016
70. Ay TR, Hirsch AM, Broihier ML, Miller CM. Disease progression-dependent effects of TREM2 deficiency in a mouse model of alzheimer's disease. J Neurosci (2017) 37(3)::637–647. doi: 10.1523/JNEUROSCI.2110-16.2016
71. Ulland TK, Song WM, Huang SC, Ulrich JD. TREM2 maintains microglial metabolic fitness in alzheimer's disease. Cell (2017) 170(4):649–663.e13. doi: 10.1016/j.cell.2017.07.023
72. Kluss JH, Mamais A, Cookson MR. LRRK2 links genetic and sporadic parkinson's disease. Biochem Soc Trans (2019) 47(2)::651–661. doi: 10.1042/BST20180462
73. Islam MS, Moore DJ. Mechanisms of LRRK2-dependent neurodegeneration: role of enzymatic activity and protein aggregation. Biochem Soc Trans (2017) 45(1):163–72. doi: 10.1042/BST20160264
74. Berwick DC, Heaton GR, Azeggagh S, Harvey K. LRRK2 biology from structure to dysfunction: research progresses, but the themes remain the same. Mol Neurodegener (2019) 14(1):49. doi: 10.1186/s13024-019-0344-2
75. Russo I, Kaganovich A, Ding J, Landeck N. Transcriptome analysis of LRRK2 knock-out microglia cells reveals alterations of inflammatory- and oxidative stress-related pathways upon treatment with α-synuclein fibrils. Neurobiol Dis (2019) 129:67–78. doi: 10.1016/j.nbd.2019.05.012
76. Daher JP, Volpicelli-Daley LA, Blackburn JP, Moehle MS. Abrogation of α-synuclein-mediated dopaminergic neurodegeneration in LRRK2-deficient rats. Proc Natl Acad Sci U S A (2014) 111(25):9289–94. doi: 10.1073/pnas.1403215111
77. Gillardon F, Schmid R, Draheim H. Parkinson's disease-linked leucine-rich repeat kinase 2(R1441G) mutation increases proinflammatory cytokine release from activated primary microglial cells and resultant neurotoxicity. Neuroscience (2012) 208::41–8. doi: 10.1016/j.neuroscience.2012.02.001
78. Choi I, Kim B, Byun JW, Baik SH. LRRK2 G2019S mutation attenuates microglial motility by inhibiting focal adhesion kinase. Nat Commun (2015) 6:8255. doi: 10.1038/ncomms9255
79. Andoh M, Ikegaya Y, Koyama R. Synaptic pruning by microglia in epilepsy. J Clin Med (2019) 8(12):2170. doi: 10.3390/jcm8122170
80. Rappold PM, Lynd-Balta E, Joseph SA. P2X7 receptor immunoreactive profile confined to resting and activated microglia in the epileptic brain. Brain Res (2006) 1089(1):171–8. doi: 10.1016/j.brainres.2006.03.040
81. Avignone E, Ulmann L, Levavasseur F, Rassendren F. Status epilepticus induces a particular microglial activation state characterized by enhanced purinergic signaling. J Neurosci (2008) 28(37):9133–44. doi: 10.1523/JNEUROSCI.1820-08.2008
82. Vezzani A, French J, Bartfai T, Baram TZ. The role of inflammation in epilepsy. Nat Rev Neurol (2011) 7(1):31–40. doi: 10.1038/nrneurol.2010.178
83. Gross A, Benninger F, Madar R, Illouz T. Toll-like receptor 3 deficiency decreases epileptogenesis in a pilocarpine model of SE-induced epilepsy in mice. Epilepsia (2017) 58(4):586–96. doi: 10.1111/epi.13688
84. Maroso M, Balosso S, Ravizza T. Toll-like receptor 4 and high-mobility group box-1 are involved in ictogenesis and can be targeted to reduce seizures. Nat Med (2010) 16:413–9. doi: 10.1038/nm.2127
