- 1Division of Infection and Immunity, The Roslin Institute and R(D)SVS, University of Edinburgh, Roslin, United Kingdom
- 2Department of Pathobiology and Population Sciences, Royal Veterinary College, Hatfield, United Kingdom
- 3Cobb-Vantress Inc., Siloam Springs, AR, United States
Eimeria maxima is a common cause of coccidiosis in chickens, a disease that has a huge economic impact on poultry production. Knowledge of immunity to E. maxima and the specific mechanisms that contribute to differing levels of resistance observed between chicken breeds and between congenic lines derived from a single breed of chickens is required. This study aimed to define differences in the kinetics of the immune response of two inbred lines of White Leghorn chickens that exhibit differential resistance (line C.B12) or susceptibility (line 15I) to infection by E. maxima. Line C.B12 and 15I chickens were infected with E. maxima and transcriptome analysis of jejunal tissue was performed at 2, 4, 6 and 8 days post-infection (dpi). RNA-Seq analysis revealed differences in the rapidity and magnitude of cytokine transcription responses post-infection between the two lines. In particular, IFN-γ and IL-10 transcript expression increased in the jejunum earlier in line C.B12 (at 4 dpi) compared to line 15I (at 6 dpi). Line C.B12 chickens exhibited increases of IFNG and IL10 mRNA in the jejunum at 4 dpi, whereas in line 15I transcription was delayed but increased to a greater extent. RT-qPCR and ELISAs confirmed the results of the transcriptomic study. Higher serum IL-10 correlated strongly with higher E. maxima replication in line 15I compared to line C.B12 chickens. Overall, the findings suggest early induction of the IFN-γ and IL-10 responses, as well as immune-related genes including IL21 at 4 dpi identified by RNA-Seq, may be key to resistance to E. maxima.
Introduction
Coccidiosis, which in poultry is caused by apicomplexan parasites of the genus Eimeria, causes huge economic losses to the global poultry industry through decreased feed efficiency, reduced weight gain, increased mortality, and the cost of prophylaxis and therapy. It is the most economically important parasitic condition of poultry (1, 2). One of seven Eimeria species that can infect chickens, Eimeria maxima, is commonly diagnosed in commercial chicken flocks (3, 4) and specifically invades and parasitizes enterocytes of the jejunum where it can cause pathological lesions, resulting in villus destruction and malabsorptive disease symptoms (5). Currently, control of Eimeria is primarily achieved through in-feed prophylaxis with anticoccidial drugs or by vaccination with live or live-attenuated parasites. However, resistance to anticoccidial drugs is common (6) and vaccination is complex, requiring the preparation and administration of admixtures of between three and eight different lines of parasite to confer adequate protection against field challenge (7). A potential alternative method of control could be to selectively breed chickens that have enhanced resistance to Eimeria; however, this requires knowledge of natural host immunoprotective responses to Eimeria and the identification of biomarkers of resistance.
Understanding the immunological basis of resistance to Eimeria is an important step towards identifying biomarkers of resistance for the selection of relatively resistant individuals within commercial breeding stocks. Inbred lines 15I (MHC type B15) and C.B12 (MHC type B12) are White Leghorn chickens which display differential resistance and susceptibility to E. maxima based on oocyst output (parasite replication). Following primary infection line C.B12 chickens shed fewer oocysts compared to line 15I, but both lines display complete immune protection against homologous secondary infection after which no oocysts are produced (8, 9). In line with this, two-fold higher levels of E. maxima DNA have been detected in the intestinal tissue of line 15I compared to line C.B12 chickens at 5 days post-infection (dpi) (10). Another study reported that line FP (MHC type B15/B21) chickens produce more oocysts than line SC (MHC type B2) chickens after infection with E. maxima (11). All these chicken lines were bred for specific MHC types, however the immunological basis underlying resistance and susceptibility to E. maxima is not well characterized.
Following E. maxima infection, cell-mediated immunity and variation in T-cell responses appear to be central to the induction of protective immunity (8, 12). Although parasite-specific antibodies can protect against E. maxima infection, (13–15), bursectomized (B-cell deficient) chickens were no more susceptible to E. maxima challenge than non-bursectomized control birds (16), suggesting that antibodies are not necessary for elimination of the parasite. An array of cell-mediated responses are a prominent feature of coccidiosis and attempts have been made to correlate these responses with immunity. Primary E. maxima infection leads to increased percentages of CD8 (from 7 to 23 dpi) and γδ T cells (from 14 to 28 dpi) in peripheral blood leukocytes (PBL) in relatively resistant (line C) chickens compared to relatively susceptible (line 15I and 61) chickens, whereas there was no significant difference in CD4 and αβ2 T cells between these lines of chickens (8). Increased numbers of CD4 lamina propria lymphocytes (LPL), but not intraepithelial lymphocytes (IEL), were observed in relatively susceptible Light Sussex chickens at 3 dpi (17), while CD8 LPL and IEL were increased at 4 dpi (18). During E. maxima infection, significantly increased γδ and αβ1 T cells were reported in the epithelium at later time points (11 dpi), while αβ2 T cells in the lamina propria increased at 4 and 11 dpi (18, 19), although there was induction-time variation dependent on the genetic background of the chickens and the nature of the challenge dose.
Interferon (IFN)-γ, a key signature cytokine of Th1-controlled immune responses, is a major cytokine that mediates immune responses against many intracellular pathogens including viruses (20, 21), Salmonella spp. (22) and Eimeria spp. (23, 24). Early studies showed that increased serum IFN-γ protein and gut IFNG mRNA levels are strongly associated with E. acervulina (24, 25), E. maxima (15) and E. tenella (26) infection. During E. maxima infection, significantly increased IFN-γ protein was observed in both the gut and serum of relatively susceptible (line SC) chickens, and serum IFN-γ levels are positively correlated with fecal oocyst shedding (15). Additionally, E. maxima infection leads to induction of IFNG mRNA levels in the IEL population of relatively susceptible (line SC) chickens during primary infection, but not secondary infection (19), suggesting IFN-γ is involved in the response to primary Eimeria spp. infection.
Interleukin (IL)-10 is an anti-inflammatory and regulatory cytokine and is important in balancing inflammatory responses to pathogens. During the characterization of biological roles of chicken IL-10, its potential role as a biomarker for Eimeria spp. infection was suggested. Increased IL10 mRNA levels were observed in the spleen and the small intestine of relatively susceptible (line 15I) chickens during E. maxima infection compared to non-infected chickens, but not in relatively resistant (line C.B12) chickens (27). Moreover, uninfected relatively susceptible chickens had significantly higher IL10 mRNA levels in the spleen compared to relatively resistant chickens (27), suggesting that levels of constitutive IL-10 expression may be dependent on host genetics. Further studies showed increased IL10 mRNA levels in the liver and cecum (28) and IL-10 protein in the serum (29) during E. tenella infection. Furthermore, antibody-mediated depletion of luminal IL-10 reduced oocyst shedding in broilers given an attenuated Eimeria spp. vaccine (30).
The present study aimed to characterize in detail the kinetics of the immune responses of relatively resistant (line C.B12) and susceptible (line 15I) inbred chickens to E. maxima infection. To identify phenotypes that associate with resistance to E. maxima, we investigated differences in gene expression and the systemic and local kinetics of the IFN-γ, and IL-10 response between the two lines. Transcriptomic analysis revealed that interferon-mediated immune responses were induced in line C.B12 chickens at 4 dpi compared to the relatively susceptible line 15I chickens at 6 dpi. Both IFNG and IL10 were expressed in similar patterns during the course of infection in each line. Line C.B12 chickens produced higher levels of IFN-γ and IL-10 proteins in the jejunum and serum until 5 dpi compared to line 15I chickens, whereas by 6-8 dpi line 15I chickens produced higher levels of both. We also found that IFN-γ and IL-10 protein expression and mRNA transcription was highly correlated with parasite burden, with the strongest correlation between parasitemia and serum IL-10 in line 15I chickens. Furthermore, IL21 was identified as a novel gene linked to resistance in line C.B12, with an expression pattern following that of IL10 and IFNG during the course of E. maxima infection.
Materials and Methods
Ethics Statement
Animal work was carried out in strict accordance with the Animals (Scientific Procedures) Act 1986, an Act of Parliament of the United Kingdom, following approval by the Royal Veterinary College Animal Welfare Ethical Review Body (AWERB) and the United Kingdom Government Home Office.
Animals and Parasites
Chickens of two inbred White Leghorn lines were used in this study. Inbred line 15I, relatively susceptible to E. maxima infection, originate from the Regional Poultry Research Laboratory (East Lansing, MI). Reaseheath C (line C, C.B12) chickens, relatively resistant to E. maxima infection, originate from the University of Cambridge (Cambridge, UK). Both flocks were maintained at the National Avian Research Facility (NARF; The Roslin Institute, UK).
The Weybridge (W) strain of E. maxima was used (31). Parasites were passaged at frequent intervals through dosing and fecal recovery as described previously (32), and used less than one month after sporulation.
Experimental Design, Sampling, and Data Collection
Line C.B12 and 15I chickens were supplied at day-of-hatch without prior vaccination to the Royal Veterinary College, where chickens were reared in coccidia-free, environmentally enriched conditions with feed and water provided ad libitum. Chickens were housed following Defra stocking density guidelines and raised under industry-standard conditions. Prior to inoculation, chickens (n = 60 and n = 62 for lines C.B12 and 15I, respectively) were randomly allocated to four different pens corresponding to the two lines and two different experimental treatments: control and infected. The absence of prior coccidian infection was confirmed by fecal flotation. Three-week-old chickens were orally infected with 100 sporulated E. maxima oocysts (test) or sterile water (control).
In order to analyze differential kinetic immune responses elicited by E. maxima infection, blood and small intestine (jejunum) were collected from 3 chickens in the control groups and a minimum of 5 chickens in the infected groups at 2, 4, 5, 6, 7, 8 and 13 dpi. Body weight was recorded individually two days prior to infection and prior to culling at each sampling point and the percentage weight gain calculated. Chickens were culled by cervical dislocation following the Schedule 1 method, and death confirmed by permanent cessation of circulation. Blood was collected from the jugular vein immediately after culling. For serum, blood samples were allowed to clot at room temperature, followed by centrifugation at 1,500 x g for 3 min and the separated serum stored at -20°C.
Approximately 10 cm of small intestine, spanning 5 cm anterior and posterior to the Meckel’s diverticulum (the mid-point of the intestinal area infected by E. maxima) was excised (32), and parasite-related lesions scored as described by Johnson and Reid (33). Approximately 0.5 cm of jejunum, 1 cm anterior to the Meckel’s diverticulum, was collected into RNAlater® Stabilization solution (Life Technologies, CA, USA) for gene expression analysis and by snap-freezing in liquid nitrogen for analysis of tissue protein levels. For histology, 1 cm of jejunum tissue was snap frozen in optimum cutting temperature (OCT) compound on liquid nitrogen and stored at -80°C until use. For parasite quantification, the remaining excised tissues were stored in RNAlater® Stabilization solution at 4°C overnight then at -20°C after removal of the reagent.
Isolation of Genomic DNA and Quantitative PCR (qPCR) for E. maxima Replication
Total genomic DNA (gDNA) was isolated from the excised small intestine as described previously (34). Briefly, tissue samples were weighed and suspended in an equal volume (w/v) of tissue lysis buffer (Buffer ATL, Qiagen, Crawley, UK), and homogenized employing a TissueRuptor (Qiagen). Subsequently, the equivalent of ≤ 25 mg of the homogenate was used to carry out the gDNA isolation using a DNeasy® Blood and Tissue kit (Qiagen) according to the manufacturer’s instructions. The gDNA was stored at -20°C, until further investigation.
Quantitative PCR (qPCR) was performed as previously described (34) using a CFX96 Touch® Real-Time PCR Detection System (Bio-Rad Laboratories, CA, USA). For the quantification of E. maxima total genome copy numbers, we used the primers EmMIC1_For (forward: 5’-TCG TTG CAT TCG ACA GAT TC-3’) and EmMIC1_Rev (reverse: 5’-TAG CGA CTG CTC AAG GGT TT-3’) (10). The chicken cytoplasmic β-actin (actb) gene was used for data normalization, amplified using the primers actb_FW (forward: 5’-GAG AAA TTG TGC GTG ACA TCA-3’) and actb_RV (reverse: 5’-CCT GAA CCT CTC ATT GCC A-3’) (35). Briefly, each sample was amplified in triplicate in a 20 µL volume containing 1 µL of total gDNA, 300 nM of each primer, 10 µL of SsoFast™ EvaGreen® Supermix (Bio-Rad Laboratories), and 8.8 µL of nuclease-free water (Life Technologies) with qPCR cycling conditions that consisted of 95°C for 2 min as initial denaturation, followed by 40 cycles of denaturation at 95°C for 15 sec and annealing/extension at 60°C for 30 sec. Dissociation curves were generated to analyze individual PCR products after 40 cycles. Each qPCR assay included the relevant gDNA dilution series as standards (34) and no template controls. The genome copy numbers from the chicken (actb) and the E. maxima parasites (EmMIC1) were estimated by comparison with the gDNA dilution series. Triplicate data arising from each test sample were averaged and standardized by comparison with the concentration of chicken genome as a ratio of E. maxima genomes/chicken genomes.
