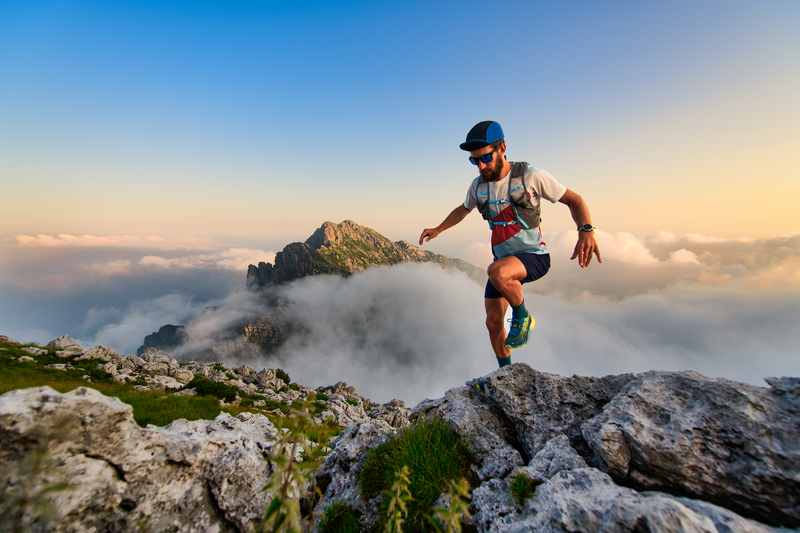
95% of researchers rate our articles as excellent or good
Learn more about the work of our research integrity team to safeguard the quality of each article we publish.
Find out more
MINI REVIEW article
Front. Immunol. , 22 May 2017
Sec. Inflammation
Volume 8 - 2017 | https://doi.org/10.3389/fimmu.2017.00590
This article is part of the Research Topic Interaction of nanomaterials with the immune system: role in nanosafety and nanomedicine View all 20 articles
The inflammatory response, mediated by tissue-resident or newly recruited macrophages, is an underlying pathophysiological condition for many diseases, including diabetes, obesity, neurodegeneration, atherosclerosis, and cancer. Paradoxically, inflammation is a double-edged sword in oncology. Macrophages are, generally speaking, the major drivers of inflammatory insult. For many solid tumors, high density of cells expressing macrophage-associated markers have generally been found in association with a poor clinical outcome, characterized by inflamed microenvironment, a high level of dissemination and resistance to conventional chemotherapies. On another hand, radiation treatment also triggers an inflammatory response in tumors (often referred to as pseudoprogression), which can be associated with a positive treatment response. As such, non-invasive imaging of cancer inflammation and tumor-associated macrophages (TAMs) provides a revolutionary diagnostic tool and monitoring strategy for anti-inflammatory, immuno- and radiotherapies. Recently, quantitative T2-weighted magnetic resonance imaging (qT2wMRI), using injection of superparamagnetic iron oxide nanoparticles (SPIONs), has been reported for the assessment of TAMs non-invasively in animal models and in human trials. The SPIONs are magnetic resonance imaging (MRI) contrast agents that significantly decrease T2 MR relaxation times in inflamed tissues due to the macrophage-specific uptake and retention. It has been shown that macrophage-populated tumors and metastases will accumulate iron oxide nanoparticles and decrease T2-relaxation time that will result in a negative (dark) contrast in qT2wMRI. Non-invasive imaging of TAMs using SPION holds a great promise for staging the inflammatory microenvironment of primary and metastatic tumors as well monitoring the treatment response of cancer patients treated with radiation and immunotherapy.
The tumor microenvironment subsidizes to tumor progression, invasion, metabolic reprograming, and resistance to therapy. In the past decade, it has become increasingly clear that immune-competent cells, including macrophages, represent one of the main contributors to the aggressive tumor milieu (1, 2). Macrophages are phagocyting cells that penetrate into and reside in the affected tissue; they originate from circulating blood monocytes (3). Two distinct classes of macrophages have been described: classically activated M1 macrophages and alternatively activated M2 macrophages (4, 5). In most tumors, the inflamed microenvironment is driven by M2-type macrophages (6, 7).
Given the growing body of evidence of the tumor-associated macrophages (TAMs) playing a significant role in tumorigenesis, a non-invasive assessment of TAMs to detect the level of tumor inflammation becomes a critical and limiting factor. The gold standard for TAM assessment, as of now, is immunohistochemistry, histological examination, and, in rare case, flow cytometry on excised biopsies—all techniques can be applied ex vivo only (8). A biopsy comes at great costs to the patient, its invasiveness can have detrimental health consequences, and since it is very challenging, if not impossible, to perform sequential biopsies, these protocols are limited in the assessment of TAM infiltration over time. Unfortunately, the circulating levels of monocytes, which can be assessed by semi-invasive venipuncture (phlebotomy), do not bear any therapeutic values for correlating with the levels of TAMs. Molecular and cellular imaging is a fast growing area in translational and clinical research. Dozens of novel molecularly targeted imaging probes have been tested in animal models of cancer and some of them are successfully used in human imaging (9–11). Fortunately, macrophages are well known as “professional phagocytes” and are responsible for “cleaning” various exogenous microbes, toxins, and nanoparticles. Also, macrophages are responsible for endogenous and exogenous iron metabolism. Fortunate again, iron-based nanoparticles have been known as T2-weighted contrast for magnetic resonance imaging (MRI). The recent studies have shown that superparamagnetic iron oxide nanoparticles (SPIONs) have a potential for non-invasive T2-weighted MRI assessment on tissue residential macrophages, including TAMs (12). This approach has a high-translational potential, since several of the existing SPION agents are approved in Europe for MR imaging and commercially available for human use. Ferumoxytol is another ultrasmall SPION formulation (with an average particle size of 25–30 nm) that is approved by the US Food and Drug Administration as an iron supplement for intravenous treatment of iron deficiency in renal failure patients (13). Ferumoxytol has superb magnetic properties and has been safely used in animal and human trials as an off-label MRI contrast agent (12, 14–22).
