- 1School of Chinese Materia Medica, Tianjin University of Traditional Chinese Medicine, Tianjin, China
- 2School of Life Sciences, Tian Jin University, Tian Jin, China
- 3College of Pharmaceutical Engineering of Traditional Chinese Medicine, Tianjin University of Traditional Chinese Medicine, Tianjin, China
γδ T cells are the unique T cell subgroup with their T cell receptors composed of γ chain and δ chain. Unlike αβ T cells, γδ T cells are non-MHC-restricted in recognizing tumor antigens, and therefore defined as innate immune cells. Activated γδ T cells can promote the anti-tumor function of adaptive immune cells. They are considered as a bridge between adaptive immunity and innate immunity. However, several other studies have shown that γδ T cells can also promote tumor progression by inhibiting anti-tumor response. Therefore, γδ T cells may have both anti-tumor and tumor-promoting effects. In order to clarify this contradiction, in this review, we summarized the functions of the main subsets of human γδ T cells in how they exhibit their respective anti-tumor or pro-tumor effects in cancer. Then, we reviewed recent γδ T cell-based anti-tumor immunotherapy. Finally, we summarized the existing problems and prospect of this immunotherapy.
Introduction
γδ T cells are the non-classical cell subgroup characterized by expression of γδ heterodimeric T cell receptor (TCRγδ) on cell surface. They only account for 1% to 5% of T lymphocytes in peripheral blood circulation and lymphatic circulation, and predominantly reside in the mucosal tissues such as skin, intestine, lung, and uterus (1–3). γδ T cells are the intermediate group of cells between innate and adaptive immune cells, serving as a bridge between innate immunity and adaptive immune response (4, 5). They play important roles in tumor immunity. Depending on the microenvironment, different γδ T cell subgroups can have anti-tumor or pro-tumor activities.
Compare with αβ T cells, γδ T cells have different antigen recognition mechanisms and capabilities without the histocompatibility complex (MHC) and the second signal (CD28 and CD80/86) (6). They can use TCRγδ and natural killer cell receptors (NKR) to recognize a variety of tumor-associated antigens (TAA), including non-peptidic prenyl-pyrophosphate antigens (PAg) and stress proteins (7). The PAg are products of isoprenoid biosynthesis pathways, such as isoprene pyrophosphate (IPP) from mammalian cells and (E)-4-Hydroxy-3-Methylbut-2-Enyl Diphosphate (HMBPP, the strongest stimulant of γδ T cells) from pathogenic microorganisms (8–12). Besides, the stress proteins will up-regulate or ectopically express under the stress conditions, such as apolipoprotein A1-F1-ATPase complex (F1-ATPase Apo A1) (13), MHC-like molecules MICA/B, UL16 Binding protein (ULBP) (14–18), endothelial cell protein C receptor (EPCR) (19, 20), heat shock protein (21–23) and human MutS homolog 2 (24–26). These antigens can activate γδ T cells to secrete interferon γ (IFN-γ) and tumor necrosis factor α (TNF-α) (6), or kill tumor cells through Fas/FasL and antibody-dependent cell-mediated cytotoxicity (ADCC) (27–30). Moreover, γδ T cells can also enhance the anti-tumor ability of other immune cells by secreting cytokines or expressing costimulatory molecules. For example, human γδ T cells can stimulate the cytotoxicity of NK cells through expressed the costimulatory molecule CD137L (31). γδ T cells have been used in clinic for the treatment of non-small cell lung cancer and breast cancer. Such γδ T-based immunotherapy appeared to be safe and well-tolerated in patients (32–35).
However, it was reported that γδ T cells could also promote cancer development (36). For example, as one of the main sources of interleukin-17 (IL-17), tumor-infiltrating γδ T cells were shown to promote tumor development and metastasis by enhancing angiogenesis and recruiting inhibitory cells (37–40). Tumor-infiltrating γδ T cells could also directly induce the apoptosis of anti-tumor immune cells (41).
In this review, we introduced the classification of human γδ T cells and summarized how γδ T cell subsets play different roles in tumorigenesis. We further discussed the γδ T cell-based anti-tumor immunotherapy which has been widely used in clinic. Finally, we briefly summarized the current limitation and caveats associated with such therapy, and proposed new approach for optimization. We believe that the summary of biological functions of different γδ T cells can help us improve our understanding of tumor microenvironment, and provide novel insights for anti-tumor immunity.
Classification of γδ T Cells
Human γδ T cells can be classified into different groups based on the expression of TCRγ chains or TCRδ chains, and they can be further classified by the expression of different CD molecules (42, 43).
Classification Based on the Expression of TCRγ Chain or TCRδ Chain
Different TCRγ chains (Vγ2/3/4/5/8/9) and TCRδ chains (Vδ1/2/3/5) can be combined to form different types of γδ T cells. Interestingly, each TCRδ chain usually forms with one or several dominant TCRγ chains a fixed combination pattern, rather than with random combinations (44–47).
Different γδ T cells have diversified distribution and functions. Vδ1 chain can interact with different γ chains to form various γδ T cells. They are mainly distributed in the skin, intestine, liver, spleen and mucosal tissues. The role of Vδ1 T cells is controversial. In certain situations, they have been shown to have strong anti-tumor effects in colorectal cancer, multiple myeloma, chronic lymphocytic leukemia (48–50). On the other hand, tumor-infiltrating Vδ1 T cells often demonstrate strong immunosuppressive effects. They secreted IL-17 and transforming growth factor-β (TGF-β) (51), expressed programmed cell death 1 ligand 1 (PD-L1), and inhibited the activation of other immune cells (41).
The Vδ2 chain only combines with the Vγ9 chain to form the Vγ9Vδ2 T cells, which mainly exist in peripheral blood. Given that the Vγ9Vδ2 T cells have strong anti-tumor effects in various types of tumors, they were widely used in clinics (52–55). In addition, they have also been shown to kill the cancer stem cells (CSC) in various tumors including colon cancer, ovarian cancer, and neuroblastoma (56, 57).
The Vδ3 chain mainly interacts with the Vγ2 and Vγ3 chains. Vδ3 T cells mainly exist in the liver, and also in a small amount in the peripheral blood of patients with chronic lymphocytic leukemia. The functions of Vδ3 T cells in tumors have not been elucidated in depth (58–61).
The Vδ5 chain usually combines with the Vγ4 chain to form the Vγ4Vδ5 T cells. They mainly exist in peripheral blood. The TCR of Vγ4Vδ5 T cells could directly bind to the endothelial protein C receptor (EPCR) to recognize epithelial tumor cells. Like Vδ3 T cells, they were rarely studied for their tumor-related functions (19, 62) (Table 1).
Classifications Based on the Phenotype of CD Molecules
Human γδ T cells can be classified based on the expression of CD27 and CD45RA. The naive type (Tnaive, CD27+CD45RA+) and the central-memory phenotype (TCM, CD27+CD45RA-), mainly exist in the secondary lymphoid organs. TCM can maintain immune memory for a long time and quickly mediate immune response after receiving antigen stimulation. The effector-memory type (TEM, CD27-CD45RA-) and terminally-differentiated type (TEMRA, CD27-CD45RA+) mainly exist at the site of inflammation and exert instant effects, namely secreting cytokines and exerting cytotoxicity (63, 64).
Classification of γδ T Cells According to Their Cellular Function
Based on their varied functions, γδ T cells can be divided into several subtypes. Similar to αβ T cells, effector γδ T cells can exert an anti-tumor effect through various pathways. Regulatory γδ T cells (CD4+CD25+Foxp3+) or inhibitory γδ T cells can regulate the immune balance and maintain immune tolerance (17, 51). In addition, γδ T17 cells can produce IL-17 to promote tumor development (6, 36, 41).
γδ T Cells Play a Direct Anti-Tumor Role
The Tumor-Associated Antigens Recognition by γδ T Cells
Vγ9Vδ2 T cells recognizes TAA through TCRγδ and NKR and Vγ9Vδ2TCR can recognize PAg. This type of antigen was a product of isoprenoid biosynthesis pathway in eukaryotic cells, such as IPP and the adenylated, thymidylated, and uridylated triphosphate derivatives. In tumor cells, the isoprenoid biosynthetic pathway is enhanced to ensure energy supply and PAg accumulation, prompting recognition by the Vγ9Vδ2 T cells (65–68). Vγ9Vδ2TCR requires the help of Butyrophilin (BTN) 3A1 to recognize tumor cells. BTN3A1 is an immunoglobulin-like molecule with immunomodulatory function, which could mediate the interaction between γδ T cells and PAg, and could also be directly recognized by Vγ9Vδ2TCR (69–71). There were two theories on how BTN3A1 helps Vγ9Vδ2TCR recognize PAg. The first proposed mechanism was that BTN3A1 is a sensor that senses the level of PAg inside the cell. The intracellular B30.2 domain of the BTN3A1 molecule is a positively charged pocket that could directly bind to PAg, lead to changes in the structure of the extracellular dimer of BTN3A1 that can be recognized by Vγ9Vδ2TCR, and then activate the γδ T cells (72–77). The second proposed mechanism was that BTN3A1 formed a BTN3A1-PAg complex with PAg, presented PAg to the outside of the cell, and directly bound to Vγ9Vδ2TCR to activate γδ T cells (78). The latest study found that BTN2A1, which was in the same family as BTN3A1, was also a ligand for Vγ9Vδ2TCR and necessary for Vγ9Vδ2 T cells to recognize PAg. BTN2A1 and BTN3A1 can be found on the surface of tumor cells and recognized by two sites of Vγ9Vδ2TCR. BTN2A1 is recognized by the Vγ9 area, and BTN3A1 is recognized by the Vδ2 area (79, 80). In addition, Vγ9Vδ2TCR could recognize the F1-ATPaseApoA1 complex. This complex are normally expressed in the inner membrane of mitochondria, but some tumor cells, such as human leukemia (K562) cells and lymphoma (Raji) cells, could ectopic express it on the cell membrane. ApoA1 in the complex could not directly activate Vγ9Vδ2 T cells, instead it plays a function in stabilizing the interaction between Vγ9Vδ2TCR and F1-ATPase (13, 81).
Vγ9Vδ2 T cells could also recognize TAA through NKR, such as the natural killer 2D receptor (NKG2D) and DNAX accessory molecule 1 (DNAM-1). NKG2D is a lectin-type activation receptor, expressed on most natural killer cells (NK) and natural killer T (NKT) cells and partly expressed on γδ T cells and antigen-activated CD8+ T cells (82). When γδ T cells contacted by the tumor cells, Vγ9+ subpopulations rapidly proliferated, and γδ T cells up-regulated their NKG2D expression (83). NKG2D ligands on tumor cells include MICA, MICB and ULBP1~4 (84, 85). They could be recognized by NKG2D and enable γδ T cells to exert anti-tumor function (82). DNAM-1 is expressed on the γδ T cells and believed to promote the secretion of cytokines and enhance the cytotoxicity of immune cells. Vγ9Vδ2 T cells used DNAM-1 to recognize Nectin-2 and PVR, which were widely expressed on the tumor cells (86–88). Shielding DNAM-1 from the surface of γδ T cells could significantly inhibit its ability to kill tumor cells (89). It was shown that DNAM-1 is one of the important factors mediating γδ T cells to recognize tumor cells.
Vδ1 T cells also recognize tumor cells through TCRγδ and NKR. Vδ1TCR could recognize MHC-like molecule CD1d and the lipid antigen presented by it (90, 91). CD1d is expressed on a variety of cancers, such as myeloma, breast cancer and prostate cancer (92–94). The decrease of CD1d molecules on the primitive neuroectodermal tumor cells would cause these cells to evade immune recognition (95). In addition, Vδ1TCR could recognize tumor cells through MICA, but the MICA bindings by Vδ1TCR and NKG2D were mutually exclusive (96). Vδ1 T cells also express NKR. These cells recognize ULBP3 which is expressed on chronic lymphocytic leukemia of B-cell type (B-CLL) through NKR (97). They recognize human breast cancer cells through NKG2D, significantly preventing the disease progression (35). In addition to NKG2D and DNAM-1, Vδ1 T cells stimulated by IL-2 or IL-15 also express NKp30, NKp44 and NKp46 (48, 98), and have strong IFN-γ secretion ability (99, 100). Moreover, it has been confirmed that in acute myeloid leukemia, the ligand of NKp30 is B7-H6, a member of the B7 family (101).
Other studies have also confirmed that Vγ4Vδ5TCR can recognize EPCR, which is expressed on the epithelial tumor cells (19, 20) (Figure 1).
