- Division of Bacterial, Parasitic, and Allergenic Products, Office of Vaccines Research and Review, Center for Biologics Evaluation and Research, US Food and Drug Administration, Silver Spring, MD, United States
Type I interferons (IFN-I) were first discovered as an antiviral factor by Isaacs and Lindenmann in 1957, but they are now known to also modulate innate and adaptive immunity and suppress proliferation of cancer cells. While much has been revealed about IFN-I, it remains a mystery as to why there are 16 different IFN-I gene products, including IFNβ, IFNω, and 12 subtypes of IFNα. Here, we discuss shared and unique aspects of these IFN-I in the context of their evolution, expression patterns, and signaling through their shared heterodimeric receptor. We propose that rather than investigating responses to individual IFN-I, these contexts can serve as an alternative approach toward investigating roles for IFNα subtypes. Finally, we review uses of IFNα and IFNβ as therapeutic agents to suppress chronic viral infections or to treat multiple sclerosis.
Introduction
Type I interferons (IFN-I) are monomeric cytokines that are best known for their antiviral activity but that also suppress proliferation of cancer cells and modulate innate and adaptive immune responses. IFN-I were first discovered as an antiviral factor by Isaacs and Lindenmann in 1957 and were subsequently revealed to include IFNβ and multiple subtypes of IFNα (1, 2). We now know that human type I IFNs comprise a family of 17 functional genes and 9 pseudogenes clustered on chromosome 9 (3) that encode 16 proteins: IFNβ, ϵ, -κ, -ω, and 12 subtypes of IFNα (Figure 1). Since protein sequences for mature IFNα1 and IFNα13 are identical, we will collectively refer to them as IFNα1.

Figure 1 Gene map of the human IFN-I gene cluster. Above the line are pseudogenes for IFNν (NNP), IFNα subtypes, IFNω, and for the functional KLHL9 gene. On the line are the 17 functional type I IFN genes. Genes for IFNα subtypes are labeled only by number.
IFNβ may be considered the “primary” IFN-I because it is expressed by all nucleated cells and may be expressed in isolation of all other IFN-I (except IFNα1, discussed below). Two IFN-I genes are selectively expressed in specific organs or by specific cell types: IFNϵ is hormonally regulated and primarily expressed in the female genital tract (4) and has recently been reviewed elsewhere. IFNκ is primarily expressed by keratinocytes (5) where it has recently been shown to have a role in protection against cutaneous herpes simplex virus (6), papilloma virus (7), and cutaneous lupus erythematosus (8). Like IFNϵ, IFNκ is constitutively expressed (9). By contrast, IFNκ expression is activated and suppressed by TGFβ and ERK1/2 kinases, respectively (7, 10).
While IFNω is the least studied IFN-I in human biology, feline IFNω is well characterized and licensed as a veterinary antiviral therapeutic. In felines, IFNω is leukocyte specific (11, 12). While little is known about IFNω expression patterns, the presence of neutralizing autoantibodies is indirect proof that it is expressed and suggest a role in human disease. For example, in 2006, Meager et al. reported that 100% of AIRE-deficient patients with the autoimmune polyendocrinopathy syndrome have high titers of neutralizing autoantibodies against both IFNω and IFNα (13). More recently, Bastard et al. reported that ~1% of patients with severe Covid19 has selective neutralizing auto-antibodies against IFNω (14), suggesting that the importance of this type I IFN is in viral infections is underappreciated.
Mature IFNβ and eleven of the 12 IFNα subtypes are 166 a.a. in length (IFNα2 is 165 a.a. due to deletion of D44) with a MW of ~20 kD. IFNϵ and IFNω are 187 a.a. and 174 a.a., respectively, both due to an elongated carboxy-terminal, while IFNκ is 179 a.a. due to an insertion following residue 116. As shown in Supplemental Figure 1 (15), IFNβ and IFNω share 31%–38% and 55%–60% identity with all IFNα subtypes, respectively, whereas identity among the IFNα subtypes ranges from 76%–96%. IFNβ, IFNω, and two IFNα subtypes are glycosylated; IFNβ at N80 (16), IFNω at N78 (17), IFNα2 at T108 (18), and IFNα14 at N72 (19).
Despite sharing only ~30% identity across all IFN-I, the three-dimensional structures are remarkably similar (20, 21). The salient structural features of all IFN-I, which are reviewed in detail by Walter et al. in this series include: 1) cylindrical proteins that consist of five 11-24 residue α-helices (labeled A–E), each parallel to the long axis of the cylinder; 2) Loops that connect the helices, of which the AB loop is relatively long and includes three short 310 helices (22, 23); 3) conserved bonding including disulfide bridges (one in IFNβ, two each in IFNω and all IFN subtypes) and a network of hydrogen bonds to form and stabilize the tertiary structure; 4) IFNAR2 binding residues in Helix A, the AB loop and Helix E, and IFNAR1 binding sites spaced among helices B–D and the CD loop (21).
All IFN-I signal through a heterodimeric receptor that is comprised of two subunits, IFNAR1 and IFNAR2. In the classical model of IFN signaling, IFN first binds IFNAR2 forming a high-affinity binary complex which then recruits IFNAR1 to form a functional ternary structure that triggers phosphorylation of Jak1 and Tyk2-initiating “canonical” signaling (24). In canonical IFN-I signaling (Figure 2), activation of Jak1 and Tyk2 is followed by phosphorylation of STAT1 and STAT2, which trimerize with IRF9 to form the transcription factor interferon-stimulated growth factor-3 (ISGF3) (25). Once assembled, ISGF3 translocates to the nucleus and binds to interferon stimulated response elements (ISRE) to promote transcription of interferon stimulated genes (ISGs). Through this canonical pathway, many genes are highly susceptible to shifts in expression with small amounts of IFN-I, thus earning the label of “robust” ISGs (26). Robust ISGs include most antiviral effectors from which the name “interferon” was derived.
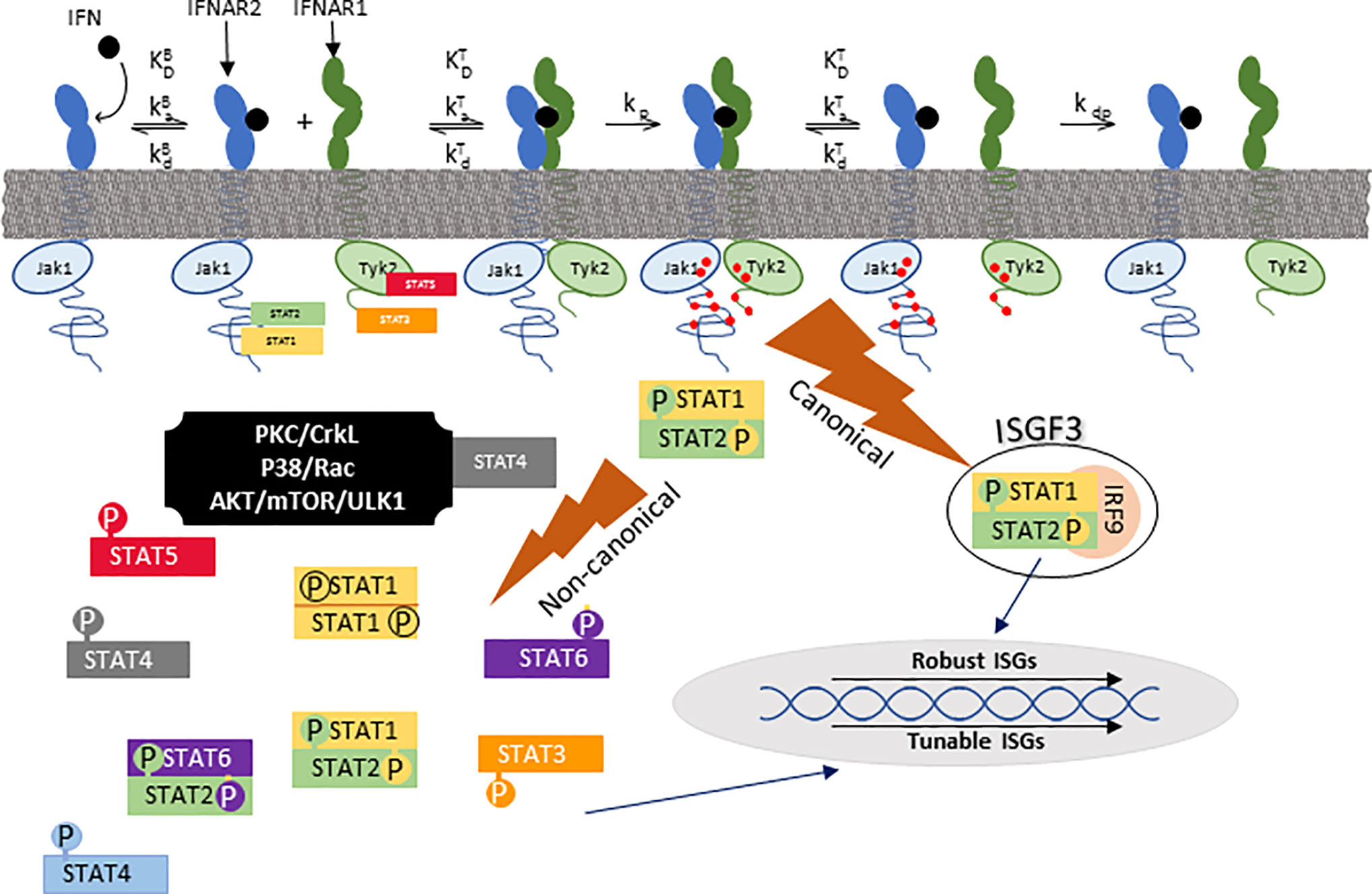
Figure 2 Canonical and noncanonical IFN signaling. IFN first binds to IFNAR2 after which the IFN/IFNAR2 binary complex recruits IFNAR1 to form a functional ternary signaling complex (IFN/IFNAR1/IFNAR2). Following that, Jak1 and Tyk2 kinases, which are pre-associated with IFNAR2 and IFNAR1 respectively, phosphorylate each other and tyrosine residues on each receptor (red dots) upon which STAT (signal transducers and activators of transcription) family members dock. Canonical signaling consists of a trimer of pSTAT1, pSTAT2, and IRF9 which is referred to as ISGF3 (interferon-stimulated gene factor 3). ISGF3 translocates to the nucleus to bind ISRE (interferon-stimulated response elements) to stimulate transcription of robust ISGs. There are many non-canonical signaling pathways, one of which is formation of phosphorylated STAT1 homodimers that bind to GAS (gamma activation site) promoter elements. ka and kd are association and disassociation rates, respectively. KD is the equilibrium disassociation constant (kd/ka). kp and kdp are rates of phosphorylation and dephosphorylation, respectively. KB and KT refer to binary (IFN/IFNAR2) and ternary (IFN/IFNAR2/IFNAR1) complexes, respectively. This figure was adapted from Figure 1 of (24).