The relationship between chronic inflammation and cancer development was recognized well before the molecular origins of both diseases had been deciphered—Rudolph Virchow postulated an association between these two diseases in the 1860s (23). Inflammation is triggered by a cellular response, mediated mostly by neutrophils and macrophages, in response to pathophysiological stimuli. In general, macrophages are large white blood cells that ingest pathogens, microbes, and other invading substances. While neutrophils represent the first immune defense during the acute inflammation stage, the macrophages are predominant in chronic inflammation. All macrophages, including TAMs, are recruited through the local expression of chemoattractant stimuli such as macrophage chemoattractant protein 1 and colony-stimulating factor 1 (24–27). Overexpression of both these factors is correlated with poor prognosis in various tumors, including most aggressive breast cancer, pancreatic adenocarcinomas, lung cancer, and high-grade gliomas. In many solid tumor types, poor prognosis directly correlates with the abundance of TAMs. In breast cancer, for example, TAMs play a crucial role in epithelial/stromal cross talk, as shown for invasive ductal carcinoma and ductal carcinoma in situ (28). Macrophage depletion in animal models leads to the impaired lung tumor growth and the decreased metastatic spread to the lung from breast cancer (29–31). TAMs have been directly linked to matrix remodeling, angiogenesis, stimulation of tumor growth, and motility (27, 32, 33)—all these functions are also reported during wound healing; as such, tumors are often described as “wounds that never heal” (1, 7). Similar to Virchow, our contemporary scientists, Gonda et al. concluded that chronic inflammation results in a myriad of molecular event that produce a microenvironment that is favorable for the development of cancer (34). Agents that control the inflammatory cascade, such as ibuprofen and other non-steroidal anti-inflammatory drugs, are thought to reduce cancer risk or enhance other anticancer treatments.
In another scenario, TAMs can be recruited from circulating monocytes as a result of therapy-induced apoptosis resulting in tumor inflammation after, for example, radiation or chemotherapy. Radiation induces a genetic signature of chronic inflammation, which is enriched in genes regulating transendothelial migration, monocyte maturation, and leukocyte chemoattraction (35–37). In this case, surprisingly, the recruited macrophages can accelerate antitumoral effects of radiation treatment (38). As such, a non-invasive assessment of TAMs can serve a surrogate marker for a specific and early response to several anticancer therapies.
Recently, several phenotypes (or “states”) of macrophages/macrophage activation have been identified: two most extreme states are known as antitumor M1 and protumor M2 macrophages (39). M2-type TAMs promote tumor growth, angiogenesis, and metastases by promoting high-level expression of epidermal growth factor receptor and secretion of vascular endothelial growth factors. M1 phenotype, on the other hand, can directly or indirectly mediate tumor phagocytosis. Two classes have distinct molecular signatures—the antitumor M1 phenotype has relatively low IL-10 and high IL-12 expression, whereas protumor M2 macrophages express high IL-10 and low IL-12 levels. It has been hypothesized that the bad “protumor” M2 phenotype is responsible for intrinsically inflamed solid tumors promoting fast cell proliferation, angiogenesis, dissemination, and immunosuppression, while the good M1 macrophages mature in response to radiation and chemotherapy and, as such, can help other immunocompetent T-cells to recognized and fight cancer (40, 41). Nevertheless, clinically, most of the current IHC protocols are not capable to distinguish between two TAM phenotypes. Hence, it becomes increasingly imperative to non-invasively characterize patient’s tumor microenvironment for the presence of TAMs in order to stratify the patients to TAM-depleting and/or -directed therapies and to repeatedly monitor their treatment response.
The cytoplasm of a macrophage contains granules (also called packets) consisting of several enzymes and chemicals that are wrapped in a membrane; its membrane has an arsenal of highly effective scavenger receptors. They allow the macrophage to engulf a broad spectrum of invading microorganisms, pathogens, and nanoparticles as well as endogenous cell debris and apoptotic bodies. In fact, macrophages are known as “professional phagocytes” (42), and their phagocyting and pro-inflammatory abilities are directly linked to each other. There are some differences in terminology, which are related to the size of the digested material—some nanoscientists have introduced the term of “pinocytosis” for the uptake of soluble material or a nanoparticle, in contrast to the uptake of large material (“phagocytosis”) (43). But the fact remains undisputable—the macrophages take their responsibility of engulfing large and small nanoparticles very seriously; their macrophage scavenger receptors represent a very efficient system for recognizing a broad spectrum of surface modification and coating.