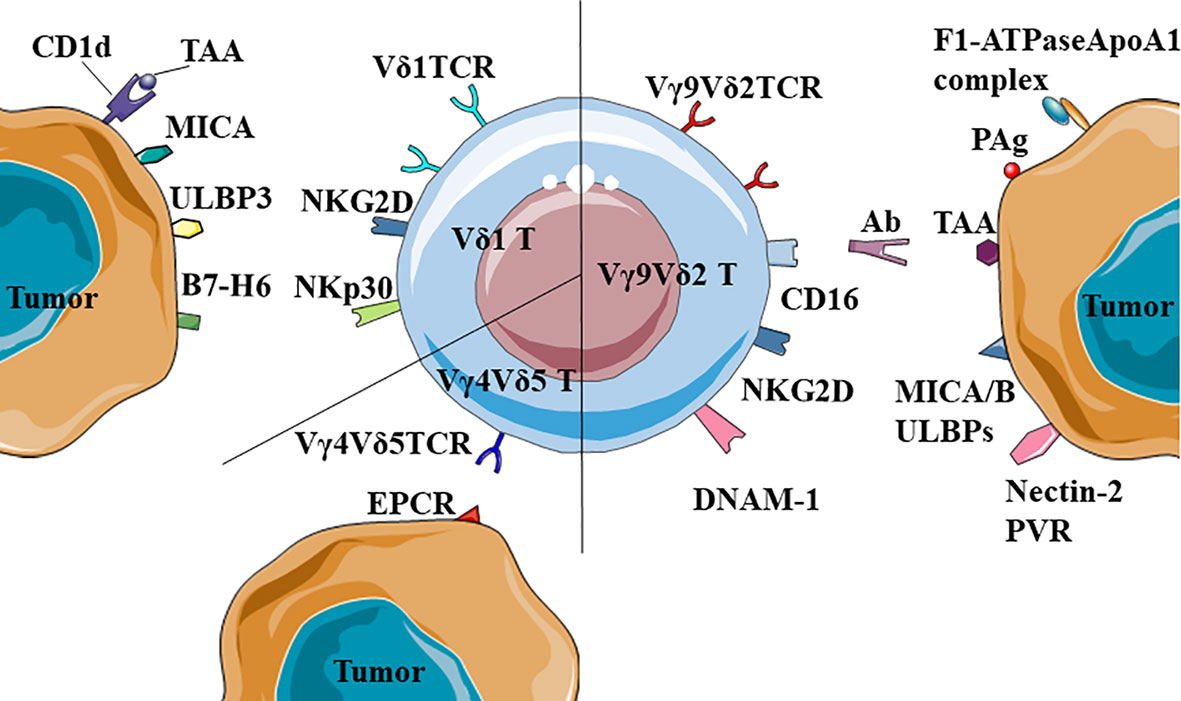
Figure 1 Recognition of tumor-associated antigens (TAA) by different γδ T cells. Vδ1TCR could recognize MICA or the complexes of CD1d and TAA; NKR+ (NKG2D and NKp30) Vδ1 T cells could recognize ULBP3 and B7-H6. Besides, Vγ9Vδ2 T cells could also recognize and kill tumors by CD16-mediated antibody-dependent cell-mediated cytotoxicity (ADCC). Notably, Vγ4Vδ5TCR could recognize the antigen of epithelial tumor cells, EPCR.
Anti-Tumor Mechanism of γδ T Cells
First, γδ T cells could kill tumor cells directly through secreting perforin and granzyme B (82). γδ T cells recognize tumor cells and release perforin and granzyme B into the synaptic space. They could further activate caspases to break DNA of tumor cells and lead to tumor cell death (102–105). γδ T cells could kill the human squamous cell carcinoma through perforin and granzyme B (106). Perforin and granzyme B inhibitor significantly reduce the ability of Vγ9Vδ2 T cells to lyse breast cancer cells in vitro (107). Moreover, in patients with renal carcinoma, activated Vγ9Vδ2 T cells showed a strong cytotoxicity to autologous tumor cells through perforin and granzyme B (108).
Second, γδ T cells kill tumor cells through ADCC. The Fab and Fc segment of antibody could bind to the TAA and γδ T cells, respectively. Then γδ T cells are activated to kill the tumor cells. Upon interaction with tumor cells, the expression of CD16 (FcγRIIIA) could be up-regulated on γδ T cells to induce tumor death through ADCC (82, 109, 110). In chronic lymphocytic leukemia and breast cancer patients, the cytotoxicity of Vγ9Vδ2 T cells is significantly enhanced after treatment with monoclonal antibodies including rituximab, trastuzumab and alemtuzumab (111–113).
Third, γδ T cells kill tumors through the Fas/FasL pathway and TRAIL (106). FasL expressed on γδ T cells could bind to Fas, and formed Fas trimer, which lead to the binding of the death effector domain (DED) to Fas-associated death domain–containing protein (FADD), and then activate caspases to induced the apoptosis of target cells (114–116). Similar to Fas/FasL, TRAIL also activates caspases through FADD, and then leads to apoptosis of tumor cells (117–124). In addition, IFN-γ could enhance the cytotoxicity of γδ T cells by up-regulating the expression of Fas on osteosarcoma cells (125, 126).
Similar to Vγ9Vδ2 T cells, Vδ1 T cells could kill tumor cells through the perforin-granzyme B, Fas/FasL and TRAIL pathway (49, 50, 98, 101). For example, human skin Vδ1 T cells could secrete perforin to kill melanoma cells (127). Granzyme B+ Vδ1 T cells and TRAIL+ Vδ1 T cells showed strong cytotoxicity to lymphoma cells and chronic lymphocytic leukemia (128–130). Beyond that, ex vivo expanded Vδ1 T cells highly express FasL, and have strong cytotoxicity on colon cancer cells (131).
γδ T Cells Enhance the Anti-Tumor Ability of Other Immune Cells
γδ T cells share similar functions as antigen presenting cells (APC), which could activate CD8+T cells (132, 133). When co-cultured with chronic myeloid leukemia (CML) cell lysates, the expression of co-stimulatory molecules (CD40, CD80 and CD86) and antigen-presenting molecule HLA-DR on Vγ9Vδ2 T cells could be strongly up-regulated. When these γδ T cells were co-cultured with CD8+ T cells, the proliferation rate of CD8+ T cells became 3 times faster than that of the control group (134, 135). Tumor cell fragments activate MAPK signaling pathways through Vγ9Vδ2TCR, up-regulate the expression of scavenger receptor CD36, enhance antigen uptake and processing of Vγ9Vδ2 T cells, and then induced tumor antigen-specific CD8+T cell response (136). Furthermore, γδ T cells toned to interact with cell surface-bound antibodies to acquire the ability of APC (137).
In addition, activated γδ T cells could secrete IFN-γ, which stimulates CSC to up-regulate the expression of MHC I molecules and CD54, and enhance the killing effect of CD8+T cells on tumor cells (138). Activated γδ T cells could also express CD137L to stimulate NK cells that upon proliferation exhibit strong anti-tumor activity through cell-to-cell contact (31).
The interaction between γδ T cells and dendritic cells (DC) is mutual. γδ T cells promote the maturation of DC, and mature DC induces the activation and proliferation of γδ T cells, which yield enhanced anti-tumor effect (139, 140). For example, activated Vγ9Vδ2 T cells could secrete IFN-γ and TNF-α to promote DC maturation and increase the expression of CD86 and MHC-I molecules on DC (141, 142). Mature DC could activate γδ T cells through presenting IPP, which synergizes with ATP-binding cassette transporter A1 (ABCA1), ApoA1 and BTN3A1 (143) (Figure 2).
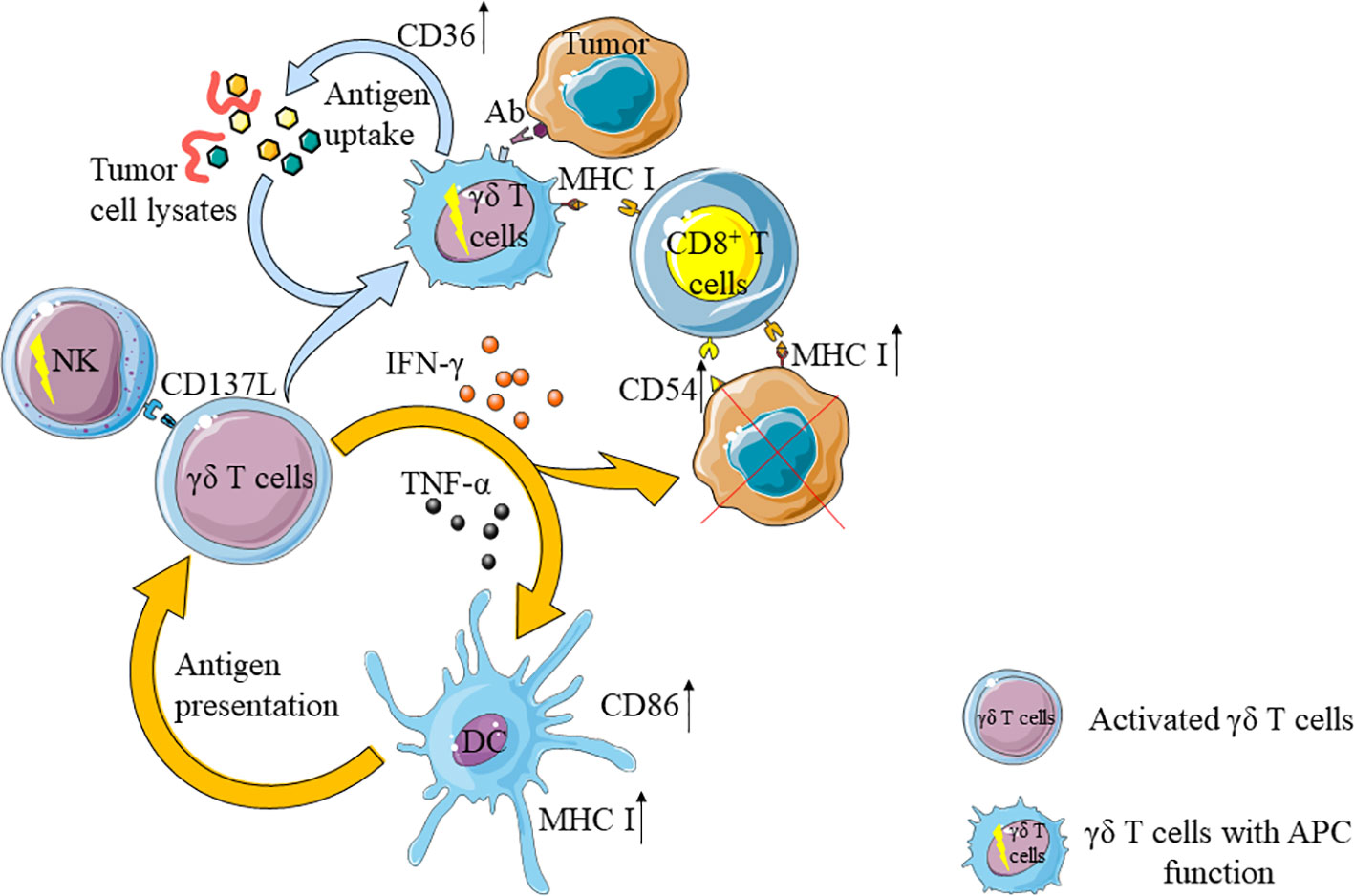
Figure 2 γδ T cells enhance the anti-tumor ability of other immune cells. On the one hand, activated γδ T cells can activate natural killer cells (NK) and dendritic cells (DC), activated DC can further activate γδ T cells. On the other hand, activated γδ T cells can up-regulate the expression of CD36 and enhance their antigen uptake ability after uptake of tumor cell lysates. At the same time, through contact with tumor cells with antibodies, the ability of antigen-presenting cells (APC) is obtained and CD8+T cells are activated. In addition, IFN- γ secreted by γδ T cells can up-regulate the expression of CD54 and MHC I molecules in tumor cells, and further enhance the anti-tumor effect of CD8+T cells.