Non-canonical IFN-I signaling includes cell-specific pathways such as those mediated by STAT1 homodimerization, other STAT family members, and MAP- or PI3-kinases (Figure 2). To better characterize these pathways, Urin and colleagues used HeLa cell signaling-component deletion mutants to show that except for the formation of STAT1 homodimers or STAT2/IRF9 heterodimers, non-canonical signaling depends on phosphorylation of both STAT1 and STAT2 (27). For the most part, non-canonical signaling induces “tunable” ISGs (26), which exhibit gradual rather than steep dose-response curves, and higher IFN concentrations for peak expression (26). Non-canonical pathways such as suppression of cell proliferation best correlates with the stability of the IFN/IFNAR1/IFNAR2 ternary complex [defined as (IFN-I KD IFNAR1* IFN-I KD IFNAR2)] (24). Non-canonical signaling also mediates expression of chemokines and cytokines that modulate innate or adaptive immunity, transcription factors that modulate cell phenotype, and some antiviral responses. As examples, APOBEC3, a cytidine deaminase that blocks HIV replication in macrophages, and IRF1, a transcription factor that mediates IFN-dependent and -independent viral immunity (28–31), share characteristics of tunable ISGs. While IFNAR2-independent signaling has been reported in mice (32), there are no data to controvert the current model that both IFNAR1 and IFNAR2 are necessary for signaling in humans.
Why there are so many IFN-I genes, and specifically so many IFNα subtypes, remains a mystery. As would be predicted by their common use of a shared receptor, evidence to date points to quantitative rather than qualitative differences among the IFN-I. In other words, differences in gene expression, antiviral, or antiproliferative activity at subsaturation are equalized by dose adjustments or in the extreme, by receptor saturation. Thus, while their evolutionary history and expression patterns suggest that at least some IFN-I serve specific functions, very few have been defined. Here, we focus on differences among IFNβ and the IFNα subtypes to propose a model by which patterns of expression mirror their evolutionary history, and thus provide an alternative approach toward deciphering their roles in human biology.
Evolution of Type I Interferons
Types I and III IFNs evolved from a common ancestor gene that shares the 5-exon/4-intron organizational structure of the IL-10 family of cytokines. The intronless IFN-I genes of all higher order primates evolved and diversified from those of cartilaginous and bony fish. As shown in Figure 3A, IFNκ was the first to evolve from the “most recent common ancestor” (MRCA), followed by IFNβ. Both were present ~200 million years ago (MYA) before eutherians and marsupials diverged. IFNε arose from IFNβ, which later duplicated to give rise to IFNω and the IFNα genes (15). Primate IFN-I are highly divergent from other mammals. For example, in bats and ungulates, IFNω emerged as a multigene subtype (33) while primates have one functional IFNω gene and multiple IFNα subtypes.
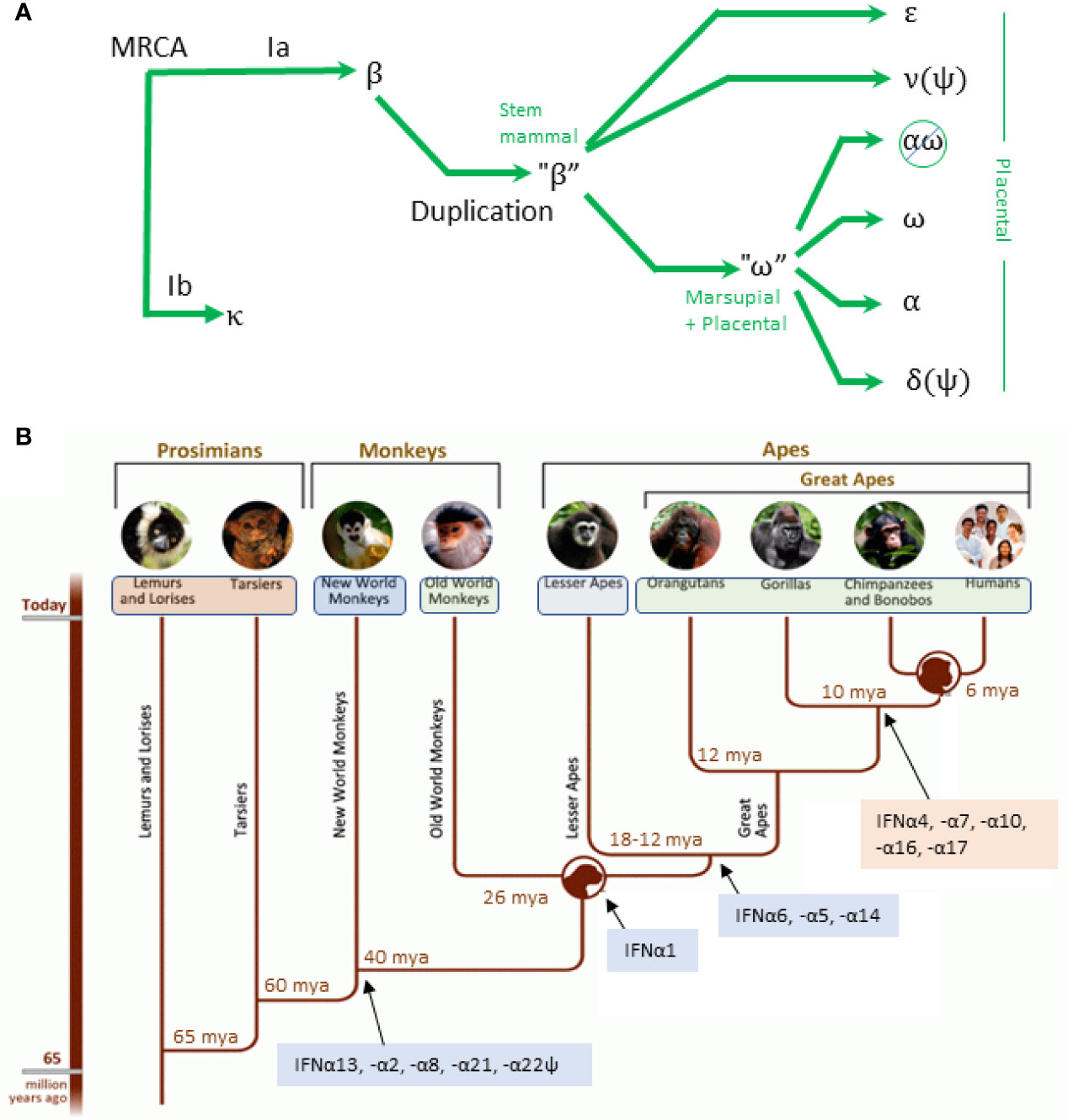
Figure 3 Evolution of IFN-I. (A) Simplified evolution of type IFN-I in mammals adapted from Krause and Petska. The most recent common ancestor (MRCA) gave rise to IFNκ and a progenitor for IFNβ. A duplicate of the IFNβ progenitor gave rise to IFNϵ, IFNν (a pseudogene in mammals), and a progenitor for IFNω. The IFNω progenitor gave rise to the remaining subtypes. In simiiforms, IFNαω is deleted and IFNδ is a pseudogene. (B) Evolution of IFN subtypes from simians to homininae showing conserved (blue) and variant (orange) subtypes. Figure adapted from: http://humanorigins.si.edu/evidence/genetics.
The first IFNA gene appeared 95–105 MYA, which through duplication and conversion gave rise to an expanded set of IFNα subtypes in a subset of placental mammals (15). IFNA gene duplication and conversion that occurred before speciation gave rise to a conserved cluster of IFNα subtypes that are dissimilar, but that are shared across species. Conversely, duplication after speciation gave rise to variant clusters that are highly similar within each species but are not shared across species. As shown in Figure 3B, the first IFNα subtypes that are present in humans and simiiforms—IFNA13, -A2, -A8, and -A21—were present before the divergence of new world and old world monkeys (NWM and OWM) 65-47 MYA. NWM have one gene each for IFNA13 (syntenic with IFNA13 in monkeys and apes), IFNA2 and IFNA21, and two genes each that are similar to IFNA8 and IFNA5 in higher order primates. Subsequently, IFNA13 duplicated to give rise to IFNA1 (present in OWM and apes), and IFNA5, IFNA6, and IFNA14 arose to complete the set of IFNA subtypes that are conserved during primate evolution (Figure 3B, blue background). The subset of human IFNA subtypes that are variant among primates (pink background) arose after orangutans and the other great apes diverged. It has been proposed that IFNA4, IFNA10, IFNA17 are products of partial conversions from IFNA14 or IFNA21 (IFNA4, -A10, and -A17) (15) and that IFNA10 may have converted IFNA7 or vice versa (34).
Based upon a detailed analysis of human polymorphisms in sub-Saharan African, Asian, and European populations, Manry et al. (35) found the fewest polymorphisms in IFNA6, -A8, -A13, and -A14. Exclusion of IFNA1 from this group appeared to be based on the A137V substitution (residue 114 of the mature peptide), that is predicted to have no damaging effects, and in our experience, is not functionally different from A137 IFNα1 (36). Manry et al. concluded that these evolutionarily conserved subtypes have have undergone selection against nonsynonymous variants. Taken together, the conserved cluster may have evolved to counter pathogens common that threatened the MRCA to OWM and great apes, and there is a selective advantage for having two genes, IFNA1 and IFNA13, that express IFNα1.