Fortunately for the MRI scientific community, iron-based nanoparticles represent even a higher level of attraction for the hard-working macrophages. Indeed, the macrophages are trained to maintain endogenous iron homeostasis while recycling and storing iron from senescent erythrocytes and other damaged cells (44, 45). They are very capable to store excessive levels of iron and, in response to systemic iron requirements, they can also release iron from their intracellular compartment into plasma. Therefore, after digesting (pinocyting) an iron-containing nanoparticle (SPION), a macrophage will dutifully retain iron as long as the circulating iron load remains within its physiological range. This macrophage-retained iron load can be easily detected by T2-weighted MRI.
Modern oncologic imaging offers a variety of different modalities for the non-invasive detection and characterization of cancerous lesions (46–50). The common modalities include MRI, computed tomography, ultrasound, positron emission tomography (PET), single-photon emission computerized tomography, and optical imaging. Each modality has its advantages and disadvantages (46) and offers various non-invasive imaging endpoints related to tumor dimensions, tissue cellularity, angiogenesis, cancer metabolism, proliferation, and metastatic spread, just to mention a few. MRI is a non-invasive radiological technique with no ionizing radiation and high-spatial resolution, which is widely clinically used to detect, follow, and characterize solid tumors and metastases. MRI has complex physics and is based on physical properties of protons (mostly hydrogens) in a strong external magnetic field. Since water (H2O) is the main metabolite in all mammalian tissues, MRI detects small but distinct changes in spin frequencies of water hydrogens based on their surroundings when exposed to a high-magnetic field and radiofrequency excitation. The typical magnetic strength of human MRI scanner is 1.5 and 3 T, and for small animal imaging 4.7 and 7 T—however, the scanners tuned to even higher field, such as 9.4 T, 14 even 20 T, can be found in dedicated research facilities. One of the main strength of MRI is its ability to detect small changes (intrinsic contrast) within soft tissues and cell populations, which can be further enhanced by the use of intravenous contrast agents.
It is important to understand the relationship between superparamagnetic nanoparticles and their effect on MR relaxation of the surrounding tissue water. Contrast agents can be principally divided into T1- and T2-relaxing contrast agents (Figure 1A). Paramagnetic contrast agents, such as gadolinium chelates also known as gadolinium-based contrast agents (GBCA), which are broadly used in the clinic, they predominantly shorten the spin–lattice T1 relaxation time (51). The shortening in T1 relaxation produces an increased signal intensity on a T1-weighted MRI images (Figures 1A–C). Clinically used GBCA (Magnevist, Omniscan, Mutihance, etc.) are intravascular contrast agents, not tailored to any specific cell type, and their specific accumulation in tumorous tissues is strictly based on liking vasculature of cancer. Figure 1C demonstrates an appearance of a small brain metastasis in GBCA-enhanced T1-weighted MRI (bright yellow arrow) in a melanoma patient.
Figure 1. Two major classes of magnetic resonance imaging (MRI) contrast agents: (A) paramagnetic gadolinium-based contrast agents (GBCA) are considered T1-positive contrast agents, by decreasing the spin–lattice T1 relaxation time, they produce bright T1 images; superparamagnetic iron oxide (SPION) is negative T2 contrast, iron oxide decreases the spin–spin T2-relaxation time producing darkening of T2-weighted images; (B) pre- and (C) post-GBCA T1-weighted MRI on a brain metastasis in a melanoma patient (15 min postinjection); (D) pre- and (E) post-SPION T2-weighted MRI on inflamed mouse mammary gland tumors (24 h postinjection).
On the other hand, all T2-shortening contrast agents consist of iron oxide nanoparticles, which are known as superparamagnetic (hence, the SPION abbreviation). By reducing the spin–spin T2-relaxation time of surrounding tissue water, the SPION (Feridex, Resovist, Ferumoxytol, etc.) produce darker contrast on T2-weighted MRI (Figures 1A,D,E) (52). Most importantly, unlike gadolinium, iron is a naturally occurring element in human bodies with low toxicity; and, the SPION are highly attractive to all phagocyting cells including macrophages (as well as Kupffer cells and the reticuloendothelial system, RES). The precise changes in T2-relaxation times (calculated from quantitative T2-weighted magnetic resonance imaging) can be used as a semi-quantitative assessment of TAM presence in a cancerous lesion. The Figure 1E shows a pronounced darkening in the inflamed mammary gland of a mouse by T2-weighted MRI (dark yellow arrows).
Gadolinium-based contrast agents are, without any doubt, the major class of MRI contrast agents used in the clinic. Over the past 25 years, more than 100 million patients have undergone GBCA-enhanced T1-MRI. However, increased concerns about gadolinium deposition and toxicity of free gadolinium have impacted how GBCA are currently used. A severe side effect, known as nephrogenic systemic fibrosis, is associated with decreased renal clearance of GBCA in renally impaired patients (53), since acute toxicity of free gadolinium has been known for several decades. It can significantly limit the GBCA use for MRI in cancer patients with chemotherapy-induced low glomerulofiltration rate. Most recently, a very concerning study was published in Radiology on residual gadolinium deposition in the brain of patients after multiple GBCA injections for MRI (54). Alternative contrast agents to gadolinium chelates are being discussed, and the SPIONs are being increasingly used for various clinical scenarios in the recent years (20, 55).