Total RNA Preparation and Quantitative Real-Time PCR (qRT-PCR)
Total RNA was extracted from the jejunum using the RNeasy® Mini Spin Column Kit (Qiagen) following the manufacturer’s instruction. Briefly, approximately 25 mg of tissues were homogenized in 2 mL tubes containing 600 μL of Buffer RLT with 2% β-mercaptoethanol and a stainless steel bead (5 mm, Qiagen) using a TissueLyser II system (Qiagen). The supernatant was collected and applied to a QIAshredder column (Qiagen) to improve the quality of total RNA. The flow-through was mixed with an equal volume of 70% ethanol and applied to an RNeasy® Spin column (Qiagen). Contaminating gDNA was digested by on-column DNase treatment using RNase-free DNase (Qiagen) and total RNA was eluted with 80 μL of nuclease-free water (Qiagen). The absorbance at 230, 260 and 280 nm was measured using a NanoDrop 1000 spectrophotometer (Thermo Scientific). For the transcriptomic study, the quantity and quality of total RNA was assessed using a Qubit® RNA BR assay kit (Life Technologies) by Qubit® 3.0 fluorometer (Life Technologies) and an RNA ScreenTape (Agilent Technologies, USA) by 2200 TapeStation System (Agilent Technologies), respectively.
The mRNA levels of target cytokines were quantified by TaqMan® real-time quantitative PCR (RT-qPCR) as described previously (36) (Table 1). TaqMan assays were performed using the One-Step RT-PCR Master Mix reagent, and amplification and detection were performed using the TaqMan Fast Universal PCR Master mix in the AB 7500 FAST Real-Time PCR System (Applied Biosystems). Standard curves for each target gene were generated as previously described (37). Each RT-qPCR assay contained triplicate no-template controls, test samples and a log10 dilution series of standard RNA. Relative gene expression of the infected birds to control birds was calculated using the Pfaffl method as described by Sutton et al. (36) and the results were presented as log10 fold-change of target gene in each line at each time point.
RNA-Seq Library Construction, Sequencing, and Data Analysis
The total RNA of 64 samples were submitted to Edinburgh Genomics, where libraries from each of the 64 individuals were generated using automated TruSeq stranded mRNA-Seq library, and the individual jejunum transcriptomes were sequenced by 150 cycles generating paired-end reads using Illumina HiSeq 4000 technology to yield at least 290M reads. The 64 samples included 3 control and 5 E. maxima infected samples from lines C.B12 and 15I at 2, 4, 6 and 8 dpi.
Reads were trimmed using Trimmomatic (ver. 0.36) (38) to remove adaptor sequences of the TruSeq Stranded mRNA kit and for quality. After trimming, reads were required to have a minimum length of 75 bases. The RNA-seq reads were mapped to the reference genomes using the STAR aligner software package (ver. 2.5.1b) (39). The reference genome used for mapping was the Gallus gallus (Gallus_gallus-5.0) and Eimeria maxima (EMW001) genomes from Ensembl (https://www.ensembl.org/index.html). The annotation used for counting was derived from the Gallus gallus genome only, such that reads mapping to E. maxima were not counted in downstream analysis. Raw counts for each annotated gene were obtained using the featureCounts software (ver. 1.5.2) (40).
Differential gene expression analysis was performed using the Bioconductor edgeR package (ver. 3.16.5) (41). Statistical assessment of differential expression was carried out with the likelihood-ratio test. Differentially expressed genes were defined as those with FDR <0.05 and logFC > 1.6. Heatmaps were constructed in R using the pheatmap package. Overrepresentation of GO terms was investigated using the PANTHER Overrepresentation Test (released December 5, 2017) using Fisher’s Exact test with FDR correction for multiple testing. Network analysis for both sample-sample and gene-gene networks was performed using BioLayout 3D (42) which performs a Pearson correlation to obtain a matrix calculated for each pair of samples or genes, using a modified Fruchterman-Rheingold algorithm, with correlation cut offs of r = 0.93 (sample-sample) and r =0.87 (gene-gene). Clustering was performed on these networks using the Markov clustering algorithm (MCL) with an inflation value of 2.4 (sample-sample) and 1.4 (gene-gene). The IPA program (Ingenuity® System) was used to identify cellular canonical pathways and physiological functions that are affected by E. maxima infection in the host (p-value < 0.05 and q-value < 0.05).
Preparation of Protein Lysates From Tissue Samples and Capture ELISA Assays
To determine protein levels of cytokines in tissues, protein lysates were prepared from the collected jejunum using the modified protein lysis buffer (20 mM Tris (pH 7.5), 100 mM NaCl, 0.5% NP-40 (IGEPAL® CA-630, Sigma), 0.5 M EDTA, 0.5 mM phenylmethylsulfonyl fluoride (Sigma) and 0.5% protease inhibitor cocktail (Sigma)). Approximately 20 mg of jejunum were mixed with 600 μL of the prepared protein lysis buffer and homogenized using 5 mm stainless steel beads (Qiagen) and a TissueLyser II system (Qiagen), twice at 25 Hz for 2 min with a 5 min incubation on ice between the two homogenizations. The samples were centrifuged at 13,000 x g for 10 min at 4°C and the supernatants transferred to chilled microcentrifuge tubes. The concentrations of the protein lysates were measured using the BCA Protein Assay kit (Thermo Scientific) according to the manufacturer’s instructions.
IL-10 and IFN-γ protein levels in serum and tissues were measured by ELISA. IL-10 was quantified using an in house-developed ELISA system (kindly provided by Dr. Z. Wu) for serum as described previously (29) and was adapted for use with tissue lysates. Briefly, assay plates (Nunc Immuno MaxiSorp, Thermo Scientific) were coated with 3 μg/mL of capture antibody diluted in carbonate/bicarbonate buffer at 4°C overnight. Plates were incubated with 50 μL of 2-fold serially diluted standards, sera or protein lysates for 1 hr, followed by incubation with 1 μg/mL of detection antibody for 1 hr. The plates were incubated with the Pierce High Sensitivity streptavidin-HRP (1:10,000 dilution, Thermo Scientific) for a further hour before adding 50 μL of 1-Step Turbo TMB (Thermo Scientific). After 10 min, the reaction was stopped by adding 50 μL of 2 N sulfuric acid. The absorbance was read at 450 nm (650 nm as a reference). Serum and tissue IFN-γ levels were quantified using the Chicken IFN-γ CytoSet kit (Life Technologies) as per the manufacturer’s instructions.
The standard curve was fitted to a four-parameter logistic regression curve and final concentration measures were determined using the online program provided by elisaanalysis.com (http://www.elisaanalysis.com/). The quantity of IL-10 and IFN-γ protein in the jejunum was converted from the concentration determined by ELISA to the quantity of protein in 1 mg of tissue by correcting for the amount of protein lysate used in the ELISA and the total protein lysate in 1 mg of tissue.
Immunohistochemistry (IHC)
Immunohistochemistry was performed to determine differences in cell populations in the jejunum of line C.B12 and 15I chickens at 4 dpi with E. maxima. Cryostat sections (7 μm thick) were picked onto Superfrost® glass slides (Thermo Scientific) and air-dried. Sections were fixed in acetone with 0.75% H2O2 for 10 min at room temperature and air-dried for a further 5 min. The sections were incubated with monoclonal antibodies (purchased from Southern Biotech, Cambridge, UK, Table 2) specific for various leukocyte subpopulations. The Vectastain Elite ABC (Mouse IgG) Kit (Vector Laboratories, CA, USA) was used to detect monoclonal antibodies and peroxidase activity developed using the AEC staining kit (Sigma) following the manufacturer’s instructions. Subsequently, sections were counterstained with hematoxylin Z (CellPath, Newtown, UK), and bluing performed with Scott’s Tap Water (tap water, 2% magnesium sulfate, 0.35% sodium bicarbonate). Slides were mounted in Aquamount AQ (Vector Laboratories) and images were captured with an Eclipse Ni microscope (Nikon, Tokyo, Japan), followed by quantification of the subpopulation of T lymphocytes using ZEN lite 2012 software (blue edition, Carl Zeiss). To enumerate cell sub-populations in the jejunum, the number of lamina propria lymphocytes (LPL) and IEL were counted per 300 μm length of villi and per 150 x 150 μm2 area of crypts. Cells were counted from 3 different areas per section and 3 villi or crypt regions were selected per area.
Statistical Analysis
All statistical analysis was conducted with Minitab 17 software (Minitab Inc., USA). Data were analyzed for normality using the Anderson-Darling test. Data were not normal and significance was therefore assessed by the Mann-Whitney U test. The Spearman’s rank correlation coefficient was calculated to evaluate relationships between parasitemia and host immune responses, and each cytokine in the serum and jejunum.
Results
Comparison of Body Weight Gain and E. maxima Load Between Relatively Resistant and Susceptible Chickens
To examine the impact of E. maxima infection on the growth of line C.B12 (relatively resistant) and line 15I (relatively susceptible) chickens, the percentage weight gains were calculated for individual birds from 2 days prior to E. maxima infection to the time of culling (Figure 1A). E. maxima infection did not affect body weight gain (BWG) compared to control birds and there was no significant difference between the two lines during the course of the experiment. The low challenge dose did not result in lesions in the gut of either line.
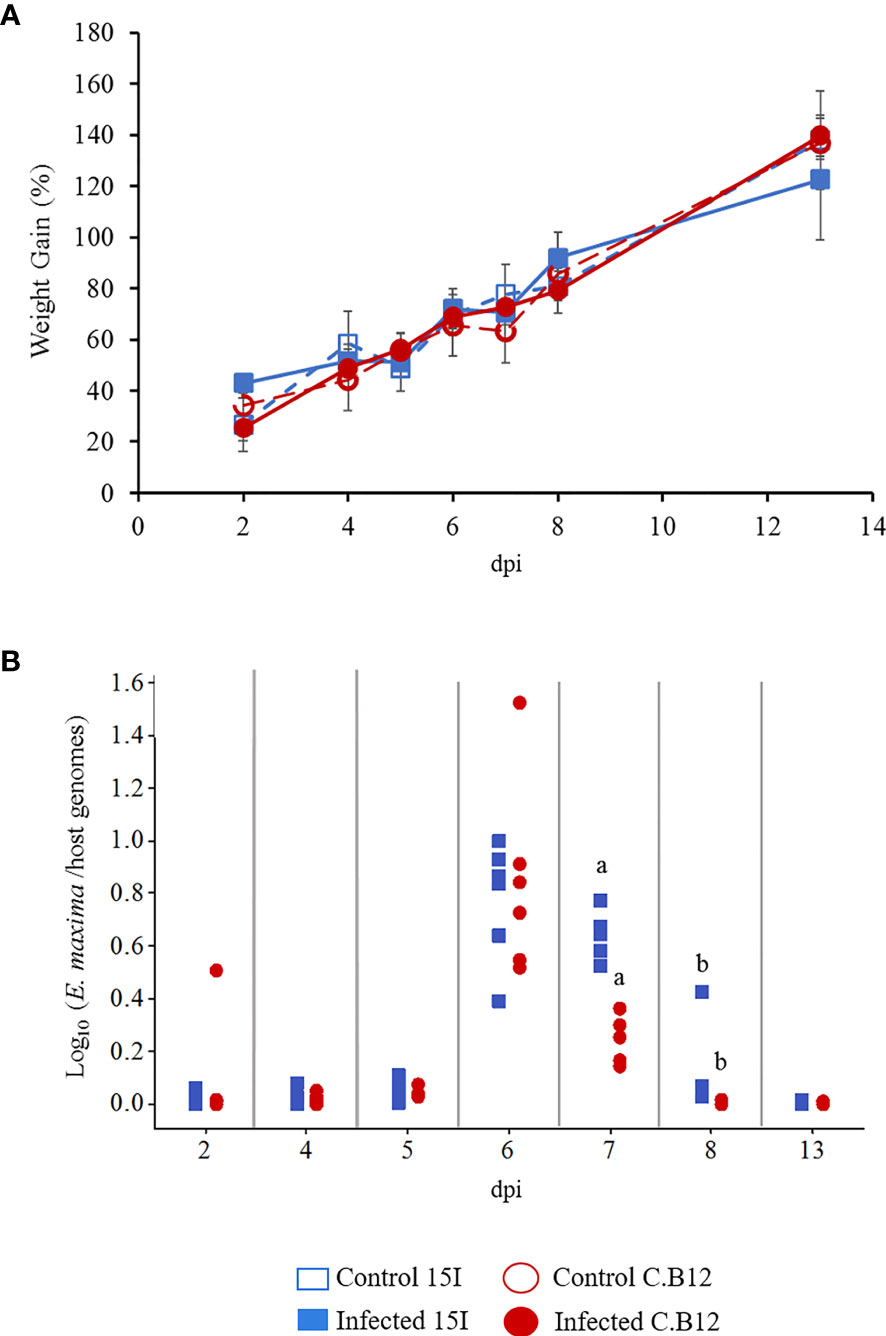
Figure 1 Body weight gains and parasite replication in line C.B12 and 15I chickens following E. maxima infection. Three-week-old birds were orally infected with 100 sporulated E. maxima oocysts (n = 5 per line) or sterile water (n = 3 per line). (A) Percentage of body weight gains were calculated for individual birds from 2 days prior to inoculation to time of culling at time points as indicated. The results were presented as the mean percentage of body weight gain and error bars represent standard deviation. (B) Eimeria maxima replication was quantified by qPCR targeting the MIC1 gene. The results were presented as the ratios of parasite genome vs host genome copy numbers for individual birds. Matching letters indicate significant differences between the two lines at p < 0.05 on the same day (n = 5 per time point).