Regulation of Type I Interferon Expression by IRF3 and IRF7
Comparing promoter regions and transcription factor usage provides insight toward specialized roles for the different IFN-I. The interferon regulatory factor (IRF) family members are the dominant transcription factors that regulate IFN-I expression. While IRF1, -2, -5, and -8 have been shown to regulate IFN-I expression, this review will focus on the two most important members, IRF3 and IRF7.
IFNβ is expressed after stimulation of pattern-recognition receptors (PRRs) such as RIG-I-like receptors (RLRs) and toll-like receptors (TLRs) by pathogen-specific molecular motifs referred to as pathogen associated molecular patterns (PAMPs) [reviewed in (37)]. Once activated, PRRs trigger signaling cascades that activate assembly of the “enhanceosome,” which consists of the transcription factors ATF-2/c-Jun, NFκB (p50/65 heterodimer) and two interferon response factor (IRF) dimers [Figure 4 (39)] that bind to four promoter regulatory domains (PRDs). Based primarily on mouse models, it was initially thought that PRDs III and I required either IRF7 homodimers or IRF3/IRF7 heterodimers for a functional enhanceosome (40). In most cells, however, basal IRF7 expression is low while IRF3 is ubiquitously expressed. Thus, in most cells, viral PAMPs trigger activation of IRF3, which homodimerizes to complete the functional enhanceosome and initiate transcription of IFNβ. Subsequently, autocrine/paracrine IFNβ increases expression of IRF7 (a robust ISG) in infected and bystander cells—a well-documented critical step in a forward feedback loop for IFNβ to enhance its own expression (41).
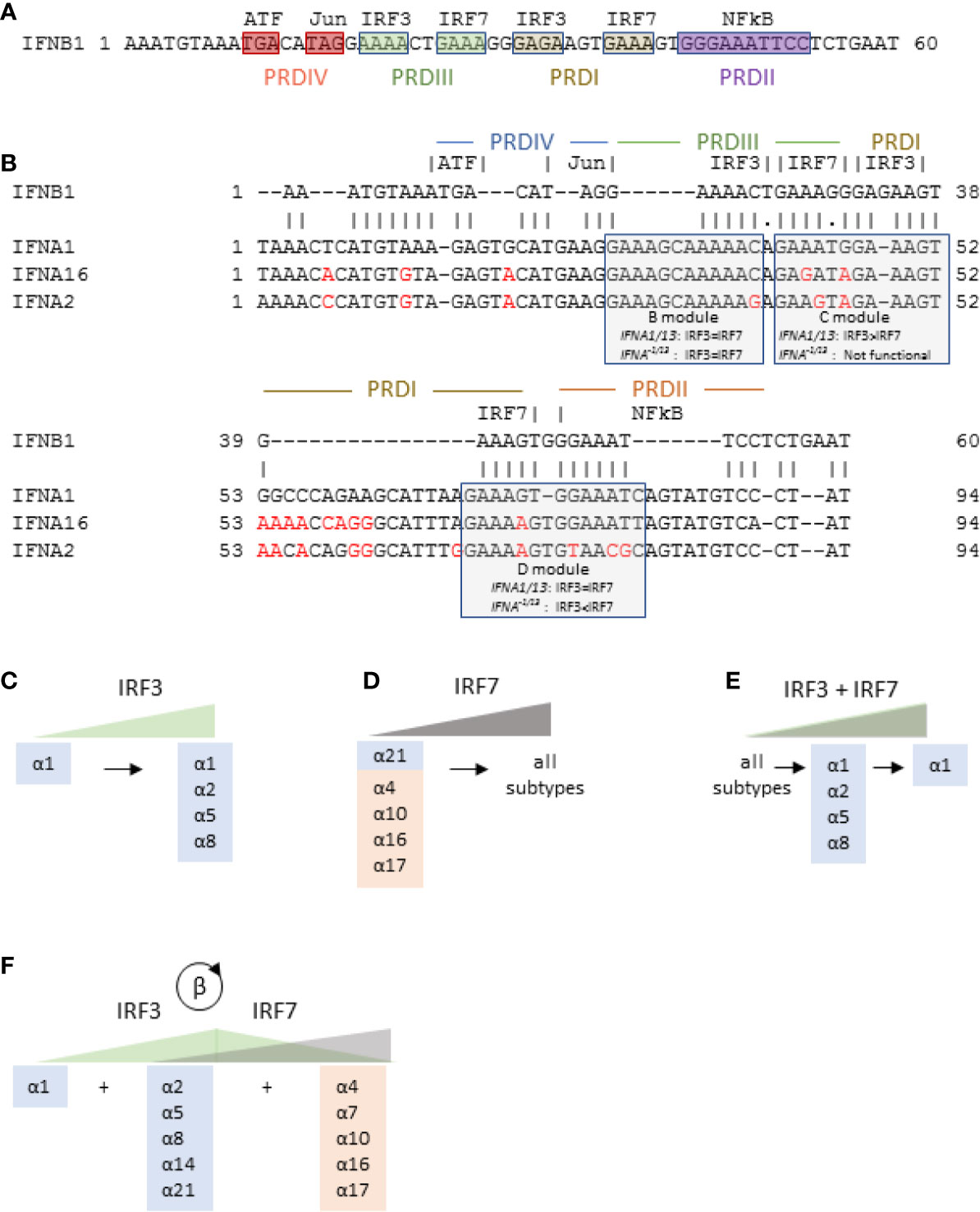
Figure 4 IFNB1 and IFNA gene transcription is controlled by IRF3 and IRF7. (A) Promoter region of IFNB1 gene showing the four promoter regulatory domains (PRD), all of which must be engaged for gene transcription. (B) Promoter regions of IFNA1, IFNA16, and IFNA2 aligned with the promoter region of IFNB1 showing the three IRF regulatory modules and their relative sensitivity to IRF3 and IRF7. Differences from IFNA1 promoter are shown in red. The promoter region of IFNA16 is representative of IFNA21 and the variant subtypes (IFNA17, IFNA16, IFNA10, IFNA7, and IFNA4). The IFNA2 promoter region is representative of all IFNA−1/13 conserved subtypes except IFNA21. (C–E) Model of differential regulation of human IFNA genes. Blue and orange shading show evolutionarily conserved and variant IFNα subtypes, respectively, IFNA genes expressed in response to increasing levels of activated IRF3 alone (C), IRF7 alone (D), or IRF3 and IRF7 together (E) as described by Genin et al. (38). (F) Proposed model of IFNα subtype expression in the context of initial activation of IRF3 followed by IRF7 expression (and subsequent activation) in response to a forward feedback loop initiated by IFNβ.
The critical importance of IRF3 toward initiating IFN expression is emphasized by the number of pathogens with gene products that antagonize its activation (41) and by reports that cells from IRF3-deficient patients express little or no IFNβ (42, 43). The critical importance of the IRF7-mediated forward feedback loop is supported by an in vitro study in which the percentage of IFNβ-expressing cells after viral infection was dependent on cell density, and secretion of IFNβ (44), and reports that IRF7 deficient patients poorly express IFNβ (45, 46). By contrast, cells that constitutively express IRF7, as is the case for macrophages and plasmacytoid dendritic cells (pDC) (47) highly express IFN-I in response to synthetic ligands (imiquimod or CpG oligonucleotides) or pathogens such as influenza (48, 49). Taken together, the IFNβ-IRF7 forward feedback loop is a sentinel at the early stages of viral infection in local environments that enhances the antiviral state of common target cells for viral infection such as respiratory or gastrointestinal epithelium.
After the crystal structure of the IFNB1 enhanceosome was published, Genin et al. described promoter regions of the IFNA genes (38) and modulated cellular expression of IRF3 and IRF7 to determine their effects on IFNα subtype expression. Figure 4A shows the promoter region of IFNB1, and Figure 4B shows the IFNB1 promoter region aligned to representative IFNA subtypes up to −30 bp from the transcription start site. Overall, the IFNA promoter regions align well to that of IFNB1 with 95% identity excluding several insertions and three short deletions. As shown in Figure 4B, the insertions into the IFNA promoters shift the IRF binding sites, referred to as modules B, C, and D, 5’ from the transcriptional start site such that the B module ends half-way through IFNB1 PRDIII, the IFNA C module straddles IFNB1 PRDIII and PRDI, and the IFNA D module straddles IFNB1 PRDI and PRDII (to which NFκB binds in the IFNB1 promoter). Among the three modules, only module B, which is equally responsive to IRF3 and IRF7, is essentially identical among all the subtypes. By contrast, module C, which preferentially binds to IRF3, is functional only in the IFNA1 (and IFNA13) promoter. Module D also differs between IFNA1/13 and the other subtypes. For IFNA1, module D binds equally to IRF3 and IRF7, while for all the other IFNA subtypes, module D preferentially binds to IRF7. Binding of IRF3 to IFNA1 promoter modules C and D explains why IFNA1 and IFNB1 can be co-expressed in the absence of any other IFNA subtypes (38, 49–51).
The promoter regions of the IFNA subtypes other than IFNA1 (which we will refer to as IFNA−1/13 or IFNα−1/13 for the gene and protein, respectively) cluster into two groups. The first cluster consists of IFNA4, -A7, -A10, -A16, -A17, and -A21, (represented by IFNA16 in Figure 4B). Note that this set includes all the evolutionarily variant IFNA subtypes (15) along with IFNA21, from which the variant subtypes may have arisen. The substitutions in the C modules of these subtypes renders them nonfunctional, and the 73G/A substitution in their D modules renders them more sensitive to IRF7. The B, C, and D modules are identical among the IFNA subtypes in this cluster.
