- Institute of Clinical Microbiology and Hygiene, University Hospital of Regensburg, University of Regensburg, Regensburg, Germany
Low oxygen environments and accumulation of hypoxia-inducible factors (HIFs) are features of infected and inflamed tissues. Here, we summarize our current knowledge on oxygen levels found in Leishmania-infected tissues and discuss which mechanisms potentially contribute to local tissue oxygenation in leishmanial lesions. Moreover, we review the role of hypoxia and HIF-1 on innate antileishmanial immune responses.
Introduction
Low oxygen (O2) environments are a key feature of infected and inflamed tissue. Several lines of evidence demonstrate that oxygen levels of afflicted tissues are much lower than these currently used in standard cell culture experiments and, in general, correspond to values below 4% O2 [reviewed in Ref. (1, 2)] Low oxygen levels are able to incapacitate oxygen-dependent antimicrobial effector enzymes such as the phagocytes oxidase or inducible NO synthase which both require oxygen as cosubstrate in order to produce their antimicrobial reactive oxygen species (ROS) and reactive nitrogen species (RNS) [reviewed in Ref. (1, 2)]. Moreover, hypoxia is not only a state of reduced availability of oxygen but also in addition induces a transcriptional response, which is governed by the transcription factors (TFs) hypoxia-inducible factor (HIF)-1 and HIF-2. Both TFs belong to the basic helix–loop–helix-PAS family of TF, consisting of HIF-1α or HIF-2α and its dimerization partner aryl hydrocarbon receptor nuclear translocator (ARNT) [reviewed in Ref. (3)]. Prolyl-hydroxylase domain (PHD) enzymes play a key role in the regulation of HIF-1α and HIF-2α since oxygen is a critical substrate for the PHD enzymes. Under conditions of ample oxygen, they hydroxylate HIF-α subunits that target HIF-α in a von Hippel-Lindau tumor suppressor-dependent manner to proteasomal degradation [reviewed in Ref. (4–6)].
Subsequent studies revealed that HIF-1α is not only involved in adaption of cells to low oxygen environments but that this TF is also stabilized upon infectious and inflammatory stimuli under conditions of ample oxygen as well. Furthermore, HIF-1α is required for inflammatory responses of innate immune cells in vitro and in vivo [reviewed in Ref. (1, 7–11)]. Mechanistically, normoxic, inflammatory HIF-1α stabilization is closely linked to nuclear factor (NF)-κB activation (12, 13), and involves transcriptional and posttranslational signaling events (14–16). Altogether, these findings demonstrate that hypoxic and inflammatory responses are intertwined.
Therefore, there is a growing interest to uncover the impact of hypoxia and HIF-1α in infectious diseases and its impact on host–pathogen interaction [reviewed in Ref. (1, 2, 17–21)]. In this review, we will summarize the evidence of hypoxia and the TF HIF-1α and its impact on innate immune responses directed against infection with Leishmania major, Leishmania amazonensis, and Leishmania donovani, which are able to cause cutaneous, mucocutaneous and systemic (visceral) diseases, respectively (Table 1).
Oxygen Level in Leishmanial Lesions
In guinea-pigs, intravenous challenge experiments with guinea-pig adapted Leishmania enrietti demonstrate that skin tissue predisposes to the development of leishmanial lesions (22). Given that skin tissue is known to display low oxygen levels (23–25), these data suggest that low oxygen microenvironments might provide a safe haven for Leishmania parasites (22). Araújo et al. assessed lesional tissue oxygen levels with an immunohistochemical method in cutaneous L. amazonensis infection (26). For that purpose, they injected a 2-nitroimidazol derivative into L. amazonensis infected mice. Upon injection, these compounds are enriched in tissues with very low oxygen tensions and form adducts that can be visualized after staining with adduct-specific antibodies (27, 28). Using this technology, they found that in L. amazonensis-induced lesions very low oxygen tensions are present (26).
To the best of our knowledge, there are no data available on tissue oxygen levels after infection with L. donovani in liver tissue. Since it is known that steep oxygen gradients exist in the liver (27), it is very likely that L. donovani-infected liver tissues display low oxygen levels as well. Using 2-nitroimidazol based techniques to visualize hypoxic tissues, Hammami et al. revealed that in L. donovani-infected spleens, very low tissue oxygen prevail (29).
Although staining of hypoxic areas with 2-nitroimidazol-derivatives allows for identification of severely hypoxic tissue, this method does not provide quantitative data and is not suitable for continuous recording of lesional tissue oxygen levels (25). Alternative methodologies to quantitatively assess tissue oxygen levels rely on polarographic oxygen sensors or imaging of tissue oxygenation by using oxygen-dependent luminescence quenching (25). For instance, non-invasive luminescence-based oxygen imaging using sensor foils can be used to quantitatively assess tissue oxygenation in a non-invasive manner over time (25). Using this technology in a mouse model of self-healing cutaneous leishmaniasis, Mahnke et al. observed that oxygen tension in leishmanial skin lesions displayed oxygen levels around ~2.8% O2 when the lesions reached their maximum size (30). Resolution and healing of the lesion was paralleled by an increase of tissue oxygenation (30). Altogether, these findings indicate that low oxygen levels prevail in Leishmania-infected tissue.
Potential Factors Regulating Tissue Oxygen Levels in Leishmania-Infected Tissues
The mechanisms that drive low oxygen levels in Leishmania infected tissue are, however, largely unknown. Recent studies demonstrate that influx of granulocytes results in increased O2 demand which ultimately results in low tissue oxygen levels (31). Low tissue oxygenation in L. major-infected tissue might result from either (i) infection-induced disturbance of local tissue perfusion or (ii) an increased local O2 consumption by the infectious agent and/or infiltrating immune cells (Figure 1). However, the contribution of infiltrating granulocytes, monocytes, NK cells, and T cells on tissue oxygen levels in Leishmania infected tissues is unexplored. Moreover, Leishmania might interfere with inflammation induced angiogenesis. For instance, Leishmania is able to scavenge angiogenic factors such as VEGF-A. Thereby Leishmania might interfere with inflammation-associated angiogenesis and disturb local oxygen supply (32). Nevertheless infection with L. major eventually triggers a vascular endothelial growth factor (VEGF)-A/VEGF receptor (VEGFR)-2-dependent proliferation of endothelial cells (33). Underscoring the potential important role of VEGF-A/VEGFR-2 signaling, Araújo and Giorgio suggest that VEGF-A levels upon infection might predict the outcome of L. amazonensis-infection. They recorded higher VEGF-A levels in healer mice compared to non-healer mice (34). Since VEGF-A is a known HIF-1α target (35) and HIF-1α is present in L. amazonensis- and L. major-infected lesions in humans and in preclinical models (26, 33, 34, 36), it is possible that HIF-1α plays a role in infection-induced proliferation of the endothelial cells. However, to the best of our knowledge, this has not been tested in L. major and L. amazonensis mouse models yet. In addition to maintaining oxygenation of infected tissue, endothelial cell proliferation might curtail L. major induced-inflammatory responses (37). The activity of endothelial NO synthase plays an important role in endothelial cell mediated anti-inflammatory activity (38–40). Endothelial cell mediated anti-inflammatory activity might reduce the influx of immune cells and/or their O2-consumption, thereby increase tissue oxygen levels and facilitate subsequent resolution of disease. Since endothelial NO synthase expression is linked to HIF-1α-accumulation (41), endothelial HIF-1α might promote anti-inflammatory properties in endothelial cells as well. Further studies, however, are required to investigate this issue. Earlier findings demonstrate that the “myeloid cell differentiation antigen carcinoembryonic antigen-related cell adhesion molecule 1” (CEACAM1) is involved in angiogenesis and antileishmanial control (42). Whether CEACAM1-dependent signaling affects VEGF-A/VEGFR and/or HIF-1α signaling in this state of affair is unknown. Further investigations are required to understand the interplay of inflammation-triggered angiogenesis, enhanced O2 demand in inflamed tissues, and its impact on overall tissue oxygenation in leishmaniasis.