E. maxima genome copy numbers sharply increased at 6 dpi to similar levels in both lines of birds (Figure 1B). Thereafter the genome copy numbers decreased in both lines but was significantly higher in the jejunum of line 15I compared with line C.B12 chickens at 7 and 8 dpi. By 13 dpi, no significant difference in E. maxima genome copy number was apparent between the two lines. Eimeria genomes remained detectable 1 day later in relatively susceptible line 15I chickens.
Comparison of Global Kinetic Gene Expression Profiles Between Relatively Resistant and Susceptible Chickens During E. maxima Infection
To explore host responses to E. maxima infection and the genetics underlying the relative differences in resistance and susceptibility between line C.B12 and 15I chickens, transcriptome analysis was performed. Differentially expressed genes (DEGs) were identified within the jejunum anterior to the Meckel’s diverticulum, the site of peak E. maxima replication, between control and infected chickens of each line at 2, 4, 6 and 8 dpi under the following conditions: False Discovery Ratio (FDR) < 0.05 and log(Fold Change (FC)) > 1.6 (Tables 3; S1, S2). Line 15I chickens showed very little response at 2 dpi (5 DEGs) and 4 dpi (3 DEGs), but had a large number of DEGs at 6 dpi (1124 DEGs). In contrast, line C.B12 had already established a substantial response by 4 dpi (177 DEGs), but also demonstrated a peak response at 6 dpi (666 DEGs). In line C.B12, 42.2% and 26.8% of the DEGs were immune-related in function at 4 and 6 dpi, respectively. In line 15I, there was no differential expression in immune-related genes at 4 dpi, while 29.2% of DEGs at 6 dpi were immune-related. Immune genes upregulated strongly in both lines at day 6 included IFNG, chemokines and complement components. Analysis using the Markov clustering algorithm indicated that samples from line C.B12 at 6 dpi and line 15I at 8 dpi were the furthest distance from controls, indicating that globally the peak responses may occur at these times (Figure 2A). A network graph of unbiased gene-to-gene clustering was constructed (Figure 2B, Table S3). Out of 12 clusters, cluster 5 revealed a set of 163 genes (Figure 2C), which included IFNG and IL10, that were strongly elevated at 6 dpi in both lines of chickens, but also earlier at 4 dpi in line C.B12 chickens. Further functional analysis revealed that genes of this cluster are mainly involved in interferon signaling, the Th1 pathway, and the Th1 and Th2 activation pathways. Genes in Cluster 5 included the IFN-α/β receptor (IFNAR), IFNG, interferon regulatory factor (IRF), protein tyrosine phosphatase (PTPN2), signal transducer and activator of transcription 1 and 2 (STAT1 and STAT2), transporter 1 ATP binding cassette (TAP1, participates in the interferon signaling pathway), CD80, CD274, delta like canonical Notch ligand 4 (DLL4), IL10, IL12A (participates in the Th1 pathway, and Th1 and Th2 activation pathways), C-C motif chemokine ligand 1 (CCL1), CCL4, complement components 1s (C1S), C1R, and genes involved in the JAK-STAT cascade.
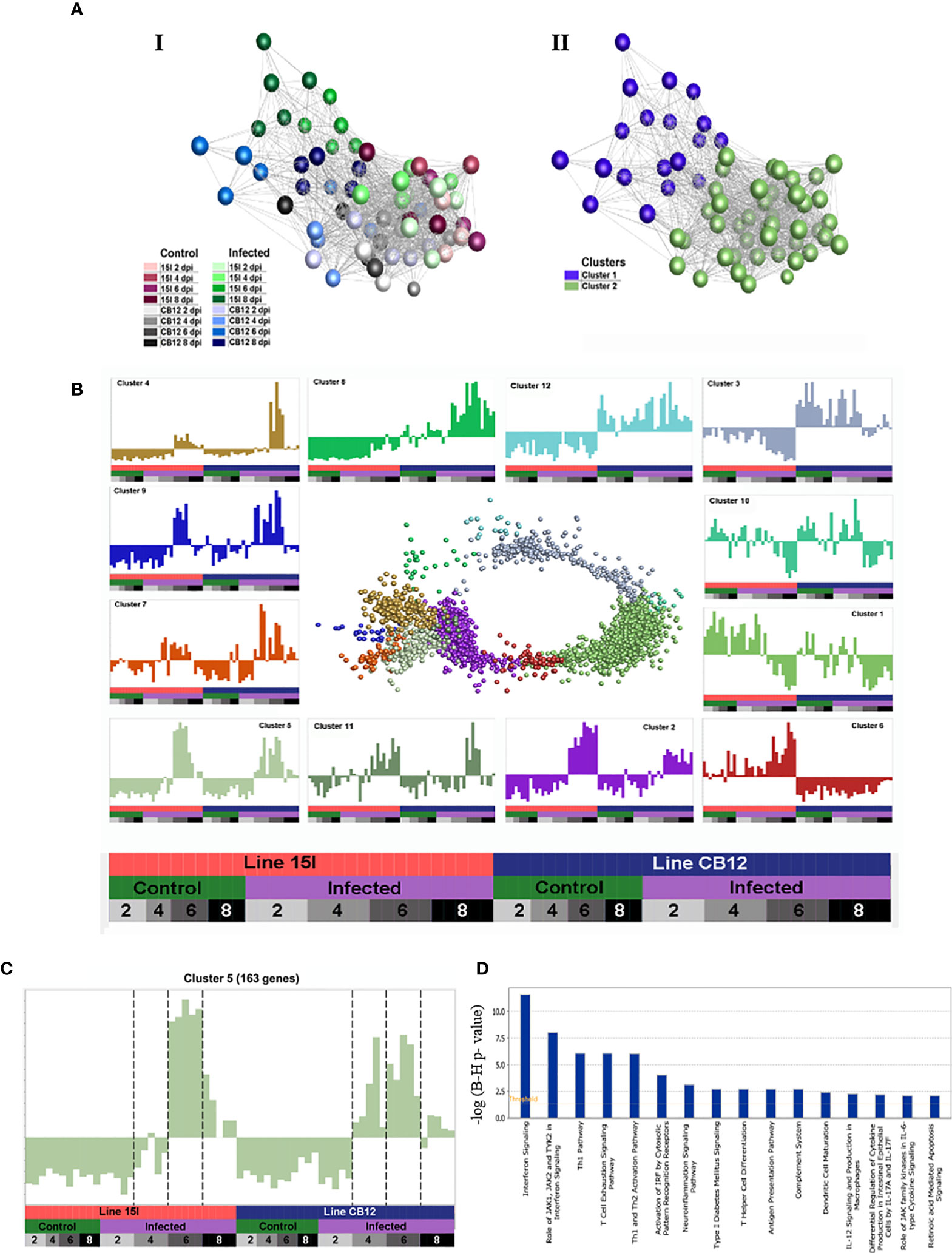
Figure 2 A network graph of unbiased sample-to-sample and gene-to-gene clustering. Sample-sample networks colored by treatment group (A-I) and Markov clustering (A-II). Gene-gene network graph of Markov clustered genes (B), with normalized expression across samples (mean-centered scaling) of each cluster in the surrounding charts. Genes in cluster 5 (C) including IFN-γ and IL-10 have strongly elevated expression at 6 dpi in both lines of chickens, but also earlier at 4 dpi in line C.B12 chickens. Pathways enriched in cluster 5 are shown in (D).
Kinetics of Differential Gene Expression in Relatively Resistant and Susceptible Chickens
At 2 and 4 dpi, E. maxima-infected line 15I chickens had only 5 and 3 significant DEGs respectively, compared to control birds, although none of these were immune-related (Table S1). At 6 dpi, the largest increase in the expression of immune-related genes in line 15I was observed with 25% of upregulated genes with known functions having immune roles (Table S1). The pathways associated with the response of line 15I chickens at 6 dpi were primarily involved in T cell differentiation including differentiation into Th1 and Th2 subsets (Figure 3A). Gene ontology (GO) term enrichment analysis also highlighted the IL-21, IL-2 and IFN-γ pathways (Table S4). The highest upregulated protein coding genes were a complement receptor (homolog of CR1), IFNG and a gene involved in lipid metabolism (ELOVL3). Significant upregulation of immune-related genes was still observed at 8 dpi in line 15I, with 14.8% of 638 DEGs being immune-related. Upregulated genes at 8 dpi are involved in the complement and cell replication pathways, while genes associated with coagulation were downregulated (Figure 3B, Table S4). IFNG and IL10 continued to be significantly upregulated at 8 dpi, and chemokines CCL26 and chCCLi7 were highly upregulated.
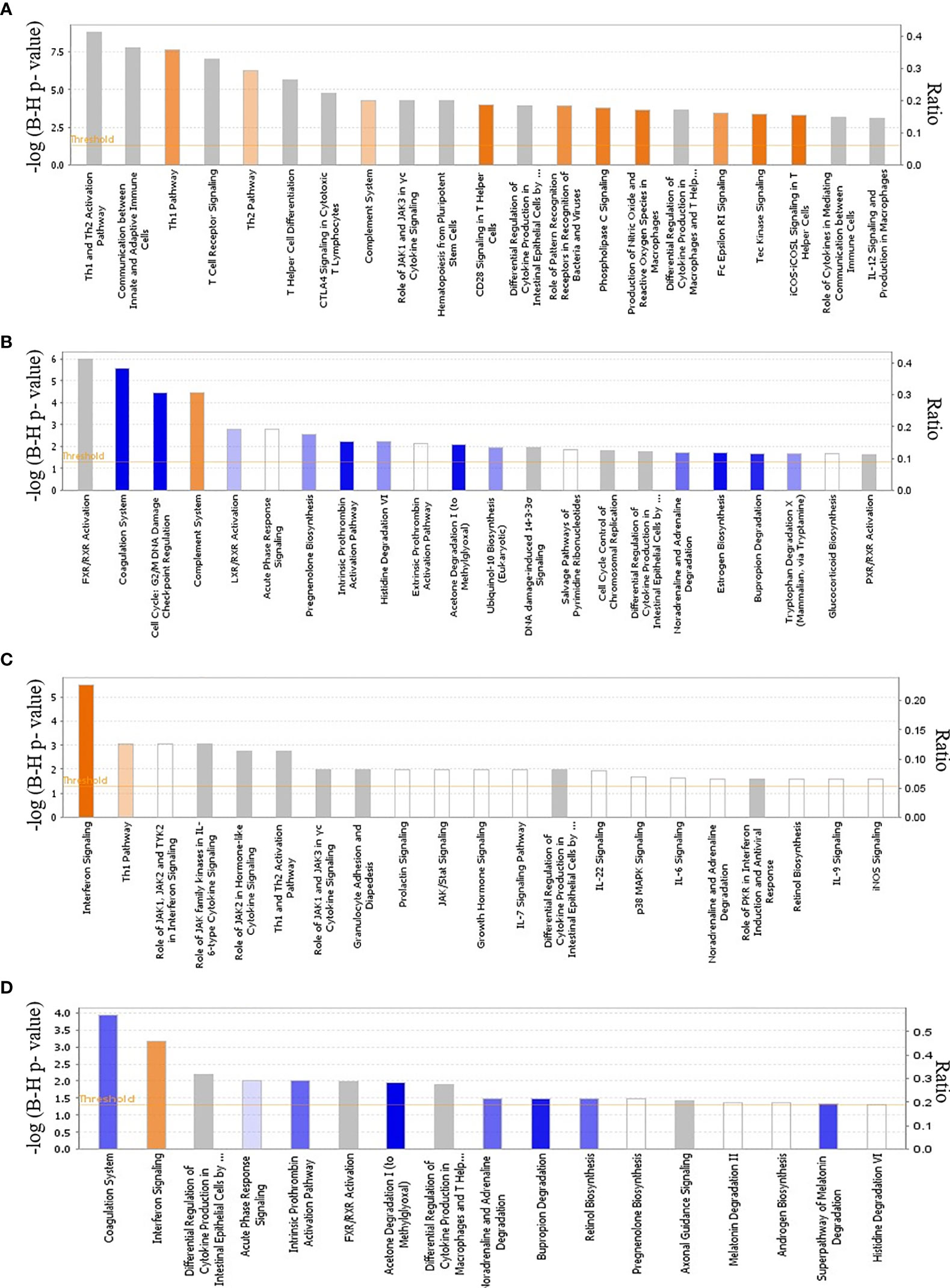
Figure 3 Global transcriptional analysis of cellular canonical pathways and physiological functions. Ingenuity pathway analysis during E. maxima infection in line 15I at 6 (A) and 8 (B) dpi and line C.B12 chickens at 4 (C) and 6 (D) dpi. Color based on Z-score with orange indicating activated pathways and blue indicating de-activated pathways.