The second cluster of IFNA−1/13 subtypes is represented by IFNA2 and includes IFNA5, -A6, -A8, and -A14. These are all evolutionarily conserved subtypes. The C module for this cluster is also non-functional, and their D modules include the 73G/A substitution that renders them more sensitive to IRF7. Unlike the cluster represented by IFNA16, however, there are substitutions in the B and D modules that may affect their relative sensitivity to IRF3 and IRF7 (52).
Based on analysis of the IFNA promoter regions and expression studies with EBV-transformed B cells, Genin et al. proposed a model for differential regulation of the IFNA genes by either activation of IRF3 or IRF7, or by co-activation of both IRF3 and IRF7 Genin, 2009 #71} (15, 52). In this model, low activation of IRF3 is sufficient to induce expression of IFNα1, while increased IRF3 activation may induce expression of IFNα2, -α5, and -α8 (Figure 4C). Similarly, increasing levels of IRF7 activation will first induce expression of IFNα21 and the evolutionarily variant subtypes followed by the remaining subtypes (Figure 4D). Co-activation of IRF3 and IRF7 at low levels induces expression of all subtypes, but coactivation increases, IRF3 inhibits IRF7 and thus limits the number of subtypes expressed (Figure 4E).
Patterns of Human Type I Interferon Expression in Response to Synthetic Ligands and Viral Infection
To characterize expression patterns of IFNα subtypes in response to synthetic ligands or viral infection, transcripts are usually measured with RT-qPCR. Table 1 summarizes human IFNβ and IFNα subtype expression patterns reported in the literature. As predicted by Genin et al., IFNα1 is co-expressed with IFNβ after activation of IRF3 with poly I:C. Additionally, when potently stimulated, pDC (which constitutively express IRF7) express all IFNα subtypes, while weaker stimulation of IRF7 with CpG B class oligodeoxynucleotides (ODN) induced expression of a set of IFNα subtypes that share the IRF7-sensitive promoter region exemplified by IFNA16 (Figure 4B). By contrast, stimulation of cells that do not constitutively express IRF7 with viral RNA or the synthetic analog poly I:C primarily induces expression of a core set of conserved subtypes. Table 1 also suggests the possibility that specific pathogens such as influenza virus, HIV, or hepatitis C may preferentially induce IFNα5.
Of particular interest is the report by Zaritsky et al., who infected the U937 histiocytic cell line with Sendai virus at low and high multiplicity of infections (MOI). While the U937 cells expressed all IFNα subtypes after infection at low MOI, expression was limited almost exclusively to the conserved set after infection at a high MOI. Furthermore, while IFNAR2 blockade (which repressed the IFNβ-IRF7 forward feedback loop) did not affect the expression pattern in the high MOI infection, it significantly repressed all subtypes except IFNα1, -α2, and -α8 after low MOI infection (60). Taken together, these studies support the model of Genin et al. in which activated IRF3 alone induces expression of conserved IFNα subtypes (Figure 4C), and IRF7 alone first induces IFNα21 and variant subtypes and subsequently induces expression of all subtypes (Figure 4D). In the context of the IFNβ-IRF7 forward feedback loop, however, Table 1 suggests that conserved subtypes are first expressed, followed by variant subtypes (Figure 4F).
It is important to note that the evolutionarily conserved or variant IFNα subtype clusters are not expressed en bloc. One possible explanation is that unlike the variant subtypes, the B and D promoter modules vary by one or two bp, which may affect their relative sensitivity to IRF3 or IRF7 (52). Another factor is that IRF3 and IRF7 are not the only mediators of subtype expression. For example, a set of IFNA transcripts is regulated by a competing endogenous RNA (ceRNA) network. Kimura and colleagues first described stabilization of IFNA1 transcripts by a natural antisense transcript (NAT) that spans the coding region and extends well beyond the 3’ poly-A UTR (61). They subsequently determined that the IFNA1 NAT includes binding sites for microRNA-1270 (i.e., a microRNA response element) which otherwise represses IFNA1 transcript levels. Additionally, NAT for IFNA8, -A10, -A14, and -A17 (Kimura et al., personal communication) also sequester miRNA-1270 to enhance their transcript levels (62).
IFNβ, the High-Affinity Sentinel
In addition to its evolutionary emergence as the first non-tissue specific IFN-I and its high sensitivity to IRF3/IRF7, IFNβ also has exceptionally high affinities for IFNAR1 and IFNAR2 (KD = 0.1 uM and 0.1 nM, respectively). As estimated by the product of IFNAR1 and IFNAR2 affinities (KD IFNAR1 * KD IFNAR2), the stability of the IFNβ/IFNAR1/IFNAR2 ternary complex is 10-fold higher than for IFNω and at least 50-fold higher than the highest affinity IFNα subtypes, IFNα14 and IFNα6 (Figure 5). As reviewed elsewhere in this series, a consequence of its high affinity is more effective internalization of ternary receptor complexes (66) into early endosomes where signaling may be amplified and prolonged, or more rapidly terminated due to shuttling of IFNAR1 to proteasomes for degradation (67).
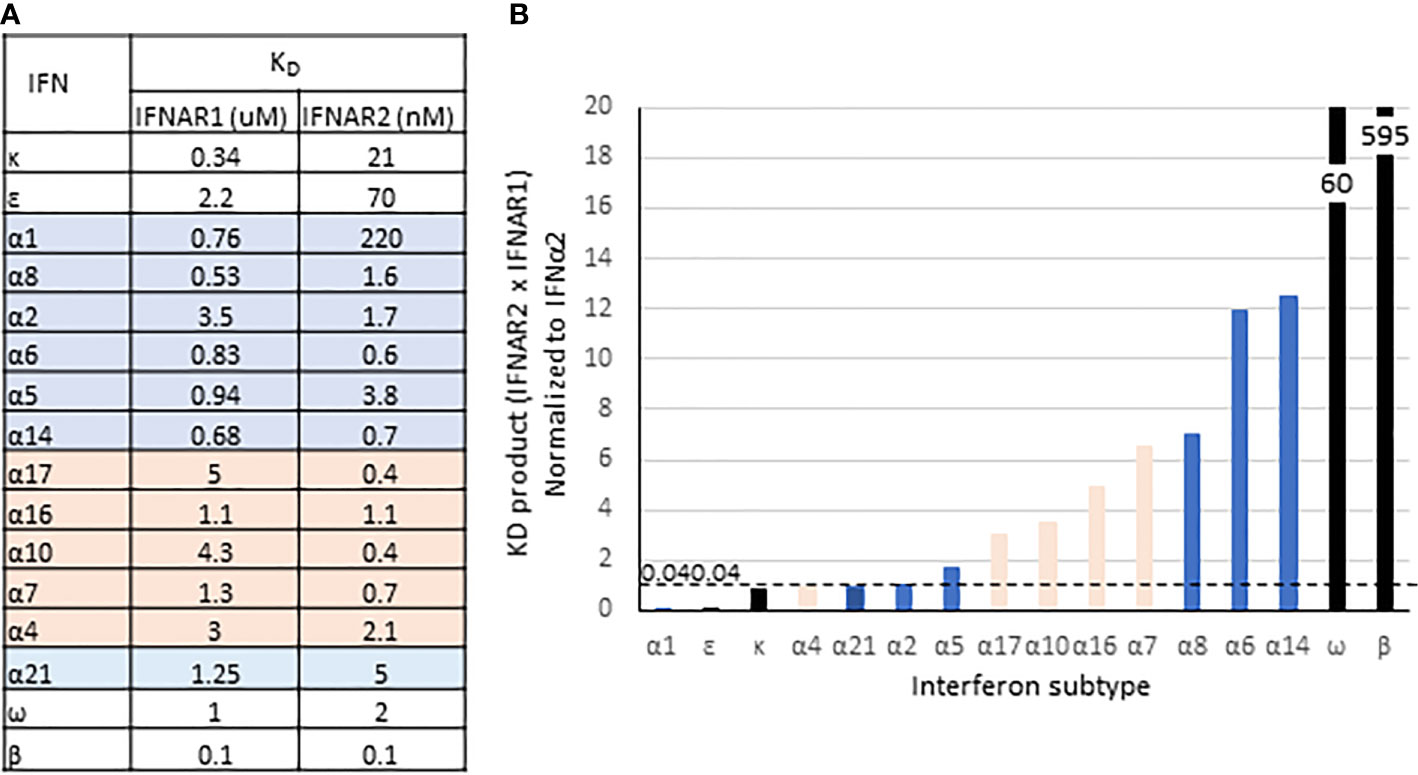
Figure 5 Binding affinities of IFN-I. (A) Equilibrium disassociation constants for the IFN-I. IFNα subtypes are from (63); IFNβ from (64), and IFNϵ, -κ, and -ω from (65). (B) Product of KD for IFNAR1 and IFNAR2, normalized to IFNα2. Highlighting and bar colors indicate conserved (blue) and variant subtypes (orange).
A second consequence of the high affinity that IFNβ has for the receptor is that unlike the other IFN-Is, signaling is unaffected by ubiquitin-specific protease-18 (USP18). USP18 is a deubiquitinating enzyme that deconjugates the ubiquitin-like interferon-stimulated gene-15 (ISG15) from its target proteins (68). Conversely, ISG15 prevents ubiquitination and proteolytic degradation of USP18, thus stabilizing its expression (69). Unrelated to its enzymatic function, USP18 is shuttled by STAT2 to IFNAR2, which sterically blocks binding of Jak2 to interfere with recruitment of IFNAR1 to assemble a stable ternary complex (68, 70, 71). Since USP18 is an ISG (72), this inhibitory function is considered a negative feedback regulator of IFN signaling. Due to its very high affinity for IFNAR1, however, IFNβ can override USP18 and recruit IFNAR1 to form a ternary complex to initiate signaling (70). Thus, the negative feedback regulation by USP18 is selective and is presumed to affect all IFN-I other than IFNβ. To our knowledge, selective inhibition has been demonstrated by comparing IFNβ induced signaling with that of IFNα2, but not higher affinity IFNAR1 ligands such as IFNα6 or -α8, or those with higher IFNAR1 × IFNAR2 KD products such as IFNω or IFNα14 (Figure 5). The critical importance of USP18-mediated inhibition of IFN signaling is exemplified by pseudo-TORCH syndrome, a severely incapacitating or fatal “interferonopathy” in patients deficient in ISG15, USP18, or with a mutation to the STAT2 binding site for USP18 (69, 73, 74).