Initially, all SPIONs were used for diagnostic liver imaging (mostly in hepatocellular carcinoma or liver metastases) and considered as safe MRI contrast (56). Their use was based on the high uptake of the SPION by the Kupffer cells: a drop of T2 signal was seen in normal hepatic parenchyma due to the Kupffer cell uptake, with no signal changes in liver lesions. There were also attempt to stage lymph node metastases due to SPION retention and phagocytosis in the RES and lymph nodes (57, 58). As all nanoparticles, SPIONs have enhanced permeability and retention (EPR) in solid tumors (52). However, the previous generation of SPION with larger particle sizes (around and above 50 nm) had been mostly captured by the RES, decreasing their half-life times and, as such, their penetration into tumors.
Ferumoxytol is a colloid-based ultrasmall SPION, which consists of an iron oxide core with a size of ca. 6 nm and a carboxymethyldextran coat, resulting in a hydrodynamic diameter of 30 nm. Unlike larger SPIONs (e.g., Resovist), ferumoxytol has a prolonged circulating half-life time (>14 h in humans and 2 h in rodents), mostly because of it partially escaping phagocytosis by the RES (spleen, liver, and bone marrow). As such, ferumoxytol has a favorable EPR profile and a potential for higher tumoral biodistribution. It slowly leaks across highly permeable tumor vasculature into the tumor interstitial space; after that, ferumoxytol nanoparticles are attacked by the TAMs and slowly phagocyted—a process that takes hours. Our studies and those from others have shown that the pick of iron accumulation in a tumor (Tmax) lies between 16 and 24 h after intravenous injection of ferumoxytol (12, 15, 59). Figure 2A shows representative quantitative T2-MRI maps of a high-grade inflamed glioma allograft (a mouse flank model) before (top) and 24 h after ferumoxytol infusion (bottom). The T2 histograms on Figure 2B show a clear decrease in tumoral T2-rerelaxation times after 24 h of SPION injection (from 58 to 44 ms), with all iron being completely localized intracellularly in TAMs. The same effect can be seen in humans; a residual reduction in T2-relaxation times in inflamed cancerous lesions can sometimes be observed a week after ferumoxytol administration (15, 19, 60).
Figure 2. (A) T2-weighted magnetic resonance imaging (MRI) maps showing spatial distribution of nanoparticles (as dark signal intensities) in a high-grade glioma inflamed allograft in a mouse; (B) quantitative assessment of T2-weighted MRI presented as spatial T2 histograms with a T2 pre-contrast tumoral value of 58 ms; and 44 ms post-contrast. Pre-contrast images/histograms (top) and post-superparamagnetic iron oxide nanoparticle data (bottom) are 24 h apart.
Nanotechnology and nanomedicine have been increasingly utilized in translational and clinical practice in the past decade. This development has been supported by both federal and pharmaceutical funds, ever since, in 2004, the National Cancer Institute announced the Alliance for Nanotechnology in Cancer (61). The majority of nanoparticle research in cancer is focused on the targeted drug delivery of chemotherapeutic drugs, mostly for increasing tumor accumulation and decreasing systemic toxicity (62). Recently, an exciting area of nanomedicine has evolved, known as theranostics, based on the idea that the same drug carriers can be design as potent imaging agents (63–65). SPIONs are increasingly used for T2wMRI in oncology, including fast-evolving macrophage imaging. Macrophage-driven uptake of iron allows for the non-invasive assessment of the tissue inflammation status in cancer, diabetes, and ischemia/reperfusion injury. In the future, the same SPIONs can be loaded with an anti-inflammatory or chemotherapeutic agent to selectively deliver a therapy to the inflamed lesion.
An alternative to the use of SPION agents is the use of perfluocarbons that can be visualized for fluorine (19F) magnetic resonance spectroscopy (MRS) for cell tracking of inflammatory cells (66). Some limitations to the 19F-MRS application include low sensitivity to the target (usually, in the millimolars range). Another alternative might arise from the use of hyperpolarized 13C-arginine by 13C-MRS (67), since upregulated expression of arginase has been found in M2-like macrophages. However, the hyperpolarized 13C-MRS approach is technically and clinically challenging and available only at the very limited number of academic hospitals. For PET, the uptake of radioactive glucose analog (18F-fluoro-deoxyglucose) by inflamed tissue is well known, but unfortunately, is rather non-specific since the tumor cells also have elevated glucose uptake (68, 69). Most recent studies try to use a specific 18F-tracer for the translocator protein to image activated microglial cells and, possibly, TAMs in inflamed gliomas (70). But, as of today, the SPION-based T2-MRI approach appears to be clinically the most feasible path to image TAMs and to follow the response to anti-inflammatory treatment non-invasively. In the future, the combined PET/MRI might be the best available option for human imaging, since the first multimodality scanners have recently became available (71). Ideally, the future imaging studies should be designed to non-invasively discriminate the protumor M2 versus antitumor M1 macrophages, since this phenotyping might play a crucial role in assessing tumor response to novel checkpoint inhibitors and other immunotherapies (72). A non-invasive TAM imaging will enable to characterize the inflamed tumor microenvironment, selectively deliver novel anti-inflammatory and anticancer drugs, and monitor their efficacy non-invasively and in the real time, providing new horizons for oncological imaging well above the limitations of conventional “volumetric” criteria.