In comparison with relatively susceptible (line 15I) chickens, relatively resistant (line C.B12) chickens developed immune responses to E. maxima infection as early as 2 and 4 dpi. A total of 13 DEGs were identified between E. maxima-infected line C.B12 compared to non-infected chickens at 2 dpi (Table 1). Most of these genes upregulated were associated with erythrocytes. At 4 dpi in line C.B12, 42% of 177 DEGs were immune-related genes (Table S2). Further functional analysis revealed that genes involved in the interferon signaling and Th1 pathways were strongly upregulated (Figure 3C) including interferon-induced protein with tetratricopeptide repeats 1 (IFIT1), MX dynamin GTPase 1 (MX1, participates in the interferon signaling pathway), CD274, suppressor of cytokine signaling 3 (SOCS3, participates in the Th1 pathway), IFNG, SOCS1 and signal transducer and activator of transcription 1 (STAT1, participates in both pathways). GO term enrichment analysis indicated genes associated with T-cell activity, the IFN-γ pathway, the JAK-STAT cascade and response to virus were strongly upregulated (Table S5). The highest upregulated protein coding genes were IFNG, a homolog of lysozyme-G (ENSGALG00000044778), CCL4, and GTPase very large interferon inducible pseudogene 1 (GVINP1). Some of the upregulated interferon-stimulated genes such as radical S-adenosyl methionine domain containing 2 (RSAD2), IFIT1, MX1 and 2’-5’-oligoadenylate synthetase like (OASL) were not significantly upregulated at any time point in line 15I chickens (Table S1). At 6 dpi, interferon and T-cell related genes continued to be upregulated in line C.B12, with the highest peak of IFNG and IL10 expression observed (Figure 3D; Table S2). By 8 dpi, the response of line C.B12 chickens had subsided with only 171 DEGs (Table S2). These genes varied, and no significantly enriched GO terms were identified. Ingenuity pathway analysis revealed that only the coagulation pathway – regulated by fibrinogen gamma (FGG), kininogen 1 (KNG1), plasminogen (PLG)—was significantly downregulated in line C.B12 at 8 days post E. maxima infection.
Comparison of the Immune Responses Between Line C.B12 and Line 15I Chickens
To directly compare the response to infection in the two chicken lines, the DEGs with the highest mean difference in logFC during E. maxima infection between the lines were examined, and the top 50 were plotted in a heatmap (Figure 4A). DEGs uniquely upregulated in line 15I included cytokines and genes associated with chemotaxis (TNF receptor superfamily member 13C (TNFRSF13C), C-X-C motif chemokine ligand 13 (CXCL13), chemokine ah221 (CCL9) and Pre-B lymphocyte protein 3 (VPREB3). A group of interferon-stimulated viral response genes (IFIT5, RSAD2, MX1, OASL) and ubiquitin specific peptidase 18 (USP18) were upregulated at 4 and 6 dpi in line C.B12 but not line 15I chickens, further highlighting that this pathway is responding at a relatively higher level in line C.B12 compared to line 15I chickens. An additional group of genes was strongly upregulated in line C.B12 at 6 dpi only. Many of these genes are involved in epidermis development (keratin 75 (KRT75), KRT15, KRT12, ALX homeobox 4 (ALX4), homeobox B13 (HOXB13), suggesting that tissue repair is occurring at this time point in line C.B12, but may be delayed in line 15I.
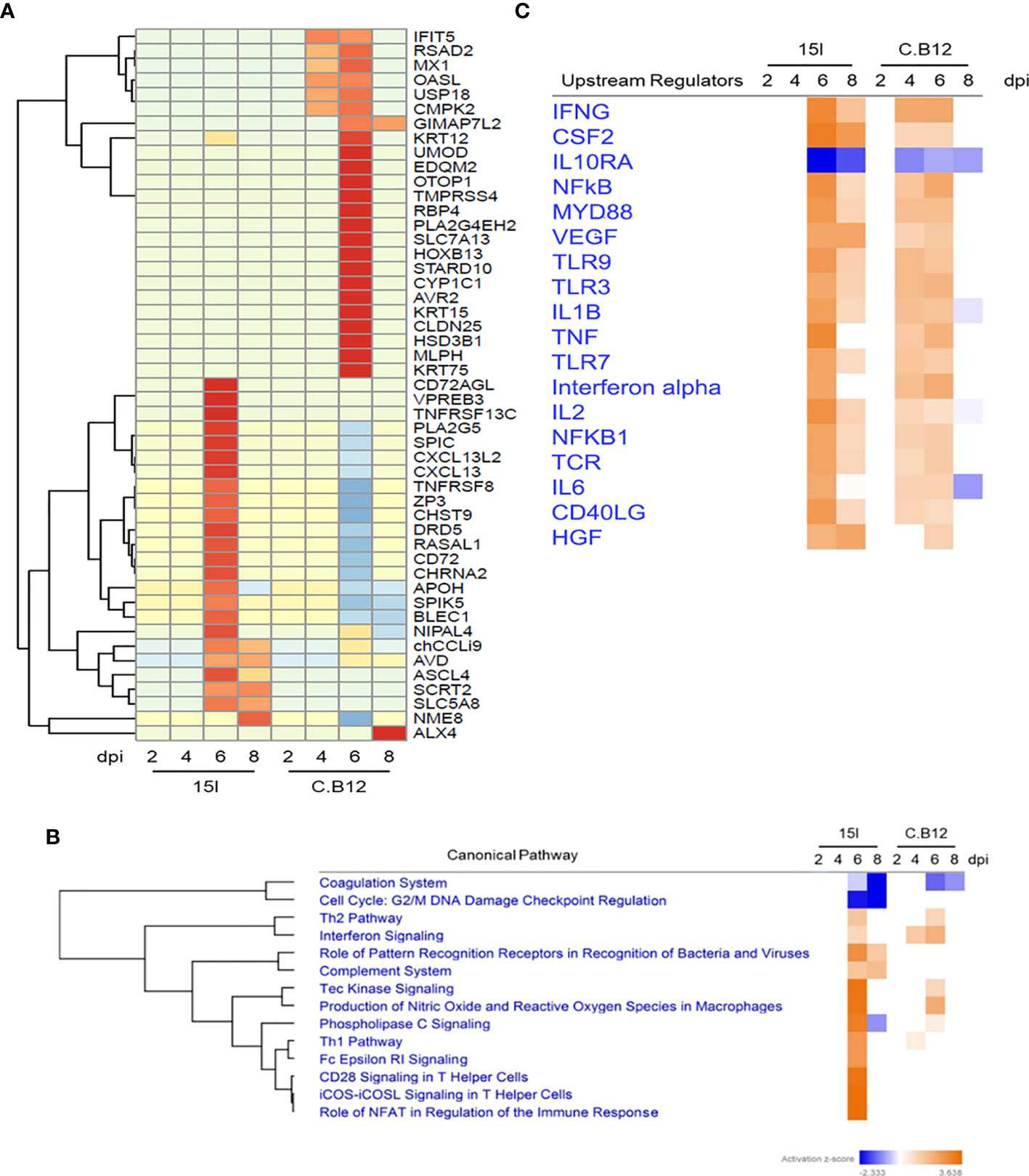
Figure 4 Comparison of line 15I and C.B12 chickens during E. maxima infection. Heatmap (A) showing genes that presented the highest mean fold difference between lines. Functional pathway analysis (B) and predicted upstream regulators in both lines (C) are presented with color based on Z-score; orange indicating activated pathways or regulators and blue indicating de-activated pathways or regulators.
To further investigate the differences between the two chicken lines, we compared pathways enriched in each line using IPA software (Figure 4B). This highlighted commonalities and differences between the responses of the lines. The coagulation pathway was downregulated in both lines at 6 and 8 dpi, while genes associated with cell cycle regulation were uniquely downregulated in line 15I. The Th2 and the Tec kinase signaling pathways were upregulated in both lines at 6 dpi, as was interferon signaling, although the latter pathway was significantly enriched already at 4 dpi in line C.B12 chickens. Pathways that showed a stronger enrichment in line 15I compared to C.B12 chickens included the Th1, T helper cell and complement pathways. Analysis of the predicted upstream regulators revealed that both chicken lines share many of the same upstream regulators including IFNG, CSF2 and vascular endothelial growth factor A (VEGF) although the activation of these generally occurred at 4 and 6 dpi in line C.B12 and at 6 and 8 dpi in line 15I chickens (Figure 4C).
A previous genome-wide association study using an F2 intercross between lines C.B12 and 15I revealed a 35 MB region of chromosome 2 is significantly associated with resistance to coccidiosis (43, 44). We identified genes in this region that were differentially regulated at one or more time points (Table S6). Forty-seven genes in this region were differentially regulated in at least one condition. Out of 47, 10 genes were differentially expressed between two lines, including ATP binding cassette subfamily A member 13 (ABCA13H), Dermatan sulfate epimerase like (DSEL), Serpin family B member 2 (SERPINB2) and Sad1 and UNC84 domain containing 3 (SUN3). The F-box protein 15 (FBXO15), which is involved in the MHC class I processing pathway, was downregulated earlier in the line C.B12 compared to line 15I chickens during E. maxima infection. The interferon alpha inducible protein 6 (IFI6), which plays a role in cell apoptosis, was upregulated in line C.B12 at 6 dpi but not in line 15I chickens, compared to non-infected chickens.
Differential Kinetics of IFN-γ and IL-10 Expression in the Jejunum of Relatively Resistant and Susceptible Chickens Following E. maxima Infection
During the analysis of RNA-Seq results, we discovered that IFNG and IL10 expression increased more rapidly post-infection in line C.B12 (4 dpi) compared to line 15I (6 dpi) (Figure 5A). Expression of IFNG was significantly increased (FDR < 0.05) at 6 and 8 dpi in line 15I, but in line C.B12 at 4, 6 and 8 dpi, while IL10 was significantly upregulated at 6 and 8 dpi in line 15I and at 6 dpi in line C.B12. Although IL10 was not significantly upregulated at 4 dpi in line C.B12, this is likely due to the high variance between birds at this time point, with some samples showing elevated IL10 counts.
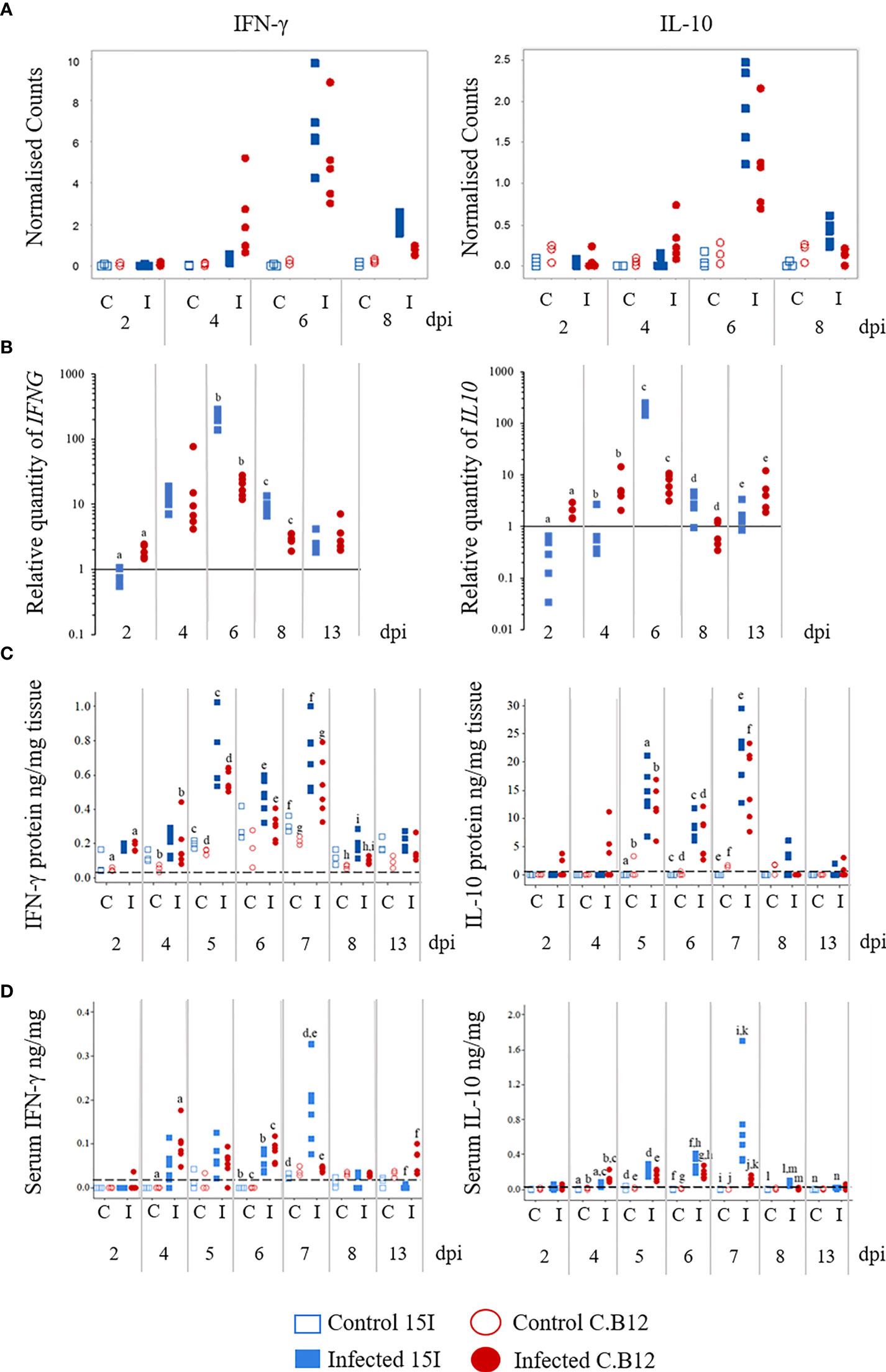
Figure 5 Kinetics of IFNG and IL10 mRNA transcription by RNA-Seq (A) and RT-qPCR (B), protein levels in the jejunum (C) and protein levels in the serum (D) of E. maxima-infected chickens. Three-week old birds were orally inoculated with 100 oocysts of E. maxima (solid markers) or sterile water (control birds; hollow markers) and jejunum and serum samples collected at various days post-infection as indicated. Data are presented as individual birds. For RT-qPCR data, the relative quantity (RQ) of mRNA transcription of individual infected birds was calculated relative to the mean of control birds of the same line at individual time points and normalized using the 28S reference gene. Matching letters denote significant differences between groups on the same day (p < 0.05, n = 3 for control and n = 5 for infected groups). C, control; I, infected; Line C.B12 shown as red circles, line 15I as blue squares.