Two additional qualities of IFNβ bear discussion. First, in addition to IFNκ (9), IFNβ also binds to highly sulfated proteoglycans (PG), proposed to be mediated through a heparin binding site in an arginine-rich region of IFNβ that spatially separates the binding sites for IFNAR1 and IFNAR2 (75). PG binding of IFNβ may sequester it to buffer IFN-I signaling, which can be reversed by desulfation or shedding the IFNβ-bound PG (75) which may result in a depot effect. Second, amino acid residues 25-27 uniquely contain the sequence motif NGR which binds CD13. Asparagine residues undergo spontaneous deamidation, which may be increased during oxidative conditions. Deaminated NGR gives rise to DGR, which binds to αVβ3 and possibly other integrins that similar to CD13, are expressed in blood vessels during angiogenesis (76) and by some cancer stem cells (77) and mediates tumor invasion (78). It is proposed that CD13 or αVβ3 in tumors or tumor vasculature may sequester IFNβ and thus limit its antiproliferative effects (79). In addition to these biologic effects, binding of IFNβ to abundant PG and integrins (in addition to its propensity to stick to plastic) may limit its detection in biological fluids or tissue culture supernatants.
IFNα1, the Low-Affinity Subtype
As discussed above, IFNα1 stands apart from the IFNα−1/13 subtypes for its responsiveness to IRF3, for having two genes (IFNA1 and IFNA13) on chromosome 9, and for the low frequency of polymorphisms in either of those genes. Most remarkable, however, is the low affinity of IFNα1 for IFNAR2, at least 100-fold lower than most other IFNα subtypes while it binds with relatively high affinity to IFNAR1 (Figure 5). Figure 6 shows the protein sequences of the IFNα subtypes aligned to IFNα1, with secondary structures and receptor contact points. Residues 20-35 cover most of the AB loop, including two 310 helices. In this span, two substitutions in IFNα1 contribute to the low affinity of IFNα1 for IFNAR2: F27S, which decreases its affinity for IFNAR2 by 4-fold as the polar side chain of serine is predicted to disrupt the hydrophobic interaction otherwise stabilized by phenylalanine (80), and R22S, which together with S27 decreases affinity by ~14-fold (65). Although not a contact point, the substitution K31M in IFNα1 may also contribute to its decreased affinity for IFNAR2 by disrupting the second 310 helix.
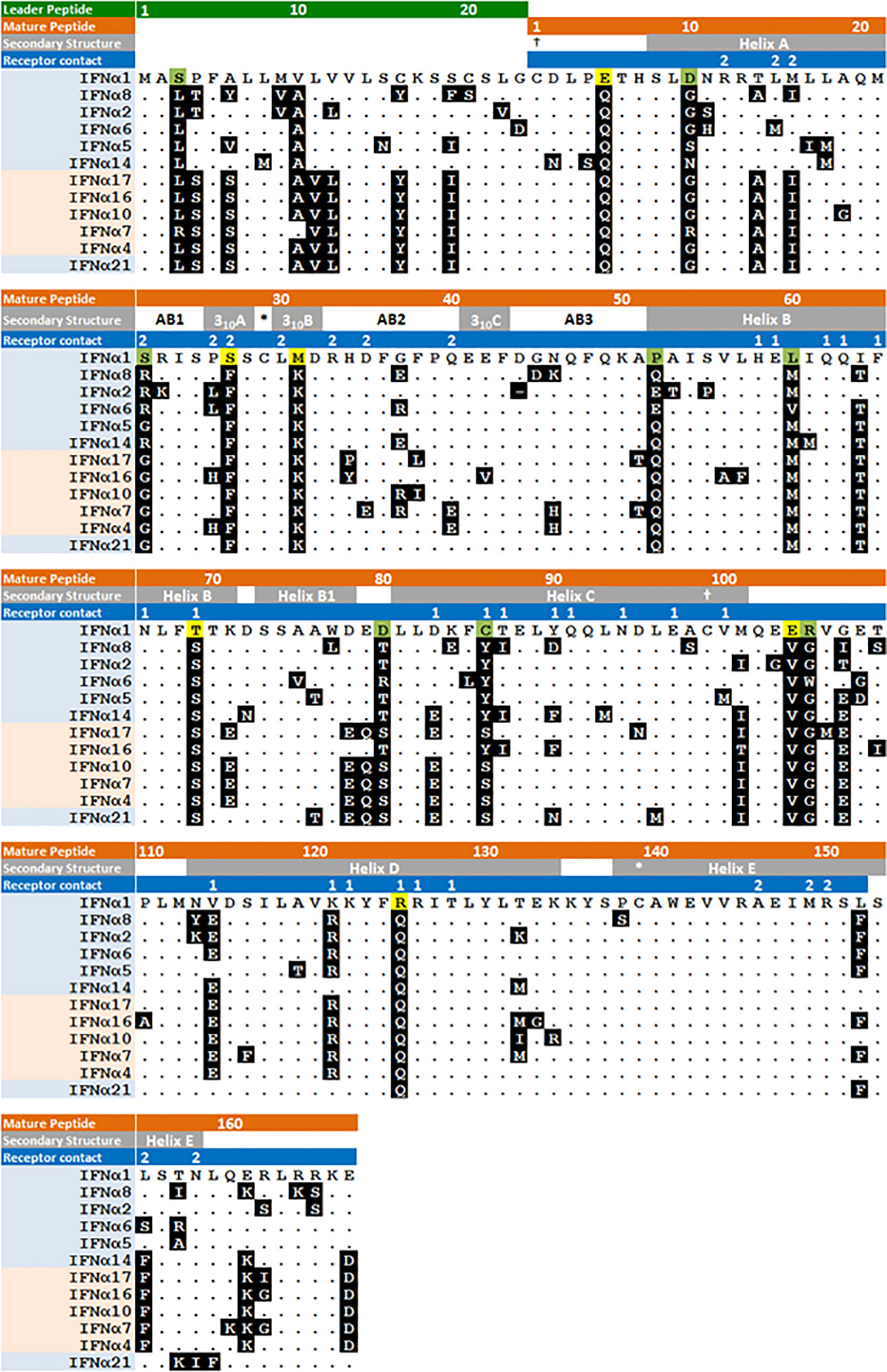
Figure 6 Amino acid sequence of human IFNα subtypes. IFNα subtypes are shown in order of arrangement on chromosome 9 with evolutionarily conserved and variant subtypes highlighted in blue and pink respectively. Secondary structure and IFNAR1/2 contact residues, labeled 1 and 2 respectively, are shown in the gray and blue highlighted text. Amino acids are shown with IFNα1 as the comparator, showing those that are unique to IFNα1 and otherwise identical among all the IFNα−1/13 subtypes, or otherwise varies among the other IFNα1/13 subtypes. * and † indicate cysteine disulfide bonds. Figure modified from (80).
While the low affinity of IFNα1 for IFNAR2 suggests the possibility of a qualitative difference in signaling or functional outcome, the evidence to date only supports a quantitative difference. Reports of IFNAR2-independent signaling in mice (32) have not been replicated in human cells, for which it has been reported that both IFNAR1 and IFNAR2 are essential for signaling and gene expression (27). Additionally, while IFNα1 also has unique substitutions at contact points for IFNAR1 that may affect its conformation at the SD2-SD3 hinge that affect binding affinity (81), conformational changes do not necessarily indicate an effect in IFN signaling (25).
The substitutions that decrease the affinity of IFNα1 for IFNAR2 also decrease its affinity for B18R, a soluble receptor antagonist encoded by vaccinia virus. According to this model, secreted B18R (or other poxvirus orthologues) block high affinity IFN-I from binding their receptors, while leaving these low affinity IFNs relatively unaffected (65). Similarly, the organ-specific IFN-I, IFNκ, and IFNϵ also bind to IFNAR2 and B18R with low affinity. While IFNκ and IFNϵ may protect against poxviruses that infect local environments (skin and female reproductive tract), IFNα1 may defend against invasive strains such as variola. It is intriguing to speculate that the low frequency of polymorphisms in human IFNA1 and IFNA13 (35) is a consequence of a selective advantage toward surviving smallpox.
Among the IFNα−1/13 subtypes, there are fewer substantial differences in their peptide sequences. Figure 6 shows the shared residues that account for the high levels of identity among the evolutionarily conserved subtypes (Supplementary Figure 1) and differences in the unstructured C-terminal tail that contribute to higher antiviral and antiproliferative potencies of IFNα8 (82). Since the receptor contact points are conserved, variation in their binding affinities is apparently due to substitutions in adjacent residues.
Therapeutic Uses of Type I Interferon
The antiviral and antiproliferative activities of interferons led to the development of their use as therapeutics. In 1986, IFNα2b (Intron A®, Merck Sharp & Dohme) was the first IFN-I approved for use in the United States (83). The current U.S. market for interferons, including IFNγ for chronic granulomatous disease and malignant osteopetrosis, has grown to $5B per year. Table 2 shows the nine IFN-I licensed in the United States along with indications for use. As discussed elsewhere in this series of reviews (84), there are several ongoing clinical studies to test efficacy of IFN-I and IFN-III to treat Covid19.
IFNα2a or IFNα2b, which differ only at residue 23 (lysine or arginine, respectively), are prescribed for their antiviral or antiproliferative activity. These products are injectable preparations of either native or pegylated IFN proteins. Pegylation is modification of proteins with linear or branched polyethylene glycol to retards degradation and increase its serum half-life (85). While IFNα2 was used to treat chronic hepatitis C, it has been replaced with the highly specific inhibitors of HCV NS3/4A, NS5A, and NS5B proteins, which may be curative and are associated with fewer adverse events (86).
