- 1Crop Science and Production Systems, NIAB East Malling, East Malling, United Kingdom
- 2Fruit Breeding Group, Department of Plant Breeding, Centro de Edafología y Biología Aplicada del Segura - Consejo Superior de Investigaciones Científicas (CEBAS-CSIC), Murcia, Spain
- 3School of Agriculture, Policy and Development, University of Reading, Reading, United Kingdom
Dormancy enables apple trees (Malus × domestica Borkh) to survive unfavorable weather conditions. The accumulation of cold temperatures during winter is required to release dormancy, whilst heat accumulation in spring promotes bud break and blooming. Chilling and heat requirements are used to anticipate cultivars’ suitability to local agroclimatic conditions. This review summarizes recent advances on the physiological and genetic mechanisms regulating dormancy in apple trees; and presents a compilation of available chilling and heat requirements for apple cultivars. Information shows a wide range of chilling requirements in existing cultivars. However, results reported for the same cultivar are highly variable between locations and methods used to estimate chilling; raising concerns on the suitability of using chill requirements to inform planting decisions. In the context of climate change, it is essential to ensure current knowledge on the physiological and genetic mechanisms regulating bud break guides the development of improved models that can generate better estimates of chilling and heat requirements in apple.
1 Introduction
The cultivated apple, Malus × domestica Borkh. (Korban and Skirvin, 1984), belongs to the Rosaceae family, genus Malus, and it is the third most important fruit crop worldwide in terms of production (FAOSTAT, 2021). Annual average production reached 93.1 million metric tons in 2021, with China, the United States of America, Turkey, Poland, and Iran being the top producing countries (FAOSTAT, 2021). Around 7500 apple cultivars have been documented (Watkins, 1984) but only a few cultivars dominate the market. Malus × domestica Borkh. is the result of a long evolutionary process; its wild ancestor and the main contributor to the domesticated apple gene pool is Malus sieversii, which originated in central Asia (Cornille et al., 2019).
Apple fruit buds are borne as mixed buds, containing both leaves and flowers. For buds to start floral initiation, a critical number of nodes in a shoot must be reached before dormancy; this number varies from 16 to 20, depending on the variety (Jackson, 2003). Different floral components are formed inside the buds during autumn and winter, but final flower development occurs between bud break and anthesis during the following spring; with the full process being completed over two seasons (Pratt, 1988). As flower initiation for the following season occurs when current fruits are developing, heavy cropping in one year can reduce the proportion of flowering buds the next year, known as biennial cropping (Jonkers, 1979). Most apple cultivars are self-incompatible and therefore successful fruit set requires cross-pollination with other genetically compatible varieties and depends on insects as pollen vectors (Ramírez and Davenport, 2013). Commercial apple trees are compound trees, formed of a scion and a rootstock. The scion (grafted part) is taken from a mature tree and grafted onto a rootstock with particular characteristics. Dwarfing rootstocks combined with specific orchard management practices (branch and root pruning) are used to control tree size, which then facilitates higher planting densities and thus more productive orchards per unit area (Webster, 1995). Like most woody perennial species that evolved in cold climates, apple trees spend the winter months in a dormant state that allows them to survive unfavourable weather conditions (Saure, 1985; Faust et al., 1997; Campoy et al., 2011b). Winter dormancy also determines the timing and quality of flowering, ultimately influencing final fruit production (Saure, 1985; Faust et al., 1997; Rohde and Bhalerao, 2007).
The cultivated apple is grown worldwide, from warm regions such as Kenya (Griesbach, 2007) and South Africa (Cook, 2010), to cold areas such as Finland (Kaukoranta et al., 2010) and the western Indian Himalaya region (Kumar et al., 2008). However, successful production is variable, particularly if cultivars are grown in areas beyond their climatic requirements. Apple trees require cold temperatures during the winter as insufficient chilling can reduce bud break and flowering (Petri and Leite, 2004). Chilling requirements are highly variable between cultivars (Hauagge and Cummins, 1991; Parkes et al., 2020; Delgado et al., 2021a), and fruit production will be compromised if cultivars with high-chill requirements are grown in regions with mild and/or short winters. Future winter chilling is predicted to decline in all major fruit tree growing regions as a consequence of climate change (Luedeling et al., 2011; Darbyshire et al., 2016a). The application of dormancy breaking agents such us hydrogen cyanamide is often used to induce bud break and mitigate de adverse effects of insufficient chilling accumulation (Griesbach, 2007); however, it has been banned in many countries due to phytotoxicity concerns (Siller-Cepeda et al., 2019). Warmer temperatures in spring are also of concern as they might induce earlier bud break; advances in blooming dates in apple have already been observed in many countries (Legave et al., 2013; Drepper et al., 2020). If buds open too soon there is an increased risk of frost damage (Figure 1), which can cause significant yield losses (DEFRA et al., 2017). Warmer spring temperatures might also cause asynchrony between flowering and pollinator emergence, leading to negative impacts on final fruit production (Korösi et al., 2018).
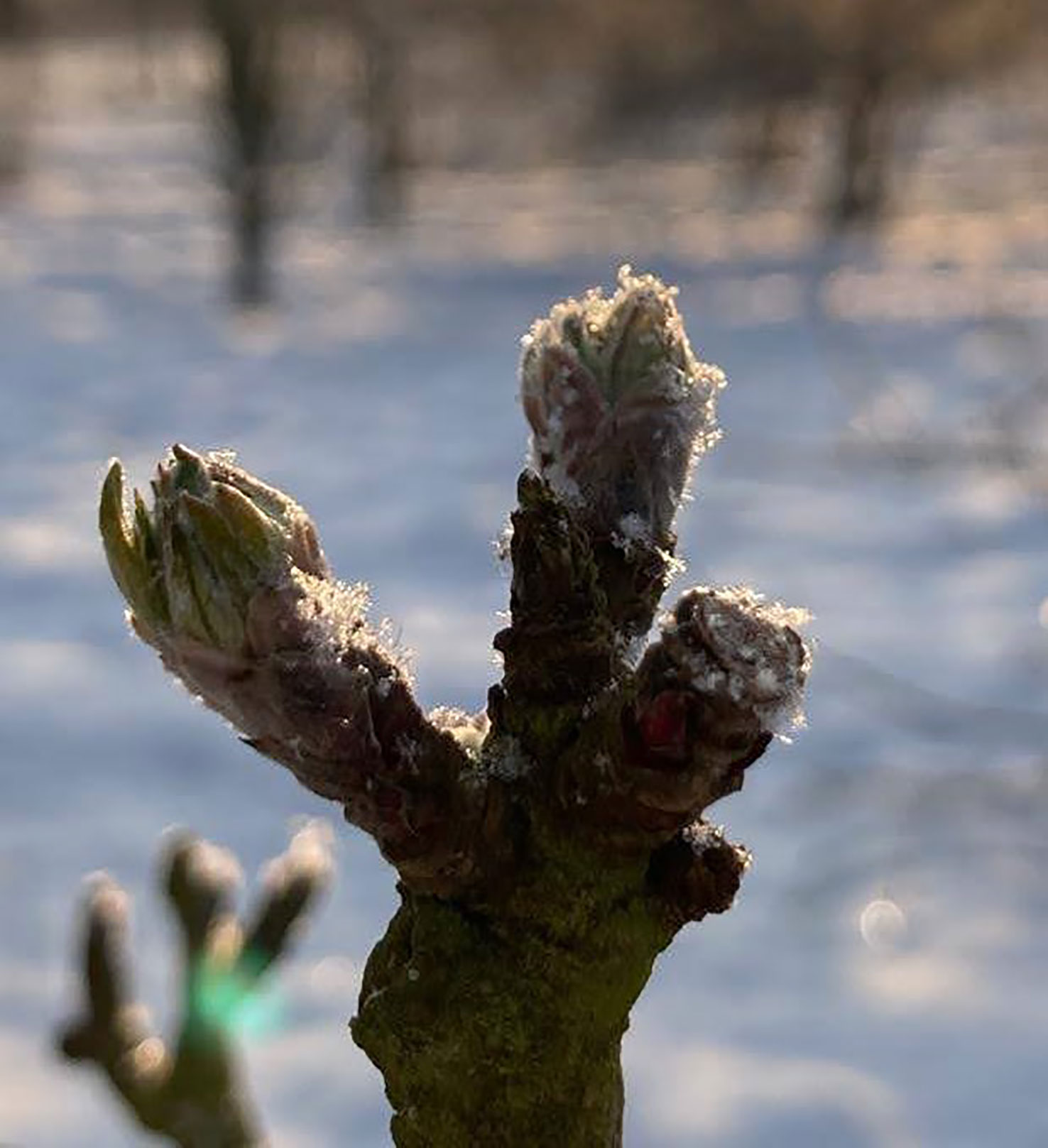
Figure 1 Open flower buds of the early apple cultivar “Anna” after a snowfall in February 2021 (East Malling, UK).
Unless drastic cuts in global greenhouse gas emissions are efficiently implemented, mean global temperatures are predicted to reach 1.5°C above pre-industrial levels by 2040 (IPCC, 2022). The dormancy cycle in apple trees is regulated by temperature (Heide and Prestrud, 2005), making the domesticated apple especially vulnerable to changes in the climate. With both winter and summer temperatures predicted to increase, severe consequences for temperate fruit crops are anticipated (Else and Atkinson, 2010; Luedeling, 2012; Atkinson et al., 2013). It is essential to understand how different apple cultivars are likely to respond to changes in climatic conditions so that adaptation and mitigation measures can be readily implemented to reduce the impact on food production. With the objective of identifying current knowledge gaps and generate information to improve cultivar selection; we review the latest findings regarding dormancy regulation in apple and present a compilation of published chilling and heat requirements for a range of apple cultivars (Figure 2).
2 Dormancy definition and environmental regulation
Dormancy is part of the annual cycle of perennial woody plants growing in temperate regions, and is defined as the temporary suspension of visible growth of any plant structure containing meristems (Lang et al., 1987). Dormancy has been artificially divided into three phases which are often referred to by different names (Doorenbos, 1953; Saure, 1985; Lang et al., 1987). Although these phases were described earlier by other authors (Doorenbos, 1953; Saure, 1985), Lang’s terminology (Lang et al., 1987) is the most widely adopted and will be used throughout this review. Lang et al. (1987) named the three dormancy phases as (i) paradormancy, (ii) endodormancy, and (iii) ecodormancy; and proposed that these are differentiated mainly by two parameters, (i) the event responsible for changing the state of dormancy (i.e. temperature) and (ii) the tissue where this change is perceived (i.e. the bud).
During paradormancy, inhibition of growth is regulated by intrinsic physiological factors located outside the organ undergoing dormancy, and is mostly related to the apical dominance by which the terminal bud inhibits growth of axillary buds on growing shoots (Champagnat, 1989). Paradormancy influences trees structure (Champagnat, 1989) and as discussed in more detail in section 4.1, apical dominance is strongly regulated by phytohormones.
Between the end of summer and the beginning of autumn, shorter and colder days trigger growth cessation, bud set and induce the transition towards endodormancy. Photoperiod and temperature are the two environmental cues regulating this change, but their relative importance varies between species (Garner and Allard, 1923). Short photoperiods have a clear dormancy-inducing role for many species (Garner and Allard, 1923; Olsen et al., 1995; Rohde et al., 2011); but apple does not react to this environmental cue and it is low temperature that induces growth cessation and endodormancy, regardless of short- or long-day conditions (Garner and Allard, 1923; Heide and Prestrud, 2005). Other species from the Rosaceae family such as pear (Pyrus communis) also do not react to photoperiod (Heide and Prestrud, 2005).
As winter progresses, endodormancy is overcome by extended periods of chilling (accumulation of chilling), which are required to remove the physiological blocks that prevent growth. In apple, it is generally assumed that the same temperature range is needed for both dormancy induction and release (Heide and Prestrud, 2005). Whilst 7°C was formerly adopted as the ideal temperature for chilling accumulation in temperate fruit trees (Samish, 1954; Vegis, 1964), it was later reported that not all temperatures contributed equally to chilling (Richardson et al., 1974; Thompson et al., 1975; Shaltout and Unrath, 1983) (see section 6). The amount of chilling needed to overcome endodormancy is known as chilling requirement (CR); CR in apple is highly variable between cultivars (Hauagge and Cummins, 1991; Parkes et al., 2020; Delgado et al., 2021a). High temperatures during dormancy induction appear to increase chilling requirements and delay bud break in apple (Cook and Jacobs, 2000); and several studies have highlighted a negative impact of high temperature interruptions during endodormancy as they can reduce bud burst (Richardson et al., 1974; Thompson et al., 1975; Anzanello et al., 2014). However, a promoting effect on bud break of high-low temperature cycles compared to moderate and low temperatures has also been observed in peach (Erez and Couvillon, 1987; Campoy et al., 2011a), and this mechanism is included in the mathematical structure of the Dynamic model (Fishman et al., 1987a; Fishman et al., 1987b) (see section 6).
After CR satisfaction, trees remain ecodormant until environmental conditions are favorable for growth, this is key not only for plant survival during winter months, but also as a mechanism to avoid the resumption of growth when environmental conditions fluctuate between favourable and unfavourable (Rohde and Bhalerao, 2007). Warmer temperatures (accumulation of heat) are needed to overcome ecodormancy and promote bud development and blooming. The minimum amount of heat needed for flowering is often referred to as the Heat Requirement (HR). The release from endodormancy and ecodormancy will determine time of bud break and flowering and so are the focus of this review.
The importance of temperature as a dormancy regulating factor is evident, however, the relationship between temperature and bud break has not been clearly defined due to the complex interaction between chilling and heat accumulation; whilst it was initially assumed that these processes occurred sequentially, a growing number of studies are indicating there is likely to be an overlap (Pope et al., 2014; Guo et al., 2015; Darbyshire et al., 2016b). A range of inter-linked factors are involved in regulating the dormancy process (Figure 3), including environmental conditions and physiological changes, but important gaps in our understanding of the cycle still exist.