To verify the transcriptomic results and to obtain insight into the role of IFN-γ and IL-10 in susceptibility to E. maxima infection, IFNG and IL10 mRNA levels in lines C.B12 and 15I were determined in the jejunum at 2, 4, 5, 6, 7, 8 and 13 dpi (Figure 5B). Across control birds of all time points, line C.B12 birds had significantly higher (p<0.01) IFNG mRNA transcription in the jejunum compared to line 15I. In both chicken lines, the greatest increase in IFNG mRNA transcription, relative to control birds of the same line, was at 6 dpi (Figure 5B). At 6 and 8 dpi, line 15I exhibited significantly greater increases in IFNG mRNA levels compared to line C.B12 chickens. Analysis of IFN-γ protein in the jejunum by ELISA revealed biphasic increases in IFN-γ production at 5 and 7 dpi in both chicken lines (Figure 5C). During E. maxima infection, line C.B12 exhibited significantly increased IFN-γ protein in the jejunum at 2, 4, 5, 7 and 8 dpi, whereas line 15I had significantly increased IFN-γ protein at 5 and 7 dpi compared to their non-infected counterparts. Following infection, line 15I birds exhibited higher levels of IFN-γ protein in the jejunum at 6 and 8 dpi compared to line C.B12.
At 4 dpi, line C.B12 transcribed higher levels of IL10 mRNA in the jejunum relative to age-matched control birds of the same line and control or infected line 15I birds (Figure 5B). However, the transcription of IL10 mRNA in the jejunum of line 15I was dramatically increased, relative to controls, at 6 dpi, whereas line C.B12 expressed similar increases in IL10 mRNA levels at 2, 4, 6 and 13 dpi. There was no significant difference in the basal transcription of IL10 between control birds of the two lines across all time points. Similarly to IFN-γ protein levels in the jejunum, there were two peaks of IL-10 protein levels in the jejunum at 5 and 7 dpi (Figure 5C). The levels of IL-10 protein in the jejunum of the control birds was either lower than the limit of detection (as in line 15I) or very little was present (as in line C.B12) across all time points. At 5 dpi, there were significantly increased IL-10 protein levels in the jejunum of both lines of chicken. The increased IL-10 protein induced by E. maxima infection then slightly decreased at 6 dpi, but increased again at 7 dpi. Unlike mRNA levels, there was no significant difference in IL-10 protein levels between the two lines at any of the time points.
We also measured mRNA levels of Th17-associated genes IL17A, IL17F, IL21, IL2 and IL6 (Figures 6 and S1). Although the expression of IL2 mRNA levels at 2 and 6 dpi seemed to be upregulated, the change was not significant due to the high variance between chickens (Figure S1). Among the measured genes, only IL21 showed a significant increase in the jejunum of both lines of chickens during E. maxima infection (at 4 and 6 dpi) compared to their non-infected counterparts (Figure 6A). Additionally, at 4 and 6 dpi, line 15I transcribed significantly higher IL21 mRNA levels compared to line C.B12 chickens whereas at 13 dpi, IL21 mRNA was higher in line C.B12 birds. However, the protein levels of IL-21 in jejunum and serum were either very low or below the detection limit of the ELISA.
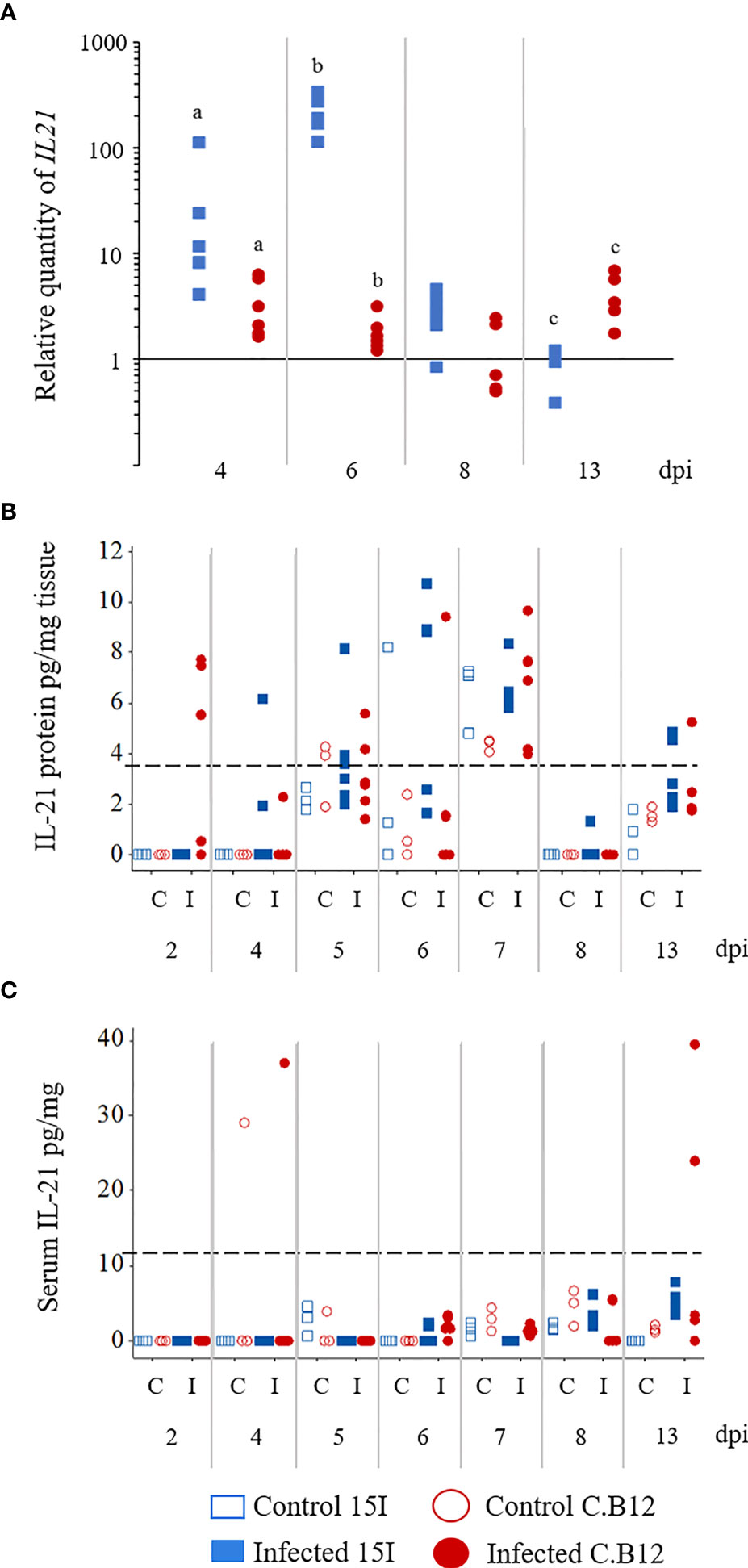
Figure 6 Kinetics of IL21 mRNA transcription (A) and protein expression (B) in the jejunum and IL-21 protein levels in the serum (C) of E. maxima-infected chickens. Three-week old birds were orally inoculated with 100 oocysts of E. maxima or sterile water (control birds) and jejunum and serum samples collected at various days post-infection as indicated. Data are presented as individual birds. For RT-qPCR data, the relative quantity (RQ) of mRNA transcription of individual infected birds was calculated relative to the mean of control birds of the same line at individual time points and normalized using the 28S reference gene. Matching letters denote significant differences between groups on the same day (p < 0.05, n = 3 for control and n ≥ 5 for infected groups). C, control; I, infected.
Differential Kinetics of IFN-γ and IL-10 Levels in the Serum of Relatively Resistant and Susceptible Chickens Following E. maxima Infection
Unlike the levels of IFN-γ protein in the jejunum, the kinetics of serum IFN-γ differed between the lines with C.B12 peaking at 4 and 6 dpi and line 15I at 7 dpi (Figure 5D). E. maxima-infected line C.B12 exhibited significantly higher levels of serum IFN-γ at 4, 6 and 13 dpi compared to non-infected chickens. In line 15I, significantly higher serum IFN-γ was observed at 6 and 7 dpi compared to non-infected chickens. Compared to infected line C.B12, infected line 15I chickens had significantly higher serum IFN-γ at 7 dpi.
Serum IL-10 levels were significantly increased in line C.B12 following E. maxima infection at 4, 5, 6 and 7 dpi, while in line 15I, significantly increased serum IL-10 was observed from 4 to 13 dpi during E. maxima infection (Figure 5D). At 4 dpi, line C.B12 had higher levels of serum IL-10 compared to line 15I following E. maxima infection. However, serum IL-10 levels in the E. maxima-infected line 15I were significantly higher than that of C.B12 chickens at 6, 7 and 8 dpi. The levels of serum IL-10 in line 15I were dramatically increased at 7 dpi during E. maxima infection, whereas in line C.B12 chickens, serum IL-10 levels remained similar to those observed at 5 and 6 dpi.
Correlation Between Local and Systemic IFN-γ and IL-10 Production and Parasite Burden
To investigate the effect of IFN-γ and IL-10 on E. maxima burden, the correlation between jejunum and serum IFN-γ and IL-10 protein levels and E. maxima replication were calculated (Table 4). Both local (jejunum) and systemic (serum) IFN-γ and IL-10 levels in both lines of chickens correlated positively with E. maxima burden. Serum IFN-γ in line 15I correlated more strongly with E. maxima burden than in line C.B12 chickens, whereas tissue IFN-γ correlated more strongly with E. maxima burden in line C.B12 compared to line 15I chickens. Both serum and jejunum IL-10 in line 15I correlated more strongly with E. maxima burden compared to line C.B12 chickens. We also measured the effect of IFN-γ and IL-10 on BWG. Although the expression of IFN-γ and IL-10 in the jejunum and serum correlated negatively with BWG, the correlation was not significant (p > 0.05) (data not shown).
Cellular Changes Following E. maxima Infection
To investigate and compare changes to the immune cell populations in the two lines of chickens at the early stages of E. maxima infection, IHC was performed with jejunum collected at 4 dpi (Figure 7). We first compared jejunum of uninfected birds to establish if intrinsic differences between the lines existed. There was no significant difference in the number of cells expressing any of the measured cell markers in the villus lamina propria (Figure 7A) or epithelium (Figure 7B) between the two lines, although line C.B12 displayed slightly higher numbers of CD4+, CD8α+, γδ+ T and αβ1+ T cells.