The original data provided in Figures 1 and 2 were acquired in NS group.
NS was solely responsible for literature search and composing this manuscript.
The author declares that the research was conducted in the absence of any commercial or financial relationships that could be construed as a potential conflict of interest.
The reviewer, LD, and handling editor declared their shared affiliation, and the handling editor states that the process nevertheless met the standards of a fair and objective review.
This work was supported by the NIH P30 CA046934, UL1 TR001082, R21 CA194477, and 1S10 OD023485 grants.
1. Pollard JW. Tumour-educated macrophages promote tumour progression and metastasis. Nat Rev Cancer (2004) 4(1):71–8. doi:10.1038/nrc1256
2. Bingle L, Brown NJ, Lewis CE. The role of tumour-associated macrophages in tumour progression: implications for new anticancer therapies. J Pathol (2002) 196(3):254–65. doi:10.1002/path.1027
3. Gouon-Evans V, Lin EY, Pollard JW. Requirement of macrophages and eosinophils and their cytokines/chemokines for mammary gland development. Breast Cancer Res (2002) 4(4):155–64. doi:10.1186/bcr441
4. Gordon S. Alternative activation of macrophages. Nat Rev Immunol (2003) 3(1):23–35. doi:10.1038/nri978
5. Mosser DM, Edwards JP. Exploring the full spectrum of macrophage activation. Nat Rev Immunol (2008) 8(12):958–69. doi:10.1038/nri2448
6. DeNardo DG, Johansson M, Coussens LM. Immune cells as mediators of solid tumor metastasis. Cancer Metastasis Rev (2008) 27(1):11–8. doi:10.1007/s10555-007-9100-0
7. Hanahan D, Coussens LM. Accessories to the crime: functions of cells recruited to the tumor microenvironment. Cancer Cell (2012) 21(3):309–22. doi:10.1016/j.ccr.2012.02.022
8. Bates SE. Molecular diagnostics: are we there yet? Clin Cancer Res (2012) 18(6):1514. doi:10.1158/1078-0432.CCR-11-2207
9. Blasberg R, Piwnica-Worms D. Imaging: strategies, controversies, and opportunities. Clin Cancer Res (2012) 18(3):631–7. doi:10.1158/1078-0432.CCR-11-2020
10. El-Deiry WS, Sigman CC, Kelloff GJ. Imaging and oncologic drug development. J Clin Oncol (2006) 24(20):3261–73. doi:10.1200/JCO.2006.06.5623
11. Linden HM, Dehdashti F. Novel methods and tracers for breast cancer imaging. Semin Nucl Med (2013) 43(4):324–9. doi:10.1053/j.semnuclmed.2013.02.003
12. Daldrup-Link HE, Golovko D, Ruffell B, Denardo DG, Castaneda R, Ansari C, et al. MRI of tumor-associated macrophages with clinically applicable iron oxide nanoparticles. Clin Cancer Res (2011) 17(17):5695–704. doi:10.1158/1078-0432.CCR-10-3420
13. Coyne DW. Ferumoxytol for treatment of iron deficiency anemia in patients with chronic kidney disease. Expert Opin Pharmacother (2009) 10(15):2563–8. doi:10.1517/14656560903224998
14. Muehe AM, Feng D, von Eyben R, Luna-Fineman S, Link MP, Muthig T, et al. Safety report of ferumoxytol for magnetic resonance imaging in children and young adults. Invest Radiol (2016) 51(4):221–7. doi:10.1097/RLI.0000000000000230
15. Fredrickson J, Serkova NJ, Wyatt SK, Carano RA, Pirzkall A, Rhee I, et al. Clinical translation of ferumoxytol-based vessel size imaging (VSI): feasibility in a phase I oncology clinical trial population. Magn Reson Med (2016) 77(2):814–25. doi:10.1002/mrm.26167
16. Khurana A, Nejadnik H, Gawande R, Lin G, Lee S, Messing S, et al. Intravenous ferumoxytol allows noninvasive MR imaging monitoring of macrophage migration into stem cell transplants. Radiology (2012) 264(3):803–11. doi:10.1148/radiol.12112393
17. Nayak AB, Luhar A, Hanudel M, Gales B, Hall TR, Finn JP, et al. High-resolution, whole-body vascular imaging with ferumoxytol as an alternative to gadolinium agents in a pediatric chronic kidney disease cohort. Pediatr Nephrol (2015) 30(3):515–21. doi:10.1007/s00467-014-2953-x
18. McCullough BJ, Kolokythas O, Maki JH, Green DE. Ferumoxytol in clinical practice: implications for MRI. J Magn Reson Imaging (2013) 37(6):1476–9. doi:10.1002/jmri.23879
19. Bashir MR, Bhatti L, Marin D, Nelson RC. Emerging applications for ferumoxytol as a contrast agent in MRI. J Magn Reson Imaging (2015) 41(4):884–98. doi:10.1002/jmri.24691