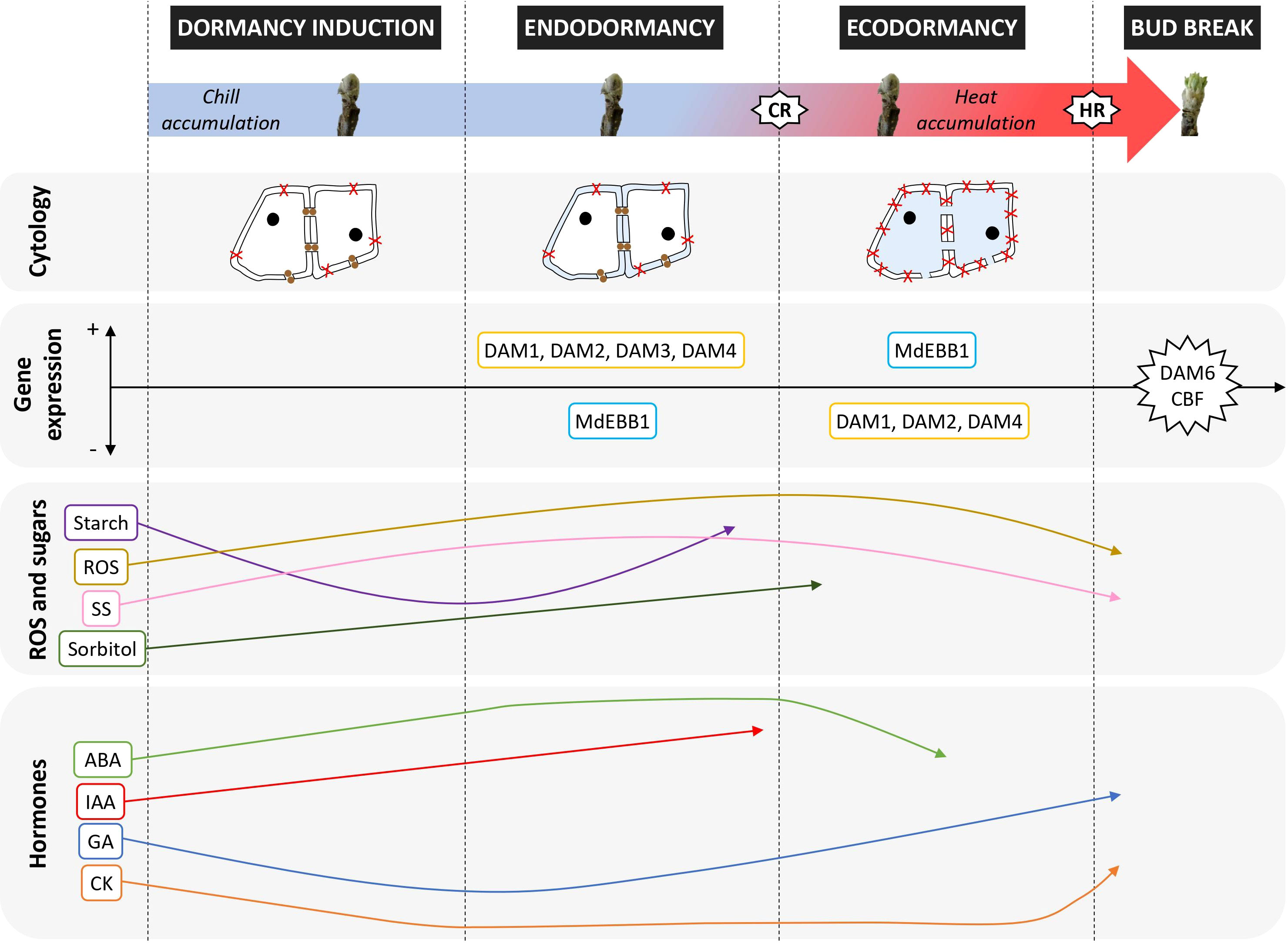
Figure 3 Cytological, physiological and genetic changes observed during dormancy in apple studies (references throughout this review). CR, Chill requirements satisfied; HR, Heat requirements satisfied; ROS, reactive oxygen species; SS, Soluble sugars; ABA, Abscisic Acid; IAA, auxin; GA, Gibberellins; CK, cytokinin. In the cytology section: blue coloured areas indicate changes in water status in the cells; red crosses (X) are polar lipids; openings between cells are plasmodesmata and brown circular dots represent callose deposits (these changes in cell-to-cell communication have not been reported in apple). Genes above or below the line represent high or low expression levels except in DAM6 and CBF; overexpression of these genes in transgenic plants alter bud break patterns. In ROS, sugars and hormones, the position of a compound within a dormancy phase does not indicate relative abundance; arrows only represent changes in concentration within each sugar or hormone type.
3 Approaches to study dormancy
One of the main difficulties facing researchers studying dormancy is that there are no visible changes in the buds during the transition from endo to ecodormancy, making it difficult to pinpoint when the transition occurs. As recently reviewed by Fadón and Rodrigo (2018), a range of methodologies have been used over the years to explore dormancy progression and to determine the endodormancy release date, but a lack of standardization has hindered the development of clear protocols and comparisons between studies (Dennis, 2003).
The most common approach to determine endodormancy break and subsequent chilling requirements is to investigate the dormancy status of the plant. This is done by exposing plant material (usually excised shoots, potted trees, or single-node cuttings) to chilling conditions for different durations of time before transferring them to warmer temperatures to induce bud break (Ruiz et al., 2007; Campoy et al., 2012; Jones et al., 2013; Parkes et al., 2020). Chilling requirements are considered fulfilled when it takes less than a fixed number of days to reach a predefined percentage of bud break (10 to 50%). Although now widely used, this approach is rather arbitrary and different values for the number of days, the percentage threshold and the environmental conditions during the forcing period can be found in the literature (Hauagge and Cummins, 1991; Naor et al., 2003; Ruiz et al., 2007; Campoy et al., 2012; Jones et al., 2013; Parkes et al., 2020). The quantification of cultivar-specific CR using excised shoots can result in widely different estimates depending on aspects such as the type of buds (Campoy et al., 2011a), rootstock (Webster, 1995), altitude (Alburquerque et al., 2008) or experimental design (Dennis, 2003).
Another approach to determine the date of endodormancy release is the Tabuenca test (Tabuenca, 1964), which considers trees to have entered ecodormancy when a significant increase in fresh weight is observed in buds placed under forcing conditions for a week, compared to buds collected from the field. This method has some important limitations as external factors, such as water availability, can impact bud fresh weight (Bartolini et al., 2020) and mask the effect of dormancy progression. Some studies with apple have suggested that the approach may be especially misleading in areas with mild winters (Malagi et al., 2015). Once the date of endodormancy release has been determined, mathematical models are used to quantify chilling and heat accumulated according to the temperatures recorded before and after endodormancy release, respectively (see section 6).
To better understand the physiological mechanisms regulating dormancy, analytical or histochemical techniques are usually combined with one of the two methods described above to establish chilling requirements (Fadón and Rodrigo, 2018). This is important as it links any physiological observations to the dormancy status of the plant. Analytical techniques aim to identify and quantify compounds linked to dormancy progression, such as hormones and carbohydrates. These studies are usually based on quantifying the concentration of these substances in floral buds (Wen et al., 2016; Fernandez et al., 2019), or on application of these compounds to determine their role (Guak and Fuchigami, 2001). They are destructive and time-consuming methods, which have the intrinsic limitation that a singular bud or plant cannot be followed throughout the dormancy cycle, as samples are destroyed after analyses. These limitations are also encountered when using histochemical techniques, which allow visualization of internal parts of the plant at different dormancy stages, such as bud cells or other structures. Microscopic observations, for example, have allowed identification of different changes at the cellular level during dormancy (Rinne et al., 2001; Fadón et al., 2018).
Molecular approaches have also been used in the study of dormancy, including the use of transgenic individuals to determine the role of specific genes (Wisniewski et al., 2015; Wu et al., 2017), or transcriptomic analyses to understand changes in gene expression during dormancy progression (Porto et al., 2015; Porto et al., 2016). These methodologies have provided key information on the molecular mechanisms regulating dormancy, and as they become more easily accessible, a greater contribution to our understanding of the dormancy cycle is anticipated.
4 Physiological processes regulating dormancy
Although no apparent external changes occur in the buds during endodormancy, many physiological processes take place, including changes in the balance of hormones (Michalczuk, 2005; Cooke et al., 2012), in the composition of the cell membrane (Rinne et al., 2001; Horvath et al., 2003), and in the concentration of different carbohydrates (Ito et al., 2012; Fernandez et al., 2019). Recent reviews have summarized key physiological processes taking place during dormancy in woody perennials (Liu and Sherif, 2019; Fadón, et al., 2020a; Yang et al., 2021). Here, we highlight studies focusing on apple and pear in which dormancy is regulated only by temperature (Garner and Allard, 1923; Heide and Prestrud, 2005). Examples from other temperate fruit trees are cited where no apple or pear studies were available.
4.1 Hormonal regulation
Plant hormones regulate many growth and developmental processes, and the theory that dormancy is induced, terminated, and regulated by changes in the balance of growth inhibitors and promoters, known as the Linear hormonal hypothesis, was one of the first attempts to try to explain the dormancy process in seeds (Amen, 1968). A range of hormones are implicated in the dormancy cycle, including gibberellins (GA), auxin (indole-3-acetic acid, IAA), cytokinins (CK); Abscisic Acid (ABA) and ethylene (ET). A complex crosstalk network between these hormones regulates growth cessation, dormancy induction, endodormancy release, and growth initiation (Liu and Sherif, 2019).
4.1.1 Gibberellins
Gibberellins promote many plant growth processes including shoot elongation, seed germination and floral induction (Lewak, 2011; Hedden and Sponsel, 2015).
In apple, GA have been linked to biennial bearing, as high concentration of GAs in seeds can inhibit flower production (Wilkie et al., 2008; Mutasa-Gottgens and Hedden, 2009). Applications of GA can stimulate vegetative growth and reduce and delay flower bud production in apple; but the magnitude of this effect depends on the GA used and the timing of the application (Tromp, 1982; Bertelsen et al., 2002; Mostafa and Saleh, 2006; Zhang et al., 2016).
Changes in the concentration of different endogenous GAs have been reported in the literature throughout dormancy; in general, GA concentrations decline during dormancy induction, and increase as dormancy progresses until bud break (Cooke et al., 2012; Seif El-Yazal et al., 2014; Liu and Sherif, 2019; Sapkota et al., 2021a). A rapid increase of GA20 content in apple buds before bud break was linked to flowering time, as the increase occurred earlier in the early-blooming cultivar “Cripps Pink” compared to the late-blooming “Honeycrisp” (Sapkota et al., 2021a). Studies have shown that gibberellins promote bud break by degrading DELLA proteins, which work to repress GA responses such as flower development and germination in Arabidopsis (Fleet and Sun, 2005). In apple and pear, RGL genes which encode for DELLA proteins are upregulated in dormant buds and downregulated as buds enter ecodormancy (Foster et al., 2007; Bai et al., 2013; Wisniewski et al., 2015). In a study with transgenic apple trees showing reduced growth and delayed bud break, higher expression of RGL genes was observed in transformed trees compared to controls (Wisniewski et al., 2015). Although the study did not report expression data of GA-pathway genes, changes in RGL expression suggest a link between GA biosynthesis and catabolism, and dormancy break (Wisniewski et al., 2015).
Studies with other species have highlighted the role of GAs in different processes linked to dormancy break, including the restoration of plasmodesmata connectivity after endodormancy release in poplar (Rinne et al., 2011), however, this process has not been studied in apple or pear.
4.1.2 Abscisic acid
ABA regulates cell division and seed germination, and plays a key role in plants’ responses to environmental stresses such as drought or cold (Finkelstein, 2013). ABA concentrations fluctuate during dormancy in an opposite pattern to GA, increasing during growth cessation, peaking in dormant buds and decreasing with the transition to ecodormancy (Rinne et al., 1994; Tamura et al., 2002; Wen et al., 2016; Li et al., 2018a; Sapkota et al., 2021a).
Genetic studies investigating the link between ABA metabolism and dormancy have shown differential gene expression in ABA biosynthesis and catabolism pathways during dormancy progression (Bai et al., 2013; Liu and Sherif, 2019; Sapkota et al., 2021a). In pear, enzymes involved in ABA biosynthesis were upregulated at dormancy induction whilst expression of genes linked to ABA catabolism increased during endodormancy release (Bai et al., 2013; Li et al., 2018a). In particular, PpCYP707A-3 expression was linked to increased cold accumulation and reduced ABA content in the buds (Li et al., 2018a). A link between ABA and chilling accumulation was also observed by Tamura et al. (2002), as ABA injections inhibited bud break in Japanese pear shoots exposed to 200-600 chill units (CU), but not in shoots accumulating more than 800 CU. In apple, different ABA concentrations during endodormancy have also been linked to blooming times, as higher expression of ABA-biosynthesis genes was observed in the late-blooming cultivar “Honeycrisp”, compared to the early-flowering “Cripps Pink” (Sapkota et al., 2021a).
Foliar ABA sprays applied at the end of the summer reduced shoot elongation, induced growth cessation, and enhanced dormancy development in “Fuji” apple nursery plants (Guak and Fuchigami, 2001); and the transition from para to endodormancy was also accelerated in “Suli” pear (Pyrus pyrifolia) excised shoots incubated with their bases in an ABA solution (Li et al., 2018a).
Studies with other woody perennials have provided key information on the ABA-regulated mechanisms in relation to dormancy. Tylewicz et al. (2018) observed that short-days induced growth cessation equally in ABA-insensitive transgenic hybrid aspen and in the wild type, however, only transgenic lines reinitiated growth when transferred to long days. The frequency of closed plasmodesmata after 10 weeks of short-days in transgenic plants was close to 0%, whilst it had reached 83.6% in the wild type, indicating a key role of ABA mediating plasmodesmata closure as a response to short photoperiods (Tylewicz et al., 2018). Studies investigating this mechanism in species where dormancy is regulated by temperature, such as apple and pear have not been found.
4.1.3 Auxin (indole-3-acetic acid, IAA) and cytokinins
IAA and CK have been widely studied in relation to paradormancy as the balance of these hormones regulates apical dominance and branching (Cline, 1991; Cline, 2000; Leyser, 2003; Tan et al., 2019). IAA is involved in growth suppression of lateral buds (Leyser, 2003) whilst CK promote axillary bud growth (Zürcher and Müller, 2016).
Endogenous concentrations of IAA in apple buds follow a similar pattern to GAs, being low during dormancy induction and increasing at endodormancy break (Seif El-Yazal et al., 2014). Proteomic studies in pear have also shown an increase in expression in proteins linked with auxin activity just before endodormancy break (Takemura et al., 2015). Cytokinin concentration remains low during endo and ecodormancy and increase sharply before bud break (Cutting et al., 1991; Cook et al., 2001; Sapkota et al., 2021a). Expression analyses in apple have shown differential expression of several genes encoding for cytokinin biosynthesis and degradation enzymes during axillary bud break (Tan et al., 2018) and in apple cultivars presenting strong acrotony (distal branching), higher cytokinin content in distal portions of shoots has been linked to earlier bud break (Cook et al., 2001). A study comparing and early and a late-flowering apple cultivar suggested a link between the increase in CK levels and flowering time, as it occurred earlier in the early-bloom cultivar (Sapkota et al., 2021a).