Tumor-Infiltrating γδ T Cells Promote Tumor Development by Secreting IL-17
Interestingly, patients with increased number of tumor-infiltrating γδ T cells have higher recurrence rates and likelihood of metastasis (144–146). Among the tumor-infiltrating γδ T cells, Vδ1 T cells are present as the main population and secrete IL-17 to promote tumor development. IL-17 can promote the proliferation of tumor cells by activating IL-6/STAT3 and NF-κB pathways. In addition, it can also stimulate tumor cells to secrete vascular endothelial growth factor (VEGF) and matrix metalloproteinases (MMP) to further help tumor metastasis. High levels of IL-17 have been found in patients with advanced tumor or metastasized tumors (64, 147, 148). For example, in patients with solid tumors, Vδ1 T cells account for a large proportion of tumor-infiltrating γδ T cells; unlike Vδ1 T cells in adjacent non-tumor tissues, tumor-infiltrating γδ T cells do not express granzyme B, perforin, IFN-γ, FasL, TRAIL and NKR, but secrete IL-17 (149–154). Majority of the tumor-infiltrating Vδ1 T cells were TEM phenotype, while most of the Vδ1 T cells in healthy subjects were TCM phenotype (64). Similarly and compared with healthy people, cancer patients have a larger proportion of Vδ1 T cells and higher IL-17 levels in their peripheral blood (155, 156).
Tumor-Infiltrating γδ T Cells Inhibit the Anti-Tumor Function of Other Immune Cells
IL-17, secreted by tumor-infiltrating Vδ1 T cells not only acts on tumor cells directly, but can also recruit myeloid-derived suppressor cells (MDSC) to tumor (147, 148, 150). MDSC inhibits the activation of CD8+ T cells by expressing high levels of ARG1, which decomposes arginine in the tumor microenvironment (157–160).
In addition, tumor-infiltrating γδ T cells could significantly inhibit the maturation of CD4+ T cells (155). Studies in breast cancer settings showed that tumor-infiltrating γδ T cells could inhibit the maturation of CD4+ or CD8+ T cells and change their functions by arresting cell cycle in G0/G1 phase and increasing the expression of p53, P21, and P16. Through secreting IL-10 and TGF- β1, these suppressed T cells further amplified the inhibitory effect (161, 162). Beyond that, these cells express high levels of PD-L1 to promote the apoptosis of CD8+ T cells in cancer patients (41).
Vδ1 T cells could also inhibit the maturation of DC, reduce the expression of CD80/86 and HLA-DR on DC, attenuate the secretion of pro-inflammatory cytokines TNF- α, and up-regulate the expression of PD-L1 on the surface of DC (161, 163) (Figure 3).
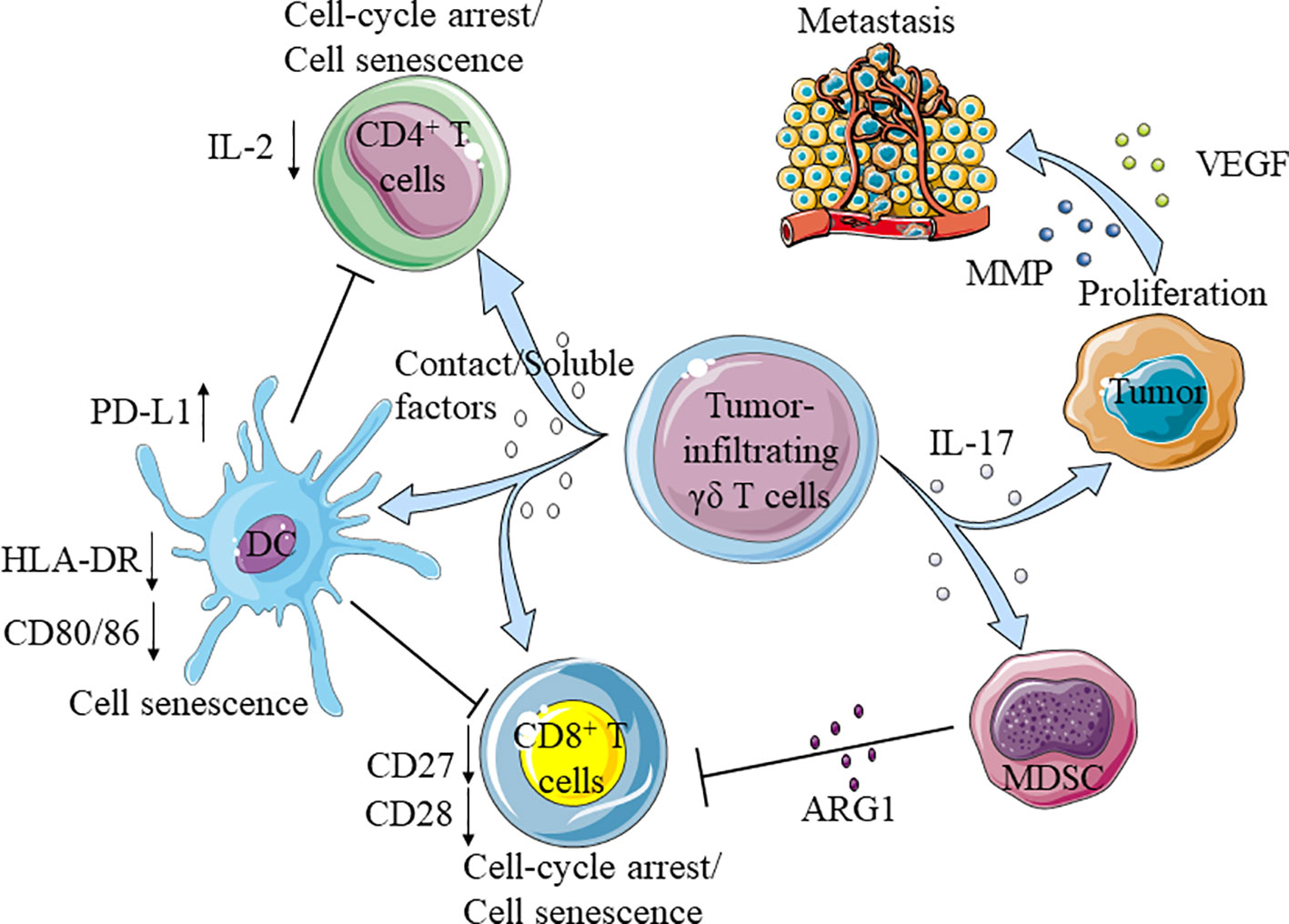
Figure 3 Tumor-infiltrating γδ T cells promote the development of tumor. Tumor-infiltrating γδ T cells secrete IL-17 promote the proliferation and metastasis of tumor cells, and recruit myeloid-derived suppressor cells (MDSC) to inhibit the function of CD8+T cells. Moreover, tumor-infiltrating γδ T cells directly impaired the function of CD4+/CD8+T cells and dendritic cells (DC), the aging DC further inhibited CD4+/CD8+T cells.
Anti-Tumor Immunotherapy With γδ T Cells
The unique antigen recognition mechanism of γδ T cells renders them the ability to kill various types of tumors. Therefore, γδ T cell-based therapies have been widely used in clinical as anti-tumor immunotherapies and achieved good results (Table 2). At present, the most routine method in these therapies is to activate the anti-tumor activity of natural γδ T cells and the Vγ9Vδ2 T cells, which as the most abundant subtype in peripheral blood are often selected and utilized through transferring back to cancer patient after stimulation in vitro or direct activation in vivo.
The most widely used stimulants for expanding Vγ9Vδ2 T cells in vitro are zoledronic acid (ZOL) and IL-2. As a kind of bisphosphate, ZOL could specifically inhibit farnesyl pyrophosphate synthase (FPPS) in isoprene biosynthesis pathway, thus causing the accumulation of endogenous PAg in cells and promoting the activation of Vγ9Vδ2 T cells (65). This method could effectively expand and activate Vγ9Vδ2 T cells from patients or healthy people in vitro (52). In addition, another kind of PAg, 2-methyl-3-butenyl-1-pyrophosphate(2M3B1-PP) could also effectively stimulate and expand Vγ9Vδ2 T cells (164, 165). The activated immune cells are transferred back into the patients to produce anti-tumor effects. In order to track the activated Vγ9Vδ2 T cells, they are typically labeled with indium111. Studies have confirmed that these cells mainly metastasize to the lung, liver and spleen, as well as to the tumor sites (166). In the treatment of patients with multiple myeloma, the stimulated Vγ9Vδ2 T cells expressed high levels of NKG2D and IFN-γ, but not IL-17. After treatment, the number of Vγ9Vδ2 T cells in the tumor microenvironment increased, lasting as long as 4 weeks (167). In patients with renal cell carcinoma and non-small cell lung cancer, repeated injections of IL-2 has demonstrated good safety (168), enhanced the cytotoxicity of Vγ9Vδ2 T cells. As results, the deterioration of tumor was alleviated with patients’ condition stabilized, and the survival time was pro-longed (164, 165, 169, 170). In the clinical study of malignant ascites, after transferring back the activated Vγ9Vδ2 T cells, the number of tumor cells in ascites decreased significantly and the level of IFN-γ in ascites increased. During the course of treatment, there were no significant adverse effects and the amounts of ascites decreased significantly (171). In addition to the direct anti-tumor effect of Vγ9Vδ2 T cells, the numbers of CD4+ T and CD8+ T cells could also get increased after the allogeneic Vγ9Vδ2 T cells were transferred into the patients, as shown in another study of cholangio-carcinoma (52).
Vγ9Vδ2 T cells could also be activated in vivo. Injection of ZOL and IL-2 could directly activate these cells in cancer patients. Serval clinical trials have demonstrated that, upon injection and activation of Vγ9Vδ2 T cells in vivo, IFN-γ was strongly induced and the deterioration of the tumor was controlled (53, 172–175). Besides ZOL, Vγ9Vδ2 T cells could also be activated by synthetic PAg or bromohydrin pyrophosphate (BrHPP, IPH1101) with good safety tolerability in patients (176).
Optimization of γδ T Cell-Based Immunotherapy
In clinic, repeated use of ZOL and IL-2 carry the liability in inhibiting the proliferation of Vγ9Vδ2 T cells (172), which could be alleviated by vitamin C (VC) and L-ascorbic acid 2-phosphate (pVC). VC has the ability to reduce the apoptosis of γδ T cells during stimulation, and pVC may enhance the expansion of γδ T cells. Therefore, VC and pVC have been utilized to improve the efficacy of the γδ T cells in anti-tumor immunotherapy (177). In addition, cytotoxicity of Vγ9Vδ2 T cells could be improved by adding IL-21, IL-15, or IL-33 in vitro (55, 178–182). Anti-cancer drug Gemcitabine or anti-epileptic drug Valproic acid (VPA) in combination with ZOL could also enhance the anti-tumor ability of γδ T cells (183, 184).
In recent years, chitosan nanoparticles (CSNPs) and antibodies have been developed as potential anti-tumor therapies. CSNPs have been shown to regulate γδ T cells by up-regulating the expression of NKG2D, CD56 and FasL, and enhancing their anti-tumor functions (185). TIM-3 could also inhibit the killing effect of Vγ9Vδ2 T cells on tumor by reducing the expression of perforin and granzyme B. PD-1 and TIM-3 antibodies could protect anti-tumor activity of Vγ9Vδ2 T cells (186–188). Beyond these, the application of bispecific antibodies can also promote γδ T cells to inhibit tumor development. For example, in the study of hepatoblastoma and pediatric hepatocellular carcinoma, the application of EpCAM/CD3-bispecific BiTE antibody (MT110) enhanced the anti-tumor ability of γδ T cells; similarly, in epithelial ovarian cancer and pancreatic ductal adenocarcinoma, bispecific antibody [HER2xCD3] and [(HER2)2xVγ9] (tribody format) could also effectively enhance the cytotoxicity of γδ T cells (189–193).
Finally, chimeric antigen receptor engineered γδ T (CAR-γδ T) technology is another new direction in immunotherapy. CAR- γδ T cells could target GD2, a TAA on the surface of neuroblastoma cells, and effectively kill tumors. This kind of CAR-γδ T cells need to recognize GD2 to become activated. Such mechanism ensures the specificity of these cells in killing tumor cells and offering lower toxicities and side effects (194, 195). On the hand, Vγ9Vδ2TCR could also be transduced into αβ T cells. These CAR-T cells are called TEGs, which carry not only the extensive recognition ability of γδ T cells but also and the memory ability of αβ T cells (196–199).
Summary
Taken together, we described in this review that Vδ1 T cells and Vγ9Vδ2 T cells are the two most important subgroups of human γδ T cells. Peripheral Vδ1 T cells and Vγ9Vδ2 T cells could recognize tumor cells through TCRγδ and NKR, and kill them through perforin-granzyme B, Fas/FasL and TRAIL. Activated Vγ9Vδ2 T cells could perform the function of APC, and furthermore, they could activate NK cells and DC directly. On the contrary, tumor-infiltrating Vδ1 T cells promoted tumor development by secreting IL-17 and inhibiting the maturation of CD4+/CD8+ T cells and DC. In immunotherapy, ZOL, 2M3B1-PP or IPH1101 has been commonly used to activate Vγ9Vδ2 T cells to achieve anti-tumor effect. The failure caused by repeated application of this method can be solved by adding VC or replacing cytokines. In addition, new classes of drugs such as CSNPs, were also applied to γδ T cell-based anti-tumor immunotherapy.