IFNβ was first approved for treatment of relapsing remitting multiple sclerosis in 1993 after showing an 18-34% reduction in relapse rate. The efficacy for IFNβ was considered to be due suppression of viral infections that are associated with relapses and to direct immunomodulatory effects that include reduction of pathogenic Th1 and Th17 CD4+ T cells, and to increases in IL-10 producing Treg cells (87). All these may be mediated by increased expression of PD-L1 (CD274), an ISG that in mice is more responsive to IFNβ due to its high receptor affinity (88).
Therapeutic IFN-I has severe adverse events that are an obstacle to their use as therapeutics. The package inserts for pegylated IFNα includes black box warnings for the potential development of neuropsychiatric, autoimmune, ischemic, or infectious disorders. The package inserts also warn that treatment symptoms such as fever, fatigue, headache, myalgia, and nausea, which are usually associated with viral infections, are common side effects. More serious side effects can include cardiovascular and neurologic disorders, bone marrow, hepatic, and renal toxicity, and hypersensitivity reactions. Additionally, IFNβ for MS is associated with seizures, depression, suicide, and other psychiatric disorders. It is therefore not too surprising that as more selective therapeutic agents have been developed and licensed, use of IFN-I has become adjunctive rather than a primary treatment for chronic viral infections, cancer, or MS.
Conclusions
As reviewed here, most if not all reported biological differences among IFN-I are quantitative rather than qualitative. While the antiviral subtype that most potently neutralizes infection in vitro may vary according to pathogen (57, 59, 89, 90), these differences may be overcome by increasing doses (57, 91). Similarly, differences in antiproliferative activity are largely dose dependent (92). While this may also be true for modulation of cytokine expression (36), immunosuppressive activity (i.e., induction of PD-L1) may be dependent on the exceptionally high affinity of IFNβ for IFNAR1/2.
As for the IFNα subtypes, other than escape from poxvirus soluble receptor antagonists (such as B18R by IFNα1), any suggestion of specialized roles is inferred from their evolutionary history or expression patterns. It is therefore possible that the primary role of IFNα is to prolong or amplify the effects of IFNβ and that multiple IFNα subtypes simply provide multiple layers of redundancy, albeit with a range of receptor affinities. However, it is also possible that unique functions for IFNα subtypes have not been revealed because the common experimental approach of comparing treatment with individual IFN-I does not reflect the biological context in which defined patterns of IFNα are co-expressed together and with with IFNβ. These patterns are likely most relevant at sub-saturating doses, which may more accurately reflect the environment of structural cells where organ specific immune responses are initiated (93).
Author Contributions
MW, SC, EL, and RR performed the literature searches and contributed to draft versions of the manuscript. RR wrote and revised the final version of the manuscript. All authors contributed to the article and approved the submitted version.
Funding
This study was supported by the Internal funds, US Food and Drug Administration. MW and SC were supported by an appointment to the Research Participation Program at the Center for Biologics Evaluation and Research administered by the Oak Ridge Institute for Science Education through an Interagency agreement between the U.S. Department of Energy and the U.S. Food and Drug Administration.
Disclaimer
This article is an informal communication that represents the authors’ judgment. These comments do not bind or obligate FDA.
Conflict of Interest
The authors declare that the research was conducted in the absence of any commercial or financial relationships that could be construed as a potential conflict of interest.
Acknowledgments
The authors wish to thank Philippa Hillyer for critical review of the manuscript.
Supplementary Material
The Supplementary Material for this article can be found online at: https://www.frontiersin.org/articles/10.3389/fimmu.2020.605673/full#supplementary-material
References
1. Isaacs A, Lindenmann J. Virus interference. I. The interferon. Proc R Soc Lond B Biol Sci (1957) 147:258–67. doi: 10.1098/rspb.1957.0048
2. Pestka S, Langer JA, Zoon KC, Samuel CE. Interferons and their actions. Annu Rev Biochem (1987) 56:727–77. doi: 10.1146/annurev.bi.56.070187.003455
3. Freaney JE, Zhang Q, Yigit E, Kim R, Widom J, Wang J-P, et al. High-density nucleosome occupancy map of human chromosome 9p21-22 reveals chromatin organization of the type I interferon gene cluster. J Interferon Cytokine Res (2014) 34:676–85. doi: 10.1089/jir.2013.0118
4. Fung KY, Mangan NE, Cumming H, Horvat JC, Mayall JR, Stifter SA, et al. Interferon-epsilon protects the female reproductive tract from viral and bacterial infection. Science (2013) 339:1088–92. doi: 10.1126/science.1233321
5. LaFleur DW, Nardelli B, Tsareva T, Mather D, Feng P, Semenuk M, et al. Interferon-kappa, a novel type I interferon expressed in human keratinocytes. J Biol Chem (2001) 276:39765–71. doi: 10.1074/jbc.M102502200
6. Li Y, Song Y, Zhu L, Wang X, Richers B, Leung DYM, et al. Interferon Kappa Is Important for Keratinocyte Host Defense against Herpes Simplex Virus-1. J Immunol Res (2020) 2020:5084682. doi: 10.1155/2020/5084682
7. Woodby BL, Songock WK, Scott ML, Raikhy G, Bodily JM. Induction of Interferon Kappa in Human Papillomavirus 16 Infection by Transforming Growth Factor Beta-Induced Promoter Demethylation. J Virol (2018) 92(8):e01714–17. doi: 10.1128/JVI.01714-17
8. Sarkar MK, Hile GA, Tsoi LC, Xing X, Liu J, Liang Y, et al. Photosensitivity and type I IFN responses in cutaneous lupus are driven by epidermal-derived interferon kappa. Ann Rheum Dis (2018) 77:1653–64. doi: 10.1136/annrheumdis-2018-213197
9. Nardelli B, Zaritskaya L, Semenuk M, Cho YH, LaFleur DW, Shah D, et al. Regulatory effect of IFN-kappa, a novel type I IFN, on cytokine production by cells of the innate immune system. J Immunol (2002) 169:4822–30. doi: 10.4049/jimmunol.169.9.4822
10. Klein K, Habiger C, Iftner T, Stubenrauch F. A TGF-beta- and p63-Responsive Enhancer Regulates IFN-kappa Expression in Human Keratinocytes. J Immunol (2020) 204:1825–35. doi: 10.4049/jimmunol.1901178
11. Yang LM, Xue QH, Sun L, Zhu YP, Liu WJ. Cloning and characterization of a novel feline IFN-omega. J Interferon Cytokine Res (2007) 27:119–27. doi: 10.1089/jir.2006.0094
12. Li SF, Zhao FR, Shao JJ, Xie YL, Chang HY, Zhang YG. Interferon-omega: Current status in clinical applications. Int Immunopharmacol (2017) 52:253–60. doi: 10.1016/j.intimp.2017.08.028
13. Meager A, Visvalingam K, Peterson P, Moll K, Murumagi A, Krohn K, et al. Anti-interferon autoantibodies in autoimmune polyendocrinopathy syndrome type 1. PLoS Med (2006) 3:e289. doi: 10.1371/journal.pmed.0030289
14. Bastard P, Rosen LB, Zhang Q, Michailidis E, Hoffmann HH, Zhang Y, et al. Autoantibodies against type I IFNs in patients with life-threatening COVID-19. Science (2020) 370(6515):eabd4585. doi: 10.1126/science.abd4585
15. Krause CD, Pestka S. Cut, copy, move, delete: The study of human interferon genes reveal multiple mechanisms underlying their evolution in amniotes. Cytokine (2015) 76:480–95. doi: 10.1016/j.cyto.2015.07.019
16. Hosoi K, Utsumi J, Kitagawa T, Shimizu H, Kobayashi S. Structural characterization of fibroblast human interferon-beta 1. J Interferon Res (1988) 8:375–84. doi: 10.1089/jir.1988.8.375
17. Adolf GR, Fruhbeis B, Hauptmann R, Kalsner I, Maurer-Fogy I, Ostermann E, et al. Human interferon omega 1: isolation of the gene, expression in Chinese hamster ovary cells and characterization of the recombinant protein. Biochim Biophys Acta (1991) 1089:167–74. doi: 10.1016/0167-4781(91)90004-6
18. Adolf GR, Kalsner I, Ahorn H, Maurer-Fogy I, Cantell K. Natural human interferon-alpha 2 is O-glycosylated. Biochem J (1991) 276(Pt 2):511–8. doi: 10.1042/bj2760511
19. Nyman TA, Tolo H, Parkkinen J, Kalkkinen N. Identification of nine interferon-alpha subtypes produced by Sendai virus-induced human peripheral blood leucocytes. Biochem J (1998) 329(Pt 2):295–302. doi: 10.1042/bj3290295
20. Renauld JC. Class II cytokine receptors and their ligands: key antiviral and inflammatory modulators. Nat Rev Immunol (2003) 3:667–76. doi: 10.1038/nri1153
21. Thomas C, Moraga I, Levin D, Krutzik PO, Podoplelova Y, Trejo A, et al. Structural linkage between ligand discrimination and receptor activation by type I interferons. Cell (2011) 146:621–32. doi: 10.1016/j.cell.2011.06.048