Exogenous CK promoted axillary bud growth in “Fuji” apple seedlings in a similar way to decapitation of the apical bud (Li et al., 2018b). Cytokinin treatments also up-regulated expression of auxin transport genes and genes involved in axillary meristem activity, cell elongation and shoot formation; highlighting the close link between IAA and CK (Li et al., 2018b).
4.1.4 Ethylene
Known as the ripening hormone, ET is involved in fruit ripening but also in other biological processes such as vegetative growth and seed germination (Smalle and Straeten, 1997; Bleecker and Kende, 2000); and several studies have indicated that it is involved in dormancy regulation and bud formation (Ruttink et al., 2007; Bai et al., 2013; Liu and Sherif, 2019). Applications of Ethephon, an ethylene producing compound, have also been used to reduce alternate bearing in apple (Bukovac et al., 2006)
In pear, increased transcript levels of 1-aminocyclopropane-1-carboxylate synthase (ACS), a gene regulating ethylene biosynthesis, were detected during endodormancy release; and changes in expression in several genes from the ethylene pathway were also observed in the transition from endo to ecodormancy (Bai et al., 2013). Wisniewski et al. (2015) identified in apple a homolog of the poplar EARLY BUD-BREAK 1 (EBB1) gene, MdEBB1, an ethylene responsive gene that plays a key role in determining time of bud break. MdEBB1 expression was low in apple dormant buds and increased during bud break. Furthermore, MdEBB1 expression and bud break occurred earlier in M.26 trees, whilst MdEBB1 expression and bud break were delayed approximately two weeks in transformed trees (T166) with overexpression of a peach CBF (C-Repeat Binding Factor) gene, indicating a link between ethylene pathways and bud break.
In a study with transgenic ethylene-insensitive birch trees, Ruonala et al. (2006) showed that under short-day conditions, mutant lines stopped growth like the wild-type; however, they did not form terminal buds and the development of dormancy was significantly delayed. A link between ABA and ethylene was also observed as no changes in ABA concentration were detected in apical buds of mutant trees when transferred to short-days, and application of ABA did not inhibit bud burst as it did in the wild-type (Ruonala et al., 2006). Mutants exhibited reduced apical dominance, producing three times more branches than the wild type and a bush-like appearance (Ruonala et al., 2006). These observations suggest an interaction not only between signalling pathways of ethylene and ABA but also with auxins, involved in the control of apical dominance (Cline, 1991; Cline, 2000).
4.2 Cytological changes
Early studies with apple reported changes in the water status in the buds during dormancy development, shifting from a bound state during endodormancy, to free water when ecodormancy was reached (Faust et al., 1991). Bound water is primarily restricted to the cell wall matrix whilst free water is mainly intracellular. The study observed that the change from bound to free water occurred earlier in the low-chill “Anna”, compared with the high-chill cultivar “Northern Spy”; and that the timing coincided with satisfaction of chilling requirements, relating bound water to cold resistance during dormancy and free water to growth resumption (Faust et al., 1991). Changes in the composition of lipids in cell membranes due to chilling exposure were reported in “Delicious” apple buds; the concentration of polar lipids increased as buds were formed and dormancy developed (Wang and Faust, 1990). As the major increase was found in linoleic acid, Erez (2000) suggested the interaction between the enzymes responsible of regulating linoleic acid (oleate desaturase and linoleate desaturase) as a key dormancy control mechanism, due to the different temperature ranges they required to be activated.
Cytological changes have been observed at different stages of the dormancy cycle in other species (Rinne et al., 2001; Gutierrez et al., 2002; Horvath et al., 2003). One of the key attributes of dormancy is the suspension of cell division and elongation (Horvath et al., 2003). In eukaryotes, the cell cycle has four phases: G1, S, G2, and M. The synthesis phase (S) is when DNA replication takes place, and the mitosis phase (M) is when the cell divides. Phases G1 and G2 are gap phases. During the first gap phase the cell expands and prepares for DNA replication whilst during G2 the cell prepares for mitosis. Based on observations with Arabidopsis and Pea plants, it is hypothesized that during dormancy, cells are arrested between phases G1 and S (Devitt and Stafstrom, 1995; Gutierrez et al., 2002).
Changes in cell-to-cell communications through plasmodesmata have also been reported in other woody perennials (Jian et al., 1997; Rinne et al., 2001). Notably, birch (Betula pubescens) plasmodesmata become blocked with deposition of callose (1,3-β-D glucan) during endodormacy, resulting in isolation of the cells until the apical meristem was exposed to the chilling conditions required to release dormancy (Rinne et al., 2001). Similarly, a decrease in the frequency of plasmodesmata in cell walls of apical buds and a reduction in the diameter of the pores was observed during dormancy induction in poplar (Populus deltoids) (Jian et al., 1997). These studies indicate an important role of plasmodesmata communication regulating dormancy, as the opening of these pathways allows the movement of certain hormones and molecules involved in dormancy control.
4.3 Reactive oxygen species and carbohydrate dynamics
Reactive oxygen species (ROS), such as Hydrogen peroxide (H2O2), are by-products of the metabolism of oxygen produced during photosynthesis and respiration in different organelles (Huang et al., 2019). ROS are involved in plant development (Considine and Foyer, 2014) and stress responses such as disease resistance (Grant and Loake, 2000). Several studies have highlighted a key role of ROS during dormancy, as they are involved in many metabolic processes regulating growth and hormone signalling pathways, as well as in glucose metabolism (Zhuang et al., 2013; Considine and Foyer, 2014; Beauvieux et al., 2018).
A gradual increase in H2O2 content with chilling accumulation, followed by a decrease after endodormancy break has been observed in apple (Sapkota et al., 2021b), pear (Kuroda et al., 2002) and grapevine flower buds (Pérez and Burgos, 2004; Pérez and Lira, 2005) amongst other crops. Furthermore, H2O2 concentrations remained low in pear buds that did not receive any chilling (Kuroda et al., 2002), and proteomic studies observed changes in the activity of enzymes regulating concentrations of H2O2 as Japanese pear trees transitioned from endo to ecodormancy, indicating a clear link between ROS and chilling accumulation (Takemura et al., 2015). At endodormancy break, Sapkota et al. (2021b) detected three times more H2O2 in the early-bloom cultivar “CrippsPink” compared to the late-flowering “Honeycrisp”, which could partially explain the difference in flowering times between these cultivars.
Concentrations of ROS and carbohydrates during dormancy are closely linked as ROS are by-products of carbohydrate metabolism (Considine and Foyer, 2014; Sapkota et al., 2021b). Temperate woody perennials, such as apple, accumulate carbohydrates before winter, usually in the form of starch. Bud development in spring depends on carbohydrates stored during the previous season, which are also essential to survive the winter months (Sauter et al., 1996). Carbohydrates play a crucial role during frost hardiness and cold acclimation by increasing freezing tolerance and supporting embolism restoration (Améglio et al., 2000; Améglio et al., 2004). As temperatures rise in spring, stored carbohydrates are degraded into soluble sugars and transported to different plant tissues (Bonhomme et al., 2010; Tixier et al., 2017). Phloem transport is highly restricted during winter, so carbohydrate transport through xylem becomes crucial for bud growth (Loescher et al., 1990; Decourteix et al., 2008; Ito et al., 2012).
Studies have shown a correlation between seasonal carbohydrate dynamics and dormancy progression in apple (Sivaci, 2006), pear (Ito et al., 2012), walnut (Bonhomme et al., 2010) and sweet cherry (Kaufmann and Blanke, 2017; Fadón et al., 2018) amongst others, with some suggesting that changes in carbohydrate concentrations could be used as physiological markers to distinguish cultivars with different chilling requirements (Fernandez et al., 2019). Carbohydrate concentration varies between species and plant tissues but overall, concentrations of soluble carbohydrate in floral buds peak during endodormancy and decrease before bud break, whilst those of starch remain low throughout winter (Bonhomme et al., 2005; Ito et al., 2012; Kaufmann and Blanke, 2017; Fernandez et al., 2019). Differences in soluble carbohydrate dynamics have been observed between apple cultivars; carbohydrate concentration in early-blooming “Cripps Pink” floral buds increased before endodormancy release but remained relatively stable in the late-flowering “Honeycrisp” (Sapkota et al., 2021b). In the same study, starch content declined as dormancy progressed, with a peak observed at the time of endodormancy release in both apple cultivars (Sapkota et al., 2021b). Similarly, Fadón et al. (2018), also reported an increase in starch in the ovary of sweet cherry floral buds at endodormancy break. Peaks of different carbohydrates during endodormancy have also been reported in xylem sap (Bonhomme et al., 2010; Ito et al., 2012; Ito et al., 2013). In apple xylem sap, sorbitol concentrations increase with colder temperatures (Raese et al., 1977; Gonzalez Noguer, 2022) and studies in pear have observed low sorbitol concentration in trees receiving insufficient chilling (Ito et al., 2013).
5 Genetic regulation of apple bud dormancy
Although the influence of environmental factors on flowering time is evident, bud break time is a highly heritable traits (Labuschagné et al., 2002; Celton et al., 2011). Important advances in our understanding of the genetics behind dormancy have occurred during the last decade and various studies have identified candidate genes for dormancy regulation in apple (Mimida et al., 2015; Wisniewski et al., 2015; Wu et al., 2017). One group of genes have received most attention are the DORMANCY-ASSOCIATED MADS-box (DAM) genes, phylogenetically related to the SHORT VEGETATIVE PHASE (SVP) genes from Arabidopsis thaliana (Mimida et al., 2015; Porto et al., 2016; Falavigna et al., 2019).
DAM genes were first discovered in a peach mutant called EVERGROWING (EVG), which showed continuous growth and an inability to enter dormancy (Bielenberg et al., 2004; Bielenberg et al., 2008). Since then, they have been identified in many fruit trees, including apple (Celton et al., 2011; Mimida et al., 2015; Wisniewski et al., 2015; Porto et al., 2016). Several DAM genes have been described and in apple there is a debate on the terminology to use as authors have assigned different names for each gene (Mimida et al., 2015; Wisniewski et al., 2015); this review uses the nomenclature proposed by Porto et al. (2016).
DAMs gene expression is highly correlated with dormancy progression, being up and down regulated at different points in the cycle (Mimida et al., 2015; Porto et al., 2016; Wu et al., 2017). Expression of MdDAM1 in “Jonathan” trees exposed to natural chilling peaked during bud set and then decreased, whilst MdDAM2 was high in summer and decreased with dormancy induction (Mimida et al., 2015). Similar results were observed under artificial chilling for the cultivars “Fuji Standard”, “Royal Gala” and “Castel Gala” (Porto et al., 2016). MdDAM2 transcription peaked in the summer whilst peaks during winter were observed for MdDAM1, MdDAM3 and MdDAM4. In this study, all cultivars followed similar patterns but MdDAM1, MdDAM3 and MdDAM4 expression in “Castel Gala” decreased quicker, indicating a cultivar-specific gene expression linked to chilling requirements as “Castel Gala” is a low-chill variety (Porto et al., 2016).
The CONSTANS (CO)/FLOWERING LOCUS T (FT) regulon plays a critical role in controlling flowering time and growth cessation in species where dormancy is induced by short days (Horvath, 2009). Whilst the CO-FT role in species with temperature-induced dormancy has not been demonstrated, FT is negatively regulated by the FLOWERING LOCUS C (FLC), a MADS-box gene which in apple has been linked to chilling accumulation and dormancy progression (Porto et al., 2015). Furthermore, FLC-like genes have been located in Linkage Group 9 (LG9), a region containing QTLs linked to bud break in apple (Allard et al., 2016; Miotto et al., 2019).
Another gene that has been linked to dormancy regulation in apple is the CBF (C-Repeat Binding Factor) gene; overexpression of a peach CBF gene in transgenic apple trees increased cold hardiness, delayed bud break, reduced growth, and induced early dormancy (Wisniewski et al., 2011; Artlip et al., 2014). Transgenic lines also showed a different expression of DAM genes MdDAM1 and MdDAM3 compared to non-transformed trees (Wisniewski et al., 2015), indicating again these genes are important candidates for dormancy regulation.
In recent years, studies using transgenic apple trees have identified the functional role of some of these DAM genes and have provided key information to better understand the genetic regulation of apple dormancy. Overexpression of DAM6 repressed bud break and inhibited growth in transgenic apple trees, it also increased abscisic acid levels and decreased cytokinins contents at the end of dormancy (Yamane et al., 2019). In another study, “Royal Gala” transgenic trees with reduced DAM/SVP gene expression were unable to enter dormancy and showed continuous growth for over three years (Wu et al., 2021). A range of other differences were observed in this genotype, including different phytohormone composition, changes in the FT pathway and in genes involved in plasmodesmata closure; providing key evidence for the role of DAM/SVP genes in apple dormancy (Wu et al., 2021).
An understanding of the genetic determinism of dormancy regulation and, particularly, chilling requirements, would improve apple breeding programmes aiming to select suitable varieties for the climate of each growing region. Molecular techniques provide a great tool to improve our understanding of the dormancy process but it will take several decades for this information to inform and impact on breeding programmes. Meanwhile, it is necessary to use other available methodologies to anticipate how existing cultivars will perform under different climates.
6 Chilling and heat accumulation models
Phenological models in temperate fruit trees use air temperature to forecast flowering dates (Darbyshire et al., 2013; Chuine et al., 2016). Models used in apple combine a chilling model to quantify chilling accumulation (i.e. (Weinberger, 1950; Richardson et al., 1974; Fishman et al., 1987a) (Table 1), with a thermal time model to calculate heat accumulation during ecodormancy (Anderson et al., 1986).