It is noteworthy to mention that although Vδ1 T cells account for the majority of tumor-infiltrating γδ T cells, the definition of γδ T cell subsets still rely on their profile in cytokine production (32, 64, 149). Secondly, the interaction mechanism between γδ T cells and the environment or other immune cells remains to be further elucidated. For example, Vγ9Vδ2 T cells could ingest LDL-cholesterol upon activation and lead to reduced function, suggesting that obesity may inhibit the anti-tumor activity of γδ T cells (200). Another myth exists in how exactly soluble molecules mediate the inhibition of γδ T cells in tumor microenvironment (161, 163). In addition, γδ T cell-based immunotherapy needs to be further optimized, with emphasis on how to carry out personalized therapy according to the actual situation of individual patient.
In summary, a more comprehensive understanding of the biological characteristics of γδ T cells, an important group of lymphocytes, will guide the improvement of their clinical application methods and provide new strategies to fight against human cancers.
Author Contributions
JZ completed the writing of the classification of γδ T cells. XC and XW completed the writing of introduction and summary. YL and GL completed the writing of the rest of this review. All authors contributed to the article and approved the submitted version.
Funding
Funding support from China Postdoctoral Science Foundation (2018M641666).
Conflict of Interest
The authors declare that the research was conducted in the absence of any commercial or financial relationships that could be construed as a potential conflict of interest.
References
1. Carding SR, Egan PJ. Gammadelta T cells: functional plasticity and heterogeneity. Nat Rev Immunol (2002) 2(5):336–45. doi: 10.1038/nri797
2. Chien YH, Meyer C, Bonneville M. γδ T cells: first line of defense and beyond. Annu Rev Immunol (2014) 32:121–55. doi: 10.1146/annurev-immunol-032713-120216
3. Poggi A, Zocchi MR. γδ T Lymphocytes as a First Line of Immune Defense: Old and New Ways of Antigen Recognition and Implications for Cancer Immunotherapy. Front Immunol (2014) 5:575. doi: 10.3389/fimmu.2014.00575
4. Holtmeier W, Kabelitz D. gammadelta T cells link innate and adaptive immune responses. Chem Immunol Allergy (2005) 86:151–83. doi: 10.1159/000086659
5. Bonneville M, O’Brien RL, Born WK. Gammadelta T cell effector functions: a blend of innate programming and acquired plasticity. Nat Rev Immunol (2010) 10(7):467–78. doi: 10.1038/nri2781
6. Chitadze G, Oberg HH, Wesch D, Kabelitz D. The Ambiguous Role of γδ T Lymphocytes in Antitumor Immunity. Trends Immunol (2017) 38(9):668–78. doi: 10.1016/j.it.2017.06.004
7. Correia DV, Lopes A, Silva-Santos B. Tumor cell recognition by γδ T lymphocytes: T-cell receptor vs. NK-cell receptors. Oncoimmunology (2013) 2(1):e22892. doi: 10.4161/onci.22892
8. Sanders JM, Ghosh S, Chan JM, Meints G, Wang H, Raker AM, et al. Quantitative structure-activity relationships for gammadelta T cell activation by bisphosphonates. J Med Chem (2004) 47(2):375–84. doi: 10.1021/jm0303709
9. Wei H, Huang D, Lai X, Chen M, Zhong W, Wang R, et al. Definition of APC presentation of phosphoantigen (E)-4-hydroxy-3-methyl-but-2-enyl pyrophosphate to Vgamma2Vdelta 2 TCR. J Immunol (2008) 181(7):4798–806. doi: 10.4049/jimmunol.181.7.4798
10. Wang H, Sarikonda G, Puan KJ, Tanaka Y, Feng J, Giner JL, et al. Indirect stimulation of human Vγ2Vδ2 T cells through alterations in isoprenoid metabolism. J Immunol (2011) 187(10):5099–113. doi: 10.4049/jimmunol.1002697
11. Puan KJ, Jin C, Wang H, Sarikonda G, Raker AM, Lee HK, et al. Preferential recognition of a microbial metabolite by human Vgamma2Vdelta2 T cells. Int Immunol (2007) 19(5):657–73. doi: 10.1093/intimm/dxm031
12. Kondo M, Izumi T, Fujieda N, Kondo A, Morishita T, Matsushita H, et al. Expansion of human peripheral blood γδ T cells using zoledronate. J Vis Exp (2011) 55):3182. doi: 10.3791/3182
13. Scotet E, Martinez LO, Grant E, Barbaras R, Jenö P, Guiraud M, et al. Tumor recognition following Vgamma9Vdelta2 T cell receptor interactions with a surface F1-ATPase-related structure and apolipoprotein A-I. Immunity (2005) 22(1):71–80. doi: 10.1016/j.immuni.2004.11.012
14. Kong Y, Cao W, Xi X, Ma C, Cui L, He W. The NKG2D ligand ULBP4 binds to TCRgamma9/delta2 and induces cytotoxicity to tumor cells through both TCRgammadelta and NKG2D. Blood (2009) 114(2):310–7. doi: 10.1182/blood-2008-12-196287
15. Peipp M, Wesch D, Oberg HH, Lutz S, Muskulus A, van de Winkel JGJ, et al. CD20-Specific Immunoligands Engaging NKG2D Enhance γδ T Cell-Mediated Lysis of Lymphoma Cells. Scand J Immunol (2017) 86(4):196–206. doi: 10.1111/sji.12581
16. Gundermann S, Klinker E, Kimmel B, Flierl U, Wilhelm M, Einsele H, et al. A comprehensive analysis of primary acute myeloid leukemia identifies biomarkers predicting susceptibility to human allogeneic Vγ9Vδ2 T cells. J Immunother (2014) 37(6):321–30. doi: 10.1097/CJI.0000000000000043
17. Lu J, Aggarwal R, Kanji S, Das M, Joseph M, Pompili V, et al. Human ovarian tumor cells escape γδ T cell recognition partly by down regulating surface expression of MICA and limiting cell cycle related molecules. PloS One (2011) 6(9):e23348. doi: 10.1371/journal.pone.0023348
18. Knight A, Arnouk H, Britt W, Gillespie GY, Cloud GA, Harkins L, et al. CMV-independent lysis of glioblastoma by ex vivo expanded/activated Vδ1+ γδ T cells. PloS One (2013) 8(8):e68729. doi: 10.1371/journal.pone.0068729
19. Willcox CR, Pitard V, Netzer S, Couzi L, Salim M, Silberzahn T, et al. Cytomegalovirus and tumor stress surveillance by binding of a human γδ T cell antigen receptor to endothelial protein C receptor. Nat Immunol (2012) 13(9):872–9. doi: 10.1038/ni.2394
20. Witherden DA, Havran WL. EPCR: a stress trigger for γδ T cells. Nat Immunol (2012) 13(9):812–4. doi: 10.1038/ni.2398
21. Zhang H, Hu H, Jiang X, He H, Cui L, He W. Membrane HSP70: the molecule triggering gammadelta T cells in the early stage of tumorigenesis. Immunol Invest (2005) 34(4):453–68. doi: 10.1080/08820130500265349
22. Thomas ML, Samant UC, Deshpande RK, Chiplunkar SV. gammadelta T cells lyse autologous and allogenic oesophageal tumours: involvement of heat-shock proteins in the tumour cell lysis. Cancer Immunol Immunother (2000) 48(11):653–9. doi: 10.1007/s002620050014
23. Laad AD, Thomas ML, Fakih AR, Chiplunkar SV. Human gamma delta T cells recognize heat shock protein-60 on oral tumor cells. Int J Cancer (1999) 80(5):709–14. doi: 10.1002/(sici)1097-0215(19990301)80:5<709::aid-ijc14>3.0.co;2-r
24. Mo C, Dai Y, Kang N, Cui L, He W. Ectopic expression of human MutS homologue 2 on renal carcinoma cells is induced by oxidative stress with interleukin-18 promotion via p38 mitogen-activated protein kinase (MAPK) and c-Jun N-terminal kinase (JNK) signaling pathways. J Biol Chem (2012) 287(23):19242–54. doi: 10.1074/jbc.M112.349936\
25. Dai Y, Chen H, Mo C, Cui L, He W. Ectopically expressed human tumor biomarker MutS homologue 2 is a novel endogenous ligand that is recognized by human γδ T cells to induce innate anti-tumor/virus immunity. J Biol Chem (2012) 287(20):16812–9. doi: 10.1074/jbc.M111.327650
26. Chen H, He X, Wang Z, Wu D, Zhang H, Xu C, et al. Identification of human T cell receptor gammadelta-recognized epitopes/proteins via CDR3delta peptide-based immunobiochemical strategy. J Biol Chem (2008) 283(18):12528–37. doi: 10.1074/jbc.M708067200
27. Meraviglia S, Caccamo N, Guggino G, Tolomeo M, Siragusa S, Stassi G, et al. Optimizing tumor-reactive γδ T cells for antibody-based cancer immunotherapy. Curr Mol Med (2010) 10(8):719–26. doi: 10.2174/156652410793384150
28. Hoeres T, Smetak M, Pretscher D, Wilhelm M. Improving the Efficiency of Vγ9Vδ2 T-Cell Immunotherapy in Cancer. Front Immunol (2018) 9:800. doi: 10.3389/fimmu.2018.00800
29. Chen J, Fan Y, Cui B, Li X, Yu Y, Du Y, et al. HMGN2: An Antitumor Effector Molecule of γδT Cells. J Immunother (2018) 41(3):118–24. doi: 10.1097/CJI.0000000000000211
30. Iovino F, Meraviglia S, Spina M, Orlando V, Saladino V, Dieli F, et al. Immunotherapy targeting colon cancer stem cells. Immunotherapy (2011) 3(1):97–106. doi: 10.2217/imt.10.87
31. Maniar A, Zhang X, Lin W, Gastman BR, Pauza CD, Strome SE, et al. Human gammadelta T lymphocytes induce robust NK cell-mediated antitumor cytotoxicity through CD137 engagement. Blood (2010) 116(10):1726–33. doi: 10.1182/blood-2009-07-234211
32. Silva-Santos B, Mensurado S, Coffelt SB. γδ T cells: pleiotropic immune effectors with therapeutic potential in cancer. Nat Rev Cancer (2019) 19(7):392–404. doi: 10.1038/s41568-019-0153-5
33. Sebestyen Z, Prinz I, Déchanet-Merville J, Silva-Santos B, Kuball J. Translating gammadelta (γδ) T cells and their receptors into cancer cell therapies. Nat Rev Drug Discovery (2020) 19(3):169–84. doi: 10.1038/s41573-019-0038-z
34. Kakimi K, Matsushita H, Murakawa T, Nakajima J. γδ T cell therapy for the treatment of non-small cell lung cancer. Transl Lung Cancer Res (2014) 3(1):23–33. doi: 10.3978/j.issn.2218-6751.2013.11.01
35. Wu Y, Kyle-Cezar F, Woolf RT, Naceur-Lombardelli C, Owen J, Biswas D, et al. An innate-like Vδ1+ γδ T cell compartment in the human breast is associated with remission in triple-negative breast cancer. Sci Transl Med (2019) 11(513):eaax9364. doi: 10.1126/scitranslmed.aax9364
36. Rei M, Pennington DJ, Silva-Santos B. The emerging Protumor role of γδ T lymphocytes: implications for cancer immunotherapy. Cancer Res (2015) 75(5):798–802. doi: 10.1158/0008-5472.CAN-14-3228
37. Wu X, Yang T, Liu X, Guo JN, Xie T, Ding Y, et al. IL-17 promotes tumor angiogenesis through Stat3 pathway mediated upregulation of VEGF in gastric cancer. Tumour Biol (2016) 37(4):5493–501. doi: 10.1007/s13277-015-4372-4
38. Pan B, Shen J, Cao J, Zhou Y, Shang L, Jin S, et al. Interleukin-17 promotes angiogenesis by stimulating VEGF production of cancer cells via the STAT3/GIV signaling pathway in non-small-cell lung cancer. Sci Rep (2015) 5:16053. doi: 10.1038/srep16053