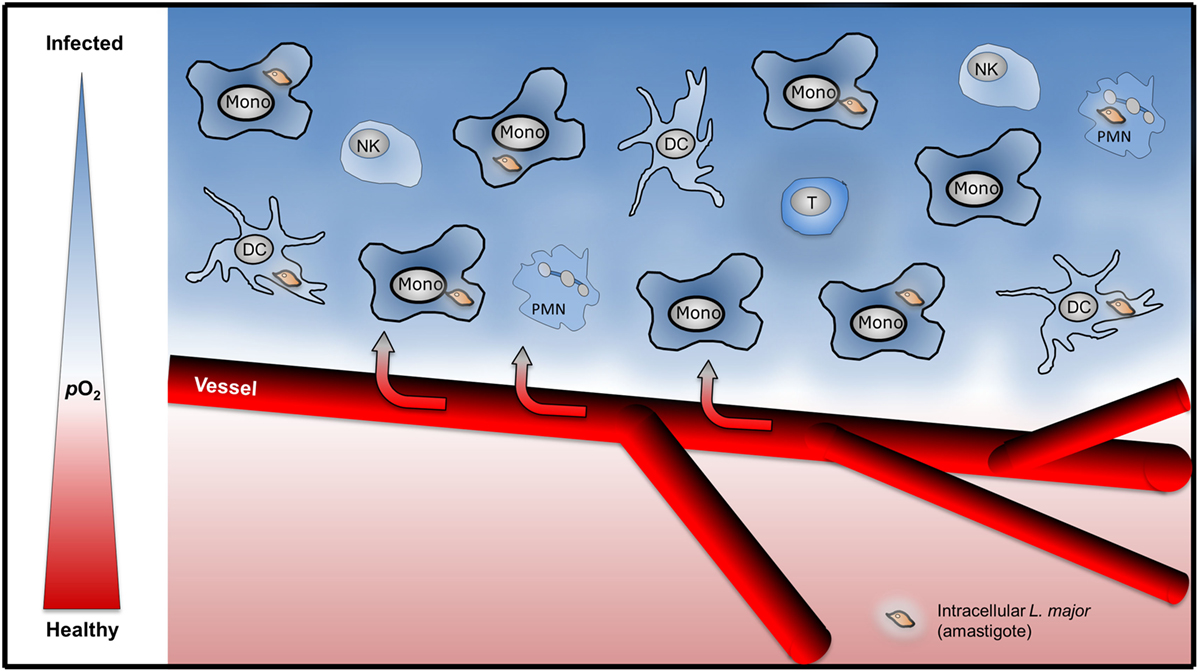
Figure 1. Infiltration of immune cells might drive low tissue oxygenation in Leishmania major-infected skin. Upon L. major-infection, infiltration of granulocytes [polymorphonuclear granulocyte (PMN)], monocytes (Mono), dendritic cells (DC), NK cells, and T cells might induce increased local consumption of O2 resulting in hypoxic tissue O2 levels.
Impact of Hypoxia on Host–Pathogen Interaction
Internalization of Leishmania and Hypoxia
After transmission of Leishmania by its vector, myeloid cells are the main host cell [reviewed in Ref. (43–45)]. Hypoxic conditions are known to modulate the phagocytic/endocytic uptake of mononuclear phagocytes (46, 47). However, to the best of our knowledge, the impact of oxygen levels below 4% O2 on Leishmania uptake or internalization is unexplored.
Polymorphonuclear Granulocytes (PMN), Hypoxia, and Leishmania
Polymorphonuclear granulocytes are quickly attracted to the site of infection. Dependent on the Leishmania species and the host’s genetic background, they play a rather detrimental role in cutaneous leishmaniasis. PMN are inefficient in killing promastigotes and undergo apoptosis which hampers the proper activation of recruited dendritic cell (DC) [reviewed in Ref. (44, 48)]. In general, PMN are relatively short-lived cells, whose survival, however, is prolonged in NF-κB- and HIF-1α-dependent manner under hypoxic conditions. Moreover, hypoxia induces the production of macrophage inflammatory protein-1β which further facilitates neutrophil survival (49). In line with this, Monceaux et al. demonstrated recently that anoxic conditions prolong PMN survival (50). Therefore, hypoxia most likely favors influx and lifespan of PMN in leishmaniasis as well. Since these cells are not able to eliminate Leishmania, this might ultimately prolong and/or exacerbate the disease. However, to the best of our knowledge, there is no detailed analysis on the specific role of hypoxia on PMN–Leishmania interaction.
DC, Hypoxia, and Leishmania
Upon Leishmania exposure, DC phagocytose the parasite, become activated and establish an antileishmanial T cell response and thereby critically contribute to the resolution of disease [reviewed in Ref. (51)]. For instance, priming and induction of Leishmania-specific TH1 response results in secretion of IFN-γ which is required for antileishmanial immunity [reviewed in Ref. (44)]. In addition, even after resolution of disease, DC still contain viable parasites which are able to maintain antigen-specific T cell responses against L. major. This contributes to the long term protection against the parasites [reviewed in Ref. (51)]. Although, there is evidence that oxygen levels impact on DC functions, there are to the best of our knowledge only limited reports on the contribution of hypoxia on DC-mediated establishment of antileishmanial T cell immunity. Hypoxia might impact on DC viability (52), migratory capacity (53), maturation, and antigen presentation (54–56).
NK Cells, Hypoxia, and Leishmania
In early phase of Leishmania infection, NK cells are activated by IL-12 and IL-18. These activated NK cells contribute to parasite control through production of IFN-γ [reviewed in Ref. (57)]. NK cells respond to hypoxia by downregulation of surface markers NKp46, NKp30, NKp44, NKG2D, and CD16, decreased cytotoxic and antiviral activity, and reduced IFN-γ expression (58–60). In contrast to these findings, Krzywinska et al. observed no mitigated IFN-γ secretion by NK cells under hypoxia (61). However, the impact of hypoxia on NK-mediated antileishmanial defense is unexplored and requires further studies.