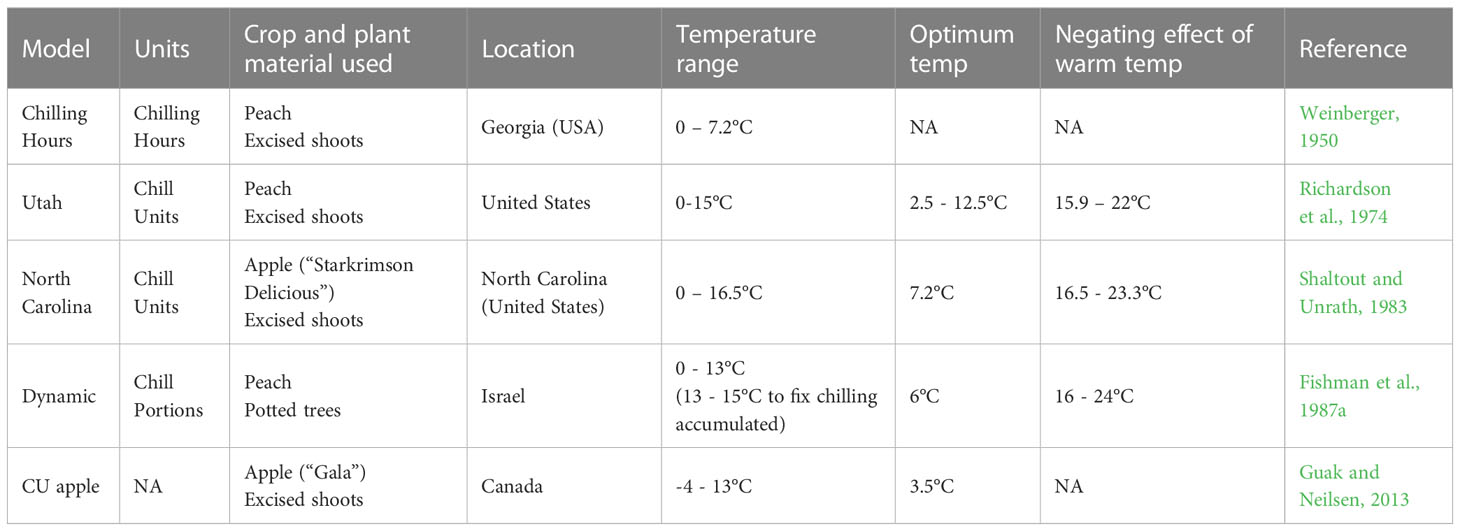
Table 1 Summary of existing chilling models; including: name of the model, units used to calculate chilling, crop and plant material used to develop the model, temperature range contributing to chilling, optimum temperature, temperature range that negates previous chilling accumulated and reference of the study where the model was developed.
Various chilling models have been developed to measure the exposure to effective cold temperatures in deciduous trees (Table 1). These models aim to estimate the chilling requirement of commercial fruit varieties with the goal of determining their regional suitability (Campoy et al., 2011b; Luedeling, 2012; Darbyshire et al., 2013). Despite major efforts to characterize the biological processes involved in tree dormancy, the currently available chill models are simply based on empirical information and do not include robust biological or physiological parameters (Luedeling, 2012; Fadón, et al., 2020a).
The Chilling Hours model, developed with peach shoots, was the first attempt to develop a chilling accumulation model and it assumes that all temperatures between 0 and 7.2°C are equally effective towards chilling accumulation (Weinberger, 1950). It is unclear how this temperature range was chosen as no experiments comparing the effectiveness of different temperatures were carried out; however, probably due to its simplicity, this model is still frequently used. The Utah model, also developed for two peach cultivars, was the first model to assign differential chilling efficiencies to distinct temperature ranges and to introduce the concept of “chill negation” (Richardson et al., 1974). The Utah model assumes that temperatures above 15.9°C have a negative effect on chill accumulation and that optimum temperatures to accumulate chilling range between 2.5 and 12.5°C (Richardson et al., 1974).
The Dynamic model (Fishman et al., 1987a; Fishman et al., 1987b) was developed in the Mediterranean climate of Israel after carrying out numerous controlled environment experiments with potted peach trees (Erez and Lavee, 1971; Erez et al., 1979; Couvillon and Erez, 1985). The Dynamic model is based on the idea that the accumulation of chill is a two-step process, the first step is chilling accumulation, promoted by low temperatures (0-13°C) and reversible by high temperatures (16-24°C). The second step, triggered by moderate temperatures (13-15°C), fixes chilling accumulated which becomes irreversible. In other words, high temperatures can negate chilling accumulated up to a certain point, after which high temperatures have no chilling-negation effect (Erez and Couvillon, 1987). This two-step process is based on the concept that there is a “thermally unstable precursor” (not yet identified) that promotes or prevents the transition from one step to the other (Fishman et al., 1987a).
The North Carolina model was the first model developed for apple, using excised shoots of the cultivar “Starkrimson Delicious” (Shaltout and Unrath, 1983). It is based on the principles of the Utah model (Richardson et al., 1974) but with temperature parameters calibrated for “Starkrimson Delicious”. A more recent adaptation of the Utah model, named CUapple, was developed for “Gala” apple (Guak and Neilsen, 2013). Two main differences to the Utah model were defined, which greatly improved the performance of CUapple, especially for predicting bud break during warmer years: (i) chilling started accumulating after harvest and (ii) sub-zero temperatures were considered to contribute to chilling (Guak and Neilsen, 2013).
Whilst a range of chilling models exist, most studies predicting flowering time in fruit trees use the Growing Degree Hours (GDH) model to quantify heat accumulation (Anderson et al., 1986). The GDH model assigns varying heat accumulation efficiencies to temperatures above a base temperature of 4°C, with an optimum temperature of 25°C and a critical temperature threshold of 36°C.
In order to forecast flowering dates, a chilling and heat model were initially combined in a sequential manner; however, more recent approaches consider an overlap between these events, representing a more gradual transition from chilling to heat accumulation (Pope et al., 2014; Guo et al., 2015; Darbyshire et al., 2016b; Drepper et al., 2020). In recent years, a Partial Least Squares (PLS) Regression approach has been proposed as a tool to delineate the chilling and heat accumulation phases (Luedeling and Gassner, 2012; Luedeling et al., 2013). This approach requires historical temperature and phenology records for each calendar day of the dormancy season and assumes that flowering is the result of the combination of winter chill and spring heat. Thus, CR and HR are accumulated independently but chilling and heat periods can overlap. Most studies using this approach have observed that, from the start of dormancy, periods of chilling and heat accumulation appear to alternate (Guo et al., 2014; Guo et al., 2015; Drepper et al., 2020).
Appropriate model selection is fundamental to predict how cultivars will perform in different growing regions and climates. As CR and HR information is rarely available, growers often base planting decisions on approximate knowledge of flowering dates for a particular cultivar. Whilst existing chilling and heat accumulation models provided accurate flowering time predictions in the cultivars and location used to develop them, results are highly variable when they are applied to other areas or species, particularly in warm regions (Luedeling and Brown, 2011). In apple, even the best predictions usually fall within more than 5 days from observed dates (Darbyshire et al., 2017; Drepper et al., 2020), and predictions in warmer locations deviated between 7.3 and 33 days from the observed dates, depending on the model used (Darbyshire et al., 2017). The bud break temperature response is different between apple cultivars (Thompson et al., 1975; Naor et al., 2003; Gonzalez Noguer, 2022), thus models should be re-calibrated for each specific cultivar and location, but unfortunately, this step is usually omitted (Guo et al., 2014; Darbyshire et al., 2016b; Parkes et al., 2020), with inevitable consequences for accuracy.
Winter chilling projections under climate change scenarios highlight the disconnect between existing chilling accumulation models (Luedeling and Brown, 2011). Although winter chill reductions are predicted with all models, the severity of these impacts is diverse and highly dependent on the chilling model used, as well as on the species and locations studied (Luedeling and Brown, 2011; Darbyshire et al., 2016b). By applying an ensemble of future climate scenarios, recent studies forecasted a significant decrease in winter chill availability in important apple growing regions (Funes et al., 2016; Parkes et al., 2020; Delgado et al., 2021a). Many authors have identified the Dynamic model as the most accurate model for temperate fruit trees in mild-winter areas (Campoy et al., 2011b; Luedeling and Brown, 2011; Zhang and Taylor, 2011; Luedeling, 2012); although the physiological basis behind its hypothesis has yet to be determined.
7 Chilling and heat requirements of apple cultivars
An accurate knowledge of the agroclimatic requirements of apple cultivars is a crucial prerequisite for selecting germplasm adapted to predicted future climatic conditions and in choosing parents for controlled hybridizations in breeding programmes. Consistently profitable yields in commercial fruit orchards require the average annual accumulated chill at a particular site to exceed the cultivar-specific chill requirement of the cultivars in at least 90% of all years (Luedeling et al., 2009). Nevertheless, it has been noted that the wide variety of methodologies and winter chill models used for measuring agroclimatic requirements (see section 6) have often resulted in marked inconsistencies in the results reported for the same cultivar across different climates (Fadón, et al., 2020b). The lack of standardization in the methodologies used to determine the endodormancy release date hampers the comparability of results among studies and partly limits the confidence in regional suitability assessments of temperate fruit cultivars (Dennis, 2003).
Accurate information on CR and HR of apple cultivars is a useful tool for orchard managers, agronomists, breeders, and researchers working on dormancy-related topics. A summary of existing CR and HR for a range apple cultivars is presented in Table 2, including the methodology used for estimating chill and heat requirements (experimental or statistical), the location where experiments were conducted and the main use of each cultivar when available (dessert, cider, cooking, crab or rootstock). To facilitate comparison, chilling requirements are only shown in Chill Hours, Chill Units and Chill Portions determined by the Chilling Hours (Weinberger, 1950), Utah (Richardson et al., 1974) and Dynamic models (Fishman et al., 1987a), respectively. Heat requirements were always calculated using the Growing Degree Hours model (Anderson et al., 1986).
This work compiles the agroclimatic requirements of 128 cultivars. For 25 cultivars, including some of the most traded cultivars in the world, more than one study is available. If the same study reported multi-site values for the same cultivar, information for each location is shown. Chill quantification was only available in Chill Hours for approximately half of the cultivars compiled, whilst chill accumulation in Chill Portions was only reported for 66 cultivars.
Chilling requirements reported in the literature varied significantly between cultivars (Table 2). According to the Dynamic Model, the cultivar “Eva” had the lowest CR (13 CP) in Brazil (El Yaacoubi et al., 2016), whereas the highest CR (90.2 CP) was reported for “Regona” in Spain (Delgado et al., 2021a). Considering the Chill Hours Model, “Ana” was the lowest-chill cultivar (218 CH) whilst “Marin Oufroy” required the highest chilling accumulation at 1423 CH (Table 2). Heat requirements (HR) ranged between 4268 GDH (“Cox Orange”) and 22402 GDH (“Gala”). Although a wide range of chill requirements have been previously reported in apple cultivars (Table 2), the most extensively planted cultivars, including “Golden Delicious”, “Granny Smith”, “Gala” or “Fuji”, have chilling requirements ranging from 40 and 70 CP.
Almost three-quarters of the agroclimatic estimates shown in Table 2 were determined through experimental approaches (i.e., forcing shoots or potted trees in a growth chamber and assessing the phenological evolution of buds after different periods of cold exposure). Young and Werner (1985) developed a procedure for forcing potted trees whereas the rest of authors used excised shoots collected from orchard trees as an experimental sample. On the other hand, nine studies statistically determined the chilling and heat requirements of 36 cultivars. The most common statistical methodology between the cited studies is based on applying PLS regression (Luedeling and Gassner, 2012) to delineate the chilling and forcing phases (Díez-Palet et al., 2019; El Yaacoubi et al., 2020; Delgado et al., 2021b). Also based on long-term phenological records, Funes et al. (2016) statistically determined CR and HR by using the chilling-forcing sequential model developed with almond by Alonso et al. (2005). In a recent study, Fernandez et al. (2021) developed a procedure for forcing potted trees to different artificial climatic conditions within the same experimental season aiming to determine the dormancy phases through PLS regression. In all these studies, after the delineation of the chilling and forcing phases, temperature dynamics during both periods were analyzed using existing chilling models.
It should be noted that a wide variation in chilling requirement estimates was found for the same cultivar at different locations (Table 2). The most obvious case is “Fuji”, as El Yaacoubi et al. (2016) reported a particularly low requirement of 24.5 CP in Brazil, whereas Parkes et al. (2020) showed that this cultivar required 77 CP to break endodormancy in Australia. Using a similar experimental design but with a varying length of the forcing period in the growth chamber, the popular apple variety “Granny Smith” was shown to need 72.8 CP in Australia (Parkes et al., 2020) whereas Delgado et al. (2021a) reported a CR of 59.3 CP in north-western Spain. Using the same flowering dataset from north-eastern Spain, Díez-Palet et al. (2019) and Funes et al. (2016) delivered contrasting results for the same cultivar depending on the statistical approach. For instance, “Aporo” varied from 37.8 to 66.4 CP and “Red Chief” from 40.2 to 66.4 CP. Likewise, using the Tabuenca test to determine endodormancy release dates (Tabuenca, 1964), El Yaacoubi et al. (2016) found large differences for the same cultivar in divergent environments. For example, cv. “Gala” was shown to have a chill requirement of 58.3 CP in France, contrasting with a significantly lower chill requirement in Brazil (25.6 CP). Relatively homogeneous results between the different studies were found for cv. “Golden Delicious” ranging from 50 to 62 CP (Table 2).
To the best of our knowledge, there are only few published studies reporting experimentally and statistically the CR and HR for the same cultivar and location. A comparison between experimental and statistical approaches for “Cripps Pink” in Applethorpe (Australia) revealed marked differences; Darbyshire et al. (2017) reported that this cultivar needed 52 CP according to the PLS regression analysis whereas Parkes, Darbyshire and White, (2020) found a much higher requirement of 73 CP by forcing shoots throughout the dormant period. Similar variation was found for the cultivar “Gala” in northern Morocco where El Yaacoubi et al. (2016) reported 61 CP in a controlled environment experiment, contrasting with 44 CP found by applying the PLS approach (El Yaacoubi et al., 2020). Of particular interest are the studies conducted in north-western Spain by Delgado et al. (2021a) and Delgado et al. (2021b), who evaluated the agroclimatic requirements for a set of apple landraces using both approaches. Here, substantial differences in the cultivar-specific CR estimates were obtained for the same cultivar when both methodologies were compared. For example, the smallest variation between approaches according to the Dynamic model was found in cv. “Perico” (9.8 CP) and the highest in cv. “Collaos” (24.3 CP; Table 2). All these studies comparing experimentally and statistically derived CR estimates showed that the PLS regression consistently predicted lower requirements for the same cultivar and geographical location than any experimental approach.
Based on the information compiled in Table 2, we suggest a classification of cultivars in terms of their chilling requirements; from very low (less than 30 CP, 600 CU and 300 CH) to very high (above 80 CP, 1400 CU and 1500 CH) (Table 3). According to this classification and only based on the Dynamic model outputs, approximately half the cultivars are included in the categories low to moderate and moderate to high chill (i.e., from 40 to 60 CP). Only 5% of the cultivars can be considered to need very low chill, and a similar percentage of cultivars comprised in the very high-chill group has been reported.
8 Discussion
Most temperate fruit tree species, including apple, require adaptation strategies to climate change in many parts of the world. As the consequences of climate change become more evident, understanding the responses of apple cultivars to temperature is more important than ever. To date, chilling requirements and, to a lesser extent, heat requirements, are the most important existing metrics to evaluate the suitability of apple cultivars to different growing regions and climates. However, the variability of CR values compiled here for the same apple cultivar, and those previously reported for stone fruit varieties (Fadón et al., 2020b), raises questions as to the accuracy of these parameters for informing planting decisions. Differences in CR and HR reported for the same cultivar highlight the limitations of existing statistical and empirical methods used in their estimation, as identified in recent reviews (Campoy et al., 2011b; Luedeling, 2012; Fadón and Rodrigo, 2018).