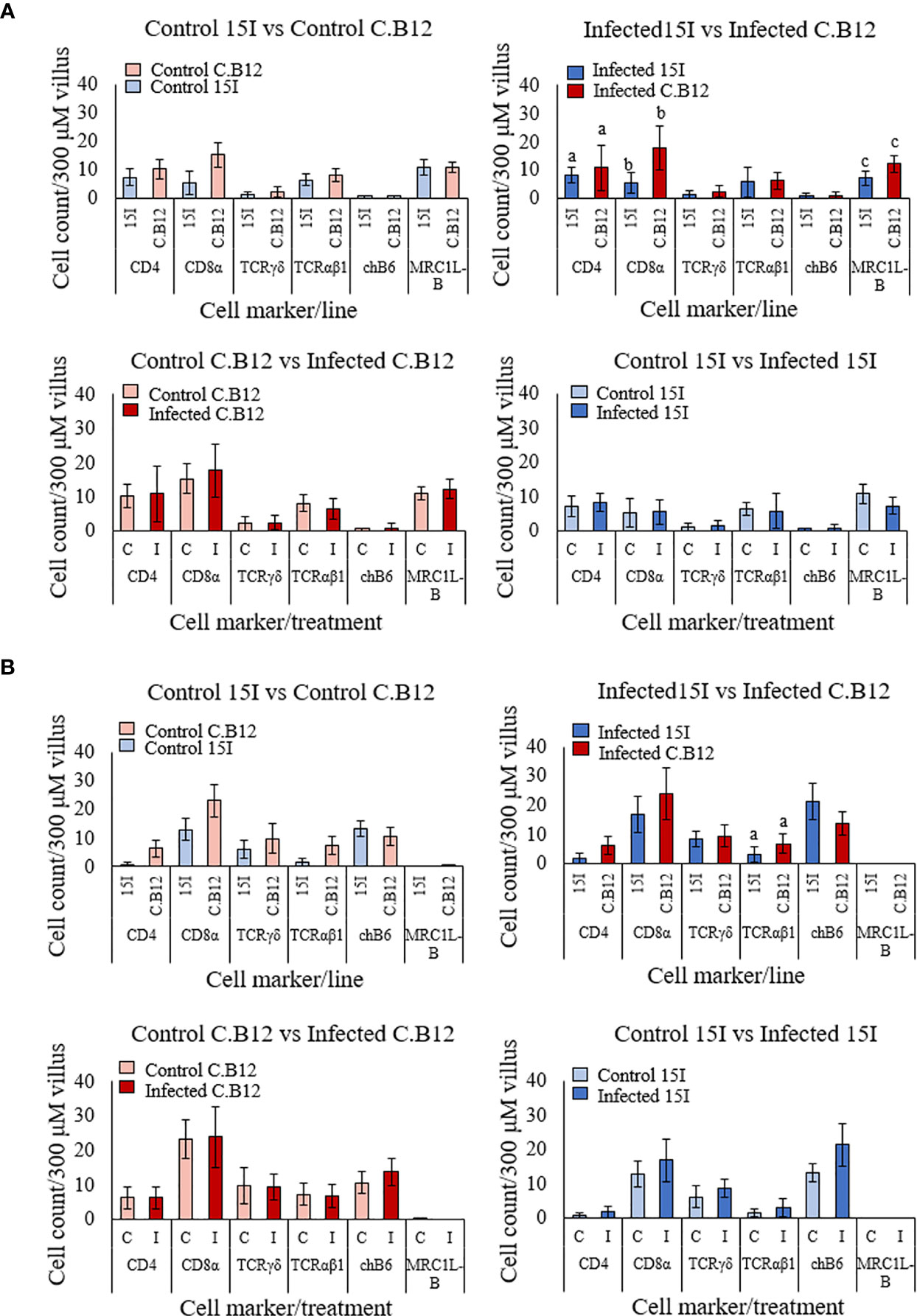
Figure 7 Populations of CD4, CD8α, γδ T cells, αβ1 T cells, chB6 and MRC1L-B LPL (A) and IEL (B) in the jejunal villi of line C.B12 and line 15I chickens at 4 dpi with E. maxima. Shown are data comparing control birds of both lines, infected birds of both lines, control and infected line C.B12 birds and control and infected line 15I birds. LPL and IEL were counted from nine villi of one section per bird (n = 3 for uninfected and n ≥ 5 for infected groups). Each bar represents the mean number of cells per 300 μm of villus (± SD). Matching letters denote significant differences between groups (p < 0.05). C, Control; I, infected.
At 4 dpi, there was no difference in the population of the measured cell markers between E. maxima-infected and non-infected chickens in the jejunal lamina propria or epithelium of either chicken line. However, comparison of the number of cells in E. maxima-infected tissues revealed significantly lower numbers of CD4+, CD8α+ and MRC1L-B+ cells in the lamina propria (Figure 7A) and αβ1+ T cells in the epithelium of the villi (Figure 7B) in line 15I compared to line C.B12 chickens.
We also measured changes to the immune cell populations in the lamina propria and epithelium of the crypts in both lines of chicken (Figure S2). There was no significant difference in the number of cells between uninfected chickens of line C.B12 and line 15I, or between E. maxima-infected and non-infected chicken within each line. Comparison of the number of cells in the crypts of E. maxima-infected chickens revealed significantly lower numbers of αβ1+ T cells and higher numbers of chB6+ cells in the epithelium of line 15I compared to line C.B12 chickens (Figure S2B).
Discussion
Understanding the basis of host resistance to E. maxima is important for the commercial poultry industry as it would enable identification of quantifiable resistance or susceptible phenotypes, allowing for the selective breeding of chickens for resistance against this and possibly other Eimeria species. Thus, investigation of host responses to Eimeria infection in the relatively resistant and susceptible White Leghorn chicken lines C.B12 and 15I has important economic implications for the poultry production industry, in addition to avian well-being and food security. In this study, we characterized the kinetics of differential gene expression in these two lines of chicken, as well as the kinetics of local and systemic protein expression and mRNA transcription of IFN-γ, IL-10, IL-21 and Th17 responses. We have also investigated cellular differences between control and infected birds of both lines during the early stages of infection. The results indicate the importance of early activation of interferon signaling pathways, with IFN-γ, IL-10 and IL-21 responses during the innate phase of infection associated with resistance to E. maxima. This research builds on previous work, investigating the importance of these responses from transcriptome to protein levels in the jejunum, the site of E. maxima infection, and systemically at the protein level in the serum.
Transcriptomic analysis of jejunal tissue from chicken lines C.B12 and 15I infected with E. maxima revealed differences in the kinetics of the host immune response and provided information on the different biological pathways involved. Commonalities between the two lines included strong upregulation of IFNG, various chemokines and complement components at 6 dpi, which agrees with previous transcriptome based analysis of chicken cecal epithelial responses to E. tenella (45). Although there was no difference in E. maxima replication at 4 dpi between the two lines, early immune responses observed in relatively resistant line C.B12 at this time point, in particular interferon responses, may be sufficient to reduce E. maxima replication by 7 dpi. In line 15I these responses did not occur until 6 dpi, potentially leading to a delay in the inhibition of E. maxima replication. While the level of E. maxima replication varies between line C.B12 and 15I chickens, it appears that the timing and progression of the parasite lifecycle does not, illustrated by the duration and profile of oocyst excretion (10) and transcription of zoite-specific transcripts such as immune mapped protein 1 (IMP1) (46). Pathways involved in Th1 and Th2 responses were also upregulated at 4 dpi in line C.B12. Although 4 dpi is likely too early for such adaptive responses, higher numbers of CD4, CD8α and αβ1 T cells were present in the jejunum of control and infected line C.B12 compared to line 15I birds, and are cell types associated with these responses. Regardless of resistance and susceptibility to E. maxima, both chicken lines share many of the same upstream regulators including IFN-γ, IL-10RA and IL-2 that may cause changes in gene expression. However similar to functional pathway analysis, all the predicted upstream regulators affect expression in line C.B12 at 4 dpi and 6 dpi, whereas line 15I chickens are not affected by the same upstream regulators until 6 dpi, supporting the importance of the early immune responses in resistance to E. maxima infection. Transcriptomic analysis also revealed a set of interferon-stimulated genes that were uniquely responding in line C.B12, including MX1, RSAD2 and OASL, that may be involved in the relative resistance displayed by line C.B12.
One of the important findings of this study was that higher increases in early (2 and 4 dpi) IFN-γ and IL-10 production were present in the E. maxima-resistant chicken line, whereas a more gradual increase (a minor increase at 2 and 4 dpi but a dramatic increase at 6 and 8 dpi) in production of these cytokines was observed in the relatively E. maxima-susceptible line. These findings indicate that the timing at which the immune response is mounted is paramount to resistance. These results were evaluated by IHC, showing that, although not statistically significant, an intrinsically higher presence of IFN-γ-producing [CD4+, αβ1+ T cells and MRC1L-B+ macrophages (47)] LPL and IEL were present in relatively resistant line C.B12 in the villi of control birds than in line 15I at 4 dpi. Moreover, significantly higher numbers of MRC1L-B+ macrophages, CD4+ and CD8α+ cells were detected in the lamina propria of infected line C.B12 compared to line 15I birds, indicating macrophage and NK cell involvement at 4 dpi. Chicken intestinal IEL include NK cells which may express CD8α (48), chB6 (49) or TCRγδ (50). Likewise, Wakelin et al. (51) showed Con A-responsive cells in the mesenteric lymph nodes appeared earlier and produced more IFN-γ in E. vermiformis-resistant mice following infection. Taken together, significantly up-regulated IFN-γ expression in the jejunum of E. maxima-infected chickens is likely due to the recruitment and stimulation of MCR1L-B+, CD4+ and CD8α+ cells. Hong et al., showed that IL10 and IFNG mRNA transcription was robustly increased at 4 and 6 dpi in CD4 and CD8 cell subpopulations following E. maxima infection (19). The current study identified higher numbers of CD4 and CD8α IEL and LPL in line C.B12, both prior to and following infection. In support of these findings, higher numbers of CD4+ IEL were detected in the duodenum during early E. acervulina infection in resistant chickens (52) and increased CD4+ LPL were detected within 24 h of intra-cecal inoculation of E. tenella sporozoites (53). These results imply that CD4+ cells are effectors of Eimeria resistance early on during infection and could be a source of the early IL-10 and IFN-γ observed in this study.
IL-10 is a pleiotropic cytokine and in addition to maintaining the Th1/Th2 balance, it is also important to normal gut homeostasis, regulating NK cell and macrophage activity, limiting proinflammatory cytokine production and promoting epithelial cell proliferation amongst other functions (54). The impact of IL-10 on the outcome of Eimeria infection is likely dependent on both the timing and magnitude of its production. Early IL-10 may be involved in mediating innate responses; pegylated recombinant human IL-10 induces IFN-γ, perforin and granzyme B secretion in CD8+ T cells (55). Other publications have indicated that IL-10 reduces the efficacy of the immune response to Eimeria. Antibody-mediated IL-10 depletion in broilers enhanced weight gain and decreased oocyst production following inoculation with an attenuated Eimeria spp. vaccine (E. maxima, E. tenella and E. acervulina) (30) and did not appear to affect adaptive immunity as IL-10-depleted-chickens displayed similar weight gains following vaccination then challenge as control birds (56). Additionally, in broilers treated with CitriStim, a yeast mannan-based feed additive, and given an attenuated vaccine (E. maxima, E. tenella and E. acervulina), reduced IL10 mRNA was found in the cecal tonsils which was accompanied by reduced oocyst shedding and improved feed efficiency and weight gains (57). Although in the study by Rothwell et al. (27), IL10 transcripts were detected in the spleen of control birds, we did not detect IL-10 at a protein level in the serum in our study. Rothwell et al. (27) also observed extremely low basal levels of IL10 mRNA in the jejunum of uninfected chickens whereas the current results suggest that IL10 mRNA is transcribed in the jejunum under normal homeostatic conditions. This discrepancy is attributable to the increased sensitivity of the primer and probe sequences used in this study (data not shown). Levels of IL-10 protein positively correlated with E. maxima replication and it is plausible E. maxima is inducing IL-10 as an immune evasion strategy. Similar to the findings by Hong et al. (19), each vaccination with E. maxima led to increased serum IL-10, however the extent to which increases were observed gradually decreased with each subsequent vaccination, whereas serum IFN-γ was only increased after the first vaccination (in our unpublished data). The current study suggests that an early, modest induction of IL-10 does not negatively impact resistance to E. maxima infection, but excessive IL-10 production disrupts the efficacy of the protective response. These findings imply that IL-10 can be suitable as a biomarker of susceptibility at late time points with E. maxima infection, but less suitable as a predictor of susceptibility prior to or early on during infection.
IL-17A and IL-17F are mainly considered cytokines of the Th17 cell lineage, which functions in autoimmune disease and defense against bacterial, fungal and parasitic pathogens (58–60). More recently IL-17A and IL-17F have been related to innate cells including NK and γδ T cells and macrophages. They are important mediators of mucosal immunity and innate responses, with functions including neutrophil recruitment, macrophage activation and IFN-γ production and chemokine and antimicrobial peptide production in epithelial cells (61, 62). As our study implies, early innate responses are key to resistance to E. maxima and previous studies have indicated that Eimeria spp. infection in chickens leads to the increased transcription of IL17A, as well as IL2 and IL6 mRNA (19, 63). In contrast, our RT-qPCR and RNA-Seq data revealed there was no significant change in IL17A, IL17F, IL2 and IL6 mRNA levels during E. maxima infection. Although IL17A and IL2 mRNA levels at 2 and 6 dpi seemed to be upregulated, the change was not significant due to the high variance between chickens within the same group. Previously it has been suggested that IL-17A impairs immunity to Eimeria spp. infection. Zhang et al. (64) showed increased IL17A mRNA transcription at 6 hours post infection with E. tenella. IL-17A depletion reduced heterophil infiltration and associated immunopathology in the ceca, but also reduced oocyst output indicating that IL-17A is involved in susceptibility to E. tenella. In addition, Del Cacho et al. (65) also found that IL-17A reduced E. tenella schizont development and migration. Among the Th17-associated genes tested, only IL21 mRNA levels were increased in the jejunum of both lines of chickens during E. maxima infection compared to non-infected chickens. A member of the IL-2 family, IL-21 plays important roles not only in Th17 differentiation, but also in innate immunity, with functions including enhancement of cytotoxicity and IFN-γ production in NK and CD8 T cells (66, 67). Additionally, IL-21 plays key roles in autoimmune disease and in shaping humoral and cellular immune responses to parasitic infection (68, 69). In chickens, increased IL21 mRNA levels are reported in autoimmune vitiligo. To date, IL-21 has not been previously found to have a role during Eimeria infection. The kinetics of our study revealed that the pattern of IL21 mRNA transcription was similar to IFNG and IL10 in the jejunum, indicating that IL-21 may also be involved in resistance to E. maxima through mediating innate immunity. Similar transcription patterns of IFNG, IL10 and IL21 mRNA were reported during the development of vitiligo lesions (70). Moreover, in mice IL-21 modulates differentiation of CD4 and CD8 T cell subsets in a context-dependent manner and certain cytokines, including IL-10, may compensate for IL-21 (71). Since E. maxima infection leads to an increase in CD8α T cell numbers, it is possible that the co-expression of IL-21, IFN-γ and IL-10 may play an important role in the enhancement of CD8 T cell responses, as reflected in the higher numbers of CD8α IEL and LPL in the jejunum of line C.B12 birds observed in this study. Previously, cytotoxic CD8 cell activity was shown to be a component of protective immunity to secondary E. tenella infection (53, 72, 73) and resistance and IFN-γ production during primary E. acervulina infection in chickens (74). The early timing of this response in line C.B12, alongside the fact that no other Th17-associated genes tested were changed during infection, suggests that Th17 responses are not involved during Eimeria spp. infection.