Monocytes/Macrophages, Hypoxia, and Leishmania
A few days after L. major and L. donovani infection, recruited monocytes are highly abundant in afflicted tissues (62, 63). Inflammatory monocytes are able to upregulate their ROS production in the early stage of infection with L. major and L. donovani (64, 65). However, in inflammatory monocytes infected with L. major, ROS production does eliminate the parasites (63). Moreover, L. donovani, for instance, has the ability to overcome the ROS-mediated apoptosis of host macrophages via induction of suppressor of cytokine signaling proteins (66). It is established that IFN-γ and TNF are two key cytokines that empower macrophages (67–69) and monocytes (63) to clear Leishmania. In the mouse system this results in a robust induction of the antileishmanial effector enzyme, the inducible or type 2 NO synthase (iNOS or NOS2). A high level of NO production is prerequisite of the cutaneous defense against Leishmania major [reviewed in Ref. (70, 71)]. Moreover, in mouse models of visceral leishmaniasis, Nos2 expression is important for control of L. donovani in the liver (72), and contributes to the containment of L. donovani (73) in the spleen.
For induction of NOS2 and production of high level of leishmanicidal NO, costimulation of macrophages with TNF plus IFN-γ is required (63, 68, 72, 74–78). Low oxygen conditions, however, impair NO production of activated macrophages because the inducible NO synthase activity critically hinges on the availability of O2 as a substrate (79–81). Accordingly, under oxygen conditions below 4% O2 NO production of activated macrophages is diminished (30). L. major possesses a globin-coupled heme containing oxygen sensor soluble adenylate cyclase which allows for its adaption to hypoxic conditions (82). Hence, while L. major is able to adjust to hypoxia, low oxygen conditions impair the NO-dependent antileishmanial potential of macrophages resulting in impaired clearance and survival of L. major in activated macrophages under low oxygen conditions. However, leishmanicidal activity is restored when the cells are reoxygenated (30). These data demonstrate that local tissue oxygen levels—at least transiently—do not match the macrophages’ O2 demand to fight against L. major.
In visceral leishmaniasis, parasites express high tissue tropism toward monocytes in the spleen and bone marrow. L. donovani, e.g., induces expansion of hematopoietic stem cell-like mononuclear cells from bone marrow which serve as host cells for the parasite (62). Compared to the role of Nos2 in the defense against visceral leishmaniasis in the liver (72), the contribution of Nos2 in the control of parasite load in the spleen in visceral leishmaniasis is less pronounced. While there was no induction of Nos2 in spleen macrophages upon infection of hamsters with L. donovani (83), Nos2 expression, nevertheless, contributed to the parasite-containment in the spleen of L. donovani and L. infantum-infected mice (73, 84). In preclinical models of visceral leishmaniasis, the impact of local oxygen levels on Nos2-mediated antileishmanial defense and NO production in the spleen and liver is, however, to the best of our knowledge unexplored. It is tempting to speculate that low oxygen levels found in L. donovani-infected spleen tissue (29) incapacitate the Nos2-dependent antileishmanial defense in the spleen and, thus provide a safe niche for persistence and proliferation of L. donovani.
In contrast to L. major, exposure of L. amazonensis-infected macrophages to O2 levels of 5% and 1% O2 resulted in enhanced clearance of intracellular parasites (85, 86). These findings suggest that either (1) NO-independent mechanisms are involved in control of L. amazonensis under hypoxic conditions and/or (2) L. amazonensis is not able to adjust to low oxygen conditions. For instance, the divergent effect of hypoxia on the survival of members of the genus Leishmania in mononuclear phagocytes under hypoxia might be due to different metabolic requirements and O2-demands of the respective Leishmania species investigated. While it is known that L. major and L. donovani are able to adjust to hypoxic conditions by increasing their rate of glycolysis (87, 88), to the best of our knowledge detailed investigations on the intermediary metabolism of L. amazonensis upon exposure to low oxygen conditions are lacking.
Mononuclear Phagocytes, HIF-1α, and Antileishmanial Immunity
In addition to low oxygen conditions, various pathogens and pathogen-derived molecules are able to induce HIF-1α even in the presence of ample oxygen [reviewed in Ref. (1, 8–17)]. Inflammatory HIF-1α accumulation under normoxic conditions critically hinges on NF-κB activation (12, 13). HIF-1α transactivation potential, however, is highly contextual. For instance, hypoxic and inflammatory HIF-1α activation, both, result in activation of glycolytic HIF-1α-target genes while only inflammatory HIF-1α activation results in robust induction of inflammatory target genes such as Nos2 (89).
Leishmania and Stabilization of HIF-1α in the Presence of Ample Oxygen
Currently, there is no consensus on whether Leishmania on its own is able to induce HIF-1α accumulation under normoxic conditions (Table 1). Infection of mouse macrophages in vitro with L. amazonensis and L. donovani triggers HIF-1α accumulation under normoxic conditions without requiring any additional inflammatory signals (90, 91). Blockade of this Leishmania-induced HIF-1α accumulation with cadmium chloride or RNAi results in impaired Leishmania recovery under normoxic conditions (90–93). In contrast to these findings and in contrast to stimulation with Toll-like receptor ligands [reviewed in Ref. (1, 8, 9)] or infection with various pathogens such as S. pyogenes (35, 94), M. tuberculosis (95), Histoplasma capsulatum (96), infection of mouse macrophages with L. major does not induce HIF-1α accumulation on its own but requires exogenous stimulation by IFN-γ and/or LPS (36). In line with this, Hammami et al. demonstrated that upon L. donovani-infection HIF-1α accumulation in CD11c+ mouse mononuclear phagocytes in vivo requires interferon regulatory factor-5-dependent inflammatory signaling (97). This suggests that L. donovani infection on its own is not sufficient to induce HIF-1α accumulation in mouse mononuclear phagocytes in vivo as well (97).
The different potential of various Leishmania species to induce HIF-1α accumulation in mononuclear phagocytes is unclear. HIF-1α accumulation in mononuclear cells might, for instance, help Leishmania to fuel its metabolic needs (98, 99). Mechanistically, the differential ability of certain Leishmania species to interfere with NF-κB activation (100–102) might underlie the divergent ability of different Leishmania species to block infection-associated inflammatory HIF-1α-accumulation under normoxic conditions. However, further studies are required to assess this issue.