20. Hope MD, Hope TA, Zhu C, Faraji F, Haraldsson H, Ordovas KG, et al. Vascular imaging with ferumoxytol as a contrast agent. AJR Am J Roentgenol (2015) 205(3):W366–73. doi:10.2214/AJR.15.14534
21. Walker JP, Nosova E, Sigovan M, Rapp J, Grenon MS, Owens CD, et al. Ferumoxytol-enhanced magnetic resonance angiography is a feasible method for the clinical evaluation of lower extremity arterial disease. Ann Vasc Surg (2015) 29(1):63–8. doi:10.1016/j.avsg.2014.09.003
22. Ramanathan RK, Korn R, Raghunand N, Sachdev JC, Newbold RG, Jameson G, et al. Correlation between ferumoxytol uptake in tumor lesions by MRI and response to nanoliposomal irinotecan in patients with advanced solid tumors: a pilot study. Clin Cancer Res (2017). doi:10.1158/1078-0432.CCR-16-1990
23. Multhoff G, Molls M, Radons J. Chronic inflammation in cancer development. Front Immunol (2011) 2:98. doi:10.3389/fimmu.2011.00098
24. Lin EY, Gouon-Evans V, Nguyen AV, Pollard JW. The macrophage growth factor CSF-1 in mammary gland development and tumor progression. J Mammary Gland Biol Neoplasia (2002) 7(2):147–62. doi:10.1023/A:1020399802795
25. Pyonteck SM, Gadea BB, Wang HW, Gocheva V, Hunter KE, Tang LH, et al. Deficiency of the macrophage growth factor CSF-1 disrupts pancreatic neuroendocrine tumor development. Oncogene (2012) 31(11):1459–67. doi:10.1038/onc.2011.337
26. Pyonteck SM, Akkari L, Schuhmacher AJ, Bowman RL, Sevenich L, Quail DF, et al. CSF-1R inhibition alters macrophage polarization and blocks glioma progression. Nat Med (2013) 19(10):1264–72. doi:10.1038/nm.3337
27. Coussens LM, Werb Z. Inflammatory cells and cancer: think different! J Exp Med (2001) 193(6):F23–6. doi:10.1084/jem.193.6.F23
28. Borges VF, Schedin PJ. Pregnancy-associated breast cancer: an entity needing refinement of the definition. Cancer (2012) 118(13):3226–8. doi:10.1002/cncr.26643
29. Fritz JM, Tennis MA, Orlicky DJ, Lin H, Ju C, Redente EF, et al. Depletion of tumor-associated macrophages slows the growth of chemically induced mouse lung adenocarcinomas. Front Immunol (2014) 5:587. doi:10.3389/fimmu.2014.00587
30. Sica A, Rubino L, Mancino A, Larghi P, Porta C, Rimoldi M, et al. Targeting tumour-associated macrophages. Expert Opin Ther Targets (2007) 11(9):1219–29. doi:10.1517/14728222.11.9.1219
31. Jinushi M, Chiba S, Yoshiyama H, Masutomi K, Kinoshita I, Dosaka-Akita H, et al. Tumor-associated macrophages regulate tumorigenicity and anticancer drug responses of cancer stem/initiating cells. Proc Natl Acad Sci U S A (2011) 108(30):12425–30. doi:10.1073/pnas.1106645108
32. Allavena P, Sica A, Solinas G, Porta C, Mantovani A. The inflammatory micro-environment in tumor progression: the role of tumor-associated macrophages. Crit Rev Oncol Hematol (2008) 66(1):1–9. doi:10.1016/j.critrevonc.2007.07.004
33. Siveen KS, Kuttan G. Role of macrophages in tumour progression. Immunol Lett (2009) 123(2):97–102. doi:10.1016/j.imlet.2009.02.011
34. Gonda TA, Tu S, Wang TC. Chronic inflammation, the tumor microenvironment and carcinogenesis. Cell Cycle (2009) 8(13):2005–13. doi:10.4161/cc.8.13.8985
35. Schaue D, Micewicz ED, Ratikan JA, Xie MW, Cheng G, McBride WH. Radiation and inflammation. Semin Radiat Oncol (2015) 25(1):4–10. doi:10.1016/j.semradonc.2014.07.007
36. Nguyen DH, Oketch-Rabah HA, Illa-Bochaca I, Geyer FC, Reis-Filho JS, Mao JH, et al. Radiation acts on the microenvironment to affect breast carcinogenesis by distinct mechanisms that decrease cancer latency and affect tumor type. Cancer Cell (2011) 19(5):640–51. doi:10.1016/j.ccr.2011.03.011
37. Bower JE, Ganz PA, Tao ML, Hu W, Belin TR, Sepah S, et al. Inflammatory biomarkers and fatigue during radiation therapy for breast and prostate cancer. Clin Cancer Res (2009) 15(17):5534–40. doi:10.1158/1078-0432.CCR-08-2584
38. Russell JS, Brown JM. The irradiated tumor microenvironment: role of tumor-associated macrophages in vascular recovery. Front Physiol (2013) 4:157. doi:10.3389/fphys.2013.00157
39. Mukhtar RA, Nseyo O, Campbell MJ, Esserman LJ. Tumor-associated macrophages in breast cancer as potential biomarkers for new treatments and diagnostics. Expert Rev Mol Diagn (2011) 11(1):91–100. doi:10.1586/erm.10.97
40. Brown JM, Recht L, Strober S. The promise of targeting macrophages in cancer therapy. Clin Cancer Res (2017). doi:10.1158/1078-0432.CCR-16-3122