As time of bud break is determined by both chilling and heat accumulation, models need to capture the complex interaction between both temperature-driven processes if they are to generate accurate outputs. Studies have shown that improved phenology predictions can be achieved with a partial or complete overlap between processes (Harrington et al., 2010; Pope et al., 2014; Darbyshire et al., 2016a; Luedeling et al., 2021). Previous studies have also suggested that longer chilling can reduce heat requirement to bud break (Ruiz et al., 2007; Darbyshire et al., 2013; Guo et al., 2014). Since it is unlikely that chilling and heat accumulation are independent processes, they should be investigated in combination to generate better predictions in a climate change context (Gonzalez Noguer, 2022).
Another important factor to consider, and that is currently lacking in chilling and heat accumulation models, is that dormancy is a continuous annual process and that flower development occurs over two seasons. As such, all phases are tightly interconnected and warmer temperatures during dormancy induction have been shown to increase chilling requirements and delay bud break (Cook and Jacobs, 2000; Heide, 2003). Existing chilling models only consider the effect of temperature from the dormant state, and so any climatic variability during the initial stages of flower development and dormancy induction is not captured, which likely contributes to the variability in estimates of CR (Louw et al., 2023). With the exception of the Dynamic model, all chilling (and heat) accumulation models harbor the inherent assumption that the effect of temperature on bud break remains constant throughout the process. In the Dynamic model, temperatures can have a different effect if they occur during the first or second stage of chilling accumulation (Fishman et al., 1987a), which is likely a more plausible representation of the dormancy process and perhaps contributes to the better performance of this model. Nevertheless, the effect of cold/warm temperatures on chilling and heat accumulation is likely to be more complex than this.
Empirical methods to estimate CR lack standardization (Dennis, 2003), and differences in plant material (excised shoots, potted trees), environmental conditions during forcing, or parameters measured to establish chill requirement satisfaction each contribute to the different CR values estimated for the same cultivar (Table 2). Many investigations into the chilling requirements of fruit tree species utilize experiments with excised shoots (Hauagge and Cummins, 1991; Cook and Jacobs, 2000; Guak and Neilsen, 2013; Anzanello et al., 2014), and the inherent assumption is that outputs can be reliably extrapolated to field-grown trees. The Dynamic model was the only model developed using potted trees (Fishman et al., 1987a), perhaps contributing to its more accurate performance. A clear role for root(stock)-to-shoot signalling during dormancy has not been established, but an involvement seems logical as warmer temperatures in spring are linked to the resumption of osmotically-driven sap flow. Intact xylem and phloem connections (Améglio et al., 2000; Tixier et al., 2017), and a functional polar auxin transport pathway between roots and shoots are crucial for plant growth and development (Friml and Palme, 2002), and so whilst the response of excised shoots to environmental cues might superficially resemble those of an intact mature tree for a short time following excision, a very rapid divergence in behavior is to be expected.
Much research is required to improve model performance if phenology predictions resulting from the combination of chill and heat accumulation models are to be used to inform orchard management activities, and cultivar selection based on these criteria would be unwise. Another source of variability in phenology predictions is that many studies usually omit the chilling model calibration step (e.g. Ruiz et al., 2007; Campoy et al., 2012; Parkes et al., 2020), the inherent assumption being that the chilling temperature range and the optimum chilling temperature for any given cultivar are the same as those of the cultivars used to develop the original models. Differences in the temperature-response to chilling between apple cultivars have been observed (Gonzalez Noguer, 2022), and using cultivar-specific parameters can improve flowering time predictions (Darbyshire et al., 2017; Egea et al., 2021). Calibrating models at a cultivar level would not be practical or affordable, as new cultivars are released constantly. However, a range of model parameters based on the chilling requirement groups suggested above could be a useful tool for future research into the impacts of climate change on apple production.
Tree age, rootstock, soil type and depth, water and nutrient availability and acquisition, pests and diseases, effective pollination period, availability and activity of pollinators, and orchard practices such as pruning, canopy management, weeding strategies, etc., all influence growth and fruit production (Jackson, 2003; Naor et al., 2008; Ramírez and Davenport, 2013), and so effects on the dormancy cycle can be expected. Whilst air temperature is the main factor regulating dormancy, other factors could underpin the differences in CR reported for the same variety.
As summarized here, important advances have been made in recent years regarding our knowledge of the physiological (Liu and Sherif, 2019) and genetic (Mimida et al., 2015; Wisniewski et al., 2015; Wu et al., 2017) mechanisms regulating dormancy in apple. But unfortunately, there remains a disconnect between this knowledge and the development of chill and heat accumulation models required to estimate CR and HR. As previously highlighted (Campoy et al., 2011b; Luedeling, 2012; Fadón et al., 2020a), anticipating how different apple cultivars will respond to climate change will be challenging until physiological and genetic findings guide the development of more accurate models. Meanwhile, CR should be calculated using the Dynamic model as this is the most accurate existing model for a wide range of climates and cultivars (Luedeling and Brown, 2011).
Questions as to the suitability of using published CR estimates to establish the climatic needs of a cultivar remain. It is important to mention that some studies in apple reported that yield was not significantly impacted when winter chill accumulation in the orchard was lower that the estimated CR (Parkes et al., 2020; Delgado et al., 2021a), suggesting that a better understanding is needed to improve the quantification of these metrics. The introduction of new cultivars requires a wider risk assessment (Campoy et al., 2019) and factors such as consumer preferences, productivity and tolerance to pests and diseases are of high importance for cultivar selection. It has been suggested that low-chill apple cultivars might have low disease resistance (Inamahoro, 2020) and that heat availability for optimal fruit ripening in the summer might be of concern for some varieties (Chmielewski and Rötzer, 2002).
Given the limitations above mentioned regarding CR and HR metrics, data compiled in this review show the extensive range of CR in apple cultivars, spanning a wide range of temperature conditions. This is hopeful for breeding programmes aiming to identify varieties with lower chill requirements. Several new cultivars are released annually, but breeders or companies managing licensing of proprietary cultivars rarely provide agroclimatic requirements in their portfolios. It is essential that organizations developing new varieties carry out tests in a variety of environments that incorporate the variable growing conditions predicted as the climate changes, including reduced winter chilling and heatwaves.
A holistic and collaborative approach is required to integrate existing knowledge on the mechanisms regulating dormancy into the development of improved predictive models, so that meaningful chilling and heat requirement measurements can be generated and used by growers to select apple cultivars for future plantings.
Author contributions
All authors contributed to conception and design of the manuscript. CGN wrote the first draft of the manuscript. CGN and AD wrote sections of the manuscript. All authors contributed to manuscript revision, read, and approved the submitted version.
Funding
CGN received funding from the Agriculture and Horticulture Development Board (AHDB) (grant number CTP_FCR_2017_2) as part of the Collaborative Training Partnership (CTP) formed by the Biotechnology and Biological Sciences Research Council (BBSRC) and UK leading fruit industry organisations. AD received funding from PRIMA, program supported under H2020, the European Union’s Framework programme for research and innovation (“AdaMedOr” project; grant number PCI2020-112113 of the Spanish Ministry of Science and Innovation).
Conflict of interest
The authors declare that the research was conducted in the absence of any commercial or financial relationships that could be construed as a potential conflict of interest.
Publisher’s note
All claims expressed in this article are solely those of the authors and do not necessarily represent those of their affiliated organizations, or those of the publisher, the editors and the reviewers. Any product that may be evaluated in this article, or claim that may be made by its manufacturer, is not guaranteed or endorsed by the publisher.
References
Alburquerque N., García-Montiel F., Carrillo A., Burgos L.. (2008). Chilling and heat requirements of sweet cherry cultivars and the relationship between altitude and the probability of satisfying the chill requirements. Environ. Exp. Bot. 64 (2), 162–170. doi: 10.1016/j.envexpbot.2008.01.003
Allard A., Bink M. C. A. M., Martinez S., Kelner J. J., Legave J.-M., di Guardo M., et al. (2016). Detecting QTLs and putative candidate genes involved in budbreak and flowering time in an apple multiparental population. J. Exp. Bot. 67 (9), 2875–2888. doi: 10.1093/jxb/erw130
Alonso J. M., Ansón J. M., Espiau M. T., Company R. S.. (2005). Determination of endodormancy break in almond flower buds by a correlation model using the average temperature of different day intervals and its application to the estimation of chill and heat requirements and blooming date. J. Am. Soc. Hortic. Sci. 130 (3), 308–318. doi: 10.21273/JASHS.130.3.308
Améglio T., Guilliot A., Julien J. L., Alves G., Valentin V., Pétel G.. (2000). “Effect on bud break of walnut tree,” in Dormancy in plants: from whole plant behaviour to cellular control. (Wallingford UK: CABI Publishing), 109–120.
Améglio T., Decourteix M., Alves G., Valentin V., Sakr S., Julien J. L., et al. (2004). Temperature effects on xylem sap osmolarity in walnut trees: evidence for a vitalistic model of winter embolism repair. Tree Physiol. 24 (7), 785–793. doi: 10.1093/treephys/24.7.785
Anderson J. L., Richardson E. A., Kesner C. D. (1986). Validation of chill unit and flower bud phenology models for “Montmorency” sour cherry. Acta Hortic. 184, 71–78. doi: 10.17660/ActaHortic.1986.184.7
Anzanello R., Fialho F. B., dos Santos H. P., Bergamaschi H., Marodin G. A. B.. (2014). Bud dormancy in apple trees after thermal fluctuations. Pesquisa. Agropecuaria. Bras. 49 (6), 457–464. doi: 10.1590/S0100-204X2014000600007
Artlip T. S., Wisniewski M. E., Norelli J. L. (2014). Field evaluation of apple overexpressing a peach CBF gene confirms its effect on cold hardiness, dormancy, and growth. Environ. Exp. Bot. 106, 79–86. doi: 10.1016/j.envexpbot.2013.12.008
Ashcroft G. L., Richardson E. A., Seeley S. D. (1977). A statistical method of determining chill unit and growing degree hour requirements for deciduous fruit Trees1. HortScience 12 (4), 347–348. doi: 10.21273/HORTSCI.12.4.347
Atkinson C. J., Brennan R. M., Jones H. G. (2013). Declining chilling and its impact on temperate perennial crops. Environ. Exp. Bot. 91, 48–62. doi: 10.1016/j.envexpbot.2013.02.004
Bai S., Saito T., Sakamoto D., Ito A., Fujii H., Moriguchi T.. (2013). Transcriptome analysis of Japanese pear (Pyrus pyrifolia nakai) flower buds transitioning through endodormancy. Plant Cell Physiol. 54 (7), 1132–1151. doi: 10.1093/pcp/pct067
Bartolini S., Piccolo E., Remorini D. (2020). Different summer and autumn water deficit a ff ect the floral di ff erentiation and flower bud growth in apricot (Prunus armeniaca l.). Agronomy 10, 1–13. doi: 10.3390/agronomy10060914
Beauvieux R., Wenden B., Dirlewanger E. (2018). Bud dormancy in perennial fruit tree species: a pivotal role for oxidative cues. Front. Plant Sci. 9. doi: 10.3389/fpls.2018.00657
Bertelsen M. G., Tustin D. S., Waagepetersen R. P. (2002). Effects of GA3 and GA4 + 7 on early bud development of apple. J. Hortic. Sci. Biotechnol. 77 (1), 83–90. doi: 10.1080/14620316.2002.11511462
Bielenberg D. G., Wang Y., Fan S., Reighard G. L., Scorza R., Abbott A.G.. (2004). A deletion affecting several gene candidates is present in the evergrowing peach mutant. J. Heredity. 95 (5), 436–444. doi: 10.1093/jhered/esh057
Bielenberg D. G., Wang Y., Li Z., Zhebentyayeva T., Fan S., Reighard G. L., et al. (2008). Sequencing and annotation of the evergrowing locus in peach [Prunus persica (L.) batsch] reveals a cluster of six MADS-box transcription factors as candidate genes for regulation of terminal bud formation. Tree Genet. Genomes 4 (3), 495–507. doi: 10.1007/s11295-007-0126-9
Bleecker A. B., Kende H. (2000). Ethylene: a gaseous signal molecule in plants. Annu. Rev. Cell Dev. Biol. 16 (1), 1–18. doi: 10.1146/annurev.cellbio.16.1.1
Bonhomme M., Rageau R., Lacointe A., Gendraud M.. (2005). Influences of cold deprivation during dormancy on carbohydrate contents of vegetative and floral primordia and nearby structures of peach buds (Prunus persica l. batch). Sci. Hortic. 105 (2), 223–240. doi: 10.1016/j.scienta.2005.01.015
Bonhomme M., Peuch M., Ameglio T., Rageau R., Guilliot A., Decourteix M., et al. (2010). Carbohydrate uptake from xylem vessels and its distribution among stem tissues and buds in walnut (Juglans regia l.). Tree Physiol. 30 (1), 89–102. doi: 10.1093/treephys/tpp103