39. Yan J, Huang J. Innate γδT17 cells convert cancer-elicited inflammation into immunosuppression through myeloid-derived suppressor cells. Oncoimmunology (2014) 3(8):e953423. doi: 10.4161/21624011.2014.953423
40. Qu P, Wang LZ, Lin PC. Expansion and functions of myeloid-derived suppressor cells in the tumor microenvironment. Cancer Lett (2016) 380(1):253–6. doi: 10.1016/j.canlet.2015.10.022
41. Daley D, Zambirinis CP, Seifert L, Akkad N, Mohan N, Werba G, et al. γδ T Cells Support Pancreatic Oncogenesis by Restraining αβ T Cell Activation. Cell (2016) 166(6):1485–99.e15. doi: 10.1016/j.cell.2016.07.046
42. Lafont V, Sanchez F, Laprevotte E, Michaud HA, Gros L, Eliaou JF, et al. Plasticity of γδ T Cells: Impact on the Anti-Tumor Response. Front Immunol (2014) 5:622. doi: 10.3389/fimmu.2014.00622
43. Lee HW, Chung YS, Kim TJ. Heterogeneity of Human γδ T Cells and Their Role in Cancer Immunity. Immune Netw (2020) 20(1):e5. doi: 10.4110/in.2020.20.e5
44. Pang DJ, Neves JF, Sumaria N, Pennington DJ. Understanding the complexity of γδ T-cell subsets in mouse and human. Immunology (2012) 136(3):283–90. doi: 10.1111/j.1365-2567.2012.03582.x
45. Adams EJ, Gu S, Luoma AM. Human gamma delta T cells: Evolution and ligand recognition. Cell Immunol (2015) 296(1):31–40. doi: 10.1016/j.cellimm.2015.04.008
46. Wu D, Wu P, Qiu F, Wei Q, Huang J. Human γδT-cell subsets and their involvement in tumor immunity. Cell Mol Immunol (2017) 14(3):245–53. doi: 10.1038/cmi.2016.55
47. Fichtner AS, Ravens S, Prinz I. Human γδ TCR Repertoires in Health and Disease. Cells (2020) 9(4):800. doi: 10.3390/cells9040800
48. Mikulak J, Oriolo F, Bruni E, Roberto A, Colombo FS, Villa A, et al. NKp46-expressing human gut-resident intraepithelial Vδ1 T cell subpopulation exhibits high antitumor activity against colorectal cancer. JCI Insight (2019) 4(24):e125884. doi: 10.1172/jci.insight.125884
49. Knight A, Mackinnon S, Lowdell MW. Human Vdelta1 gamma-delta T cells exert potent specific cytotoxicity against primary multiple myeloma cells. Cytotherapy (2012) 14(9):1110–8. doi: 10.3109/14653249.2012.700766
50. Siegers GM, Dhamko H, Wang XH, Mathieson AM, Kosaka Y, Felizardo TC, et al. Human Vδ1 γδ T cells expanded from peripheral blood exhibit specific cytotoxicity against B-cell chronic lymphocytic leukemia-derived cells. Cytotherapy (2011) 13(6):753–64. doi: 10.3109/14653249.2011.553595
51. Kühl AA, Pawlowski NN, Grollich K, Blessenohl M, Westermann J, Zeitz M, et al. Human peripheral gammadelta T cells possess regulatory potential. Immunology (2009) 128(4):580–8. doi: 10.1111/j.1365-2567.2009.03162.x
52. Alnaggar M, Xu Y, Li J, He J, Chen J, Li M, et al. Allogenic Vγ9Vδ2 T cell as new potential immunotherapy drug for solid tumor: a case study for cholangiocarcinoma. J Immunother Cancer (2019) 7(1):36. doi: 10.1186/s40425-019-0501-8
53. Meraviglia S, Eberl M, Vermijlen D, Todaro M, Buccheri S, Cicero G, et al. In vivo manipulation of Vgamma9Vdelta2 T cells with zoledronate and low-dose interleukin-2 for immunotherapy of advanced breast cancer patients. Clin Exp Immunol (2010) 161(2):290–7. doi: 10.1111/j.1365-2249.2010.04167.x
54. Di Carlo E, Bocca P, Emionite L, Cilli M, Cipollone G, Morandi F, et al. Mechanisms of the antitumor activity of human Vγ9Vδ2 T cells in combination with zoledronic acid in a preclinical model of neuroblastoma. Mol Ther (2013) 21(5):1034–43. doi: 10.1038/mt.2013.38
55. Joalland N, Chauvin C, Oliver L, Vallette FM, Pecqueur C, Jarry U, et al. IL-21 Increases the Reactivity of Allogeneic Human Vγ9Vδ2 T Cells Against Primary Glioblastoma Tumors. J Immunother (2018) 41(5):224–31. doi: 10.1097/CJI.0000000000000225
56. Lai D, Wang F, Chen Y, Wang C, Liu S, Lu B, et al. Human ovarian cancer stem-like cells can be efficiently killed by γδ T lymphocytes. Cancer Immunol Immunother (2012) 61(7):979–89. doi: 10.1007/s00262-011-1166-4
57. Nishio N, Fujita M, Tanaka Y, Maki H, Zhang R, Hirosawa T, et al. Zoledronate sensitizes neuroblastoma-derived tumor-initiating cells to cytolysis mediated by human γδ T cells. J Immunother (2012) 35(8):598–606. doi: 10.1097/CJI.0b013e31826a745a
58. Bartkowiak J, Kulczyck-Wojdala D, Blonski JZ, Robak T. Molecular diversity of gammadelta T cells in peripheral blood from patients with B-cell chronic lymphocytic leukaemia. Neoplasma (2002) 49(2):86–90. doi: 10.1038/sj.neo.7900211
59. Kenna T, Golden-Mason L, Norris S, Hegarty JE, O’Farrelly C, Doherty DG. Distinct subpopulations of gamma delta T cells are present in normal and tumor-bearing human liver. Clin Immunol (2004) 113(1):56–63. doi: 10.1016/j.clim.2004.05.003
60. Petrasca A, Melo AM, Breen EP, Doherty DG. Human Vδ3+ γδ T cells induce maturation and IgM secretion by B cells. Immunol Lett (2018) 196:126–34. doi: 10.1016/j.imlet.2018.02.002
61. Mangan BA, Dunne MR, O’Reilly VP, Dunne PJ, Exley MA, O'Shea D, et al. Cutting edge: CD1d restriction and Th1/Th2/Th17 cytokine secretion by human Vδ3 T cells. J Immunol (2013) 191(1):30–4. doi: 10.4049/jimmunol.1300121
62. Zhao Y, Niu C, Cui J. Gamma-delta (γδ) T cells: friend or foe in cancer development? J Transl Med (2018) 16(1):3. doi: 10.1186/s12967-017-1378-2
63. Qin G, Liu Y, Zheng J, Xiang Z, Ng IH, Malik Peiris JS, et al. Phenotypic and functional characterization of human γδ T-cell subsets in response to influenza A viruses. J Infect Dis (2012) 205(11):1646–53. doi: 10.1093/infdis/jis253
64. Lo Presti E, Toia F, Oieni S, Buccheri S, Turdo A, Mangiapane LR, et al. Squamous Cell Tumors Recruit γδ T Cells Producing either IL17 or IFNγ Depending on the Tumor Stage. Cancer Immunol Res (2017) 5(5):397–407. doi: 10.1158/2326-6066.CIR-16-0348
65. Gober HJ, Kistowska M, Angman L, Jenö P, Mori L, De Libero G. Human T cell receptor gammadelta cells recognize endogenous mevalonate metabolites in tumor cells. J Exp Med (2003) 197(2):163–8. doi: 10.1084/jem.20021500
66. Silva-Santos B, Serre K, Norell H. γδ T cells in cancer. Nat Rev Immunol (2015) 15(11):683–91. doi: 10.1038/nri3904
67. Mullen PJ, Yu R, Longo J, Archer MC, Penn LZ. The interplay between cell signalling and the mevalonate pathway in cancer. Nat Rev Cancer (2016) 16(11):718–31. doi: 10.1038/nrc.2016.76
68. Moulin M, Alguacil J, Gu S, Mehtougui A, Adams EJ, Peyrottes S, et al. Vγ9Vδ2 T cell activation by strongly agonistic nucleotidic phosphoantigens. Cell Mol Life Sci (2017) 74(23):4353–67. doi: 10.1007/s00018-017-2583-0
69. Kabelitz D. CD277 takes the lead in human γδ T-cell activation. Blood (2012) 120(11):2159–61. doi: 10.1182/blood-2012-07-442731
70. Peigné CM, Léger A, Gesnel MC, Konczak F, Olive D, Bonneville M, et al. The Juxtamembrane Domain of Butyrophilin BTN3A1 Controls Phosphoantigen-Mediated Activation of Human Vγ9Vδ2 T Cells. J Immunol (2017) 198(11):4228–34. doi: 10.4049/jimmunol.1601910
71. Gu S, Sachleben JR, Boughter CT, Nawrocka WI, Borowska MT, Tarrasch JT, et al. Phosphoantigen-induced conformational change of butyrophilin 3A1 (BTN3A1) and its implication on Vγ9Vδ2 T cell activation. Proc Natl Acad Sci U.S.A. (2017) 114(35):E7311–20. doi: 10.1073/pnas.1707547114
72. Sandstrom A, Peigné CM, Léger A, Crooks JE, Konczak F, Gesnel MC, et al. The intracellular B30.2 domain of butyrophilin 3A1 binds phosphoantigens to mediate activation of human Vγ9Vδ2 T cells. Immunity (2014) 40(4):490–500. doi: 10.1016/j.immuni.2014.03.003
73. Wang H, Morita CT. Sensor Function for Butyrophilin 3A1 in Prenyl Pyrophosphate Stimulation of Human Vγ2Vδ2 T Cells. J Immunol (2015) 195(10):4583–94. doi: 10.4049/jimmunol.1500314
74. Gu S, Nawrocka W, Adams EJ. Sensing of Pyrophosphate Metabolites by Vγ9Vδ2 T Cells. Front Immunol (2015) 5:688. doi: 10.3389/fimmu.2014.00688
75. Nguyen K, Li J, Puthenveetil R, Lin X, Poe MM, Hsiao CC, et al. The butyrophilin 3A1 intracellular domain undergoes a conformational change involving the juxtamembrane region. FASEB J (2017) 31(11):4697–706. doi: 10.1096/fj.201601370RR
76. Vantourout P, Laing A, Woodward MJ, Zlatareva I, Apolonia L, Jones AW, et al. Heteromeric interactions regulate butyrophilin (BTN) and BTN-like molecules governing γδ T cell biology. Proc Natl Acad Sci U S A (2018) 115(5):1039–44. doi: 10.1073/pnas.1701237115
77. Yang Y, Li L, Yuan L, Zhou X, Duan J, Xiao H, et al. A Structural Change in Butyrophilin upon Phosphoantigen Binding Underlies Phosphoantigen-Mediated Vγ9Vδ2 T Cell Activation. Immunity (2019) 50(4):1043–1053.e5. doi: 10.1016/j.immuni.2019.02.016
78. Vavassori S, Kumar A, Wan GS, Ramanjaneyulu GS, Cavallari M, El Daker S, et al. Butyrophilin 3A1 binds phosphorylated antigens and stimulates human γδ T cells. Nat Immunol (2013) 14(9):908–16. doi: 10.1038/ni.2665
79. Karunakaran MM, Willcox CR, Salim M, Paletta D, Fichtner AS, Noll A, et al. Butyrophilin-2A1 Directly Binds Germline-Encoded Regions of the Vγ9Vδ2 TCR and Is Essential for Phosphoantigen Sensing. Immunity (2020) 52(3):487–498.e6. doi: 10.1016/j.immuni.2020.02.014
80. Rigau M, Ostrouska S, Fulford TS, Johnson DN, Woods K, Ruan Z, et al. Butyrophilin 2A1 is essential for phosphoantigen reactivity by γδ T cells. Science (2020) 367(6478):eaay5516. doi: 10.1126/science.aay5516
81. Mookerjee-Basu J, Vantourout P, Martinez LO, Perret B, Collet X, Périgaud C, et al. F1-adenosine triphosphatase displays properties characteristic of an antigen presentation molecule for Vgamma9Vdelta2 T cells. J Immunol (2010) 184(12):6920–8. doi: 10.4049/jimmunol.0904024
82. Dhar P, Wu JD. NKG2D and its ligands in cancer. Curr Opin Immunol (2018) 51:55–61. doi: 10.1016/j.coi.2018.02.004
83. Niu C, Jin H, Li M, Xu J, Xu D, Hu J, et al. In vitro analysis of the proliferative capacity and cytotoxic effects of ex vivo induced natural killer cells, cytokine-induced killer cells, and gamma-delta T cells. BMC Immunol (2015) 16:61. doi: 10.1186/s12865-015-0124-x