The present study suggests that the timing of the immune response is crucial for E. maxima resistance. Immunity to Eimeria arises during sporozoite translocation through the lamina propria in chickens (72, 75, 76). Resistance to Eimeria spp. relies on the host response in the first few days of infection, when sporozoites are present in the lamina propria and in contact with LPL as well as when intracellular in parasitophorous vacuoles (77). The increased IL-10 observed in line 15I in the serum suggests that systemic IL-10 production supports susceptibility to E. maxima, but the positive correlation of IL-10, IFN-γ and IL-21 with one another and the higher expression in resistant chickens at early time points implies that the balance between the three is imperative for effective immunity to E. maxima.
Data Availability Statement
The data sets presented in this study can be found in online repositories. The names of the repository/repositories and accession number(s) can be found below: https://www.ebi.ac.uk/ena, PRJEB42179.
Ethics Statement
The animal study was reviewed and approved by the Royal Veterinary College Animal Welfare Ethical Review Body (AWERB) and the United Kingdom Government Home Office.
Author Contributions
The project was conceived and funding obtained by PK, DPB, RH, FT, and LV. AB, SK, LV, MN, and DPB contributed to the experimental design and analysis of the study. AB, SK, and MN executed the experiment, and collected and processed samples. KM provided assistance with the analysis of RNA-Seq data and prepared figures. ZW provided reagents and advice regarding the IL-10 ELISA. DB provided advice and assistance with qRT-PCR analysis. The manuscript was drafted by AB and SK with input of LV and DPB. All authors contributed to the article and approved the submitted version.
Funding
This research was supported by the Biotechnology and Biological Sciences Research Council (BBSRC) grants BB/L502455/1 and BB/L004046/1, in partnership with Cobb-Vantress Inc. This work was also supported by the Biotechnology and Biological Sciences Research Council Institute Strategic Program Grant BBS/E/D/20231760 and BBS/E/D/20231762 to The Roslin Institute. This research has also received funding from the European Union’s Horizon 2020 Programme for research, technological development and demonstration under the Grant Agreement no. 633184 (Saphir). This publication reflects the views only of the author, and not the European Commission (EC). The EC is not liable for any use that may be made of the information contained herein. The funders had no role in study design, data collection and interpretation, or the decision to submit the work for publication.
Conflict of Interest
Author RH was employed by Cobb-Vantress Inc.
The remaining authors declare that the research was conducted in the absence of any commercial or financial relationships that could be construed as a potential conflict of interest.
Acknowledgments
We are thankful to the staff at the Bumstead Unit at the National Avian Research Facility (NARF) for the supply of the chickens used in this study and to the staff at the Biological Services Unit at the RVC for their assistance in the care of the animals. We are also grateful to Edinburgh Genomics for their assistance with the transcriptome analysis and to Dr Kate Sutton for her assistance with the production of the figures. Also with thanks to Professor John Hopkins and Dr Mitch Abrahamson for their advice throughout this research.
Supplementary Material
The Supplementary Material for this article can be found online at: https://www.frontiersin.org/articles/10.3389/fimmu.2021.653085/full#supplementary-material
References
1. Williams RB, Carlyle WW, Bond DR, Brown IA. The efficacy and economic benefits of Paracox, a live attenuated anticoccidial vaccine, in commercial trials with standard broiler chickens in the United Kingdom. Int J Parasitol (1999) 29:341–55. doi: 10.1016/s0020-7519(98)00212-4
2. Dalloul RA, Lillehoj HS. Poultry coccidiosis: recent advancements in control measures and vaccine development. Expert Rev Vaccines (2006) 5:143–63. doi: 10.1586/14760584.5.1.143
3. Long PL, Joyner LP. Problems in the identification of species of Eimeria. J Protozool (1984) 31:535–41. doi: 10.1111/j.1550-7408.1984.tb05498.x
4. Clark EL, Macdonald SE, Thenmozhi V, Kundu K, Garg R, Kumar S, et al. Cryptic Eimeria genotypes are common across the southern but not northern hemisphere. Int J Parasitol (2016) 46:537–44. doi: 10.1016/j.ijpara.2016.05.006
5. Long PL, Millard BJ. Studies on site finding and site specificity of Eimeria praecox, Eimeria maxima and Eimeria acervulina in chickens. Parasitology (1976) 73:327–36. doi: 10.1017/s0031182000047004
6. Yadav A, Gupta SK. Study of resistance against some ionophores in Eimeria tenella field isolates. Vet Parasitol (2001) 102:69–75. doi: 10.1016/s0304-4017(01)00512-x
7. Blake DP, Tomley FM. Securing poultry production from the ever-present Eimeria challenge. Trends Parasitol (2014) 30:12–9. doi: 10.1016/j.pt.2013.10.003
8. Bumstead JM, Bumstead N, Rothwell L, Tomley FM. Comparison of immune responses in inbred lines of chickens to Eimeria maxima and Eimeria tenella. Parasitology (1995) 111(Pt 2):143–51. doi: 10.1017/s003118200006488x
9. Smith AL, Hesketh P, Archer A, Shirley MW. Antigenic diversity in Eimeria maxima and the influence of host genetics and immunization schedule on cross-protective immunity. Infect Immun (2002) 70:2472–9. doi: 10.1128/iai.70.5.2472-2479.2002
10. Blake DP, Hesketh P, Archer A, Shirley MW, Smith AL. Eimeria maxima: the influence of host genotype on parasite reproduction as revealed by quantitative real-time PCR. Int J Parasitol (2006) 36:97–105. doi: 10.1016/j.ijpara.2005.09.011
11. Lillehoj HS, Ruff MD. Comparison of disease susceptibility and subclass-specific antibody response in SC and FP chickens experimentally inoculated with Eimeria tenella, E. acervulina, or E. maxima. Avian Dis (1987) 31:112–9. doi: 10.2307/1590782
12. Lillehoj HS. Immune response during coccidiosis in SC and FP chickens. I. In vitro assessment of T cell proliferation response to stage-specific parasite antigens. Vet Immunol Immunopathol (1986) 13:321–30. doi: 10.1016/0165-2427(86)90025-5
13. Smith NC, Wallach M, Miller CM, Morgenstern R, Braun R, Eckert J. Maternal transmission of immunity to Eimeria maxima: enzyme-linked immunosorbent assay analysis of protective antibodies induced by infection. Infect Immun (1994) 62:1348–57. doi: 10.1128/IAI.62.4.1348-1357.1994
14. Davis PJ, Parry SH, Porter P. The role of secretory IgA in anti-coccidial immunity in the chicken. Immunology (1978) 34:879–88.
15. Yun CH, Lillehoj HS, Zhu J, Min W. Kinetic differences in intestinal and systemic interferon-gamma and antigen-specific antibodies in chickens experimentally infected with Eimeria maxima. Avian Dis (2000a) 44:305–12. doi: 10.2307/1592544
16. Rose ME, Hesketh P. Immunity to coccidiosis: T-lymphocyte- or B-lymphocyte-deficient animals. Infect Immun (1979) 26:630–7. doi: 10.1128/IAI.26.2.630-637.1979
17. Bumstead N, Millard B. Genetics of resistance to coccidiosis: response of inbred chicken lines to infection by Eimeria tenella and Eimeria maxima. Br Poult Sci (1987) 28:705–15. doi: 10.1080/00071668708417006
18. Rothwell L, Gramzinski RA, Rose ME, Kaiser P. Avian coccidiosis: changes in intestinal lymphocyte populations associated with the development of immunity to Eimeria maxima. Parasite Immunol (1995) 17:525–33. doi: 10.1111/j.1365-3024.1995.tb00883.x
19. Hong YH, Lillehoj HS, Lillehoj EP, Lee SH. Changes in immune-related gene expression and intestinal lymphocyte subpopulations following Eimeria maxima infection of chickens. Vet Immunol Immunopathol (2006) 114:259–72. doi: 10.1016/j.vetimm.2006.08.006
20. Djeraba A, Bernardet N, Dambrine G, Quere P. Nitric oxide inhibits Marek’s disease virus replication but is not the single decisive factor in interferon-gamma-mediated viral inhibition. Virology (2000) 277:58–65. doi: 10.1006/viro.2000.0576
21. Mallick AI, Haq K, Brisbin JT, Mian MF, Kulkarni RR, Sharif S. Assessment of bioactivity of a recombinant chicken interferon-gamma expressed using a baculovirus expression system. J Interferon Cytokine Res (2011) 31:493–500. doi: 10.1089/jir.2010.0130
22. Kogut MH, Rothwell L, Kaiser P. IFN-gamma priming of chicken heterophils upregulates the expression of proinflammatory and Th1 cytokine mRNA following receptor-mediated phagocytosis of Salmonella enterica serovar enteritidis. J Interferon Cytokine Res (2005) 25:73–81. doi: 10.1089/jir.2005.25.73
23. Heriveau C, Dimier-Poisson I, Lowenthal J, Naciri M, Quere P. Inhibition of Eimeria tenella replication after recombinant IFN-gamma activation in chicken macrophages, fibroblasts and epithelial cells. Vet Parasitol (2000) 92:37–49. doi: 10.1016/s0304-4017(00)00275-2
24. Lillehoj HS, Choi KD. Recombinant chicken interferon-gamma-mediated inhibition of Eimeria tenella development in vitro and reduction of oocyst production and body weight loss following Eimeria acervulina challenge infection. Avian Dis (1998) 42:307–14. doi: 10.2307/1592481
25. Lowenthal JW, York JJ, O’Neil TE, Rhodes S, Prowse SJ, Strom DG, et al. In vivo effects of chicken interferon-gamma during infection with Eimeria. J Interferon Cytokine Res (1997) 17:551–8. doi: 10.1089/jir.1997.17.551
26. Yun CH, Lillehoj HS, Choi KD. Eimeria tenella infection induces local gamma interferon production and intestinal lymphocyte subpopulation changes. Infect Immun (2000b) 68:1282–8. doi: 10.1128/iai.68.3.1282-1288.2000
27. Rothwell L, Young JR, Zoorob R, Whittaker CA, Hesketh P, Archer A, et al. Cloning and characterization of chicken IL-10 and its role in the immune response to Eimeria maxima. J Immunol (2004) 173:2675–82. doi: 10.4049/jimmunol.173.4.2675
28. Haritova AM, Stanilova SA. Enhanced expression of IL-10 in contrast to IL-12B mRNA in poultry with experimental coccidiosis. Exp Parasitol (2012) 132:378–82. doi: 10.1016/j.exppara.2012.08.017
29. Wu Z, Hu T, Rothwell L, Vervelde L, Kaiser P, Boulton K, et al. Analysis of the function of IL-10 in chickens using specific neutralising antibodies and a sensitive capture ELISA. Dev Comp Immunol (2016) 63:206–12. doi: 10.1016/j.dci.2016.04.016
30. Arendt MK, Sand JM, Marcone TM, Cook ME. Interleukin-10 neutralizing antibody for detection of intestinal luminal levels and as a dietary additive in Eimeria challenged broiler chicks. Poult Sci (2016) 95:430–8. doi: 10.3382/ps/pev365
31. Norton CC, Joyner LP. The development of drug-resistant strains of Eimeria maxima in the laboratory. Parasitology (1975) 71:153–65. doi: 10.1017/s0031182000053233
32. Long PL, Millard BJ, Joyner LP, Norton CC. A guide to laboratory techniques used in the study and diagnosis of avian coccidiosis. Folia Vet Lat (1976) 6:201–17.