85. Zhang J, He H, Qiao Y, Zhou T. Priming of microglia with IFN-γ impairs adult hippocampal neurogenesis and leads to depression-like behaviors and cognitive defects. Glia (2020) 68(12):2674–92. doi: 10.1002/glia.23878
86. Cox AJ, West NP, Cripps ,AW. Obesity, inflammation, and the gut microbiota. Lancet Diabetes Endocrinol (2015) 3:207–15. doi: 10.1016/S2213-8587(14)70134-2
87. Ni J, Wu GD, Albenberg L, Tomov ,VT. Gut microbiota and IBD: Causation or correlation? Nat Rev Gastroenterol Hepatol (2017) 14:573–84. doi: 10.1038/nrgastro.2017.88
88. Weiss GA, Hennet T. Mechanisms and consequences of intestinal dysbiosis. Cell Mol Life Sci (2017) 74:2959–77. doi: 10.1007/S00018-017-2509-X
89. Martin CR, Osadchiy V, Kalani A, Mayer ,EA. The brain-Gut-Microbiome axis. CMGH (2018) 6:133–48. doi: 10.1016/j.jcmgh.2018.04.003
90. Samuel BS, Shaito A, Motoike T, Rey FE, Backhed F, Manchester JK, et al. Effects of the gut microbiota on host adiposity are modulated by the short-chain fatty-acid binding G protein-coupled receptor, Gpr41. Proc Natl Acad Sci U S A (2008) 105(43):16767–72. doi: 10.1073/pnas.0808567105
91. Soliman ML, Rosenberger ,TA. Acetate supplementation increases brain histone acetylation and inhibits histone deacetylase activity and expression. Mol Cell Biochem (2011) 352(1-2):173–80. doi: 10.1007/s11010-011-0751-3
92. Erny D, Hrabe de Angelis AL, Jaitin D, Wieghofer P, Staszewski O, David E, et al. Host microbiota constantly control maturation and function of microglia in the CNS. Nat Neurosci (2015) 18:965–77. doi: 10.1038/nn.4030
93. Erny D, Dokalis N, Mezö C, Castoldi A, Mossad O, Staszewski O, et al. Microbiota-derived acetate enables the metabolic fitness of the brain innate immune system during health and disease. Cell Metab (2021) 33(11):2260–76.e7. doi: 10.1016/j.cmet.2021.10.010
94. Vailati-Riboni M, Rund L, Caetano-Silva ME, Hutchinson NT, Wang SS, Soto-Díaz K, et al. Dietary fiber as a counterbalance to age-related microglial cell dysfunction. Front Nutr (2022) 9:835824. doi: 10.3389/fnut.2022.835824
95. Shi H, Ge X, Ma X, Zheng M, Cui X, Pan W, et al. A fiber-deprived diet causes cognitive impairment and hippocampal microglia-mediated synaptic loss through the gut microbiota and metabolites. Microbiome (2021) 9(1):223. doi: 10.1186/s40168-021-01172-0
96. Jiang Y, Li K, Li X, Xu L, Yang Z. Sodium butyrate ameliorates the impairment of synaptic plasticity by inhibiting the neuroinflammation in 5XFAD mice. Chemico-Biological Interact (2021) 341:109452. doi: 10.1016/J.CBI.2021.109452
97. Sadler R, Cramer J. v, Heindl S, Kostidis S, Betz D, Zuurbier XR, et al. Short-chain fatty acids improve poststroke recovery via immunological mechanisms. (2020) 40(5):1162–73. doi: 10.1523/JNEUROSCI.1359-19.2019
98. Kempermann G. Environmental enrichment, new neurons and the neurobiology of individuality. Nat Rev Neurosci (2019) 20(4):235–45. doi: 10.1038/s41583-019-0120-x
99. Marrocco F, Carpini MD, Garofalo S, Giampaoli O, de Felice E, Castro MAdi, et al. Short-chain fatty acids promote the effect of environmental signals on the gut microbiome and metabolome in mice. Commun Biol (2022) 5(1):517. doi: 10.1038/s42003-022-03468-9