Bukovac M. J., Sabbatini P., Schwallier P. G. (2006). Modifying alternate bearing of spur-type “Delicious” apple with ethephon. HortScience 14 (7). doi: 10.3389/fpls.2019.01136
Campoy J. A., Ruiz D., Cook N., Allderman L., Egea J.. (2011a). High temperatures and time to budbreak in low chill apricot “Palsteyn”. towards a better understanding of chill and heat requirements fulfilment. Sci. Hortic. 129 (4), 649–655. doi: 10.1016/j.scienta.2011.05.008
Campoy J. A., Ruiz D., Allderman L., Cook N., Egea J.. (2012). The fulfilment of chilling requirements and the adaptation of apricot (Prunus armeniaca l.) in warm winter climates: an approach in murcia (Spain) and the Western cape (South Africa). Eur. J. Agron. 37 (1), 43–55. doi: 10.1016/j.eja.2011.10.004
Campoy J. A., Darbyshire R., Dirlewanger E., Quero-García J., Wenden B.. (2019). Yield potential definition of the chilling requirement reveals likely underestimation of the risk of climate change on winter chill accumulation. Int. J. Biometeorol. 63 (2), 183–192. doi: 10.1007/s00484-018-1649-5
Campoy J. A., Ruiz D., Egea J. (2011b). Dormancy in temperate fruit trees in a global warming context: a review. Sci. Hortic. 130 (2), 357–372. doi: 10.1016/j.scienta.2011.07.011
Celton J. M., Martinez S., Jammes M. J., Bechti A., Salvi S., Legave J. M., et al. (2011). Deciphering the genetic determinism of bud phenology in apple progenies: a new insight into chilling and heat requirement effects on flowering dates and positional candidate genes. New Phytol. 192 (2), 378–392. doi: 10.1111/j.1469-8137.2011.03823.x
Champagnat P. (1989). Rest and activity in vegetative buds of trees. Ann. For. Sci. 46, 9–26. doi: 10.1051/forest:19890501
Chmielewski F.-M., Rötzer T. (2002). Annual and spatial variability of the beginning of growing season in Europe in relation to air temperature changes. Clim. Res. 19 (3), 257–264. doi: 10.3354/cr019257
Chuine I., Bonhomme M., Legave J. M., García de Cortázar-Atauri I., Charrier G., Lacointe A., et al. (2016). Can phenological models predict tree phenology accurately in the future? the unrevealed hurdle of endodormancy break. Global Change Biol. 22 (10), 3444–3460. doi: 10.1111/gcb.13383
Cline M. G. (2000). Execution of the auxin replacement apical dominance experiment in temperate woody species. Am. J. Bot. 87 (2), 182–190. doi: 10.2307/2656904
Considine M. J., Foyer C. H. (2014). Redox regulation of plant development. Antioxid. Redox Signaling 21 (9), 1305–1326. doi: 10.1089/ars.2013.5665
Cook N. C. (2010). Apple production under conditions of sub-optimal winter chilling in south Africa. Acta Hortic. 872, 199–204. doi: 10.17660/ActaHortic.2010.872.26
Cook N. C., Bellstedt D. U., Jacobs G. (2001). Endogenous cytokinin distribution patterns at budburst in granny smith and braeburn apple shoots in relation to bud growth. Sci. Hortic. 87 (1–2), 53–63. doi: 10.1016/S0304-4238(00)00161-8
Cook C., Jacobs G. (2000). Progression of apple (Malus × domestica borkh.) bud dormancy in two mild winter climates. J. Hortic. Sci. Biotechnol. 75 (2), 233–236. doi: 10.1080/14620316.2000.11511229
Cooke J. E. K., Eriksson M. E., Junttila O. (2012). The dynamic nature of bud dormancy in trees: environmental control and molecular mechanisms: bud dormancy in trees. Plant. Cell Environ. 35 (10), 1707–1728. doi: 10.1111/j.1365-3040.2012.02552.x
Cornille A., Antolín F., Garcia E., Vernesi C., Fietta A., Brinkkemper O., et al. (2019). A multifaceted overview of apple tree domestication. Trends Plant Sci. 24 (8), 770–782. doi: 10.1016/j.tplants.2019.05.007
Couvillon G. A., Erez A. (1985). Influence of prolonged exposure to chilling temperatures on bud break and heat requirement for bloom of several fruit species. J. Am. Soc. For. Hortic. Sci. 110 (1), 47–50. doi: 10.21273/JASHS.110.1.47
Cutting J. G. M., Strydom D. K., Jacobs G., Bellstedt D. U., Van Der Merwe K. J., Weiler E.W.. (1991). Changes in xylem constituents in response to rest-breaking agents applied to apple before budbreak. J. Am. Soc. Hortic. Sci. 116 (4), 680–683. doi: 10.21273/JASHS.116.4.680
Darbyshire R., Farrera I., Martinez-Lüscher J., Leite G. B., Mathieu V., El Yaacoubi A., et al. (2017). A global evaluation of apple flowering phenology models for climate adaptation. Agric. For. Meteorol. 240–241, 67–77. doi: 10.1016/j.agrformet.2017.03.021
Darbyshire R., Goodwin I., Pope K. S. (2015). Using several flowering phenology models to statistically determine’Cripps pink’apple chilling requirement. X. Int. Symposium. Model. Fruit Res. Orchard. Manage. 1160, 185–192. doi: 10.17660/ActaHortic.2017.1160.27
Darbyshire R., Measham P., Goodwin I. (2016a). A crop and cultivar-specific approach to assess future winter chill risk for fruit and nut trees. Climatic. Change 137 (3–4), 541–556. doi: 10.1007/s10584-016-1692-3
Darbyshire R., Pope K., Goodwin I. (2016b). An evaluation of the chill overlap model to predict flowering time in apple tree. Sci. Hortic. 198, 142–149. doi: 10.1016/j.scienta.2015.11.032
Darbyshire R., Webb L., Goodwin I., Barlow E. W. R. (2013). Evaluation of recent trends in Australian pome fruit spring phenology. Int. J. Biometeorol. 57 (3), 409–421. doi: 10.1007/s00484-012-0567-1
Decourteix M., Alves G., Bonhomme M., Peuch M., Baaziz K. B., Brunel N., et al. (2008). Sucrose (JrSUT1) and hexose (JrHT1 and JrHT2) transporters in walnut xylem parenchyma cells: their potential role in early events of growth resumption. Tree Physiol. 28 (2), 215–224. doi: 10.1093/treephys/28.2.215
Department for Environment, Food and Rural Affairs (DEFRA), Department of Agriculture Environment and Rural Affairs (Northern Ireland), Welsh Assembly Government, T. D. for R. A. and H., The Scottish Government Rural and Environment Science and Analytical Services. (2017). Agriculture in the united kingdom 2017. Available at: https://www.gov.uk/government/statistics/agriculture-in-the-united-kingdom-2017.
Delgado A., Dapena E., Fernandez E., Luedeling E.. (2021a). Climatic requirements during dormancy in apple trees from northwestern Spain – global warming may threaten the cultivation of high-chill cultivars. Eur. J. Agron. 130, 126374. doi: 10.1016/j.eja.2021.126374
Delgado A., Egea J. A., Luedeling E., Dapena E.. (2021b). Agroclimatic requirements and phenological responses to climate change of local apple cultivars in northwestern Spain. Sci. Hortic. 283, 110093. doi: 10.1016/j.scienta.2021.110093
Dennis F. G. (2003). Problems in standardizing methods for evaluating the chilling requirements for the breaking of dormancy in buds of woody plants. HortScience 38 (3), 347–350. doi: 10.21273/HORTSCI.38.3.347
Devitt M. L., Stafstrom J. P. (1995). Cell cycle regulation during growth-dormancy cycles in pea axUlary buds. Plant Mol. Biol. 29, 255–265. doi: 10.1007/BF00043650
Díez-Palet I., Funes I., Savé R., Biel C., De Herralde F., Miarnau X., et al. (2019). Blooming under Mediterranean climate: estimating cultivar-specific chill and heat requirements of almond and apple trees using a statistical approach. Agronomy 9 (11), 760. doi: 10.3390/agronomy9110760
Doorenbos J. (1953). Review of the literature on dormancy in buds of woody plants. Mededelingen. van. Landbouwhogeschool. Wageningen/Nederland. 53 (1), 1–24.
Drepper B., Gobin A., Remy S., van Orshoven J. (2020). Comparing apple and pear phenology and model performance: what seven decades of observations reveal. Agronomy 10 (1), 73. doi: 10.3390/agronomy10010073
Egea J. A., Egea J., Ruiz D. (2021). Reducing the uncertainty on chilling requirements for endodormancy breaking of temperate fruits by data-based parameter estimation of the dynamic model: a test case in apricot. Tree Physiol. 41 (4), 644–656. doi: 10.1093/treephys/tpaa054
El-Agamy S. Z., Mohamed A. K. A., Mostafa F. M. A., Abdallah A. Y. (2000). Chilling and heat requirements for budbreak and fruiting of” anna” and” dorsett golden” apple cultivars under warm climatic conditions. VI. Int. Symposium. Temperate. Fruit Growing. Tropics. Subtropics. 565, 103–108. doi: 10.17660/ActaHortic.2001.565.16
Else M., Atkinson C. (2010). Climate change impacts on UK top and soft fruit production. Outlook. Agric. 39 (4), 257–262. doi: 10.5367/oa.2010.0014
El Yaacoubi A., Malagi G., Oukabli A., Citadin I., Hafidi M., Bonhomme M., et al. (2016). Differentiated dynamics of bud dormancy and growth in temperate fruit trees relating to bud phenology adaptation, the case of apple and almond trees. Int. J. Biometeorol. 60 (11), 1695–1710. doi: 10.1007/s00484-016-1160-9
El Yaacoubi A., El Jaouhari N., Bourioug M., El Youssfi L., Cherroud S., Bouabid R., et al. (2020). Potential vulnerability of Moroccan apple orchard to climate change–induced phenological perturbations: effects on yields and fruit quality. Int. J. Biometeorol. 64 (3), 377–387. doi: 10.1007/s00484-019-01821-y
Erez A. (2000). “Bud dormancy: a suggestion for the control mechanism and its evolution,” in Dormancy in plants: from whole plant behaviour to cellular control (Wallingford UK: CABI Publishing), 23–33.
Erez A., Couvillon G. A. (1987). Characterization of the influence of moderate temperatures on rest completion in peach. J. Am. Soc. For. Hortic. Sci. 112 (4), 677–680. doi: 10.21273/JASHS.112.4.677
Erez A., Couvillon G. A., Hendershott C. H. (1979). Quantitative chilling enhancement and negation in peach buds by high-temperatures in a daily cycle. J. Am. Soc. For. Hortic. Sci. 104 (4), 536–540. doi: 10.21273/JASHS.104.4.536
Erez A., Lavee S. (1971). The effect of climatic conditions on dormancy development of peach buds. i. temperature. J. Am. Soc. Hortic. Sci. 96 (6), 711–714. doi: 10.21273/JASHS.96.6.711
Fadón E., Fernandez E., Behn H., Luedeling E.. (2020a). A conceptual framework for winter dormancy in deciduous trees. Agronomy 10 (2), 241. doi: 10.3390/agronomy10020241
Fadón E., Herrera S., Guerrero B., Guerra M., Rodrigo J.. (2020b). Chilling and heat requirements of temperate stone fruit trees (Prunus sp.). Agronomy 10 (3), 409. doi: 10.3390/agronomy10030409
Fadón E., Herrero M., Rodrigo J. (2018). Dormant flower buds actively accumulate starch over winter in sweet cherry. Front. Plant Sci. 9 (February). doi: 10.3389/fpls.2018.00171
Fadón E., Rodrigo J. (2018). Unveiling winter dormancy through empirical experiments. Environ. Exp. Bot. 152, 28–36. doi: 10.1016/j.envexpbot.2017.11.006
Falavigna V., da S., Guitton B., Costes E., Andrés F.. (2019). I Want to (Bud) break free: the potential role of DAM and SVP-like genes in regulating dormancy cycle in temperate fruit trees. Front. Plant Sci. 9. doi: 10.3389/fpls.2018.01990
FAOSTAT. (2021). Crop production data. Available at: https://www.fao.org/faostat/en/#data/QCL (Accessed February 2023). Food and Agriculture Organization of the United Nations.
Faust M., Liu D., Millard M. M., Stutte G. W.. (1991). Bound versus free water in dormant apple buds–a theory for endodormancy. HortScience 26 (7), 887–890. doi: 10.21273/HORTSCI.26.7.887
Faust M., Erez A., Rowland L. J., Wang S. Y., Norman H. A.. (1997). Bud dormancy in perennial fruit trees: physiological basis for dormancy induction, maintenance, and release. HortScience 32 (4), 623–629. doi: 10.21273/HORTSCI.32.4.623
Fernandez E., Cuneo I. F., Luedeling E., Alvarado L., Farias D., Saa S.. (2019). Starch and hexoses concentrations as physiological markers in dormancy progression of sweet cherry twigs. Trees 33 (4), 1187–1201. doi: 10.1007/s00468-019-01855-0
Fernandez E., Krefting P., Kunz A., Do H., Fadón E., Luedeling E.. (2021). Boosting statistical delineation of chill and heat periods in temperate fruit trees through multi-environment observations. Agric. For. Meteorol. 310, 108652. doi: 10.1016/j.agrformet.2021.108652
Fernandez E., Luedeling E., Behrend D., Van de Vliet S., Kunz A., Fadón E. (2020). Mild water stress makes apple buds more likely to flower and more responsive to artificial forcing–impacts of an unusually warm and dry summer in Germany. Agronomy 10 (2), 274. doi: 10.3390/agronomy10020274
Finetto G. A. (2013). An investigation of chilling requirement of some scab resistant apple cultivars in po valley. IX. Int. Symposium. Temperate. Zone. Fruits. Tropics. Subtropics. 1059, 115–122. doi: 10.17660/ActaHortic.2014.1059.13
Finkelstein R. (2013). Abscisic acid synthesis and response. Arabidopsis. Book. 11, e0166. doi: 10.1199/tab.0166
Fishman S., Erez A., Couvillon G. A. (1987a). The temperature dependence of dormancy breaking in plants: mathematical analysis of a two-step model involving a cooperative transition. J. Theor. Biol. 124 (4), 473–483. doi: 10.1016/S0022-5193(87)80221-7
Fishman S., Erez A., Couvillon G. A. (1987b). The temperature dependence of dormancy breaking in plants: computer simulation of processes studied under controlled temperatures. J. Theor. Biol. 126 (3), 309–321. doi: 10.1016/S0022-5193(87)80237-0
Fleet C. M., Sun T. (2005). A DELLAcate balance: the role of gibberellin in plant morphogenesis. Curr. Opin. Plant Biol. 8 (1), 77–85. doi: 10.1016/j.pbi.2004.11.015
Foster T., Kirk C., Jones W. T., Allan A. C., Espley R., Karunairetnam S., et al. (2007). Characterisation of the DELLA subfamily in apple (Malus x domestica borkh.). Tree Genet. Genomes 3 (3), 187–197. doi: 10.1007/s11295-006-0047-z
Friml J., Palme K. (2002). “Polar auxin transport [[/amp]]mdash; old questions and new concepts?,” in C. perrot-rechenmann and g. Hagen (eds) auxin molecular biology (Dordrecht: Springer Netherlands), 273–284. doi: 10.1007/978-94-010-0377-3_2
Funes I., Aranda X., Biel C., Carbó J., Camps F., Molina A. J., et al. (2016). Future climate change impacts on apple flowering date in a Mediterranean subbasin. Agric. Water Manage. 164, 19–27. doi: 10.1016/j.agwat.2015.06.013
Garner W., Allard H. (1923). Further studies in photoperiodism, in response of the plant to relative lenght of day and night. J. Agric. Res. 23 (2), 871–920. doi: 10.1126/science.55.1431.582
Gonzalez Noguer C. (2022). Apple dormancy in a changing climate: development of chilling and heat accumulation models to predict bud break (University of Reading). Available at: https://centaur.reading.ac.uk/109868/.