41. Barbay V, Houssari M, Mekki M, Banquet S, Edwards-Levy F, Henry JP, et al. Role of M2-like macrophage recruitment during angiogenic growth factor therapy. Angiogenesis (2015) 18(2):191–200. doi:10.1007/s10456-014-9456-z
42. Han CZ, Juncadella IJ, Kinchen JM, Buckley MW, Klibanov AL, Dryden K, et al. Macrophages redirect phagocytosis by non-professional phagocytes and influence inflammation. Nature (2016) 539(7630):570–4. doi:10.1038/nature20141
43. Raynal I, Prigent P, Peyramaure S, Najid A, Rebuzzi C, Corot C. Macrophage endocytosis of superparamagnetic iron oxide nanoparticles: mechanisms and comparison of ferumoxides and ferumoxtran-10. Invest Radiol (2004) 39(1):56–63. doi:10.1097/01.rli.0000101027.57021.28
44. Ganz T. Macrophages and systemic iron homeostasis. J Innate Immun (2012) 4(5–6):446–53. doi:10.1159/000336423
45. Ganz T. Macrophages and iron metabolism. Microbiol Spectr (2016) 4(5). doi:10.1128/microbiolspec.MCHD-0037-2016
46. Serkova NJ, Garg K, Bradshaw-Pierce EL. Oncologic imaging end-points for the assessment of therapy response. Recent Pat Anticancer Drug Discov (2009) 4(1):36–53. doi:10.2174/157489209787002434
47. O’Connor JP, Jackson A, Parker GJ, Roberts C, Jayson GC. Dynamic contrast-enhanced MRI in clinical trials of antivascular therapies. Nat Rev Clin Oncol (2012) 9(3):167–77. doi:10.1038/nrclinonc.2012.2
48. Kircher MF, Willmann JK. Molecular body imaging: MR imaging, CT, and US. Part II. Applications. Radiology (2012) 264(2):349–68. doi:10.1148/radiol.12111703
49. Kircher MF, Willmann JK. Molecular body imaging: MR imaging, CT, and US. Part I. Principles. Radiology (2012) 263(3):633–43. doi:10.1148/radiol.12102394
50. Wahl RL, Jacene H, Kasamon Y, Lodge MA. From RECIST to PERCIST: evolving considerations for PET response criteria in solid tumors. J Nucl Med (2009) 50(Suppl 1):122S–50S. doi:10.2967/jnumed.108.057307
51. Zhou Z, Lu ZR. Gadolinium-based contrast agents for magnetic resonance cancer imaging. Wiley Interdiscip Rev Nanomed Nanobiotechnol (2013) 5(1):1–18. doi:10.1002/wnan.1198
52. Anchordoquy TJ, Barenholz Y, Boraschi D, Chorny M, Decuzzi P, Dobrovolskaia MA, et al. Mechanisms and barriers in cancer nanomedicine: addressing challenges, looking for solutions. ACS Nano (2017) 11(1):12–8. doi:10.1021/acsnano.6b08244
53. Hasebroock KM, Serkova NJ. Toxicity of MRI and CT contrast agents. Expert Opin Drug Metab Toxicol (2009) 5(4):403–16. doi:10.1517/17425250902873796
54. Kanal E, Tweedle MF. Residual or retained gadolinium: practical implications for radiologists and our patients. Radiology (2015) 275(3):630–4. doi:10.1148/radiol.2015150805
55. Cantow K, Pohlmann A, Flemming B, Ferrara F, Waiczies S, Grosenick D, et al. Acute effects of ferumoxytol on regulation of renal hemodynamics and oxygenation. Sci Rep (2016) 6:29965. doi:10.1038/srep29965
56. Onishi H, Murakami T, Kim T, Hori M, Hirohashi S, Matsuki M, et al. Safety of ferucarbotran in MR imaging of the liver: a pre- and postexamination questionnaire-based multicenter investigation. J Magn Reson Imaging (2009) 29(1):106–11. doi:10.1002/jmri.21608
57. Mouli SK, Zhao LC, Omary RA, Thaxton CS. Lymphotropic nanoparticle enhanced MRI for the staging of genitourinary tumors. Nat Rev Urol (2010) 7(2):84–93. doi:10.1038/nrurol.2009.254
58. Oghabian MA, Gharehaghaji N, Amirmohseni S, Khoei S, Guiti M. Detection sensitivity of lymph nodes of various sizes using USPIO nanoparticles in magnetic resonance imaging. Nanomedicine (2010) 6(3):496–9. doi:10.1016/j.nano.2009.11.005
59. Serkova NJ, Renner B, Larsen BA, Stoldt CR, Hasebroock KM, Bradshaw-Pierce EL, et al. Renal inflammation: targeted iron oxide nanoparticles for molecular MR imaging in mice. Radiology (2010) 255(2):517–26. doi:10.1148/radiol.09091134
60. Harman A, Chang KJ, Dupuy D, Rintels P. The long-lasting effect of ferumoxytol on abdominal magnetic resonance imaging. J Comput Assist Tomogr (2014) 38(4):571–3. doi:10.1097/RCT.0000000000000086
61. Grodzinski P, Farrell D. Future opportunities in cancer nanotechnology – NCI strategic workshop report. Cancer Res (2014) 74(5):1307–10. doi:10.1158/0008-5472.CAN-13-2787