84. Corvaisier M, Moreau-Aubry A, Diez E, Bennouna J, Mosnier JF, Scotet E, et al. V gamma 9V delta 2 T cell response to colon carcinoma cells. J Immunol (2005) 175(8):5481–8. doi: 10.4049/jimmunol.175.8.5481
85. Wang Z, Wang Z, Li S, Li B, Sun L, Li H, et al. Decitabine Enhances Vγ9Vδ2 T Cell-Mediated Cytotoxic Effects on Osteosarcoma Cells via the NKG2DL-NKG2D Axis. Front Immunol (2018) 9:1239. doi: 10.3389/fimmu.2018.01239
86. Toutirais O, Cabillic F, Le Friec G, Salot S, Loyer P, Le Gallo M, et al. DNAX accessory molecule-1 (CD226) promotes human hepatocellular carcinoma cell lysis by Vgamma9Vdelta2 T cells. Eur J Immunol (2009) 39(5):1361–8. doi: 10.1002/eji.200838409
87. Gertner-Dardenne J, Castellano R, Mamessier E, Garbit S, Kochbati E, Etienne A, et al. Human Vγ9Vδ2 T cells specifically recognize and kill acute myeloid leukemic blasts. J Immunol (2012) 188(9):4701–8. doi: 10.4049/jimmunol.1103710
88. Deniger DC, Maiti SN, Mi T, Switzer KC, Ramachandran V, Hurton LV, et al. Activating and propagating polyclonal gamma delta T cells with broad specificity for malignancies. Clin Cancer Res (2014) 20(22):5708–19. doi: 10.1158/1078-0432.CCR-13-3451
89. Wang X, Mou W, Han W, Xi Y, Chen X, Zhang H, et al. Diminished cytolytic activity of γδ T cells with reduced DNAM-1 expression in neuroblastoma patients. Clin Immunol (2019) 203:63–71. doi: 10.1016/j.clim.2019.04.006
90. Luoma AM, Castro CD, Adams EJ. γδ T cell surveillance via CD1 molecules. Trends Immunol (2014) 35(12):613–21. doi: 10.1016/j.it.2014.09.003
91. Luoma AM, Castro CD, Mayassi T, Bembinster LA, Bai L, Picard D, et al. Crystal structure of Vδ1 T cell receptor in complex with CD1d-sulfatide shows MHC-like recognition of a self-lipid by human γδ T cells. Immunity (2013) 39(6):1032–42. doi: 10.1016/j.immuni.2013.11.001
92. Dhodapkar MV, Geller MD, Chang DH, Shimizu K, Fujii S, Dhodapkar KM, et al. A reversible defect in natural killer T cell function characterizes the progression of premalignant to malignant multiple myeloma. J Exp Med (2003) 197(12):1667–76. doi: 10.1084/jem.20021650
93. Shyanti RK, Sehrawat A, Singh SV, Mishra JPN, Singh RP. Zerumbone modulates CD1d expression and lipid antigen presentation pathway in breast cancer cells. Toxicol In Vitro (2017) 44:74–84. doi: 10.1016/j.tiv.2017.06.016
94. Nowak M, Arredouani MS, Tun-Kyi A, Schmidt-Wolf I, Sanda MG, Balk SP, et al. Defective NKT cell activation by CD1d+ TRAMP prostate tumor cells is corrected by interleukin-12 with α-galactosylceramide. PloS One (2010) 5(6):e11311. doi: 10.1371/journal.pone.0011311
95. Vermeulen JF, van Hecke W, Spliet WG, Villacorta Hidalgo J, Fisch P, Broekhuizen R, et al. Pediatric Primitive Neuroectodermal Tumors of the Central Nervous System Differentially Express Granzyme Inhibitors. PloS One (2016) 11(3):e0151465. doi: 10.1371/journal.pone.0151465
96. Xu B, Pizarro JC, Holmes MA, McBeth C, Groh V, Spies T, et al. Crystal structure of a gammadelta T-cell receptor specific for the human MHC class I homolog MICA. Proc Natl Acad Sci U S A (2011) 108(6):2414–9. doi: 10.1073/pnas.1015433108
97. Poggi A, Venturino C, Catellani S, Clavio M, Miglino M, Gobbi M, et al. Vdelta1 T lymphocytes from B-CLL patients recognize ULBP3 expressed on leukemic B cells and up-regulated by trans-retinoic acid. Cancer Res (2004) 64(24):9172–9. doi: 10.1158/0008-5472.CAN-04-2417
98. Almeida AR, Correia DV, Fernandes-Platzgummer A, da Silva CL, da Silva MG, Anjos DR, et al. Delta One T Cells for Immunotherapy of Chronic Lymphocytic Leukemia: Clinical-Grade Expansion/Differentiation and Preclinical Proof of Concept. Clin Cancer Res (2016) 22(23):5795–804. doi: 10.1158/1078-0432.CCR-16-0597
99. Hudspeth K, Silva-Santos B, Mavilio D. Natural cytotoxicity receptors: broader expression patterns and functions in innate and adaptive immune cells. Front Immunol (2013) 4:69. doi: 10.3389/fimmu.2013.00069
100. Silva-Santos B, Strid J. Working in “NK Mode”: Natural Killer Group 2 Member D and Natural Cytotoxicity Receptors in Stress-Surveillance by γδ T Cells. Front Immunol (2018) 9:851. doi: 10.3389/fimmu.2018.00851
101. Di Lorenzo B, Simões AE, Caiado F, Tieppo P, Correia DV, Carvalho T, et al. Broad Cytotoxic Targeting of Acute Myeloid Leukemia by Polyclonal Delta One T Cells. Cancer Immunol Res (2019) 7(4):552–8. doi: 10.1158/2326-6066.CIR-18-0647
102. Voskoboinik I, Whisstock JC, Trapani JA. Perforin and granzymes: function, dysfunction and human pathology. Nat Rev Immunol (2015) 15(6):388–400. doi: 10.1038/nri3839
103. Praper T, Sonnen A, Viero G, Kladnik A, Froelich CJ, Anderluh G, et al. Human perforin employs different avenues to damage membranes. J Biol Chem (2011) 286(4):2946–55. doi: 10.1074/jbc.M110.169417
104. Prager I, Liesche C, van Ooijen H, Urlaub D, Verron Q, Sandström N, et al. NK cells switch from granzyme B to death receptor-mediated cytotoxicity during serial killing. J Exp Med (2019) 216(9):2113–27. doi: 10.1084/jem.20181454
105. van Tetering G, Bovenschen N, Meeldijk J, van Diest PJ, Vooijs M. Cleavage of Notch1 by granzyme B disables its transcriptional activity. Biochem J (2011) 437(2):313–22. doi: 10.1042/BJ20110226
106. Alexander AA, Maniar A, Cummings JS, Hebbeler AM, Schulze DH, Gastman BR, et al. Isopentenyl pyrophosphate-activated CD56+ {gamma}{delta} T lymphocytes display potent antitumor activity toward human squamous cell carcinoma. Clin Cancer Res (2008) 14(13):4232–40. doi: 10.1158/1078-0432.CCR-07-4912
107. Dhar S, Chiplunkar SV. Lysis of aminobisphosphonate-sensitized MCF-7 breast tumor cells by Vγ9Vδ2 T cells. Cancer Immun (2010) 10:10.
108. Viey E, Fromont G, Escudier B, Morel Y, Da Rocha S, Chouaib S, et al. Phosphostim-activated gamma delta T cells kill autologous metastatic renal cell carcinoma. J Immunol (2005) 174(3):1338–47. doi: 10.4049/jimmunol.174.3.1338
109. Lafont V, Liautard J, Liautard JP, Favero J. Production of TNF-alpha by human V gamma 9V delta 2 T cells via engagement of Fc gamma RIIIA, the low affinity type 3 receptor for the Fc portion of IgG, expressed upon TCR activation by nonpeptidic antigen. J Immunol (2001) 166(12):7190–9. doi: 10.4049/jimmunol.166.12.7190
110. Fisher JP, Yan M, Heuijerjans J, Carter L, Abolhassani A, Frosch J, et al. Neuroblastoma killing properties of Vδ2 and Vδ2-negative γδT cells following expansion by artificial antigen-presenting cells. Clin Cancer Res (2014) 20(22):5720–32. doi: 10.1158/1078-0432.CCR-13-3464
111. Tokuyama H, Hagi T, Mattarollo SR, Morley J, Wang Q, So HF, et al. V gamma 9 V delta 2 T cell cytotoxicity against tumor cells is enhanced by monoclonal antibody drugs–rituximab and trastuzumab. Int J Cancer (2008) 122(11):2526–34. doi: 10.1002/ijc.23365
112. Gertner-Dardenne J, Bonnafous C, Bezombes C, Capietto AH, Scaglione V, Ingoure S, et al. Bromohydrin pyrophosphate enhances antibody-dependent cell-mediated cytotoxicity induced by therapeutic antibodies. Blood (2009) 113(20):4875–84. doi: 10.1182/blood-2008-08-172296
113. Capietto AH, Martinet L, Fournié JJ. Stimulated γδ T cells increase the in vivo efficacy of trastuzumab in HER-2+ breast cancer. J Immunol (2011) 187(2):1031–8. doi: 10.4049/jimmunol.1100681
114. Tatsuta T, Shiraishi A, Mountz JD. The prodomain of caspase-1 enhances Fas-mediated apoptosis through facilitation of caspase-8 activation. J Biol Chem (2000) 275(19):14248–54. doi: 10.1074/jbc.275.19.14248
115. Sprick MR, Rieser E, Stahl H, Grosse-Wilde A, Weigand MA, Walczak H. Caspase-10 is recruited to and activated at the native TRAIL and CD95 death-inducing signalling complexes in a FADD-dependent manner but can not functionally substitute caspase-8. EMBO J (2002) 21(17):4520–30. doi: 10.1093/emboj/cdf441
116. Milhas D, Cuvillier O, Therville N, Clavé P, Thomsen M, Levade T, et al. Caspase-10 triggers Bid cleavage and caspase cascade activation in FasL-induced apoptosis. J Biol Chem (2005) 280(20):19836–42. doi: 10.1074/jbc.M414358200
117. Todaro M, D’Asaro M, Caccamo N, Iovino F, Francipane MG, Meraviglia S, et al. Efficient killing of human colon cancer stem cells by gammadelta T lymphocytes. J Immunol (2009) 182(11):7287–96. doi: 10.4049/jimmunol.0804288
118. Dieli F, Vermijlen D, Fulfaro F, Caccamo N, Meraviglia S, Cicero G, et al. Targeting human {gamma}delta} T cells with zoledronate and interleukin-2 for immunotherapy of hormone-refractory prostate cancer. Cancer Res (2007) 67(15):7450–7. doi: 10.1158/0008-5472.CAN-07-0199
119. Li Z, Peng H, Xu Q, Ye Z. Sensitization of human osteosarcoma cells to Vγ9Vδ2 T-cell-mediated cytotoxicity by zoledronate. J Orthop Res (2012) 30(5):824–30. doi: 10.1002/jor.21579
120. Dokouhaki P, Schuh NW, Joe B, Allen CA, Der SD, Tsao MS, et al. NKG2D regulates production of soluble TRAIL by ex vivo expanded human γδ T cells. Eur J Immunol (2013) 43(12):3175–82. doi: 10.1002/eji.201243150
121. Fiandalo MV, Schwarze SR, Kyprianou N. Proteasomal regulation of caspase-8 in cancer cell apoptosis. Apoptosis (2013) 18(6):766–76. doi: 10.1007/s10495-013-0821-y
122. Li M, Le W, Zhang XM, Zhang YJ, Jiang J, Liu PY. The M476W/Q482H mutation of procaspase-8 restored caspase-8-mediated apoptosis. Biochem Biophys Res Commun (2019) 514(3):653–8. doi: 10.1016/j.bbrc.2019.05.023
123. Mao ZG, Jiang CC, Yang F, Thorne RF, Hersey P, Zhang XD. TRAIL-induced apoptosis of human melanoma cells involves activation of caspase-4. Apoptosis (2010) 15(10):1211–22. doi: 10.1007/s10495-010-0513-9
124. Seo J, Lee EW, Shin J, Seong D, Nam YW, Jeong M, et al. K6 linked polyubiquitylation of FADD by CHIP prevents death inducing signaling complex formation suppressing cell death. Oncogene (2018) 37(36):4994–5006. doi: 10.1038/s41388-018-0323-z
125. Li Z, Xu Q, Peng H, Cheng R, Sun Z, Ye Z. IFN-γ enhances HOS and U2OS cell lines susceptibility to γδ T cell-mediated killing through the Fas/Fas ligand pathway. Int Immunopharmacol (2011) 11(4):496–503. doi: 10.1016/j.intimp.2011.01.001