22. Karpusas M, Nolte M, Benton CB, Meier W, Lipscomb WN, Goelz S. The crystal structure of human interferon beta at 2.2-A resolution. Proc Natl Acad Sci U S A (1997) 94:11813–8. doi: 10.1073/pnas.94.22.11813
23. Radhakrishnan R, Walter LJ, Hruza A, Reichert P, Trotta PP, Nagabhushan TL, et al. Zinc mediated dimer of human interferon-alpha 2b revealed by X-ray crystallography. Structure (1996) 4:1453–63. doi: 10.1016/S0969-2126(96)00152-9
24. Piehler J, Thomas C, Garcia KC, Schreiber G. Structural and dynamic determinants of type I interferon receptor assembly and their functional interpretation. Immunol Rev (2012) 250:317–34. doi: 10.1111/imr.12001
25. Sharma N, Longjam G, Schreiber G. Type I Interferon Signaling Is Decoupled from Specific Receptor Orientation through Lenient Requirements of the Transmembrane Domain. J Biol Chem (2016) 291:3371–84. doi: 10.1074/jbc.M115.686071
26. Levin D, Schneider WM, Hoffmann HH, Yarden G, Busetto AG, Manor O, et al. Multifaceted activities of type I interferon are revealed by a receptor antagonist. Sci Signal (2014) 7:ra50. doi: 10.1126/scisignal.2004998
27. Urin V, Shemesh M, Schreiber G. CRISPR/Cas9-based Knockout Strategy Elucidates Components Essential for Type 1 Interferon Signaling in Human HeLa Cells. J Mol Biol (2019) 431:3324–38. doi: 10.1016/j.jmb.2019.06.007
28. Novatt H, Theisen TC, Massie T, Massie T, Simonyan V, Voskanian-Kordi A, et al. Distinct Patterns of Expression of Transcription Factors in Response to Interferonbeta and Interferonlambda1. J Interferon Cytokine Res (2016) 36:589–98. doi: 10.1089/jir.2016.0031
29. Panda D, Gjinaj E, Bachu M, Squire E, Novatt H, Ozato K, et al. IRF1 Maintains Optimal Constitutive Expression of Antiviral Genes and Regulates the Early Antiviral Response. Front Immunol (2019) 10:1019. doi: 10.3389/fimmu.2019.01019
30. Vazquez N, Schmeisser H, Dolan MA, Bekisz J, Zoon KC, Wahl SM. Structural variants of IFNalpha preferentially promote antiviral functions. Blood (2011) 118:2567–77. doi: 10.1182/blood-2010-12-325027
31. Yamane D, Feng H, Rivera-Serrano EE, Selitsky SR, Hirai-Yuki A, Das A, et al. Basal expression of interferon regulatory factor 1 drives intrinsic hepatocyte resistance to multiple RNA viruses. Nat Microbiol (2019) 4:1096–104. doi: 10.1038/s41564-019-0425-6
32. de Weerd NA, Vivian JP, Nguyen TK, Mangan NE, Gould JA, Braniff SJ, et al. Structural basis of a unique interferon-beta signaling axis mediated via the receptor IFNAR1. Nat Immunol (2013) 14:901–7. doi: 10.1038/ni.2667
33. Shields LE, Jennings J, Liu Q, Lee J, Ma W, Blecha F, et al. Cross-Species Genome-Wide Analysis Reveals Molecular and Functional Diversity of the Unconventional Interferon-omega Subtype. Front Immunol (2019) 10:1431. doi: 10.3389/fimmu.2019.01431
34. Woelk CH, Frost SD, Richman DD, Higley PE, Kosakovsky Pond SL. Evolution of the interferon alpha gene family in eutherian mammals. Gene (2007) 397:38–50. doi: 10.1016/j.gene.2007.03.018
35. Manry J, Laval G, Patin E, Fornarino S, Itan Y, Fumagalli M, et al. Evolutionary genetic dissection of human interferons. J Exp Med (2011) 208:2747–59. doi: 10.1084/jem.20111680
36. Hillyer P, Raviv N, Gold DM, Dougherty D, Liu J, Johnson TR, et al. Subtypes of type I IFN differentially enhance cytokine expression by suboptimally stimulated CD4(+) T cells. Eur J Immunol (2013) 43:3197–208. doi: 10.1002/eji.201243288
37. Durbin RK, Kotenko SV, Durbin JE. Interferon induction and function at the mucosal surface. Immunol Rev (2013) 255:25–39. doi: 10.1111/imr.12101
38. Genin P, Vaccaro A, Civas A. The role of differential expression of human interferon–a genes in antiviral immunity. Cytokine Growth Factor Rev (2009) 20:283–95. doi: 10.1016/j.cytogfr.2009.07.005
39. Panne D, Maniatis T, Harrison SC. An atomic model of the interferon-beta enhanceosome. Cell (2007) 129:1111–23. doi: 10.1016/j.cell.2007.05.019
40. Honda K, Yanai H, Negishi H, Asagiri M, Sato M, Mizutani T, et al. IRF-7 is the master regulator of type-I interferon-dependent immune responses. Nature (2005) 434:772–7. doi: 10.1038/nature03464
41. Schwanke H, Stempel M, Brinkmann MM. Of Keeping and Tipping the Balance: Host Regulation and Viral Modulation of IRF3-Dependent IFNB1 Expression. Viruses (2020) 12:733. doi: 10.3390/v12070733
42. Andersen LL, Mork N, Reinert LS, Kofod-Olsen E, Narita R, Jorgensen SE, et al. Functional IRF3 deficiency in a patient with herpes simplex encephalitis. J Exp Med (2015) 212:1371–9. doi: 10.1084/jem.20142274
43. Thomsen MM, Jorgensen SE, Storgaard M, Kristensen LS, Gjedsted J, Christiansen M, et al. Identification of an IRF3 variant and defective antiviral interferon responses in a patient with severe influenza. Eur J Immunol (2019) 49:2111–4. doi: 10.1002/eji.201848083
44. Patil S, Fribourg M, Ge Y, Batish M, Tyagi S, Hayot F, et al. Single-cell analysis shows that paracrine signaling by first responder cells shapes the interferon-beta response to viral infection. Sci Signal (2015) 8:ra16. doi: 10.1126/scisignal.2005728
45. Ciancanelli MJ, Huang SX, Luthra P, Garner H, Itan Y, Volpi S, et al. Infectious disease. Life-threatening influenza and impaired interferon amplification in human IRF7 deficiency. Science (2015) 348:448–53. doi: 10.1126/science.aaa1578
46. Thomsen MM, Jorgensen SE, Gad HH, Storgaard M, Gjedsted J, Christiansen M, et al. Defective interferon priming and impaired antiviral responses in a patient with an IRF7 variant and severe influenza. Med Microbiol Immunol (2019) 208:869–76. doi: 10.1007/s00430-019-00623-8
47. Izaguirre A, Barnes BJ, Amrute S, Yeow WS, Megjugorac N, Dai J, et al. Comparative analysis of IRF and IFN-alpha expression in human plasmacytoid and monocyte-derived dendritic cells. J Leukoc Biol (2003) 74:1125–38. doi: 10.1189/jlb.0603255
48. Szubin R, Chang WL, Greasby T, Beckett L, Baumgarth N. Rigid interferon-alpha subtype responses of human plasmacytoid dendritic cells. J Interferon Cytokine Res (2008) 28:749–63. doi: 10.1089/jir.2008.0037
49. Hillyer P, Mane VP, Schramm LM, Puig M, Verthelyi D, Chen A, et al. Expression profiles of human interferon-alpha and interferon-lambda subtypes are ligand- and cell-dependent. Immunol Cell Biol (2012) 90:774–83. doi: 10.1038/icb.2011.109
50. Novikov A, Cardone M, Thompson R, Shenderov K, Kirschman KD, Mayer-Barber KD, et al. Mycobacterium tuberculosis triggers host type I IFN signaling to regulate IL-1beta production in human macrophages. J7nbsp;Immunol (2011) 187:2540–7. doi: 10.4049/jimmunol.1100926
51. Hillyer P, Shepard R, Uehling M, Krenz M, Sheikh F, Thayer KR, et al. Differential Responses by Human Respiratory Epithelial Cell Lines to Respiratory Syncytial Virus Reflect Distinct Patterns of Infection Control. J Virol (2018) 92(15):e02202–17. doi: 10.1128/JVI.02202-17
52. Genin P, Lin R, Hiscott J, Civas A. Differential regulation of human interferon A gene expression by interferon regulatory factors 3 and 7. Mol Cell Biol (2009) 29:3435–50. doi: 10.1128/MCB.01805-08
53. Puig M, Tosh KW, Schramm LM, Grajkowska LT, Kirschman KD, Tami C, et al. TLR9 and TLR7 agonists mediate distinct type I IFN responses in humans and nonhuman primates in vitro and in vivo. J Leukoc Biol (2012) 91:147–58. doi: 10.1189/jlb.0711371
54. Larrea E, Alberdi A, Castelruiz Y, Boya P, Civeira MP, Prieto J. Expression of interferon-alpha subtypes in peripheral mononuclear cells from patients with chronic hepatitis C: a role for interferon-alpha5. J Viral Hepat (2001) 8:103–10. doi: 10.1046/j.1365-2893.2001.00273.x
55. Hillyer P, Mane VP, Chen A, Dos Santos MB, Schramm LM, Shepard RE, et al. Respiratory syncytial virus infection induces a subset of types I and III interferons in human dendritic cells. Virology (2017) 504:63–72. doi: 10.1016/j.virol.2017.01.017
56. Thomas JM, Pos Z, Reinboth J, Wang RY, Wang E, Frank GM, et al. Differential responses of plasmacytoid dendritic cells to influenza virus and distinct viral pathogens. J Virol (2014) 88:10758–66. doi: 10.1128/JVI.01501-14
57. Harper MS, Guo K, Gibbert K, Lee EJ, Dillon SM, Barrett BS, et al. Interferon-alpha Subtypes in an Ex Vivo Model of Acute HIV-1 Infection: Expression, Potency and Effector Mechanisms. PLoS Pathog (2015) 11:e1005254. doi: 10.1371/journal.ppat.1005254
58. Menachery VD, Eisfeld AJ, Schafer A, Josset L, Sims AC, Proll S, et al. Pathogenic influenza viruses and coronaviruses utilize similar and contrasting approaches to control interferon-stimulated gene responses. mBio (2014) 5:e01174–01114. doi: 10.1128/mBio.01174-14