Role of HIF-1α Expression in Mononuclear Phagocytes
Earlier findings demonstrate a requirement of HIF-1α for NOS2-induction (89, 94, 103–105). In line with this, LPS/INFγ-coactivated HIF-1α-deficient macrophages displayed a diminished NOS2-dependent production of NO under normoxic conditions and impaired killing of L. major in vitro. Conditional targeting of HIF-1α in macrophages revealed that HIF-1α-activation is required for antileishmanial defense against cutaneous L. major-infection under normoxic conditions (36). This was paralleled by a blunted induction of NOS2 in lesional myeloid cells (36). However, it remains unclear whether in addition to Nos2 other HIF-1α-regulated targets are involved in cutaneous containment of Leishmania as well.
While HIF-1α in mononuclear phagocytes is protective in L. major-induced cutaneous leishmaniasis, HIF-1α expression in these cells emerged as detrimental factor in visceral leishmaniasis. Hammami et al. used CD11c Cre-deleter mice to target HIF-1α in Cd11c+ mononuclear phagocytes and to test the contribution of HIF-1α in a L. donovani-induced preclinical model of visceral leishmaniasis (29). In line with an inhibitory role of HIF-1α in DC-mediated T cell responses (106), Hammami et al. provide evidence that in L. donovani-infected mice HIF-1α in CD11c+ cells limits the expansion of short lived effector CD8+ T cells and thereby exacerbates disease at early time points after infections (29). In a recently published follow-up study, they demonstrate that HIF-1α in CD11c+ cells limits the frequency and numbers of granulocyte–monocyte progenitors and inflammatory monocytes. Moreover, HIF-1α in CD11c+ cells enhances the inhibitory function of splenic mononuclear phagocytes while this TF is not involved in tissue neovascularization and splenomegaly (29). Of note, Hammami et al. demonstrate that HIF-1α negatively impacts on the expression of Nos2 in L. donovani-infected mononuclear phagocytes. Discrepancies of this finding to the published literature on the requirement of HIF-1α on Nos2-expression and NO production might be caused by the different context of HIF-1α stabilization (29). This issue, however, demands further detailed investigation. Overall, the data suggest that HIF-1α in CD11c+ cells favors a myeloid-derived suppressor cell-like (MDSC) behavior of these cells. These findings were paralleled by an impaired antileishmanial control in the bone marrow of L. donovani-infected mice at late time points (29).
Taken together, these findings demonstrate that HIF-1α expression in CD11c+ mononuclear phagocytes favors development of visceral leishmaniasis in spleen and bone marrow, while HIF-1α in LysM+ myeloid cells supports defense against cutaneous L. major infection (Table 1). These findings underscore that the role of HIF-1α in myeloid cells is highly dependent on the respective context. HIF-1α is known to promote proinflammatory function of mononuclear phagocytes (35, 36, 94, 95, 105). However, the very same TF is able to induce regulatory and immunosuppressive functions in mononuclear phagocytes as well (107–109). Therefore, it is very likely that the differential context of HIF-1α stabilization in visceral and cutaneous leishmaniasis impacts on the functional consequence of HIF-1α activation. Further studies are needed to uncover the distinct signals that ultimately result in differential HIF-1α-dependent innate immune cell function.
Author Contributions
VS, PN, and FR: contributed to writing of the manuscript. PN: provided the figure. JJ: conception of the manuscript, together with VS, PN, and FR writing of the article.
Conflict of Interest Statement
The authors declare that the research was conducted in the absence of any commercial or financial relationships that could be construed as a potential conflict of interest.
Funding
Jonathan Jantsch receives support from the Deutsche Forschungsgemeinschaft and from the Bayerisches Staatsministerium für Wirtschaft und Medien, Energie und Technologie.
References
1. Jantsch J, Schödel J. Hypoxia and hypoxia-inducible factors in myeloid cell-driven host defense and tissue homeostasis. Immunobiology (2015) 220:305–14. doi:10.1016/j.imbio.2014.09.009
2. Arena ET, Tinevez JY, Nigro G, Sansonetti PJ, Marteyn BS. The infectious hypoxia: occurrence and causes during Shigella infection. Microbes Infect (2017) 19:157–65. doi:10.1016/j.micinf.2016.10.011
3. Semenza GL. A compendium of proteins that interact with HIF-1alpha. Exp Cell Res (2017) 356:128–35. doi:10.1016/j.yexcr.2017.03.041
4. Kaelin WG Jr, Ratcliffe PJ. Oxygen sensing by metazoans: the central role of the HIF hydroxylase pathway. Mol Cell (2008) 30:393–402. doi:10.1016/j.molcel.2008.04.009
5. Semenza GL. Hypoxia-inducible factors in physiology and medicine. Cell (2012) 148:399–408. doi:10.1016/j.cell.2012.01.021
6. Bishop T, Ratcliffe PJ. Signaling hypoxia by hypoxia-inducible factor protein hydroxylases: a historical overview and future perspectives. Hypoxia (Auckl) (2014) 2:197–213. doi:10.2147/HP.S47598
7. Sitkovsky M, Lukashev D. Regulation of immune cells by local-tissue oxygen tension: HIF1 alpha and adenosine receptors. Nat Rev Immunol (2005) 5:712–21. doi:10.1038/nri1685
8. Dehne N, Brune B. HIF-1 in the inflammatory microenvironment. Exp Cell Res (2009) 315:1791–7. doi:10.1016/j.yexcr.2009.03.019
9. Palazon A, Goldrath AW, Nizet V, Johnson RS. HIF transcription factors, inflammation, and immunity. Immunity (2014) 41:518–28. doi:10.1016/j.immuni.2014.09.008
10. Lin N, Simon MC. Hypoxia-inducible factors: key regulators of myeloid cells during inflammation. J Clin Invest (2016) 126:3661–71. doi:10.1172/JCI84426
11. Taylor CT, Doherty G, Fallon PG, Cummins EP. Hypoxia-dependent regulation of inflammatory pathways in immune cells. J Clin Invest (2016) 126:3716–24. doi:10.1172/JCI84433
12. Frede S, Stockmann C, Freitag P, Fandrey J. Bacterial lipopolysaccharide induces HIF-1 activation in human monocytes via p44/42 MAPK and NF-kappaB. Biochem J (2006) 396:517–27. doi:10.1042/BJ20051839