100. Lupori L, Cornuti S, Mazziotti R, Borghi E, Ottaviano E, Cas MD, et al. The gut microbiota of environmentally enriched mice regulates visual cortical plasticity. Cell Rep (2022) 38(2):110212. doi: 10.1016/j.celrep.2021.110212
101. Zhou F, Wang X, Han B, Tang X, Liu R, Ji Q, et al. Short-chain fatty acids contribute to neuropathic pain via regulating microglia activation and polarization. Mol Pain (2021) 17:1744806921996520. doi: 10.1177/1744806921996520
102. Sampson TR, Debelius JW, Thron T, Janssen S, Shastri GG, Ilhan ZE, et al. Gut microbiota regulate motor deficits and neuroinflammation in a model of parkinson’s disease. Cell (2016) 167:1469–1480.e12. doi: 10.1016/J.CELL.2016.11.018
103. Qiao C-M, Sun M-F, Jia X-B, Li Y, Zhang B-P, Zhao L-P, et al. Sodium butyrate exacerbates parkinson’s disease by aggravating neuroinflammation and colonic inflammation in MPTP-induced mice model. Neurochemical Res (2020) 45:2128–42. doi: 10.1007/s11064-020-03074-3
104. Wenzel TJ, Gates EJ, Ranger AL, Klegeris A. Short-chain fatty acids (SCFAs) alone or in combination regulate select immune functions of microglia-like cells. Mol Cell Neurosci (2020) 105:103493. doi: 10.1016/J.MCN.2020.103493
105. Zindel J, Kubes P. DAMPs, PAMPs, and LAMPs in immunity and sterile inflammation. (2019) 15:493–518. doi: 10.1146/annurev-pathmechdis
106. Netea MG, van Deuren M, Kullberg BJ, Cavaillon JM, van der Meer ,JWM. Does the shape of lipid a determine the interaction of LPS with toll-like receptors? Trends Immunol (2002) 23:135–9. doi: 10.1016/S1471-4906(01)02169-X
107. Mayer AMS, Murphy J, Macadam D, Osterbauer C, Baseer I, Hall ML, et al. Classical and alternative activation of cyanobacterium oscillatoria sp. lipopolysaccharide-treated rat microglia in vitro. Toxicol Sci (2016) 149(2):484–95. doi: 10.1093/toxsci/kfv251
108. Mayer AMS, Clifford JA, Aldulescu M, Frenkel JA, Holland MA, Hall ML, et al. Cyanobacterial microcystis aeruginosa lipopolysaccharide elicits release of superoxide anion, thromboxane b 2 , cytokines, chemokines, and matrix metalloproteinase-9 by rat microglia. Toxicological Sci (2011) 121:63–72. doi: 10.1093/toxsci/kfr045
109. Marin IA, Goertz JE, Ren T, Rich SS, Onengut-Gumuscu S, Farber E, et al. microbiota alteration is associated with the development of stress-induced despair behavior OPEN. Nat Publishing Group (2017) 7:43859. doi: 10.1038/srep43859
110. de Punder K, Pruimboom L. Stress induces endotoxemia and low-grade inflammation by increasing barrier permeability. Front Immunol (2015) 6:223. doi: 10.3389/fimmu.2015.00223
111. Brown GC. The endotoxin hypothesis of neurodegeneration. J Nueroinflammation (2019) 16(1):180. doi: 10.1186/s12974-019-1564-7
112. Zhang R, Miller RG, Gascon R, Champion S, Katz J, Lancero M, et al. Circulating endotoxin and systemic immune activation in sporadic amyotrophic lateral sclerosis (sALS). J Neuroimmunology (2009) 206:121–4. doi: 10.1016/J.JNEUROIM.2008.09.017