59. Matos ADR, Wunderlich K, Schloer S, Schughart K, Geffers R, Seders M, et al. Antiviral potential of human IFN-alpha subtypes against influenza A H3N2 infection in human lung explants reveals subtype-specific activities. Emerg Microbes Infect (2019) 8:1763–76. doi: 10.1080/22221751.2019.1698271
60. Zaritsky LA, Bedsaul JR, Zoon KC. Virus Multiplicity of Infection Affects Type I Interferon Subtype Induction Profiles and Interferon-Stimulated Genes. J Virol (2015) 89:11534–48. doi: 10.1128/JVI.01727-15
61. Kimura T, Jiang S, Nishizawa M, Yoshigai E, Hashimoto I, Nishikawa M, et al. Stabilization of human interferon-alpha1 mRNA by its antisense RNA. Cell Mol Life Sci (2013) 70:1451–67. doi: 10.1007/s00018-012-1216-x
62. Kimura T, Jiang S, Yoshida N, Sakamoto R, Nishizawa M. Interferon-alpha competing endogenous RNA network antagonizes microRNA-1270. Cell Mol Life Sci (2015) 72:2749–61. doi: 10.1007/s00018-015-1875-5
63. Lavoie TB, Kalie E, Crisafulli-Cabatu S, Abramovich R, DiGioia G, Moolchan K, et al. Binding and activity of all human alpha interferon subtypes. Cytokine (2011) 56:282–9. doi: 10.1016/j.cyto.2011.07.019
64. Jaitin DA, Roisman LC, Jaks E, Gavutis M, Piehler J, Van der Heyden J, et al. Inquiring into the differential action of interferons (IFNs): an IFN-alpha2 mutant with enhanced affinity to IFNAR1 is functionally similar to IFN-beta. Mol Cell Biol (2006) 26:1888–97. doi: 10.1128/MCB.26.5.1888-1897.2006
65. Harris BD, Schreiter J, Chevrier M, Jordan JL, Walter MR. Human interferon- and interferon-kappa exhibit low potency and low affinity for cell-surface IFNAR and the poxvirus antagonist B18R. J Biol Chem (2018) 293:16057–68. doi: 10.1074/jbc.RA118.003617
66. Marijanovic Z, Ragimbeau J, van der Heyden J, Uze G, Pellegrini S. Comparable potency of IFNalpha2 and IFNbeta on immediate JAK/STAT activation but differential down-regulation of IFNAR2. Biochem J (2007) 407:141–51. doi: 10.1042/BJ20070605
67. Chmiest D, Sharma N, Zanin N, Viaris de Lesegno C, Shafaq-Zadah M, Sibut V, et al. Spatiotemporal control of interferon-induced JAK/STAT signalling and gene transcription by the retromer complex. Nat Commun (2016) 7:13476. doi: 10.1038/ncomms13476
68. Basters A, Knobeloch KP, Fritz G. USP18 - a multifunctional component in the interferon response. Biosci Rep (2018) 38(6):BSR20180250. doi: 10.1042/BSR20180250
69. Zhang X, Bogunovic D, Payelle-Brogard B, Francois-Newton V, Speer SD, Yuan C, et al. Human intracellular ISG15 prevents interferon-alpha/beta over-amplification and auto-inflammation. Nature (2015) 517:89–93. doi: 10.1038/nature13801
70. Wilmes S, Beutel O, Li Z, Francois-Newton V, Richter CP, Janning D, et al. Receptor dimerization dynamics as a regulatory valve for plasticity of type I interferon signaling. J Cell Biol (2015) 209:579–93. doi: 10.1083/jcb.201412049
71. Arimoto K II, Lochte S, Stoner SA, Burkart C, Zhang Y, Miyauchi S, et al. STAT2 is an essential adaptor in USP18-mediated suppression of type I interferon signaling. Nat Struct Mol Biol (2017) 24:279–89. doi: 10.1038/nsmb.3378
72. Francois-Newton V, Magno de Freitas Almeida G, Payelle-Brogard B, Monneron D, Pichard-Garcia L, Piehler J, et al. USP18-based negative feedback control is induced by type I and type III interferons and specifically inactivates interferon alpha response. PLoS One (2011) 6:e22200. doi: 10.1371/journal.pone.0022200
73. Meuwissen ME, Schot R, Buta S, Oudesluijs G, Tinschert S, Speer SD, et al. Human USP18 deficiency underlies type 1 interferonopathy leading to severe pseudo-TORCH syndrome. J Exp Med (2016) 213:1163–74. doi: 10.1084/jem.20151529
74. Gruber C, Martin-Fernandez M, Ailal F, Qiu X, Taft J, Altman J, et al. Homozygous STAT2 gain-of-function mutation by loss of USP18 activity in a patient with type I interferonopathy. J Exp Med (2020) 217(5):e20192319. doi: 10.1084/jem.20192319
75. Gordts P, Foley EM, Lawrence R, Sinha R, Lameda-Diaz C, Deng L, et al. Reducing macrophage proteoglycan sulfation increases atherosclerosis and obesity through enhanced type I interferon signaling. Cell Metab (2014) 20:813–26. doi: 10.1016/j.cmet.2014.09.016
76. Schulze AB, Evers G, Kerkhoff A, Mohr M, Schliemann C, Berdel WE, et al. Future Options of Molecular-Targeted Therapy in Small Cell Lung Cancer. Cancers (Basel) (2019) 11:690. doi: 10.3390/cancers11050690
77. Liu YC, Yeh CT, Lin KH. Cancer Stem Cell Functions in Hepatocellular Carcinoma and Comprehensive Therapeutic Strategies. Cells (2020) 9:1331. doi: 10.3390/cells9061331
78. Gritsenko PG, Friedl P. Adaptive adhesion systems mediate glioma cell invasion in complex environments. J Cell Sci (2018) 13:131. doi: 10.1242/jcs.216382
79. Mastrangeli R, D’Amici F, D’Acunto CW, Fiumi S, Rossi M, Terlizzese M, et al. A deamidated interferon-beta variant binds to integrin alphavbeta3. Cytokine (2018) 104:38–41. doi: 10.1016/j.cyto.2018.01.024
80. Sharma N, O’Neal AJ, Gonzalez C, Wittling M, Gjinaj E, Parsons LM, et al. S27 of IFNalpha1 Contributes to Its Low Affinity for IFNAR2 and Weak Antiviral Activity. J Interferon Cytokine Res (2019) 39:283–92. doi: 10.1089/jir.2018.0135
81. Li H, Sharma N, General IJ, Schreiber G, Bahar I. Dynamic Modulation of Binding Affinity as a Mechanism for Regulating Interferon Signaling. J Mol Biol (2017) 429:2571–89. doi: 10.1016/j.jmb.2017.06.011
82. Slutzki M, Jaitin DA, Yehezkel TB, Schreiber G. Variations in the unstructured C-terminal tail of interferons contribute to differential receptor binding and biological activity. J Mol Biol (2006) 360:1019–30. doi: 10.1016/j.jmb.2006.05.069
83. Purple Book. Lists of Licensed Biological Products with Reference Product Exclusivity and Biosimilarity or Interchangeability Evaluations. US Food and Drug Administration.
84. Schreiber G. The Role of Type I Interferons in the Pathogenesis and Treatment of COVID-19. Front Immunol (2020) 11:595739. doi: 10.3389/fimmu.2020.595739
85. Turecek PL, Bossard MJ, Schoetens F, Ivens IA. PEGylation of Biopharmaceuticals: A Review of Chemistry and Nonclinical Safety Information of Approved Drugs. J Pharm Sci (2016) 105:460–75. doi: 10.1016/j.xphs.2015.11.015
86. AASLD-IDSA. Recommendations for testing, managing, and treating hepatitis C. Available at: http://www.hcvguidelines.org.
87. Goldschmidt CH, Hua LH. Re-Evaluating the Use of IFN-beta and Relapsing Multiple Sclerosis: Safety, Efficacy and Place in Therapy. Degener Neurol Neuromuscul Dis (2020) 10:29–38. doi: 10.2147/DNND.S224912
88. Harari D, Kuhn N, Abramovich R, Sasson K, Zozulya AL, Smith P, et al. Enhanced in vivo efficacy of a type I interferon superagonist with extended plasma half-life in a mouse model of multiple sclerosis. J Biol Chem (2014) 289:29014–29. doi: 10.1074/jbc.M114.602474
89. Foster GR, Rodrigues O, Ghouze F, Schulte-Frohlinde E, Testa D, Liao MJ, et al. Different relative activities of human cell-derived interferon-alpha subtypes: IFN-alpha 8 has very high antiviral potency. J Interferon Cytokine Res (1996) 16:1027–33. doi: 10.1089/jir.1996.16.1027
90. Dubois A, Francois C, Descamps V, Fournier C, Wychowski C, Dubuisson J, et al. Enhanced anti-HCV activity of interferon alpha 17 subtype. Virol J (2009) 6:70. doi: 10.1186/1743-422X-6-70
91. Schlaepfer E, Fahrny A, Gruenbach M, Kuster SP, Simon V, Schreiber G, et al. Dose-Dependent Differences in HIV Inhibition by Different Interferon Alpha Subtypes While Having Overall Similar Biologic Effects. mSphere (2019) 4:e00275–19. doi: 10.1128/mSphere.00637-18
92. Moll HP, Maier T, Zommer A, Lavoie T, Brostjan C. The differential activity of interferon-alpha subtypes is consistent among distinct target genes and cell types. Cytokine (2011) 53:52–9. doi: 10.1016/j.cyto.2010.09.006
Keywords: type I interferon, interferon-beta, interferon-alpha, interferon-omega, human, primate
Citation: Wittling MC, Cahalan SR, Levenson EA and Rabin RL (2021) Shared and Unique Features of Human Interferon-Beta and Interferon-Alpha Subtypes. Front. Immunol. 11:605673. doi: 10.3389/fimmu.2020.605673
Received: 12 September 2020; Accepted: 18 November 2020;
Published: 19 January 2021.
Edited by:
Helena Stabile, Sapienza University of Rome, ItalyReviewed by:
Kathrin Sutter, University of Duisburg-Essen, GermanyLuciana D’Apice, National Research Council (CNR), Italy
Copyright © 2021 Wittling, Cahalan, Levenson and Rabin. This is an open-access article distributed under the terms of the Creative Commons Attribution License (CC BY). The use, distribution or reproduction in other forums is permitted, provided the original author(s) and the copyright owner(s) are credited and that the original publication in this journal is cited, in accordance with accepted academic practice. No use, distribution or reproduction is permitted which does not comply with these terms.
*Correspondence: Ronald L. Rabin, cm9uYWxkLnJhYmluQGZkYS5oaHMuZ292