13. Rius J, Guma M, Schachtrup C, Akassoglou K, Zinkernagel AS, Nizet V, et al. NF-kappaB links innate immunity to the hypoxic response through transcriptional regulation of HIF-1alpha. Nature (2008) 453:807–11. doi:10.1038/nature06905
14. Nicholas SA, Sumbayev VV. The role of redox-dependent mechanisms in the downregulation of ligand-induced toll-like receptors 7, 8 and 4-mediated HIF-1 alpha prolyl hydroxylation. Immunol Cell Biol (2010) 88:180–6. doi:10.1038/icb.2009.76
15. Tannahill GM, Curtis AM, Adamik J, Palsson-Mcdermott EM, Mcgettrick AF, Goel G, et al. Succinate is an inflammatory signal that induces IL-1beta through HIF-1alpha. Nature (2013) 496:238–42. doi:10.1038/nature11986
16. Siegert I, Schödel J, Nairz M, Schatz V, Dettmer K, Dick C, et al. Ferritin-mediated iron sequestration stabilizes hypoxia-inducible factor-1alpha upon LPS activation in the presence of ample oxygen. Cell Rep (2015) 13:2048–55. doi:10.1016/j.celrep.2015.11.005
17. Dietz I, Jerchel S, Szaszak M, Shima K, Rupp J. When oxygen runs short: the microenvironment drives host-pathogen interactions. Microbes Infect (2012) 14:311–6. doi:10.1016/j.micinf.2011.11.003
18. Charpentier T, Hammami A, Stager S. Hypoxia inducible factor 1alpha: a critical factor for the immune response to pathogens and Leishmania. Cell Immunol (2016) 309:42–9. doi:10.1016/j.cellimm.2016.06.002
19. Devraj G, Beerlage C, Brune B, Kempf VA. Hypoxia and HIF-1 activation in bacterial infections. Microbes Infect (2017) 19:144–56. doi:10.1016/j.micinf.2016.11.003
20. Friedrich D, Fecher RA, Rupp J, Deepe GS Jr. Impact of HIF-1alpha and hypoxia on fungal growth characteristics and fungal immunity. Microbes Infect (2017) 19:204–9. doi:10.1016/j.micinf.2016.10.008
21. Lodge KM, Thompson AA, Chilvers ER, Condliffe AM. Hypoxic regulation of neutrophil function and consequences for Staphylococcus aureus infection. Microbes Infect (2017) 19:166–76. doi:10.1016/j.micinf.2016.10.005
22. Kanan MW. Mucocutaneous leishmaniasis in guinea-pigs inoculated intravenously with Leishmania enriettii. Preliminary report. Br J Dermatol (1975) 92:663–73. doi:10.1111/j.1365-2133.1975.tb03147.x
23. Eickhoff J, Ishihara S, Jacobsen E. PaO2 by skin electrode. Lancet (1979) 2:1188–9. doi:10.1016/S0140-6736(79)92413-9
24. Peyssonnaux C, Boutin AT, Zinkernagel AS, Datta V, Nizet V, Johnson RS. Critical role of HIF-1alpha in keratinocyte defense against bacterial infection. J Invest Dermatol (2008) 128:1964–8. doi:10.1038/jid.2008.27
25. Hofmann J, Meier RJ, Mahnke A, Schatz V, Brackmann F, Trollmann R, et al. Ratiometric luminescence 2D in vivo imaging and monitoring of mouse skin oxygenation. Methods Appl Fluoresc (2013) 1(4):045002. doi:10.1088/2050-6120/1/4/045002
26. Araújo AP, Arrais-Silva WW, Giorgio S. Infection by Leishmania amazonensis in mice: a potential model for chronic hypoxia. Acta Histochem (2012) 114:797–804. doi:10.1016/j.acthis.2012.01.007
27. Arteel GE, Thurman RG, Yates JM, Raleigh JA. Evidence that hypoxia markers detect oxygen gradients in liver: pimonidazole and retrograde perfusion of rat liver. Br J Cancer (1995) 72:889–95. doi:10.1038/bjc.1995.429
28. Gross MW, Karbach U, Groebe K, Franko AJ, Muellerklieser W. Calibration of misonidazole labeling by simultaneous measurement of oxygen-tension and labeling density in multicellular spheroids. Int J Cancer (1995) 61:567–73. doi:10.1002/ijc.2910610422
29. Hammami A, Abidin BM, Charpentier T, Fabie A, Duguay AP, Heinonen KM, et al. HIF-1alpha is a key regulator in potentiating suppressor activity and limiting the microbicidal capacity of MDSC-like cells during visceral leishmaniasis. PLoS Pathog (2017) 13:e1006616. doi:10.1371/journal.ppat.1006616
30. Mahnke A, Meier RJ, Schatz V, Hofmann J, Castiglione K, Schleicher U, et al. Hypoxia in Leishmania major skin lesions impairs the NO-dependent leishmanicidal activity of macrophages. J Invest Dermatol (2014) 134:2339–46. doi:10.1038/jid.2014.121
31. Campbell EL, Bruyninckx WJ, Kelly CJ, Glover LE, Mcnamee EN, Bowers BE, et al. Transmigrating neutrophils shape the mucosal microenvironment through localized oxygen depletion to influence resolution of inflammation. Immunity (2014) 40:66–77. doi:10.1016/j.immuni.2013.11.020
32. Fatoux-Ardore M, Peysselon F, Weiss A, Bastien P, Pratlong F, Ricard-Blum S. Large-scale investigation of Leishmania interaction networks with host extracellular matrix by surface plasmon resonance imaging. Infect Immun (2014) 82:594–606. doi:10.1128/IAI.01146-13
33. Weinkopff T, Konradt C, Christian DA, Discher DE, Hunter CA, Scott P. Leishmania major infection-induced VEGF-A/VEGFR-2 signaling promotes lymphangiogenesis that controls disease. J Immunol (2016) 197:1823–31. doi:10.4049/jimmunol.1600717
34. Araújo AP, Giorgio S. Immunohistochemical evidence of stress and inflammatory markers in mouse models of cutaneous leishmaniosis. Arch Dermatol Res (2015) 307:671–82. doi:10.1007/s00403-015-1564-0
35. Cramer T, Yamanishi Y, Clausen BE, Forster I, Pawlinski R, Mackman N, et al. HIF-1alpha is essential for myeloid cell-mediated inflammation. Cell (2003) 112:645–57. doi:10.1016/S0092-8674(03)00154-5
36. Schatz V, Strussmann Y, Mahnke A, Schley G, Waldner M, Ritter U, et al. Myeloid cell-derived HIF-1alpha promotes control of Leishmania major. J Immunol (2016) 197:4034–41. doi:10.4049/jimmunol.1601080
37. Fritzsche C, Schleicher U, Bogdan C. Endothelial nitric oxide synthase limits the inflammatory response in mouse cutaneous leishmaniasis. Immunobiology (2010) 215:826–32. doi:10.1016/j.imbio.2010.05.022
38. Kubes P, Suzuki M, Granger DN. Nitric oxide: an endogenous modulator of leukocyte adhesion. Proc Natl Acad Sci U S A (1991) 88:4651–5. doi:10.1073/pnas.88.11.4651
39. Lefer DJ, Jones SP, Girod WG, Baines A, Grisham MB, Cockrell AS, et al. Leukocyte-endothelial cell interactions in nitric oxide synthase-deficient mice. Am J Physiol (1999) 276:H1943–50.