113. Zhao Y, Cong L, Lukiw WJ, Chapman MR, Valero J, Kummer ,MP. Lipopolysaccharide (LPS) accumulates in neocortical neurons of alzheimer’s disease (AD) brain and impairs transcription in human neuronal-glial primary Co-cultures. Front Aging Neurosci (2017) 9:407. doi: 10.3389/fnagi.2017.00407
114. Emanuele E, Orsi P, Boso M, Broglia D, Brondino N, Barale F, et al. Low-grade endotoxemia in patients with severe autism. Neurosci Lett (2010) 471:162–5. doi: 10.1016/J.NEULET.2010.01.033
115. Furube E, Kawai S, Inagaki H, Takagi S, Miyata S. Brain region-dependent heterogeneity and dose-dependent difference in transient microglia population increase during lipopolysaccharide-induced inflammation. Sci Rep (2018) 8:2203. doi: 10.1038/s41598-018-20643-3
116. Campos AC, Rocha NP, Nicoli JR, Vieira LQ, Teixeira MM, Teixeira ,AL. Absence of gut microbiota influences lipopolysaccharide-induced behavioral changes in mice. Behav Brain Res (2016) 312:186–94. doi: 10.1016/J.BBR.2016.06.027
117. Rothhammer V, Mascanfroni ID, Bunse L, Takenaka MC, Kenison JE, Mayo L, et al. Type I interferons and microbial metabolites of tryptophan modulate astrocyte activity and central nervous system inflammation via the aryl hydrocarbon receptor. Nat Med (2016) 22(6):586–97. doi: 10.1038/nm.4106
118. Rothhammer V, Borucki DM, Tjon ,EC, Takenaka MC, Chao C-C, Ardura-Fabregat A, et al. Microglial control of astrocytes in response to microbial metabolites. (2018) 557(7707):724–8. doi: 10.1038/s41586-018-0119-x
119. Jung HJ, Nam KN, Son MS, Kang H, Hong JW, Kim JW, et al. Indirubin-3′-oxime inhibits inflammatory activation of rat brain microglia. Neurosci Lett (2011) 487:139–43. doi: 10.1016/J.NEULET.2010.10.009
120. Tanaka M, Fujikawa M, Oguro A, Itoh K, Vogel CFA, Ishihara Y. Involvement of the microglial aryl hydrocarbon receptor in neuroinflammation and vasogenic edema after ischemic stroke. Cells (2021) 10(4):718. doi: 10.3390/cells10040718
121. Janssens Y, Debunne N, De Spiegeleer A, Wynendaele E, Planas M, Feliu L, et al. PapRIV, a BV-2 microglial cell activating quorum sensing peptide. Sci Rep (2021) 11(1):10723. doi: 10.1038/s41598-021-90030-y
122. Vaughn AC, Cooper EM, DiLorenzo PM, O'Loughlin LJ, Konkel ME, Peters JH, et al. Energy-dense diet triggers changes in gut microbiota, reorganization of gut-brain vagal communication and increases body fat accumulation. Acta Neurobiol Exp (Wars) (2017) 77(1):18–30. doi: 10.21307/ane-2017-033
123. Sen T, Cawthon CR, Ihde BT, Hajnal A, DiLorenzo PM, de la Serre ,CB, et al. Diet-driven microbiota dysbiosis is associated with vagal remodeling and obesity. Physiol Behav (2017) 173:305–17. doi: 10.1016/j.physbeh.2017.02.027
124. Kim JS, Kirkland RA, Lee SH, Cawthon CR, Rzepka KW, Minaya DM, et al. Gut microbiota composition modulates inflammation and structure of the vagal afferent pathway. Physiol Behav (2020) 225:113082. doi: 10.1016/j.physbeh.2020.113082
125. Frasch MG, Szynkaruk M, Prout AP, Nygard K, Cao M, Veldhuizen R, et al. Decreased neuroinflammation correlates to higher vagus nerve activity fluctuations in near-term ovine fetuses: a case for the afferent cholinergic anti-inflammatory pathway? J Neuroinflammation (2016) 13:103. doi: 10.1186/s12974-016-0567-x