Grant J. J., Loake G. J. (2000). Role of reactive oxygen intermediates and cognate redox signaling in disease resistance. Plant Physiol. 124 (1), 21–30. doi: 10.1104/pp.124.1.21
Griesbach J. (2007). Growing Temperate Fruit Trees in Kenya. World Agroforestry Centre, Nairobi. 128pp. Available at: https://apps.worldagroforestry.org/downloads/Publications/PDFS/b15496.pdf.
Guak S., Fuchigami L. H. (2001). Effects of applied ABA on growth cessation, bud dormancy, cold acclimation, leaf senescence and n mobilization in apple nursery plants. J. Hortic. Sci. Biotechnol. 76 (4), 459–464. doi: 10.1080/14620316.2001.11511394
Guak S., Neilsen D. (2013). Chill unit models for predicting dormancy completion of floral buds in apple and sweet cherry. Horticult. Environ. Biotechnol. 54 (1), 29–36. doi: 10.1007/s13580-013-0140-9
Guo L., Dai J., Ranjitkar S., Yu H., Luedeling E.. (2014). Chilling and heat requirements for flowering in temperate fruit trees. Int. J. Biometeorol. 58, 1195–1206. doi: 10.1007/s00484-013-0714-3
Guo L., Xu J., Dai J., Cheng J., Luedeling E.. (2015). Statistical identification of chilling and heat requirements for apricot flower buds in Beijing, China. Sci. Hortic. 195, 138–144. doi: 10.1016/j.scienta.2015.09.006
Gutierrez C., Ramirez-Parra E., Castellano M. M., del Pozo J.C.. (2002). G1 to s transition: more than a cell cycle engine switch. Curr. Opin. Plant Biol. 5 (6), 480–486. doi: 10.1016/S1369-5266(02)00301-1
Harrington C. A., Gould P. J., St.Clair J. B. (2010). Modeling the effects of winter environment on dormancy release of Douglas-fir. For. Ecol. Manage. 259 (4), 798–808. doi: 10.1016/j.foreco.2009.06.018
Hauagge R., Cummins J. N. (1991). ‘Phenotypic variation of lenght of bud dormancy in apple cultivars and related malus species’. J. Am. Soc. For. Hortic. Sci. 116 (1), 100–106. doi: 10.21273/JASHS.116.1.100
Hedden P., Sponsel V. (2015). A century of gibberellin research. J. Plant Growth Regul. 34 (4), 740–760. doi: 10.1007/s00344-015-9546-1
Heide O. M. (2003). High autumn temperature delays spring bud burst in boreal trees, counterbalancing the effect of climatic warming. Tree Physiol. 23 (13), 931–936. doi: 10.1093/treephys/23.13.931
Heide O. M., Prestrud A. K. (2005). Low temperature, but not photoperiod, controls growth cessation and dormancy induction and release in apple and pear. Tree Physiol. 25 (1), 109–114. doi: 10.1093/treephys/25.1.109
Horvath D. (2009). Common mechanisms regulate flowering and dormancy. Plant Sci. 177 (6), 523–531. doi: 10.1016/j.plantsci.2009.09.002
Horvath D. P., Anderson J. V., Chao W. S., Foley M.E.. (2003). Knowing when to grow: signals regulating bud dormancy. Trends Plant Sci. 8 (11), 534–540. doi: 10.1016/j.tplants.2003.09.013
Huang H., Ullah F., Zhou D.-X., Yi M., Zhao Y.. (2019). Mechanisms of ROS regulation of plant development and stress responses. Front. Plant Sci. 10. doi: 10.3389/fpls.2019.00800
Inamahoro M. (2020). Physiological dynamics of dormancy in apple buds grown in areas with insufficient winter chill. PhD thesis, Stellenbosch University. Available at: https://scholar.sun.ac.za/server/api/core/bitstreams/60317d12-db04-4d18-8e2b-4d3f756e5de8/content.
IPCC. (2022). Climate change 2022: impacts, adaptation, and vulnerability. contribution of working group II to the sixth assessment report of the intergovernmental panel on climate change. Eds. Pörtner H.-O., Roberts D. C., Tignor M., Poloczanska E. S., Mintenbeck K., Alegría A., Craig M., Langsdorf S., Löschke S., Möller V., Okem A., Rama B. (Cambridge University Press, Cambridge, UK and New York, NY, USA: Cambridge University Press), 3056. doi: 10.1017/9781009325844
Ito A., Sugiura T., Sakamoto D., Moriguchi T.. (2013). Effects of dormancy progression and low-temperature response on changes in the sorbitol concentration in xylem sap of Japanese pear during winter season. Tree Physiol. 33 (4), 398–408. doi: 10.1093/treephys/tpt021
Ito A., Sakamoto D., Moriguchi T. (2012). Carbohydrate metabolism and its possible roles in endodormancy transition in Japanese pear. Sci. Hortic. 144, 187–194. doi: 10.1016/j.scienta.2012.07.009
Jackson J. E. (2003). The Biology of Apples and Pears (The Biology of Horticultural Crops). Cambridge: Cambridge University Press. doi: 10.1017/CBO9780511542657
Jian L., Li P. H., Sun L., Chen T. H. H.. (1997). Alterations in ultrastructure and subcellular localization of Ca 2+ in poplar apical bud cells during the induction of dormancy. J. Exp. Bot. 48 (6), 1195–1207. doi: 10.1093/jxb/48.6.1195
Jones H. G., Hillis R. M., Gordon S. L., Brennan R.M.. (2013). An approach to the determination of winter chill requirements for different ribes cultivars. Plant Biol. 15 (SUPPL.1), 18–27. doi: 10.1111/j.1438-8677.2012.00590.x
Jonkers H. (1979). Biennial bearing in apple and pear: a literature survey. Sci. Hortic. 11, 303–317. doi: 10.1016/0304-4238(79)90015-3
Kaufmann H., Blanke M. (2017). Changes in carbohydrate levels and relative water content (RWC) to distinguish dormancy phases in sweet cherry. J. Plant Physiol. 218, 1–5. doi: 10.1016/j.jplph.2017.07.004
Kaukoranta T., Tahvonen R., Ylämäki A. (2010). Climatic potential and risks for apple growing by 2040. Agric. Food Sci. 19 (2), 144–159. doi: 10.2137/145960610791542352
Korban S. S., Skirvin R. M. (1984). Nomenclature of the cultivated apple. HortScience 19 (2), 177–180. doi: 10.21273/HORTSCI.19.2.177
Korösi Á., Markó V., Kovács-Hostyánszki A., Somay L., Varga Á., Elek Z., et al. (2018). Climate-induced phenological shift of apple trees has diverse effects on pollinators, herbivores and natural enemies. PeerJ 2018 (7), 1–21. doi: 10.7717/peerj.5269
Kumar A., Singh K. N., Lal B., Singh R.D.. (2008). Mapping of apple orchards using remote sensing techniques in cold desert of himachal pradesh, India. J. Indian Soc. Remote Sens. 36 (4), 387–392. doi: 10.1007/s12524-008-0038-7
Kuroda H., Sugiura T., Ito D. (2002). Changes in hydrogen peroxide content in flower buds of Japanese pear (Pyrus pyrifolia nakai) in relation to breaking of endodormancy. J. Japanese. Soc. Hortic. Sci. 71 (5), 610–616. doi: 10.2503/jjshs.71.610
Labuschagné I. F., Louw J. H., Schmidt K., Sadie A.. (2002). Genetic variation in chilling requirement in apple progeny. J. Am. Soc. Hortic. Sci. 127 (4), 663–672. doi: 10.21273/JASHS.127.4.663
Lang G. A., Early J. D., Martin G. C., Darnell R. L.. (1987). Endo-, para- and ecodormancy: physiological terminology and classification for dormancy research. Hortscience 22, 371–377. doi: 10.21273/HORTSCI.22.3.371
Legave J. M., Blanke M., Christen D., Giovannini D., Mathieu V., Oger R.. (2013). A comprehensive overview of the spatial and temporal variability of apple bud dormancy release and blooming phenology in Western Europe. Int. J. Biometeorol. 57 (2), 317–331. doi: 10.1007/s00484-012-0551-9
Lewak S. (2011). Metabolic control of embryonic dormancy in apple seed: seven decades of research. Acta Physiol. Plant. 33 (1), 1–24. doi: 10.1007/s11738-010-0524-8
Leyser O. (2003). Regulation of shoot branching by auxin. Trends Plant Sci. 8 (11), 541–545. doi: 10.1016/j.tplants.2003.09.008
Li J., Xu Y., Niu Q., He L., Teng Y., Bai S.. (2018a). Abscisic acid (ABA) promotes the induction and maintenance of pear (Pyrus pyrifolia white pear group) flower bud endodormancy. Int. J. Mol. Sci. 19 (1), 310. doi: 10.3390/ijms19010310
Li G., Tan M., Cheng F., Liu X., Qi S., Chen H., et al. (2018b). Molecular role of cytokinin in bud activation and outgrowth in apple branching based on transcriptomic analysis. Plant Mol. Biol. 98 (3), 261–274. doi: 10.1007/s11103-018-0781-2
Liu J., Sherif S. M. (2019). Hormonal orchestration of bud dormancy cycle in deciduous woody perennials. Front. Plant Sci. 10. doi: 10.3389/fpls.2019.01136
Loescher W. H., McCamant T., Keller J. D. (1990). Carbohydrate reserves, translocation, and storage in woody plant roots. HortScience 25 (3), 274–281. doi: 10.21273/HORTSCI.25.3.274
Louw E., Allderman L., Cook N. (2023). What is wrong with winter chill models in warm climates? HortScience 58 (5), 550–556. doi: 10.21273/HORTSCI16972-22
Luedeling E. (2012). Climate change impacts on winter chill for temperate fruit and nut production: a review. Sci. Hortic. 144, 218–229. doi: 10.1016/j.scienta.2012.07.011
Luedeling E., Brown P. H. (2011). A global analysis of the comparability of winter chill models for fruit and nut trees. Int. J. Biometeorol. 55 (3), 411–421. doi: 10.1007/s00484-010-0352-y
Luedeling E., Girvetz E. H., Semenov M. A., Brown P. H.. (2011). Climate change affects winter chill for temperate fruit and nut trees. PloS One 6 (5), e20155. doi: 10.1371/journal.pone.0020155
Luedeling E., Schiffers K., Fohrmann T., Urbach C.. (2021). PhenoFlex - an integrated model to predict spring phenology in temperate fruit trees. Agric. For. Meteorol. 307, 108491. doi: 10.1016/j.agrformet.2021.108491
Luedeling E., Gassner A. (2012). Partial least squares regression for analyzing walnut phenology in California. Agric. For. Meteorol. 158–159, 43–52. doi: 10.1016/j.agrformet.2011.10.020
Luedeling E., Kunz A., Blanke M. M. (2013). Identification of chilling and heat requirements of cherry trees-a statistical approach. Int. J. Biometeorol. 57 (5), 679–689. doi: 10.1007/s00484-012-0594-y
Luedeling E., Zhang M., Girvetz E. H. (2009). ‘Climatic changes lead to declining winter chill for fruit and nut trees in California during 1950–2099’. PloS One 4 (7), e6166. doi: 10.1371/journal.pone.0006166
Malagi G., Sachet M. R., Citadin I., Herter F. G., Bonhomme M., Regnard J. L., et al. (2015). The comparison of dormancy dynamics in apple trees grown under temperate and mild winter climates imposes a renewal of classical approaches. Trees 29 (5), 1365–1380. doi: 10.1007/s00468-015-1214-3
Mankotia M. S., Chauhan P. S., Sud A., Jindal K. K. (2004). Estimation of effective chilling hours and GDH degrees c requirement and its significance in predicting full bloom in delicious apple. Acta Hortic. 662, 83–86. doi: 10.17660/ActaHortic.2004.662.8
Michalczuk L. (2005). Hormonal control of dormancy. Int. J. Fruit Sci. 5 (1), 59–73. doi: 10.1300/J492v05n01_06
Mimida N., Saito T., Moriguchi T., Suzuki A., Komori S., Wada M.. (2015). Expression of DORMANCY-ASSOCIATED MADS-BOX (DAM)-like genes in apple. Biol. Plant. 59 (2), 237–244. doi: 10.1007/s10535-015-0503-4
Miotto Y. E., Tessele C., Czermainski A. B. C., Porto D. D., Falavigna da V. S., Sartor T., et al. (2019). Spring is coming: genetic analyses of the bud break date locus reveal candidate genes from the cold perception pathway to dormancy release in apple (Malus × domestica borkh.). Front. Plant Sci. 10. doi: 10.3389/fpls.2019.00033
Mostafa E. A. M., Saleh M. M. S. (2006). Influence of spraying with gibberellic acid on behaviour of Anna apple trees. J. Appl. Sci. Res. 2 (8), 477–486.