40. Ahluwalia A, Foster P, Scotland RS, Mclean PG, Mathur A, Perretti M, et al. Antiinflammatory activity of soluble guanylate cyclase: cGMP-dependent down-regulation of P-selectin expression and leukocyte recruitment. Proc Natl Acad Sci U S A (2004) 101:1386–91. doi:10.1073/pnas.0304264101
41. Rodriguez-Miguelez P, Lima-Cabello E, Martinez-Florez S, Almar M, Cuevas MJ, Gonzalez-Gallego J. Hypoxia-inducible factor-1 modulates the expression of vascular endothelial growth factor and endothelial nitric oxide synthase induced by eccentric exercise. J Appl Physiol (1985) (2015) 118:1075–83. doi:10.1152/japplphysiol.00780.2014
42. Horst AK, Bickert T, Brewig N, Ludewig P, Van Rooijen N, Schumacher U, et al. CEACAM1+ myeloid cells control angiogenesis in inflammation. Blood (2009) 113:6726–36. doi:10.1182/blood-2008-10-184556
43. Horta MF, Mendes BP, Roma EH, Noronha FS, Macedo JP, Oliveira LS, et al. Reactive oxygen species and nitric oxide in cutaneous leishmaniasis. J Parasitol Res (2012) 2012:203818. doi:10.1155/2012/203818
44. Scott P, Novais FO. Cutaneous leishmaniasis: immune responses in protection and pathogenesis. Nat Rev Immunol (2016) 16:581–92. doi:10.1038/nri.2016.72
45. Hu S, Wei W, Korner H. The role of monocytes in models of infection by protozoan parasites. Mol Immunol (2017) 88:174–84. doi:10.1016/j.molimm.2017.06.020
46. Anand RJ, Gribar SC, Li J, Kohler JW, Branca MF, Dubowski T, et al. Hypoxia causes an increase in phagocytosis by macrophages in a HIF-1alpha-dependent manner. J Leukoc Biol (2007) 82:1257–65. doi:10.1189/jlb.0307195
47. Elia AR, Cappello P, Puppo M, Fraone T, Vanni C, Eva A, et al. Human dendritic cells differentiated in hypoxia down-modulate antigen uptake and change their chemokine expression profile. J Leukoc Biol (2008) 84:1472–82. doi:10.1189/jlb.0208082
48. Laskay T, Van Zandbergen G, Solbach W. Neutrophil granulocytes – Trojan horses for Leishmania major and other intracellular microbes? Trends Microbiol (2003) 11:210–4. doi:10.1016/S0966-842X(03)00075-1
49. Walmsley SR, Print C, Farahi N, Peyssonnaux C, Johnson RS, Cramer T, et al. Hypoxia-induced neutrophil survival is mediated by HIF-1alpha-dependent NF-kappaB activity. J Exp Med (2005) 201:105–15. doi:10.1084/jem.20040624
50. Monceaux V, Chiche-Lapierre C, Chaput C, Witko-Sarsat V, Prevost MC, Taylor CT, et al. Anoxia and glucose supplementation preserve neutrophil viability and function. Blood (2016) 128:993–1002. doi:10.1182/blood-2015-11-680918
51. von Stebut E, Tenzer S. Cutaneous leishmaniasis: distinct functions of dendritic cells and macrophages in the interaction of the host immune system with Leishmania major. Int J Med Microbiol (2017). doi:10.1016/j.ijmm.2017.11.002
52. Naldini A, Morena E, Pucci A, Miglietta D, Riboldi E, Sozzani S, et al. Hypoxia affects dendritic cell survival: role of the hypoxia-inducible factor-1alpha and lipopolysaccharide. J Cell Physiol (2012) 227:587–95. doi:10.1002/jcp.22761
53. Qu X, Yang MX, Kong BH, Qi L, Lam QL, Yan S, et al. Hypoxia inhibits the migratory capacity of human monocyte-derived dendritic cells. Immunol Cell Biol (2005) 83:668–73. doi:10.1111/j.1440-1711.2005.01383.x
54. Jantsch J, Chakravortty D, Turza N, Prechtel AT, Buchholz B, Gerlach RG, et al. Hypoxia and hypoxia-inducible factor-1 alpha modulate lipopolysaccharide-induced dendritic cell activation and function. J Immunol (2008) 180:4697–705. doi:10.4049/jimmunol.180.7.4697
55. Spirig R, Djafarzadeh S, Regueira T, Shaw SG, Von Garnier C, Takala J, et al. Effects of TLR agonists on the hypoxia-regulated transcription factor HIF-1alpha and dendritic cell maturation under normoxic conditions. PLoS One (2010) 5:e0010983. doi:10.1371/journal.pone.0010983
56. Kohler T, Reizis B, Johnson RS, Weighardt H, Forster I. Influence of hypoxia-inducible factor 1alpha on dendritic cell differentiation and migration. Eur J Immunol (2012) 42:1226–36. doi:10.1002/eji.201142053
57. Bogdan C. Natural killer cells in experimental and human leishmaniasis. Front Cell Infect Microbiol (2012) 2:69. doi:10.3389/fcimb.2012.00069
58. Balsamo M, Manzini C, Pietra G, Raggi F, Blengio F, Mingari MC, et al. Hypoxia downregulates the expression of activating receptors involved in NK-cell-mediated target cell killing without affecting ADCC. Eur J Immunol (2013) 43:2756–64. doi:10.1002/eji.201343448
59. Sarkar S, Germeraad WT, Rouschop KM, Steeghs EM, Van Gelder M, Bos GM, et al. Hypoxia induced impairment of NK cell cytotoxicity against multiple myeloma can be overcome by IL-2 activation of the NK cells. PLoS One (2013) 8:e64835. doi:10.1371/journal.pone.0064835
60. Wolter F, Glassner A, Kramer B, Kokordelis P, Finnemann C, Kaczmarek DJ, et al. Hypoxia impairs anti-viral activity of natural killer (NK) cells but has little effect on anti-fibrotic NK cell functions in hepatitis C virus infection. J Hepatol (2015) 63:1334–44. doi:10.1016/j.jhep.2015.08.008
61. Krzywinska E, Kantari-Mimoun C, Kerdiles Y, Sobecki M, Isagawa T, Gotthardt D, et al. Loss of HIF-1alpha in natural killer cells inhibits tumour growth by stimulating non-productive angiogenesis. Nat Commun (2017) 8:1597. doi:10.1038/s41467-017-01599-w
62. Abidin BM, Hammami A, Stager S, Heinonen KM. Infection-adapted emergency hematopoiesis promotes visceral leishmaniasis. PLoS Pathog (2017) 13:e1006422. doi:10.1371/journal.ppat.1006422
63. Romano A, Carneiro MBH, Doria NA, Roma EH, Ribeiro-Gomes FL, Inbar E, et al. Divergent roles for Ly6C+CCR2+CX3CR1+ inflammatory monocytes during primary or secondary infection of the skin with the intra-phagosomal pathogen Leishmania major. PLoS Pathog (2017) 13:e1006479. doi:10.1371/journal.ppat.1006479
64. Goncalves R, Zhang X, Cohen H, Debrabant A, Mosser DM. Platelet activation attracts a subpopulation of effector monocytes to sites of Leishmania major infection. J Exp Med (2011) 208:1253–65. doi:10.1084/jem.20101751
65. Novais FO, Nguyen BT, Beiting DP, Carvalho LP, Glennie ND, Passos S, et al. Human classical monocytes control the intracellular stage of Leishmania braziliensis by reactive oxygen species. J Infect Dis (2014) 209:1288–96. doi:10.1093/infdis/jiu013
66. Srivastav S, Basu Ball W, Gupta P, Giri J, Ukil A, Das PK. Leishmania donovani prevents oxidative burst-mediated apoptosis of host macrophages through selective induction of suppressors of cytokine signaling (SOCS) proteins. J Biol Chem (2014) 289:1092–105. doi:10.1074/jbc.M113.496323
67. Murray HW, Spitalny GL, Nathan CF. Activation of mouse peritoneal macrophages in vitro and in vivo by interferon-gamma. J Immunol (1985) 134:1619–22.