126. Kaczmarczyk R, Tejera D, Simon BJ, Heneka. MT. Microglia modulation through external vagus nerve stimulation in a murine model of alzheimer’s disease. J Neurochem (2017) 146(1):76–85. doi: 10.1111/jnc.14284
127. Meneses G, Bautista M, Florentino A, Díaz G, Acero G, Besedovsky H, et al. Electric stimulation of the vagus nerve reduced mouse neuroinflammation induced by lipopolysaccharide. J Inflamm (Lond.) (2016) 13:33. doi: 10.1186/s12950-016-0140-5
128. Ni SJ, Yao ZY, Wei X, Heng X, Qu SY, Zhao X, et al. Vagus nerve stimulated by microbiota-derived hydrogen sulfide mediates the regulation of berberine on microglia in transient middle cerebral artery occlusion rats. Phytother Res (2022) 36(7):2964–81. doi: 10.1002/ptr.7490
129. Liu Y, Sanderson D, Mian MF, McVey Neufeld KA, Forsythe P. Loss of vagal integrity disrupts immune components of the microbiota-gut-brain axis and inhibits the effect of lactobacillus rhamnosus on behavior and the corticosterone stress response. Neuropharmacology (2021) 195:108682. doi: 10.1016/j.neuropharm.2021.108682
130. Cordella F, Sanchini C, Rosito M, Ferrucci L, Pediconi N, Cortese B, et al. Antibiotics treatment modulates microglia-synapses interaction. Cells (2021) 10:2648. doi: 10.3390/cells10102648
131. Thion MS, Low D, Silvin A, Chen J, Grisel P, Schulte-Schrepping J, et al. Microbiome influences prenatal and adult microglia in a sex-specific manner. Cell (2018) 172:500–516.e16. doi: 10.1016/j.cell.2017.11.042
132. Çalışkan G, French T, Enrile Lacalle S, del Angel M, Steffen J, Heimesaat MM, et al. Antibiotic-induced gut dysbiosis leads to activation of microglia and impairment of cholinergic gamma oscillations in the hippocampus. Brain Behavior Immun (2022) 99:203–17. doi: 10.1016/J.BBI.2021.10.007
133. Barichella M, Severgnini M, Cilia R, Cassani E, Bolliri C, Caronni S, et al. Unraveling gut microbiota in parkinson's disease and atypical parkinsonism. Mov Disord (2019) 34(3):396–405. doi: 10.1002/mds.27581
134. Janssens Y, Nielandt J, Bronselaer A, Debunne N, Verbeke F, Wynendaele E, et al. Disbiome database: linking the microbiome to disease. BMC Microbiol (2018) 18(1):50. doi: 10.1186/s12866-018-1197-5
135. Strati F, Cavalieri D, Albanese D, De Felice C, Donati C, Hayek J, et al. New evidences on the altered gut microbiota in autism spectrum disorders. Microbiome (2017) 5(1):24. doi: 10.1186/s40168-017-0242-1
136. Vogt NM, Kerby RL, Dill-McFarland KA, Harding SJ, Merluzzi AP, Johnson SC, et al. Gut microbiome alterations in alzheimer's disease. Sci Rep (2017) 7(1):13537. doi: 10.1038/s41598-017-13601-y
137. Xu R, Wu B, Liang J, He F, Gu W, Li K, et al. Altered gut microbiota and mucosal immunity in patients with schizophrenia. Brain Behav Immun (2020) 85:120–7. doi: 10.1016/j.bbi.2019.06.039
138. Chen JJ, He S, Fang L, Wang B, Bai SJ, Xie J, et al. Age-specific differential changes on gut microbiota composition in patients with major depressive disorder. Aging (2020) 12(3):2764–76. doi: 10.18632/aging.102775