126. Reboursiere E, Gac AC, Garnier A, Salaun V, Reman O, Pham AD, et al. Increased frequencies of circulating and tumor-resident Vδ1+ T cells in patients with diffuse large B-cell lymphoma. Leuk Lymphoma (2018) 59(1):187–95. doi: 10.1080/10428194.2017.1321751
127. Ebert LM, Meuter S, Moser B. Homing and function of human skin gammadelta T cells and NK cells: relevance for tumor surveillance. J Immunol (2006) 176(7):4331–6. doi: 10.4049/jimmunol.176.7.4331
128. Zhou J, Kang N, Cui L, Ba D, He W. Anti-γδ TCR antibody-expanded γδ T cells: a better choice for the adoptive immunotherapy of lymphoid malignancies. Cell Mol Immunol (2012) 9(1):34–44. doi: 10.1038/cmi.2011.16
129. Simões C, Silva I, Carvalho A, Silva S, Santos S, Marques G, et al. Quantification and phenotypic characterization of peripheral blood Vδ1 + T cells in chronic lymphocytic leukemia and monoclonal B cell lymphocytosis. Cytometry B Clin Cytom (2019) 96(2):164–8. doi: 10.1002/cyto.b.21645
130. Correia DV, Fogli M, Hudspeth K, da Silva MG, Mavilio D, Silva-Santos B. Differentiation of human peripheral blood Vδ1+ T cells expressing the natural cytotoxicity receptor NKp30 for recognition of lymphoid leukemia cells. Blood (2011) 118(4):992–1001. doi: 10.1182/blood-2011-02-339135
131. Wu D, Wu P, Wu X, Ye J, Wang Z, Zhao S, et al. Ex vivo expanded human circulating Vδ1 γδT cells exhibit favorable therapeutic potential for colon cancer. Oncoimmunology (2015) 4(3):e992749. doi: 10.4161/2162402X.2014.992749
132. Brandes M, Willimann K, Moser B. Professional antigen-presentation function by human gammadelta T Cells. Science (2005) 309(5732):264–8. doi: 10.1126/science.1110267
133. Khan MW, Curbishley SM, Chen HC, Thomas AD, Pircher H, Mavilio D, et al. Expanded Human Blood-Derived γδT Cells Display Potent Antigen-Presentation Functions. Front Immunol (2014) 5:344. doi: 10.3389/fimmu.2014.00344
134. Mao C, Mou X, Zhou Y, Yuan G, Xu C, Liu H, et al. Tumor-activated TCRγδ+ T cells from gastric cancer patients induce the antitumor immune response of TCRαβ+ T cells via their antigen-presenting cell-like effects. J Immunol Res (2014) 2014:593562. doi: 10.1155/2014/593562
135. Sawaisorn P, Tangchaikeeree T, Chan-On W, Leepiyasakulchai C, Udomsangpetch R, Hongeng S, et al. Antigen-Presenting Cell Characteristics of Human γδ T Lymphocytes in Chronic Myeloid Leukemia. Immunol Invest (2019) 48(1):11–26. doi: 10.1080/08820139.2018.1529039
136. Muto M, Baghdadi M, Maekawa R, Wada H, Seino K. Myeloid molecular characteristics of human γδ T cells support their acquisition of tumor antigen-presenting capacity. Cancer Immunol Immunother (2015) 64(8):941–9. doi: 10.1007/s00262-015-1700-x
137. Himoudi N, Morgenstern DA, Yan M, Vernay B, Saraiva L, Wu Y, et al. Human γδ T lymphocytes are licensed for professional antigen presentation by interaction with opsonized target cells. J Immunol (2012) 188(4):1708–16. doi: 10.4049/jimmunol.1102654
138. Chen HC, Joalland N, Bridgeman JS, Alchami FS, Jarry U, Khan MWA, et al. Synergistic targeting of breast cancer stem-like cells by human γδ T cells and CD8+ T cells. Immunol Cell Biol (2017) 95(7):620–9. doi: 10.1038/icb.2017.21
139. Münz C, Steinman RM, Fujii S. Dendritic cell maturation by innate lymphocytes: coordinated stimulation of innate and adaptive immunity. J Exp Med (2005) 202(2):203–7. doi: 10.1084/jem.20050810
140. Devilder MC, Maillet S, Bouyge-Moreau I, Donnadieu E, Bonneville M, Scotet E. Potentiation of antigen-stimulated V gamma 9V delta 2 T cell cytokine production by immature dendritic cells (DC) and reciprocal effect on DC maturation. J Immunol (2006) 176(3):1386–93. doi: 10.4049/jimmunol.176.3.1386
141. Conti L, Casetti R, Cardone M, Varano B, Martino A, Belardelli F, et al. Reciprocal activating interaction between dendritic cells and pamidronate-stimulated gammadelta T cells: role of CD86 and inflammatory cytokines. J Immunol (2005) 174(1):252–60. doi: 10.4049/jimmunol.174.1.252
142. Girard P, Ponsard B, Charles J, Chaperot L, Aspord C. Potent Bidirectional Cross-Talk Between Plasmacytoid Dendritic Cells and γδT Cells Through BTN3A, Type I/II IFNs and Immune Checkpoints. Front Immunol (2020) 11:861. doi: 10.3389/fimmu.2020.00861
143. Castella B, Kopecka J, Sciancalepore P, Mandili G, Foglietta M, Mitro N, et al. The ATP-binding cassette transporter A1 regulates phosphoantigen release and Vγ9Vδ2 T cell activation by dendritic cells. Nat Commun (2017) 8:15663. doi: 10.1038/ncomms15663
144. Nabholtz JM, Abrial C, Mouret-Reynier MA, Dauplat MM, Weber B, Gligorov J, et al. Multicentric neoadjuvant phase II study of panitumumab combined with an anthracycline/taxane-based chemotherapy in operable triple-negative breast cancer: identification of biologically defined signatures predicting treatment impact. Ann Oncol (2014) 25(8):1570–7. doi: 10.1093/annonc/mdu183
145. Van Hede D, Polese B, Humblet C, Wilharm A, Renoux V, Dortu E, et al. Human papillomavirus oncoproteins induce a reorganization of epithelial-associated γδ T cells promoting tumor formation. Proc Natl Acad Sci U S A (2017) 114(43):E9056–65. doi: 10.1073/pnas.1712883114
146. Zhou BY, Gong JH, Cai XY, Wang JX, Luo F, Jiang N, et al. An imbalance between stellate cells and γδT cells contributes to hepatocellular carcinoma aggressiveness and recurrence. Hepatol Int (2019) 13(5):631–40. doi: 10.1007/s12072-019-09969-w
147. Welte T, Zhang XH. Interleukin-17 Could Promote Breast Cancer Progression at Several Stages of the Disease. Mediators Inflamm (2015) 2015:804347. doi: 10.1155/2015/804347
148. Qian X, Chen H, Wu X, Hu L, Huang Q, Jin Y. Interleukin-17 acts as double-edged sword in anti-tumor immunity and tumorigenesis. Cytokine (2017) 89:34–44. doi: 10.1016/j.cyto.2015.09.011
149. Meraviglia S, Lo Presti E, Tosolini M, La Mendola C, Orlando V, Todaro M, et al. Distinctive features of tumor-infiltrating γδ T lymphocytes in human colorectal cancer. Oncoimmunology (2017) 6(10):e1347742. doi: 10.1080/2162402X.2017.1347742
150. Wu P, Wu D, Ni C, Ye J, Chen W, Hu G, et al. γδT17 cells promote the accumulation and expansion of myeloid-derived suppressor cells in human colorectal cancer. Immunity (2014) 40(5):785–800. doi: 10.1016/j.immuni.2014.03.013
151. Aotsuka A, Matsumoto Y, Arimoto T, Kawata A, Ogishima J, Taguchi A, et al. Interleukin-17 is associated with expression of programmed cell death 1 ligand 1 in ovarian carcinoma. Cancer Sci (2019) 110(10):3068–78. doi: 10.1111/cas.14174
152. Wistuba-Hamprecht K, Di Benedetto S, Schilling B, Sucker A, Schadendorf D, Garbe C, et al. Phenotypic characterization and prognostic impact of circulating γδ and αβ T-cells in metastatic malignant melanoma. Int J Cancer (2016) 138(3):698–704. doi: 10.1002/ijc.29818
153. Wistuba-Hamprecht K, Martens A, Haehnel K, Geukes Foppen M, Yuan J, Postow MA, et al. Proportions of blood-borne Vδ1+ and Vδ2+ T-cells are associated with overall survival of melanoma patients treated with ipilimumab. Eur J Cancer (2016) 64:116–26. doi: 10.1016/j.ejca.2016.06.001
154. Rong L, Li K, Li R, Liu HM, Sun R, Liu XY. Analysis of tumor-infiltrating gamma delta T cells in rectal cancer. World J Gastroenterol (2016) 22(13):3573–80. doi: 10.3748/wjg.v22.i13.3573
155. Chen X, Shang W, Xu R, Wu M, Zhang X, Huang P, et al. Distribution and functions of γδ T cells infiltrated in the ovarian cancer microenvironment. J Transl Med (2019) 17(1):144. doi: 10.1186/s12967-019-1897-0
156. Patil RS, Shah SU, Shrikhande SV, Goel M, Dikshit RP, Chiplunkar SV. IL17 producing γδT cells induce angiogenesis and are associated with poor survival in gallbladder cancer patients. Int J Cancer (2016) 139(4):869–81. doi: 10.1002/ijc.30134
157. Sacchi A, Tumino N, Sabatini A, Cimini E, Casetti R, Bordoni V, et al. Myeloid-Derived Suppressor Cells Specifically Suppress IFN-γ Production and Antitumor Cytotoxic Activity of Vδ2 T Cells. Front Immunol (2018) 9:1271. doi: 10.3389/fimmu.2018.01271
158. Yu SJ, Ma C, Heinrich B, Brown ZJ, Sandhu M, Zhang Q, et al. Targeting the crosstalk between cytokine-induced killer cells and myeloid-derived suppressor cells in hepatocellular carcinoma. J Hepatol (2019) 70(3):449–57. doi: 10.1016/j.jhep.2018.10.040
159. Romano A, Parrinello NL, La Cava P, Tibullo D, Giallongo C, Camiolo G, et al. PMN-MDSC and arginase are increased in myeloma and may contribute to resistance to therapy. Expert Rev Mol Diagn (2018) 18(7):675–83. doi: 10.1080/14737159.2018.1470929
160. Okła K, Czerwonka A, Wawruszak A, Bobiński M, Bilska M, Tarkowski R, et al. Clinical Relevance and Immunosuppressive Pattern of Circulating and Infiltrating Subsets of Myeloid-Derived Suppressor Cells (MDSCs) in Epithelial Ovarian Cancer. Front Immunol (2019) 10:691. doi: 10.3389/fimmu.2019.00691
161. Peng G, Wang HY, Peng W, Kiniwa Y, Seo KH, Wang RF. Tumor-infiltrating gammadelta T cells suppress T and dendritic cell function via mechanisms controlled by a unique toll-like receptor signaling pathway. Immunity (2007) 27(2):334–48. doi: 10.1016/j.immuni.2007.05.020
162. Ye J, Ma C, Wang F, Hsueh EC, Toth K, Huang Y, et al. Specific recruitment of γδ regulatory T cells in human breast cancer. Cancer Res (2013) 73(20):6137–48. doi: 10.1158/0008-5472.CAN-13-0348
163. Ye J, Ma C, Hsueh EC, Eickhoff CS, Zhang Y, Varvares MA, et al. Tumor-derived γδ regulatory T cells suppress innate and adaptive immunity through the induction of immunosenescence. J Immunol (2013) 190(5):2403–14. doi: 10.4049/jimmunol.1202369
164. Kobayashi H, Tanaka Y, Yagi J, Osaka Y, Nakazawa H, Uchiyama T, et al. Safety profile and anti-tumor effects of adoptive immunotherapy using gamma-delta T cells against advanced renal cell carcinoma: a pilot study. Cancer Immunol Immunother (2007) 56(4):469–76. doi: 10.1007/s00262-006-0199-6