68. Liew FY, Li Y, Millott S. Tumor necrosis factor-alpha synergizes with IFN-gamma in mediating killing of Leishmania major through the induction of nitric oxide. J Immunol (1990) 145:4306–10.
69. Wilhelm P, Ritter U, Labbow S, Donhauser N, Rollinghoff M, Bogdan C, et al. Rapidly fatal leishmaniasis in resistant C57BL/6 mice lacking TNF. J Immunol (2001) 166:4012–9. doi:10.4049/jimmunol.166.6.4012
70. Bogdan C. Nitric oxide synthase in innate and adaptive immunity: an update. Trends Immunol (2015) 36:161–78. doi:10.1016/j.it.2015.01.003
71. Olekhnovitch R, Bousso P. Induction, propagation, and activity of host nitric oxide: lessons from Leishmania infection. Trends Parasitol (2015) 31:653–64. doi:10.1016/j.pt.2015.08.001
72. Murray HW, Nathan CF. Macrophage microbicidal mechanisms in vivo: reactive nitrogen versus oxygen intermediates in the killing of intracellular visceral Leishmania donovani. J Exp Med (1999) 189:741–6. doi:10.1084/jem.189.4.741
73. White JK, Mastroeni P, Popoff JF, Evans CA, Blackwell JM. Slc11a1-mediated resistance to Salmonella enterica serovar Typhimurium and Leishmania donovani infections does not require functional inducible nitric oxide synthase or phagocyte oxidase activity. J Leukoc Biol (2005) 77:311–20. doi:10.1189/jlb.0904546
74. Ding AH, Nathan CF, Stuehr DJ. Release of reactive nitrogen intermediates and reactive oxygen intermediates from mouse peritoneal macrophages. Comparison of activating cytokines and evidence for independent production. J Immunol (1988) 141:2407–12.
75. Green SJ, Meltzer MS, Hibbs JB Jr, Nacy CA. Activated macrophages destroy intracellular Leishmania major amastigotes by an L-arginine-dependent killing mechanism. J Immunol (1990) 144:278–83.
76. Diefenbach A, Schindler H, Donhauser N, Lorenz E, Laskay T, Macmicking J, et al. Type 1 interferon (IFNalpha/beta) and type 2 nitric oxide synthase regulate the innate immune response to a protozoan parasite. Immunity (1998) 8:77–87. doi:10.1016/S1074-7613(00)80460-4
77. Diefenbach A, Schindler H, Rollinghoff M, Yokoyama WM, Bogdan C. Requirement for type 2 NO synthase for IL-12 signaling in innate immunity. Science (1999) 284:951–5. doi:10.1126/science.284.5416.951
78. Olekhnovitch R, Ryffel B, Muller AJ, Bousso P. Collective nitric oxide production provides tissue-wide immunity during Leishmania infection. J Clin Invest (2014) 124:1711–22. doi:10.1172/JCI72058
79. Albina JE, Henry WL Jr, Mastrofrancesco B, Martin BA, Reichner JS. Macrophage activation by culture in an anoxic environment. J Immunol (1995) 155:4391–6.
80. Robinson MA, Baumgardner JE, Otto CM. Oxygen-dependent regulation of nitric oxide production by inducible nitric oxide synthase. Free Radic Biol Med (2011) 51:1952–65. doi:10.1016/j.freeradbiomed.2011.08.034
81. Wiese M, Gerlach RG, Popp I, Matuszak J, Mahapatro M, Castiglione K, et al. Hypoxia-mediated impairment of the mitochondrial respiratory chain inhibits the bactericidal activity of macrophages. Infect Immun (2012) 80(4):1455–66. doi:10.1128/IAI.05972-11
82. Sen Santara S, Roy J, Mukherjee S, Bose M, Saha R, Adak S. Globin-coupled heme containing oxygen sensor soluble adenylate cyclase in Leishmania prevents cell death during hypoxia. Proc Natl Acad Sci U S A (2013) 110:16790–5. doi:10.1073/pnas.1304145110
83. Kong F, Saldarriaga OA, Spratt H, Osorio EY, Travi BL, Luxon BA, et al. Transcriptional profiling in experimental visceral leishmaniasis reveals a broad splenic inflammatory environment that conditions macrophages toward a disease-promoting phenotype. PLoS Pathog (2017) 13:e1006165. doi:10.1371/journal.ppat.1006165
84. Nascimento MS, Carregaro V, Lima-Junior DS, Costa DL, Ryffel B, Duthie MS, et al. Interleukin 17A acts synergistically with interferon gamma to promote protection against Leishmania infantum infection. J Infect Dis (2015) 211:1015–26. doi:10.1093/infdis/jiu531
85. Colhone MC, Arrais-Silva WW, Picoli C, Giorgio S. Effect of hypoxia on macrophage infection by Leishmania amazonensis. J Parasitol (2004) 90:510–5. doi:10.1645/GE-3286
86. Degrossoli A, Giorgio S. Functional alterations in macrophages after hypoxia selection. Exp Biol Med (Maywood) (2007) 232:88–95. doi:10.3181/003797-207-2320088
87. Walsh MJ, Blum JJ. Effects of hypoxia and acute osmotic stress on intermediary metabolism in Leishmania promastigotes. Mol Biochem Parasitol (1992) 50:205–14. doi:10.1016/0166-6851(92)90217-8
88. Blum JJ. Energy metabolism in Leishmania. J Bioenerg Biomembr (1994) 26:147–55. doi:10.1007/BF00763063
89. Jantsch J, Wiese M, Schodel J, Castiglione K, Glasner J, Kolbe S, et al. Toll-like receptor activation and hypoxia use distinct signaling pathways to stabilize hypoxia-inducible factor 1alpha (HIF1A) and result in differential HIF1A-dependent gene expression. J Leukoc Biol (2011) 90:551–62. doi:10.1189/jlb.1210683
90. Degrossoli A, Bosetto MC, Lima CB, Giorgio S. Expression of hypoxia-inducible factor 1alpha in mononuclear phagocytes infected with Leishmania amazonensis. Immunol Lett (2007) 114:119–25. doi:10.1016/j.imlet.2007.09.009
91. Singh AK, Mukhopadhyay C, Biswas S, Singh VK, Mukhopadhyay CK. Intracellular pathogen Leishmania donovani activates hypoxia inducible factor-1 by dual mechanism for survival advantage within macrophage. PLoS One (2012) 7:e38489. doi:10.1371/journal.pone.0038489