139. Panza F, Lozupone M, Solfrizzi ,V, Watling M, Imbimbo BP. Time to test antibacterial therapy in alzheimer’s disease. Brain (2019) 142(10):2905–29. doi: 10.1093/brain/awz244
140. Valles-Colomer M, Falony G, Darzi Y, Tigchelaar EF, Wang J, Tito RY, et al. The neuroactive potential of the human gut microbiota in quality of life and depression. Nat Microbiol (2019) 4(4):623–32. doi: 10.1038/s41564-018-0337-x
141. Zhu F, Ju Y, Wang W, Wang ,Q, Guo R, Ma Q, et al. Metagenome-wide association of gut microbiome features for schizophrenia. Nat Commun (2020) 11(1):1612. doi: 10.1038/s41467-020-15457-9
142. Sampson TR, Debelius JW, Thron T, Janssen S, Shastri GG, Ilhan ZE, et al. Gut microbiota regulate motor deficits and neuroinflammation in a model of parkinson's disease. Cell (2016) 167(6):1469–1480 e12. doi: 10.1016/j.cell.2016.11.018
143. Shen H, Guan Q, Zhang X, Yuan C, Tan Z, Zhai L, et al. New mechanism of neuroinflammation in alzheimer's disease: The activation of NLRP3 inflammasome mediated by gut microbiota. Prog Neuropsychopharmacol Biol Psychiatry (2020) 100:109884. doi: 10.1016/j.pnpbp.2020.109884
144. Tengeler AC, Dam SA, Wiesmann M, Naaijen J, van Bodegom M, Belzer C, et al. Gut microbiota from persons with attention-deficit/hyperactivity disorder affects the brain in mice. Microbiome (2020) 8(1):44. doi: 10.1186/s40168-020-00816-x
145. Li F, Wang Y, Song X, Wang Z, Jia J, Qing S, et al. The intestinal microbial metabolite nicotinamide n-oxide prevents herpes simplex encephalitis via activating mitophagy in microglia. Gut Microbes (2022) 14(1):2096989. doi: 10.1080/19490976.2022.2096989
146. Minter MR, Zhang C, Leone V, Ringus DL, Zhang X, Oyler-Castrillo P, et al. Antibiotic-induced perturbations in gut microbial diversity influences neuro-inflammation and amyloidosis in a murine model of alzheimer’s disease OPEN. Nat Publishing Group (2016) 6:30028. doi: 10.1038/srep30028
147. Chen J, Vitetta L. The role of butyrate in attenuating pathobiont-induced hyperinflammation. Immune Network (2020) 20(2):e15. doi: 10.4110/in.2020.20.e15
148. Segain J-P. Butyrate inhibits inflammatory responses through NFB inhibition: implications for crohn’s disease. Gut (2000) 47:397–403. doi: 10.1136/gut.47.3.397
149. Cox LM, Calcagno N, Gauthier C, Madore C, Butovsky O, Weiner ,HL. The microbiota restrains neurodegenerative microglia in a model of amyotrophic lateral sclerosis. Microbiome (2021) 10(1):47. doi: 10.1186/s40168-022-01232-z
150. Ou Z, Deng L, Lu Z, Wu F, Liu W, Huang D, et al. Protective effects of akkermansia muciniphila on cognitive deficits and amyloid pathology in a mouse model of alzheimer’s disease. Nutr Diabetes (2020) 10(1):12. doi: 10.1038/s41387-020-0115-8
151. Dodiya HB, Frith M, Sidebottom A, Cao Y, Koval J, Chang E, et al. Synergistic depletion of gut microbial consortia, but not individual antibiotics, reduces amyloidosis in APPPS1-21 alzheimer’s transgenic mice. Sci Rep (2020) 10(1):8183. doi: 10.1038/s41598-020-64797-5
152. Dodiya HB, Kuntz T, Shaik SM, Baufeld C, Leibowitz J, Zhang X, et al. Sex-specific effects of microbiome perturbations on cerebral ab amyloidosis and microglia phenotypes. J Exp Med (2019) 216:1542–60. doi: 10.1084/jem.20182386