165. Kobayashi H, Tanaka Y, Yagi J, Minato N, Tanabe K. Phase I/II study of adoptive transfer of γδ T cells in combination with zoledronic acid and IL-2 to patients with advanced renal cell carcinoma. Cancer Immunol Immunother (2011) 60(8):1075–84. doi: 10.1007/s00262-011-1021-7
166. Nicol AJ, Tokuyama H, Mattarollo SR, Hagi T, Suzuki K, Yokokawa K, et al. Clinical evaluation of autologous gamma delta T cell-based immunotherapy for metastatic solid tumours. Br J Cancer (2011) 105(6):778–86. doi: 10.1038/bjc.2011.293
167. Abe Y, Muto M, Nieda M, Nakagawa Y, Nicol A, Kaneko T, et al. Clinical and immunological evaluation of zoledronate-activated Vgamma9gammadelta T-cell-based immunotherapy for patients with multiple myeloma. Exp Hematol (2009) 37(8):956–68. doi: 10.1016/j.exphem.2009.04.008
168. Bennouna J, Bompas E, Neidhardt EM, Rolland F, Philip I, Galéa C, et al. Phase-I study of Innacell gammadelta, an autologous cell-therapy product highly enriched in gamma9delta2 T lymphocytes, in combination with IL-2, in patients with metastatic renal cell carcinoma. Cancer Immunol Immunother (2008) 57(11):1599–609. doi: 10.1007/s00262-008-0491-8
169. Nakajima J, Murakawa T, Fukami T, Goto S, Kaneko T, Yoshida Y, et al. A phase I study of adoptive immunotherapy for recurrent non-small-cell lung cancer patients with autologous gammadelta T cells. Eur J Cardiothorac Surg (2010) 37(5):1191–7. doi: 10.1016/j.ejcts.2009.11.051
170. Sakamoto M, Nakajima J, Murakawa T, Fukami T, Yoshida Y, Murayama T, et al. Adoptive immunotherapy for advanced non-small cell lung cancer using zoledronate-expanded γδTcells: a phase I clinical study. J Immunother (2011) 34(2):202–11. doi: 10.1097/CJI.0b013e318207ecfb
171. Wada I, Matsushita H, Noji S, Mori K, Yamashita H, Nomura S, et al. Intraperitoneal injection of in vitro expanded Vγ9Vδ2 T cells together with zoledronate for the treatment of malignant ascites due to gastric cancer. Cancer Med (2014) 3(2):362–75. doi: 10.1002/cam4.196
172. Lang JM, Kaikobad MR, Wallace M, Staab MJ, Horvath DL, Wilding G, et al. Pilot trial of interleukin-2 and zoledronic acid to augment γδ T cells as treatment for patients with refractory renal cell carcinoma. Cancer Immunol Immunother (2011) 60(10):1447–60. doi: 10.1007/s00262-011-1049-8
173. Pressey JG, Adams J, Harkins L, Kelly D, You Z, Lamb LS Jr. In vivo expansion and activation of γδ T cells as immunotherapy for refractory neuroblastoma: A phase 1 study. Med (Baltimore) (2016) 95(39):e4909. doi: 10.1097/MD.0000000000004909
174. Kunzmann V, Smetak M, Kimmel B, Weigang-Koehler K, Goebeler M, Birkmann J, et al. Tumor-promoting versus tumor-antagonizing roles of γδ T cells in cancer immunotherapy: results from a prospective phase I/II trial. J Immunother (2012) 35(2):205–13. doi: 10.1097/CJI.0b013e318245bb1e
175. Wilhelm M, Kunzmann V, Eckstein S, Reimer P, Weissinger F, Ruediger T, et al. Gammadelta T cells for immune therapy of patients with lymphoid malignancies. Blood (2003) 102(1):200–6. doi: 10.1182/blood-2002-12-3665
176. Bennouna J, Levy V, Sicard H, Senellart H, Audrain M, Hiret S, et al. Phase I study of bromohydrin pyrophosphate (BrHPP, IPH 1101), a Vgamma9Vdelta2 T lymphocyte agonist in patients with solid tumors. Cancer Immunol Immunother (2010) 59(10):1521–30. doi: 10.1007/s00262-010-0879-0
177. Kouakanou L, Xu Y, Peters C, He J, Wu Y, Yin Z, et al. Vitamin C promotes the proliferation and effector functions of human γδ T cells. Cell Mol Immunol (2020) 17(5):462–73. doi: 10.1038/s41423-019-0247-8
178. Jiang H, Yang Z, Song Z, Green M, Song H, Shao Q. γδ T cells in hepatocellular carcinoma patients present cytotoxic activity but are reduced in potency due to IL-2 and IL-21 pathways. Int Immunopharmacol (2019) 70:167–73. doi: 10.1016/j.intimp.2019.02.019
179. Duault C, Betous D, Bezombes C, Roga S, Cayrol C, Girard JP, et al. IL-33-expanded human Vγ9Vδ2 T cells have anti-lymphoma effect in a mouse tumor model. Eur J Immunol (2017) 47(12):2137–41. doi: 10.1002/eji.201747093
180. Van Acker HH, Anguille S, Willemen Y, Van den Bergh JM, Berneman ZN, Lion E, et al. Interleukin-15 enhances the proliferation, stimulatory phenotype, and antitumor effector functions of human gamma delta T cells. J Hematol Oncol (2016) 9(1):101. doi: 10.1186/s13045-016-0329-3
181. Cairo C, Sagnia B, Cappelli G, Colizzi V, Leke RG, Leke RJ, et al. Human cord blood γδ T cells expressing public Vγ2 chains dominate the response to bisphosphonate plus interleukin-15. Immunology (2013) 138(4):346–60. doi: 10.1111/imm.12039
182. Duault C, Franchini DM, Familliades J, Cayrol C, Roga S, Girard JP, et al. TCRVγ9 γδ T Cell Response to IL-33: A CD4 T Cell-Dependent Mechanism. J Immunol (2016) 196(1):493–502. doi: 10.4049/jimmunol.1500260
183. Aoki T, Matsushita H, Hoshikawa M, Hasegawa K, Kokudo N, Kakimi K. Adjuvant combination therapy with gemcitabine and autologous γδ T-cell transfer in patients with curatively resected pancreatic cancer. Cytotherapy (2017) 19(4):473–85. doi: 10.1016/j.jcyt.2017.01.002
184. Wang S, Li H, Ye C, Lin P, Li B, Zhang W, et al. Valproic Acid Combined with Zoledronate Enhance γδ T Cell-Mediated Cytotoxicity against Osteosarcoma Cells via the Accumulation of Mevalonate Pathway Intermediates. Front Immunol (2018) 9:377:377. doi: 10.3389/fimmu.2018.00377
185. Lin L, He J, Li J, Xu Y, Li J, Wu Y. Chitosan Nanoparticles Strengthen Vγ9Vδ2 T-Cell Cytotoxicity Through Upregulation Of Killing Molecules And Cytoskeleton Polarization. Int J Nanomedicine (2019) 14:9325–36. doi: 10.2147/IJN.S212898
186. Rossi C, Gravelle P, Decaup E, Bordenave J, Poupot M, Tosolini M, et al. Boosting γδ T cell-mediated antibody-dependent cellular cytotoxicity by PD-1 blockade in follicular lymphoma. Oncoimmunology (2018) 8(3):1554175. doi: 10.1080/2162402X.2018.1554175
187. Wu K, Zhao H, Xiu Y, Li Z, Zhao J, Xie S, et al. IL-21-mediated expansion of Vγ9Vδ2 T cells is limited by the Tim-3 pathway. Int Immunopharmacol (2019) 69:136–42. doi: 10.1016/j.intimp.2019.01.027
188. Li X, Lu H, Gu Y, Zhang X, Zhang G, Shi T, et al. Tim-3 suppresses the killing effect of Vγ9Vδ2+T cells on colon cancer cells by reducing perforin and granzyme B expression. Exp Cell Res (2020) 386(1):111719. doi: 10.1016/j.yexcr.2019.111719
189. Hoh A, Dewerth A, Vogt F, Wenz J, Baeuerle PA, Warmann SW, et al. The activity of γδ T cells against paediatric liver tumour cells and spheroids in cell culture. Liver Int (2013) 33(1):127–36. doi: 10.1111/liv.12011
190. Oberg HH, Janitschke L, Sulaj V, Weimer J, Gonnermann D, Hedemann N, et al. Bispecific antibodies enhance tumor-infiltrating T cell cytotoxicity against autologous HER-2-expressing high-grade ovarian tumors. J Leukoc Biol (2020) 107(6):1081–95. doi: 10.1002/JLB.5MA1119-265R
191. Oberg HH, Peipp M, Kellner C, Sebens S, Krause S, Petrick D, et al. Novel bispecific antibodies increase γδ T-cell cytotoxicity against pancreatic cancer cells. Cancer Res (2014) 74(5):1349–60. doi: 10.1158/0008-5472.CAN-13-0675
192. Oberg HH, Kellner C, Gonnermann D, Peipp M, Peters C, Sebens S, et al. γδ T cell activation by bispecific antibodies. Cell Immunol (2015) 296(1):41–9. doi: 10.1016/j.cellimm.2015.04.009
193. Tawfik D, Groth C, Gundlach JP, Peipp M, Kabelitz D, Becker T, et al. TRAIL-Receptor 4 Modulates γδ T Cell-Cytotoxicity Toward Cancer Cells. Front Immunol (2019) 10:2044. doi: 10.3389/fimmu.2019.02044
194. Fisher J, Abramowski P, Wisidagamage Don ND, Flutter B, Capsomidis A, Cheung GW, et al. Avoidance of On-Target Off-Tumor Activation Using a Co-stimulation-Only Chimeric Antigen Receptor. Mol Ther (2017) 25(5):1234–47. doi: 10.1016/j.ymthe.2017.03.002
195. Capsomidis A, Benthall G, Van Acker HH, Fisher J, Kramer AM, Abeln Z, et al. Chimeric Antigen Receptor-Engineered Human Gamma Delta T Cells: Enhanced Cytotoxicity with Retention of Cross Presentation. Mol Ther (2018) 26(2):354–65. doi: 10.1016/j.ymthe.2017.12.001
196. Marcu-Malina V, Heijhuurs S, van Buuren M, Hartkamp L, Strand S, Sebestyen Z, et al. Redirecting αβ T cells against cancer cells by transfer of a broadly tumor-reactive γδT-cell receptor. Blood (2011) 118(1):50–9. doi: 10.1182/blood-2010-12-325993
197. Gründer C, van Dorp S, Hol S, Drent E, Straetemans T, Heijhuurs S, et al. γ9 and δ2CDR3 domains regulate functional avidity of T cells harboring γ9δ2TCRs. Blood (2012) 120(26):5153–62. doi: 10.1182/blood-2012-05-432427
198. Braham MVJ, Minnema MC, Aarts T, Sebestyen Z, Straetemans T, Vyborova A, et al. Cellular immunotherapy on primary multiple myeloma expanded in a 3D bone marrow niche model. Oncoimmunology (2018) 7(6):e1434465. doi: 10.1080/2162402X.2018.1434465
199. Straetemans T, Kierkels GJJ, Doorn R, Jansen K, Heijhuurs S, Dos Santos JM, et al. GMP-Grade Manufacturing of T Cells Engineered to Express a Defined γδTCR. Front Immunol (2018) 9:1062. doi: 10.3389/fimmu.2018.01062
Keywords: γδ T cells, tumor, human, immunity, immunotherapy
Citation: Li Y, Li G, Zhang J, Wu X and Chen X (2021) The Dual Roles of Human γδ T Cells: Anti-Tumor or Tumor-Promoting. Front. Immunol. 11:619954. doi: 10.3389/fimmu.2020.619954
Received: 21 October 2020; Accepted: 29 December 2020;
Published: 16 February 2021.
Edited by:
Jianlei Hao, Jinan University, ChinaReviewed by:
Haiyan Liu, National University of Singapore, SingaporeYangqiu Li, Jinan University, China
Manolo Sambucci, Santa Lucia Foundation (IRCCS), Italy
Copyright © 2021 Li, Li, Zhang, Wu and Chen. This is an open-access article distributed under the terms of the Creative Commons Attribution License (CC BY). The use, distribution or reproduction in other forums is permitted, provided the original author(s) and the copyright owner(s) are credited and that the original publication in this journal is cited, in accordance with accepted academic practice. No use, distribution or reproduction is permitted which does not comply with these terms.
*Correspondence: Xi Chen, YW5keWNoZW4wODAzQHNpbmEuY29t; Xiaoli Wu, d3V4aWFvbGlAdGp1LmVkdS5jbg==
†These authors have contributed equally to this work