62. Monk BJ, Brady MF, Aghajanian C, Lankes HA, Rizack T, Leach J, et al. A phase 2, randomized, double-blind, placebo controlled study of chemo-immunotherapy combination using motolimod with pegylated liposomal doxorubicin in recurrent or persistent ovarian cancer: a Gynecologic Oncology Group partners study. Ann Oncol (2017) 28(5):996–1004. doi:10.1093/annonc/mdx049
63. Lee H, Shields AF, Siegel BA, Miller KD, Krop I, Ma CX, et al. 64Cu-MM-302 positron emission tomography quantifies variability of enhanced permeability and retention of nanoparticles in relation to treatment response in patients with metastatic breast cancer. Clin Cancer Res (2017). doi:10.1158/1078-0432.CCR-16-3193
64. Fan CH, Ting CY, Lin HJ, Wang CH, Liu HL, Yen TC, et al. SPIO-conjugated, doxorubicin-loaded microbubbles for concurrent MRI and focused-ultrasound enhanced brain-tumor drug delivery. Biomaterials (2013) 34(14):3706–15. doi:10.1016/j.biomaterials.2013.01.099
65. Hadjipanayis CG, Machaidze R, Kaluzova M, Wang L, Schuette AJ, Chen H, et al. EGFRvIII antibody-conjugated iron oxide nanoparticles for magnetic resonance imaging-guided convection-enhanced delivery and targeted therapy of glioblastoma. Cancer Res (2010) 70(15):6303–12. doi:10.1158/0008-5472.CAN-10-1022
66. Waiczies S, Lepore S, Sydow K, Drechsler S, Ku MC, Martin C, et al. Anchoring dipalmitoyl phosphoethanolamine to nanoparticles boosts cellular uptake and fluorine-19 magnetic resonance signal. Sci Rep (2015) 5:8427. doi:10.1038/srep08427
67. Najac C, Chaumeil MM, Kohanbash G, Guglielmetti C, Gordon JW, Okada H, et al. Detection of inflammatory cell function using (13)C magnetic resonance spectroscopy of hyperpolarized [6-(13)C]-arginine. Sci Rep (2016) 6:31397. doi:10.1038/srep31397
68. Shroff GS, Carter BW, Viswanathan C, Benveniste MF, Wu CC, Marom EM, et al. Challenges in interpretation of staging PET/CT in thoracic malignancies. Curr Probl Diagn Radiol (2016) S0363-0188(16):30107–4. doi:10.1067/j.cpradiol.2016.11.012
69. Lammers T, Aime S, Hennink WE, Storm G, Kiessling F. Theranostic nanomedicine. Acc Chem Res (2011) 44(10):1029–38. doi:10.1021/ar200019c
70. Zinnhardt B, Pigeon H, Theze B, Viel T, Wachsmuth L, Fricke IB, et al. Combined PET imaging of the inflammatory tumor microenvironment identifies margins of unique radiotracer uptake. Cancer Res (2017) 77(8):1831–41. doi:10.1158/0008-5472.CAN-16-2628
71. Rolle AM, Hasenberg M, Thornton CR, Solouk-Saran D, Mann L, Weski J, et al. ImmunoPET/MR imaging allows specific detection of Aspergillus fumigatus lung infection in vivo. Proc Natl Acad Sci U S A (2016) 113(8):E1026–33. doi:10.1073/pnas.1518836113
Keywords: magnetic resonance imaging, iron oxide nanoparticles, tumor-associated macrophages, inflammation, cancer
Citation: Serkova NJ (2017) Nanoparticle-Based Magnetic Resonance Imaging on Tumor-Associated Macrophages and Inflammation. Front. Immunol. 8:590. doi: 10.3389/fimmu.2017.00590
Received: 11 April 2017; Accepted: 04 May 2017;
Published: 22 May 2017
Edited by:
Diana Boraschi, Consiglio Nazionale Delle Ricerche (CNR), ItalyReviewed by:
Luciana D’Apice, Consiglio Nazionale Delle Ricerche (CNR), ItalyCopyright: © 2017 Serkova. This is an open-access article distributed under the terms of the Creative Commons Attribution License (CC BY). The use, distribution or reproduction in other forums is permitted, provided the original author(s) or licensor are credited and that the original publication in this journal is cited, in accordance with accepted academic practice. No use, distribution or reproduction is permitted which does not comply with these terms.
*Correspondence: Natalie J. Serkova, natalie.serkova@ucdenver.edu
Disclaimer: All claims expressed in this article are solely those of the authors and do not necessarily represent those of their affiliated organizations, or those of the publisher, the editors and the reviewers. Any product that may be evaluated in this article or claim that may be made by its manufacturer is not guaranteed or endorsed by the publisher.
Research integrity at Frontiers
Learn more about the work of our research integrity team to safeguard the quality of each article we publish.