153. Dodiya HB, Lutz HL, Weigle IQ, Patel P, Michalkiewicz J, Roman-Santiago CJ, et al. Gut microbiota-driven brain aβ amyloidosis in mice requires microglia. J Exp Med (2021) 219(1):e20200895. doi: 10.1084/jem.20200895
154. Mezö C, Dokalis N, Mossad O, Staszewski O, Neuber J, Yilmaz B, et al. Different effects of constitutive and induced microbiota modulation on microglia in a mouse model of alzheimer's disease. Acta Neuropathol Commun (2020) 8(1):119. doi: 10.1186/s40478-020-00988-5
155. Miyake S, Kim S, Suda W, Oshima K, Nakamura M, Matsuoka T. Dysbiosis in the gut microbiota of patients with multiple sclerosis, with a striking depletion of species belonging to clostridia XIVa and IV clusters. PloS One (2015) 10(9):e0137429. doi: 10.1371/journal.pone.0137429
156. Berer K, Gerdes LA, Cekanaviciute E, Jia X, Xiao L, Xia Z, et al. Gut microbiota from multiple sclerosis patients enables spontaneous autoimmune encephalomyelitis in mice. Proc Natl Acad Sci U.S.A. (2017) 114(40):10719–24. doi: 10.7272/Q6N58JH2
157. Jangi S, Gandhi R, Cox LM, Li N, von Glehn F, Yan R, et al. Alterations of the human gut microbiome in multiple sclerosis. Nat Commun (2016) 7:12015. doi: 10.1038/ncomms12015
158. Mestre L, Carrillo-Salinas FJ, Mecha M, Feliú A, Espejo C, Álvarez-Cermeño JC, et al. Manipulation of gut microbiota influences immune responses, axon preservation, and motor disability in a model of progressive multiple sclerosis. Front Immunol (2019) 10:1374. doi: 10.3389/fimmu.2019.01374
159. Seifert HA, Benedek G, Nguyen H, Gerstner G, Zhang Y, Kent G, et al. Antibiotics protect against EAE by increasing regulatory and anti-inflammatory cells. Metab Brain Dis (2018) 33(5):1599–607. doi: 10.1007/s11011-018-0266-7
160. D’Alessandro G, Antonangeli F, Marrocco F, Porzia A, Lauro C, Santoni A, et al. Gut microbiota alterations affect glioma growth and innate immune cells involved in tumor immunosurveillance in mice. Eur J Immunol (2020) 50(5):705–11. doi: 10.1002/eji.201948354
Keywords: microglia, gut microbiota, gut-derived molecules, antibiotics, gut brain axis
Citation: D’Alessandro G, Marrocco F and Limatola C (2022) Microglial cells: Sensors for neuronal activity and microbiota-derived molecules. Front. Immunol. 13:1011129. doi: 10.3389/fimmu.2022.1011129
Received: 03 August 2022; Accepted: 24 October 2022;
Published: 08 November 2022.
Edited by:
Carla Marie Cuda, Northwestern University, United StatesCopyright © 2022 D’Alessandro, Marrocco and Limatola. This is an open-access article distributed under the terms of the Creative Commons Attribution License (CC BY). The use, distribution or reproduction in other forums is permitted, provided the original author(s) and the copyright owner(s) are credited and that the original publication in this journal is cited, in accordance with accepted academic practice. No use, distribution or reproduction is permitted which does not comply with these terms.
*Correspondence: Cristina Limatola, cristina.limatola@uniroma1.it