33. Johnson J, Reid WM. Anticoccidial drugs: lesion scoring techniques in battery and floor-pen experiments with chickens. Exp Parasitol (1970) 28:30–6. doi: 10.1016/0014-4894(70)90063-9
34. Nolan MJ, Tomley FM, Kaiser P, Blake DP. Quantitative real-time PCR (qPCR) for Eimeria tenella replication–Implications for experimental refinement and animal welfare. Parasitol Int (2015) 64:464–70. doi: 10.1016/j.parint.2015.06.010
35. Li YP, Bang DD, Handberg KJ, Jorgensen PH, Zhang MF. Evaluation of the suitability of six host genes as internal control in real-time RT-PCR assays in chicken embryo cell cultures infected with infectious bursal disease virus. Vet Microbiol (2005) 110:155–65. doi: 10.1016/j.vetmic.2005.06.014
36. Sutton KM, Hu T, Wu Z, Siklodi B, Vervelde L, Kaiser P. The functions of the avian receptor activator of NF-kappaB ligand (RANKL) and its receptors, RANK and osteoprotegerin, are evolutionarily conserved. Dev Comp Immunol (2015) 51:170–84. doi: 10.1016/j.dci.2015.03.006
37. Kaiser P, Rothwell L, Galyov EE, Barrow PA, Burnside J, Wigley P. Differential cytokine expression in avian cells in response to invasion by Salmonella typhimurium, Salmonella enteritidis and Salmonella gallinarum. Microbiology (2000) 146 Pt 12:3217–26. doi: 10.1099/00221287-146-12-3217
38. Bolger AM, Lohse M, Usadel B. Trimmomatic: a flexible trimmer for Illumina sequence data. Bioinformatics (2014) 30:2114–20. doi: 10.1093/bioinformatics/btu170
39. Dobin A, Davis CA, Schlesinger F, Drenkow J, Zaleski C, Jha S, et al. STAR: ultrafast universal RNA-seq aligner. Bioinformatics (2013) 29:15–21. doi: 10.1093/bioinformatics/bts635
40. Liao Y, Smyth GK, Shi W. featureCounts: an efficient general purpose program for assigning sequence reads to genomic features. Bioinformatics (2014) 30:923–30. doi: 10.1093/bioinformatics/btt656
41. Robinson MD, McCarthy DJ, Smyth GK. edgeR: a Bioconductor package for differential expression analysis of digital gene expression data. Bioinformatics (2010) 26:139–40. doi: 10.1093/bioinformatics/btp616
42. Theocharidis A, van Dongen S, Enright AJ, Freeman TC. Network visualization and analysis of gene expression data using BioLayout Express(3D). Nat Protoc (2009) 4:1535–50. doi: 10.1038/nprot.2009.177
43. Psifidi A, Hesketh P, Stebbings A, Archer A, Salmon N, Matika O, et al. (2013). Identification of SNP markers for resistance to coccidiosis in chickens, in: Abstract from Proceedings of the 8th European Poultry Genetics Symposium, Venice, Italy. p. 16.
44. Boulton K, Nolan MJ, Wu Z, Riggio V, Matika O, Harman K, et al. Dissecting the genomic architecture of resistance to Eimeria maxima parasitism in the chicken. Front Genet (2018) 9:528. doi: 10.3389/fgene.2018.00528
45. Guo P, Thomas JD, Bruce MP, Hinton TM, Bean AG, Lowenthal JW. The chicken TH1 response: potential therapeutic applications of ChIFN-gamma. Dev Comp Immunol (2013) 41:389–96. doi: 10.1016/j.dci.2013.05.009
46. Blake DP, Billington KJ, Copestake SL, Oakes RD, Quail MA, Wan K-L, et al. Genetic mapping identifies noval highly protective antigens for an apicomplexan parasite. PloS Pathog (2011) 7:e1001279. doi: 10.1371/journal.ppat.1001279
47. Staines K, Hunt LG, Young JR, Butter C. Evolution of an expanded mannose receptor gene family. PloS One (2014) 9:e110330. doi: 10.1371/journal.pone.0110330
48. Gobel TW, Chen CL, Shrimpf J, Grossi CE, Bernot A, Bucy RP, et al. Characterization of avian natural killer cells and their intracellular CD3 protein complex. Eur J Immunol (1994) 24:1685–91. doi: 10.1002/eji.1830240734
49. Vervelde L, Jeurissen SH. Postnatal development of intra-epithelial leukocytes in the chicken digestive tract: phenotypical characterization in situ. Cell Tissue Res (1993) 274:295–301. doi: 10.1007/BF00318748
50. Jansen CA, van de Haar PM, van Haarlem D, van Kooten P, de Wit S, van Eden W, et al. Identification of new populations of chicken natural killer (NK) cells. Dev Comp Immunol (2010) 34:759–67. doi: 10.1016/j.dci.2010.02.009
51. Wakelin D, Rose ME, Hesketh P, Else KJ, Grencis RK. Immunity to coccidiosis: genetic influences on lymphocyte and cytokine responses to infection with Eimeria vermiformis in inbred mice. Parasite Immunol (1993) 15:11–9. doi: 10.1111/j.1365-3024.1993.tb00567.x
52. Choi KD, Lillehoj HS, Zalenga DS. Changes in local IFN-gamma and TGF-beta4 mRNA expression and intraepithelial lymphocytes following Eimeria acervulina infection. Vet Immunol Immunopathol (1999) 71:263–75. doi: 10.1016/s0165-2427(99)00103-8
53. Vervelde L, Vermeulen AN, Jeurissen SH. In situ characterization of leucocyte subpopulations after infection with Eimeria tenella in chickens. Parasite Immunol (1996) 18:247–56. doi: 10.1046/j.1365-3024.1996.d01-94.x
54. Couper KN, Blount DG, Riley EM. IL-10: the master regulator of immunity to infection. J Immunol (2008) 180:5771–7. doi: 10.4049/jimmunol.180.9.5771
55. Chan IH, Wu V, Bilardello M, Mar E, Oft M, Van Vlasselaer P, et al. The potentiation of IFN-gamma and induction of cytotoxic proteins by pegylated IL-10 in guman CD8 T cells. J Interferon Cytokine Res (2015) 35:948–55. doi: 10.1089/jir.2014.0221
56. Sand JM, Arendt MK, Repasy A, Deniz G, Cook ME. Oral antibody to interleukin-10 reduces growth rate depression due to Eimeria spp. infection in broiler chickens. Poult Sci (2016) 95:439–46. doi: 10.3382/ps/pev352
57. Shanmugasundaram R, Sifri M, Selvaraj RK. Effect of yeast cell product (CitriStim) supplementation on broiler performance and intestinal immune cell parameters during an experimental coccidial infection. Poult Sci (2013) 92:358–63. doi: 10.3382/ps.2012-02776
58. Cai CW, Blase JR, Zhang X, Eickhoff CS, Hoft DF. Th17 cells are more protective than Th1 cells against the intracellular parasite Trypanosoma cruzi. PloS Pathog (2016) 12:e1005902. doi: 10.1371/journal.ppat.1005902
59. Korn T, Bettelli E, Oukka M, Kuchroo VK. IL-17 and Th17 Cells. Annu Rev Immunol (2009) 27:485–517. doi: 10.1146/annurev.immunol.021908.132710
60. Noack M, Miossec P. Th17 and regulatory T cell balance in autoimmune and inflammatory diseases. Autoimmun Rev (2014) 13:668–77. doi: 10.1016/j.autrev.2013.12.004
61. Kolls JK, Khader SA. The role of Th17 cytokines in primary mucosal immunity. Cytokine Growth Factor Rev (2010) 21:443–8. doi: 10.1016/j.cytogfr.2010.11.002
62. Veldhoen M. Interleukin 17 is a chief orchestrator of immunity. Nat Immunol (2017) 18:612–21. doi: 10.1038/ni.3742
63. Kim WH, Jeong J, Park AR, Yim D, Kim YH, Kim KD, et al. Chicken IL-17F: identification and comparative expression analysis in Eimeria-infected chickens. Dev Comp Immunol (2012) 38:401–9. doi: 10.1016/j.dci.2012.08.002
64. Zhang L, Liu R, Song M, Hu Y, Pan B, Cai J, et al. Eimeria tenella: interleukin 17 contributes to host immunopathology in the gut during experimental infection. Exp Parasitol (2013) 133:121–30. doi: 10.1016/j.exppara.2012.11.009
65. Del Cacho E, Gallego M, Lillehoj HS, Quilez J, Lillehoj EP, Ramo A, et al. IL-17A regulates Eimeria tenella schizont maturation and migration in avian coccidiosis. Vet Res (2014) 45:25. doi: 10.1186/1297-9716-45-25
66. Skak K, Frederiksen KS, Lundsgaard D. Interleukin-21 activates human natural killer cells and modulates their surface receptor expression. Immunology (2008) 123:575–83. doi: 10.1111/j.1365-2567.2007.02730.x
67. Wendt K, Wilk E, Buyny S, Schmidt RE, Jacobs R. Interleukin-21 differentially affects human natural killer cell subsets. Immunology (2007) 122:486–95. doi: 10.1111/j.1365-2567.2007.02675.x
68. Perez-Mazliah D, Ng DH, Freitas do Rosario AP, McLaughlin S, Mastelic-Gavillet B, Sodenkamp J, et al. Disruption of IL-21 signaling affects T cell-B cell interactions and abrogates protective humoral immunity to malaria. PloS Pathog (2015) 11:e1004715. doi: 10.1371/journal.ppat.1004715
69. Yi JS, Cox MA, Zajac AJ. Interleukin-21: a multifunctional regulator of immunity to infections. Microbes Infect (2010) 12:1111–9. doi: 10.1016/j.micinf.2010.08.008
70. Shi F, Erf GF. IFN-gamma, IL-21, and IL-10 co-expression in evolving autoimmune vitiligo lesions of Smyth line chickens. J Invest Dermatol (2012) 132:642–9. doi: 10.1038/jid.2011.377
71. Pot C, Jin H, Awasthi A, Liu SM, Lai CY, Madan R, et al. Cutting edge: IL-27 induces the transcription factor c-Maf, cytokine IL-21, and the costimulatory receptor ICOS that coordinately act together to promote differentiation of IL-10-producing Tr1 cells. J Immunol (2009) 183:797–801. doi: 10.4049/jimmunol.0901233
72. Jeurissen SH, Janse EM, Vermeulen AN, Vervelde L. Eimeria tenella infections in chickens: aspects of host-parasite: interaction. Vet Immunol Immunopathol (1996) 54:231–8. doi: 10.1016/s0165-2427(96)05689-9
73. Wattrang E, Thebo P, Lunden A, Dalgaard TS. Monitoring of local CD8beta-expressing cell populations during Eimeria tenella infection of naive and immune chickens. Parasite Immunol (2016) 38:453–67. doi: 10.1111/pim.12331
74. Swinkels WJ, Post J, Cornelissen JB, Engel B, Boersma WJ, Rebel JM. Immune responses to an Eimeria acervulina infection in different broilers lines. Vet Immunol Immunopathol (2007) 117:26–34. doi: 10.1016/j.vetimm.2007.01.020
75. Jenkins MC, Augustine PC, Danforth HD, Barta JR. X-irradiation of Eimeria tenella oocysts provides direct evidence that sporozoite invasion and early schizont development induce a protective immune response(s). Infect Immun (1991) 59:4042–8. doi: 10.1128/IAI.59.11.4042-4048
76. Vervelde L, Jeurissen SH. The role of intra-epithelial and lamina propria leucocytes during infection with Eimeria tenella. Adv Exp Med Biol (1995) 371B:953–8.
77. Dubey JP, Jenkins MC. Re-evaluation of the life cycle of Eimeria maxima Tyzzein chickens (Gallus domesticus). Parasitology (2018) 145:1051–8. doi: 10.1017/S0031182017002153
78. Chan MM, Chen CL, Ager LL, Cooper MD. Identification of the avian homologues of mammalian CD4 and CD8 antigens. J Immunol (1988) 140:2133–8.
79. Luhtala M, Koskinen R, Toivanen P, Vainio O. Characterization of chicken CD8-specific monoclonal antibodies recognizing novel epitopes. Scand J Immunol (1995) 42:171–4. doi: 10.1111/j.1365-3083.1995.tb03641.x
80. Chen CH, Cihak J, Lösch U, Cooper MD. Differential expression of two T cell receptors, TCR1 and TCR2, on chicken lymphocytes. Eur J Immunol (1988) 18:539–43. doi: 10.1002/eji.1830180408
81. Mast J, Goddeeris BM, Peeters K, Vandesande F, Berghman LR. Characterisation of chicken monocytes, macrophages and interdigitating cells by the monoclonal antibody KUL01. Vet Immunol Immunopathol (1998) 61:343–57. doi: 10.1016/s0165-2427(97)00152-9
Keywords: Eimeria, immune response, genetic resistance, interleukin-10, interferon
Citation: Bremner A, Kim S, Morris KM, Nolan MJ, Borowska D, Wu Z, Tomley F, Blake DP, Hawken R, Kaiser P and Vervelde L (2021) Kinetics of the Cellular and Transcriptomic Response to Eimeria maxima in Relatively Resistant and Susceptible Chicken Lines. Front. Immunol. 12:653085. doi: 10.3389/fimmu.2021.653085
Received: 13 January 2021; Accepted: 08 March 2021;
Published: 25 March 2021.
Edited by:
Falko Steinbach, University of Surrey, United KingdomReviewed by:
Sung Hyen Lee, National Institute of Agricultural Science, South KoreaXianyong Liu, China Agricultural University, China
Copyright © 2021 Bremner, Kim, Morris, Nolan, Borowska, Wu, Tomley, Blake, Hawken, Kaiser and Vervelde. This is an open-access article distributed under the terms of the Creative Commons Attribution License (CC BY). The use, distribution or reproduction in other forums is permitted, provided the original author(s) and the copyright owner(s) are credited and that the original publication in this journal is cited, in accordance with accepted academic practice. No use, distribution or reproduction is permitted which does not comply with these terms.
*Correspondence: Lonneke Vervelde, TG9ubmVrZS52ZXJ2ZWxkZUByb3NsaW4uZWQuYWMudWs=
†Deceased