Mutasa-Gottgens E., Hedden P. (2009). Gibberellin as a factor in floral regulatory networks. J. Exp. Bot. 60 (7), 1979–1989. doi: 10.1093/jxb/erp040
Naor A., Flaishman M., Stern R., Moshe A., Erez A.. (2003). Temperature effects on dormancy completion of vegetative buds in apple. J. Am. Soc. Hortic. Sci. 128 (5), 636–641. doi: 10.21273/JASHS.128.5.0636
Naor A., Naschitz S., Peres M., Gal Y.. (2008). Responses of apple fruit size to tree water status and crop load. Tree Physiol. 28 (8), 1255–1261. doi: 10.1093/treephys/28.8.1255
Olsen J. E., Junttila O., Moritz T. (1995). A localised decrease of GA1in shoot tips of salix pentandra seedings precedes cessation of shoot elongation under short photoperiod. Physiol. Plant. 95 (4), 627–632. doi: 10.1111/j.1399-3054.1995.tb05532.x
Parkes H., Darbyshire R., White N. (2020). Chilling requirements of apple cultivars grown in mild Australian winter conditions. Sci. Hortic. 260 (September 2019), 108858. doi: 10.1016/j.scienta.2019.108858
Pérez F. J., Burgos B. (2004). Alterations in the pattern of peroxidase isoenzymes and transient increases in its activity and in h 2 O 2 levels take place during the dormancy cycle of grapevine buds: the effect of hydrogen cyanamide. Plant Growth Regul. 43 (3), 213–220. doi: 10.1023/B:GROW.0000046003.33194.ac
Pérez F. J., Lira W. (2005). Possible role of catalase in post-dormancy bud break in grapevines. J. Plant Physiol. 162 (3), 301–308. doi: 10.1016/j.jplph.2004.07.011
Petri J. L., Leite G. B. (2004). Consequences of insufficient winter chilling on apple tree bud-break. Acta Hortic. 662 (1), 53–60. doi: 10.17660/ActaHortic.2004.662.4
Pope K. S., Da Silva D., Brown P. H., DeJong T. M.. (2014). A biologically based approach to modeling spring phenology in temperate deciduous trees. Agric. For. Meteorol. 198–199, 15–23. doi: 10.1016/j.agrformet.2014.07.009
Porto D. D., Bruneau M., Perini P., Anzanello R., Renou J. P., Dos Santos H. P., et al. (2015). Transcription profiling of the chilling requirement for bud break in apples: a putative role for FLC-like genes. J. Exp. Bot. 66 (9), 2659–2672. doi: 10.1093/jxb/erv061
Porto D. D., da Silveira Falavigna V., Arenhart R. A., Perini P., Buffon V., Anzanello R., et al. (2016). Structural genomics and transcriptional characterization of the dormancy-associated MADS-box genes during bud dormancy progression in apple. Tree Genet. Genomes 12 (3), 46. doi: 10.1007/s11295-016-1001-3
Pratt C. (1988). Apple flower and fruit: morphology and anatomy. Hortic. Rev. 10, 273–308. doi: 10.1016/S0304-4238(96)00958-2
Raese J. T., Williams M. W., Billingsley H. D. (1977). Sorbitol and other carbohydrates in dormant apple shoots as influenced by controlled temperatures. Cryobiology 14 (3), 373–378. doi: 10.1016/0011-2240(77)90185-7
Ramírez F., Davenport T. L. (2013). Apple pollination: a review. Sci. Hortic. 162, 188–203. doi: 10.1016/j.scienta.2013.08.007
Richardson E. A., Seeley S. D., Walker D. R. (1974). A model for estimating the completion of rest for “Redhaven” and “Elberta” peach trees. HortScience 9 (4), 331–332. doi: 10.21273/HORTSCI.9.4.331
Rinne P. L. H., Welling A., Vahala J., Ripel L., Ruonala R., Kangasjärvi J., et al. (2011). Chilling of dormant buds hyperinduces FLOWERING LOCUS T and recruits GA-inducible 1,3-β-Glucanases to reopen signal conduits and release dormancy in populus. Plant Cell 23 (1), 130–146. doi: 10.1105/tpc.110.081307
Rinne P. L. H., Kaikuranta P. M., van der Schoot C. (2001). The shoot apical meristem restores its symplasmic organization during chilling-induced release from dormancy: chilled AM restores its symplasmic network. Plant J. 26 (3), 249–264. doi: 10.1046/j.1365-313X.2001.01022.x
Rinne P., Saarelainen A., Junttila O. (1994). Growth cessation and bud dormancy in relation to ABA level in seedlings and coppice shoots of betula pubescens as affected by a short photoperiod, water stress and chilling. Physiol. Plant. 90 (3), 451–458. doi: 10.1111/j.1399-3054.1994.tb08801.x
Rohde A., Bhalerao R. P. (2007). Plant dormancy in the perennial context. Trends Plant Sci. 12 (5), 217–223. doi: 10.1016/j.tplants.2007.03.012
Rohde A., Storme V., Jorge V., Gaudet M., Vitacolonna N., Rohde A., et al. (2011). Bud set in poplar – genetic dissection of a complex trait in natural and hybrid populations. New Phytol. 189, 106–121. doi: 10.1111/j.1469-8137.2010.03469.x
Ruiz D., Campoy J. A., Egea J. (2007). Chilling and heat requirements of apricot cultivars for flowering. Environ. Exp. Bot. 61 (3), 254–263. doi: 10.1016/j.envexpbot.2007.06.008
Ruonala R., Rinne P. L. H., Baghour M., Moritz T., Tuominen H., Kangasjärvi J.. (2006). Transitions in the functioning of the shoot apical meristem in birch (Betula pendula) involve ethylene. Plant J. 46 (4), 628–640. doi: 10.1111/j.1365-313X.2006.02722.x
Ruttink T., Arend M., Morreel K., Storme V., Rombauts S., Fromm J., et al. (2007). A molecular timetable for apical bud formation and dormancy induction in poplar. Plant Cell 19 (8), 2370–2390. doi: 10.1105/tpc.107.052811
Samish R. M. (1954). Dormancy in woody plants’. Annu. Rev. Plant Physiol. 5, 183–204. doi: 10.1146/annurev.pp.05.060154.001151
Sapkota S., Liu J., Islam M. T., Ravindran P., Kumar P. P., Sherif S. M.. (2021a). Contrasting bloom dates in two apple cultivars linked to differential levels of phytohormones and heat requirements during ecodormancy. Sci. Hortic. 288, 110413. doi: 10.1016/j.scienta.2021.110413
Sapkota S., Liu J., Islam M. T., Sherif S. M. (2021b). Changes in reactive oxygen species, antioxidants and carbohydrate metabolism in relation to dormancy transition and bud break in apple (Malus × domestica borkh) cultivars. Antioxidants 10 (10), 1549. doi: 10.3390/antiox10101549
Saure M. C. (1985). Dormancy release in deciduous fruit trees. Hortic. Rev. 7, 239–300. doi: 10.1002/9781119281269
Sauter J. J., Wisniewski M., Witt W. (1996). Interrelationships between ultrastructure, sugar levels, and frost hardiness of ray parenchyma cells during frost acclimation and deacclimation in poplar (Populus × canadensis moench ‘robusta’) wood. J. Plant Physiol. 149 (3–4), 451–461. doi: 10.1016/S0176-1617(96)80148-9
Seif El-Yazal M. A., Seif El-Yazal S. A., Rady M. M. (2014). Exogenous dormancy-breaking substances positively change endogenous phytohormones and amino acids during dormancy release in “Anna” apple trees. Plant Growth Regul. 72 (3), 211–220. doi: 10.1007/s10725-013-9852-1
Severino V., Gravina A., Manzi M., Arias M. (2007). Models for quantifying effective winter chill on apple endodormancy. VIII. Int. Symposium. Temperate. Zone. Fruits. Tropics. Subtropics. 872, 113–120. doi: 10.17660/ActaHortic.2010.872.1
Shaltout A. D., Unrath C. R. (1983). Rest completion prediction model for cultivar starkrimson delicious apples malus domestica. J. Am. Soc. Hortic. Sci. 108 (6), 957–961. doi: 10.21273/JASHS.108.6.957
Siller-Cepeda J. H., Fuchigami L. H., Chen T. H. H. (2019). Hydrogen cyanamide-induced budbreak and phytotoxicity in `Redhaven’ peach buds. HortScience 27 (8), 874–876. doi: 10.21273/hortsci.27.8.874
Sivaci A. (2006). Seasonal changes of total carbohydrate contents in three varieties of apple (Malus sylvestris miller) stem cuttings. Sci. Hortic. 109 (3), 234–237. doi: 10.1016/j.scienta.2006.04.012
Smalle J., Straeten D. (1997). Ethylene and vegetative development. Physiol. Plant. 100 (3), 593–605. doi: 10.1111/j.1399-3054.1997.tb03065.x
Tabuenca M. C. (1964). Necesidades de frio invernal de variedades de albaricoquero, melocotonero y peral – Available at: http://hdl.handle.net/10261/36040.
Takemura Y., Kuroki K., Jiang M., Matsumoto K., Tamura F.. (2015). Identification of the expressed protein and the impact of change in ascorbate peroxidase activity related to endodormancy breaking in pyrus pyrifolia. Plant Physiol. Biochem. 86, 121–129. doi: 10.1016/j.plaphy.2014.11.016
Tamura F., Tanabe K., Itai A. (2002). Regulation of endodormancy in japanese pear. Acta Hortic. 587), 325–336. doi: 10.17660/ActaHortic.2002.587.44
Tan M., Li G., Qi S., Liu X., Chen X., Ma J., et al. (2018). Identification and expression analysis of the IPT and CKX gene families during axillary bud outgrowth in apple (Malus domestica borkh.). Gene 651, 106–117. doi: 10.1016/j.gene.2018.01.101
Tan M., Li G., Chen X., Xing L., Ma J., Zhang D., et al. (2019). Role of cytokinin, strigolactone, and auxin export on outgrowth of axillary buds in apple. Front. Plant Sci. 10. doi: 10.3389/fpls.2019.00616
Thompson W. K., Jones D. L., Nichols D. G. (1975). Effects of dormancy factors on the growth of vegetative buds of young apple trees. Aust. J. Agric. Res. 26 (6), 989–996. doi: 10.1071/AR9750989
Tixier A., Sperling O., Orozco J., Lampinen B., Amico Roxas A., Saa S., et al. (2017). Spring bud growth depends on sugar delivery by xylem and water recirculation by phloem münch flow in juglans regia. Planta 246 (3), 495–508. doi: 10.1007/s00425-017-2707-7
Tromp J. (1982). Flower-bud formation in apple as affected by various gibberellins. J. Hortic. Sci. 57 (3), 277–282. doi: 10.1080/00221589.1982.11515053
Tylewicz S., Petterle A., Marttila S., Miskolczi P., Azeez A., Singh R. K., et al. (2018). Photoperiodic control of seasonal growth is mediated by ABA acting on cell-cell communication. Science 360 (6385), 212–215. doi: 10.1126/science.aan8576
Vegis A. (1964). Dormancy in higher plants. Annu. Rev. Ecol. Syst. 15, 185–224. doi: 10.1146/annurev.pp.15.060164.001153
Wang S. Y., Faust M. (1990). Changes of membrane lipids in apple buds during dormancy and budbreak. J. Am. Soc. Hortic. Sci. 115 (5), 803–808. doi: 10.21273/JASHS.115.5.803
Watkins R. (1984). Apple genetic resources. Conference on Temperate Tree Fruits and Nuts Breeding 159, 21–30.
Webster A. D. (1995). Rootstock and interstock effects on deciduous fruit tree vigour, precocity, and yield productivity. New Z. J. Crop Hortic. Sci. 23 (4), 373–382. doi: 10.1080/01140671.1995.9513913
Weinberger J. H. (1950). Chilling requirements of peach varieties. Proc. Am. Soc. Hortic. Sci. 56, 122–128.
Wen L. H., Zhong W. J., Huo X. M., Zhuang W. B., Ni Z. J., Gao Z. H., et al. (2016). Expression analysis of ABA- and GA-related genes during four stages of bud dormancy in Japanese apricot (Prunus mume sieb. et zucc). J. Hortic. Sci. Biotechnol. 91 (4), 362–369. doi: 10.1080/14620316.2016.1160546
Wilkie J. D., Sedgley M., Olesen T. (2008). Regulation of floral initiation in horticultural trees. J. Exp. Bot. 59 (12), 3215–3228. doi: 10.1093/jxb/ern188
Wisniewski M., Norelli J., Bassett C., Artlip T., Macarisin D.. (2011). Ectopic expression of a novel peach (Prunus persica) CBF transcription factor in apple (Malus × domestica) results in short-day induced dormancy and increased cold hardiness. Planta 233 (5), 971–983. doi: 10.1007/s00425-011-1358-3
Wisniewski M., Norelli J., Artlip T. (2015). Overexpression of a peach CBF gene in apple: a model for understanding the integration of growth, dormancy, and cold hardiness in woody plants. Front. Plant Sci. 6. doi: 10.3389/fpls.2015.00085
Wu R., Tomes S., Karunairetnam S., Tustin S. D., Hellens R. P., Allan A. C., et al. (2017). SVP-like MADS box genes control dormancy and budbreak in apple. Front. Plant Sci. 08 (April). doi: 10.3389/fpls.2017.00477
Wu R., Cooney J., Tomes S., Rebstock R., Karunairetnam S., Allan A. C., et al. (2021). RNAi-mediated repression of dormancy-related genes results in evergrowing apple trees. Tree Physiol. 41 (8), 1510–1523. doi: 10.1093/treephys/tpab007
Yamane H., Wada M., Honda C., Matsuura T., Ikeda Y., Hirayama T., et al. (2019). ‘Overexpression of prunus DAM6 inhibits growth, represses bud break competency of dormant buds and delays bud outgrowth in apple plants’. PloS One 14 (4), e0214788. doi: 10.1371/journal.pone.0214788
Yang Q., Gao Y., Wu X., Moriguchi T., Bai S., Teng Y.. (2021). Bud endodormancy in deciduous fruit trees: advances and prospects. Horticult. Res. 8 (1), 139. doi: 10.1038/s41438-021-00575-2
Young E., Werner D. J. (1985). Chill unit and growing degree hour requirements for vegetative bud break in six apple rootstocks. J. Am. Soc. Hortic. Sci. 110 (3), 411–413. doi: 10.21273/JASHS.110.3.411
Zhang S., Zhang D., Fan S., Du L., Shen Y., Xing L., et al. (2016). Effect of exogenous GA 3 and its inhibitor paclobutrazol on floral formation, endogenous hormones, and flowering-associated genes in “Fuji” apple (Malus domestica borkh.). Plant Physiol. Biochem. 107, 178–186. doi: 10.1016/j.plaphy.2016.06.005
Zhang J., Taylor C. (2011). The dynamic model provides the best description of the chill process on “Sirora” pistachio trees in Australia. HortScience 46 (3), 420–425. doi: 10.21273/HORTSCI.46.3.420
Zhuang W., Gao Z., Wang L., Zhong W., Ni Z., Zhang Z.. (2013). Comparative proteomic and transcriptomic approaches to address the active role of GA4 in Japanese apricot flower bud dormancy release. J. Exp. Bot. 64 (16), 4953–4966. doi: 10.1093/jxb/ert284
Keywords: chilling requirements, heat requirements, endodormancy, ecodormancy, phytohormones, DAM genes, climate change
Citation: González Noguer C, Delgado A, Else M and Hadley P (2023) Apple (Malus × domestica Borkh.) dormancy – a review of regulatory mechanisms and agroclimatic requirements. Front. Hortic. 2:1217689. doi: 10.3389/fhort.2023.1217689
Received: 05 May 2023; Accepted: 30 June 2023;
Published: 20 July 2023.
Edited by:
Vicente Vives-Peris, University of Jaume I, SpainReviewed by:
Rita Lourenço Costa, Instituto Nacional Investigaciao Agraria e Veterinaria (INIAV), PortugalAlberto Continella, University of Catania, Italy
Copyright © 2023 González Noguer, Delgado, Else and Hadley. This is an open-access article distributed under the terms of the Creative Commons Attribution License (CC BY). The use, distribution or reproduction in other forums is permitted, provided the original author(s) and the copyright owner(s) are credited and that the original publication in this journal is cited, in accordance with accepted academic practice. No use, distribution or reproduction is permitted which does not comply with these terms.
*Correspondence: Carlota González Noguer, Y2FybG90YS5nb256YWxlem5vZ3VlckBuaWFiLmNvbQ==