92. Lemaire J, Mkannez G, Guerfali FZ, Gustin C, Attia H, Sghaier RM, et al. MicroRNA expression profile in human macrophages in response to Leishmania major infection. PLoS Negl Trop Dis (2013) 7:e2478. doi:10.1371/journal.pntd.0002478
93. Guerfali FZ, Lemaire J, Mkannez G, Renard P, Laouini D. Letter to the editor: hypoxia inducible factor 1alpha: a critical factor for the immune response to pathogens and Leishmania. Cell Immunol (2016) 310:211. doi:10.1016/j.cellimm.2016.08.009
94. Peyssonnaux C, Datta V, Cramer T, Doedens A, Theodorakis EA, Gallo RL, et al. HIF-1alpha expression regulates the bactericidal capacity of phagocytes. J Clin Invest (2005) 115:1806–15. doi:10.1172/JCI23865
95. Braverman J, Sogi KM, Benjamin D, Nomura DK, Stanley SA. HIF-1alpha is an essential mediator of IFN-gamma-dependent immunity to Mycobacterium tuberculosis. J Immunol (2016) 197:1287–97. doi:10.4049/jimmunol.1600266
96. Fecher RA, Horwath MC, Friedrich D, Rupp J, Deepe GS Jr. Inverse correlation between IL-10 and HIF-1alpha in macrophages infected with Histoplasma capsulatum. J Immunol (2016) 197:565–79. doi:10.4049/jimmunol.1600342
97. Hammami A, Charpentier T, Smans M, Stager S. IRF-5-mediated inflammation limits CD8+ T cell expansion by inducing HIF-1alpha and impairing dendritic cell functions during Leishmania infection. PLoS Pathog (2015) 11:e1004938. doi:10.1371/journal.ppat.1004938
98. Naderer T, Ellis MA, Sernee MF, De Souza DP, Curtis J, Handman E, et al. Virulence of Leishmania major in macrophages and mice requires the gluconeogenic enzyme fructose-1,6-bisphosphatase. Proc Natl Acad Sci U S A (2006) 103:5502–7. doi:10.1073/pnas.0509196103
99. Rodriguez-Contreras D, Aslan H, Feng X, Tran K, Yates PA, Kamhawi S, et al. Regulation and biological function of a flagellar glucose transporter in Leishmania mexicana: a potential glucose sensor. FASEB J (2015) 29:11–24. doi:10.1096/fj.14-251991
100. Ghosh S, Bhattacharyya S, Sirkar M, Sa GS, Das T, Majumdar D, et al. Leishmania donovani suppresses activated protein 1 and NF-kappaB activation in host macrophages via ceramide generation: involvement of extracellular signal-regulated kinase. Infect Immun (2002) 70:6828–38. doi:10.1128/IAI.70.12.6828-6838.2002
101. Ben-Othman R, Guizani-Tabbane L, Dellagi K. Leishmania initially activates but subsequently down-regulates intracellular mitogen-activated protein kinases and nuclear factor-kappaB signaling in macrophages. Mol Immunol (2008) 45:3222–9. doi:10.1016/j.molimm.2008.02.019
102. Gregory DJ, Godbout M, Contreras I, Forget G, Olivier M. A novel form of NF-kappaB is induced by Leishmania infection: involvement in macrophage gene expression. Eur J Immunol (2008) 38:1071–81. doi:10.1002/eji.200737586
103. Melillo G, Musso T, Sica A, Taylor LS, Cox GW, Varesio L. A hypoxia-responsive element mediates a novel pathway of activation of the inducible nitric oxide synthase promoter. J Exp Med (1995) 182:1683–93. doi:10.1084/jem.182.6.1683
104. Melillo G, Taylor LS, Brooks A, Musso T, Cox GW, Varesio L. Functional requirement of the hypoxia-responsive element in the activation of the inducible nitric oxide synthase promoter by the iron chelator desferrioxamine. J Biol Chem (1997) 272:12236–43. doi:10.1074/jbc.272.18.12236
105. Takeda N, O’Dea EL, Doedens A, Kim JW, Weidemann A, Stockmann C, et al. Differential activation and antagonistic function of HIF-{alpha} isoforms in macrophages are essential for NO homeostasis. Genes Dev (2010) 24:491–501. doi:10.1101/gad.1881410
106. Lawless SJ, Kedia-Mehta N, Walls JF, Mcgarrigle R, Convery O, Sinclair LV, et al. Glucose represses dendritic cell-induced T cell responses. Nat Commun (2017) 8:15620. doi:10.1038/ncomms15620
107. Corzo CA, Condamine T, Lu L, Cotter MJ, Youn JI, Cheng P, et al. HIF-1{alpha} regulates function and differentiation of myeloid-derived suppressor cells in the tumor microenvironment. J Exp Med (2010) 207:2439–53. doi:10.1084/jem.20100587
108. Doedens AL, Stockmann C, Rubinstein MP, Liao D, Zhang N, Denardo DG, et al. Macrophage expression of hypoxia-inducible factor-1 alpha suppresses T-cell function and promotes tumor progression. Cancer Res (2010) 70:7465–75. doi:10.1158/0008-5472.CAN-10-1439
Keywords: hypoxia-inducible factor 1, leishmaniasis, macrophages, oxygen, Nos2, hypoxia
Citation: Schatz V, Neubert P, Rieger F and Jantsch J (2018) Hypoxia, Hypoxia-Inducible Factor-1α, and Innate Antileishmanial Immune Responses. Front. Immunol. 9:216. doi: 10.3389/fimmu.2018.00216
Received: 23 October 2017; Accepted: 25 January 2018;
Published: 22 February 2018
Edited by:
Heinrich Korner, University of Tasmania, AustraliaReviewed by:
Werner Solbach, University of Lübeck, GermanySimona Stäger, Institut national de la recherche scientifique (INRS), Canada
Angel L. Corbi, Consejo Superior de Investigaciones Científicas (CSIC), Spain
Copyright: © 2018 Schatz, Neubert, Rieger and Jantsch. This is an open-access article distributed under the terms of the Creative Commons Attribution License (CC BY). The use, distribution or reproduction in other forums is permitted, provided the original author(s) and the copyright owner are credited and that the original publication in this journal is cited, in accordance with accepted academic practice. No use, distribution or reproduction is permitted which does not comply with these terms.
*Correspondence: Jonathan Jantsch, am9uYXRoYW4uamFudHNjaEB1a3IuZGU=