- 1Mt Albert Research Centre, The New Zealand Institute for Plant and Food Research Limited, Auckland, New Zealand
- 2Havelock North Research Centre, The New Zealand Institute for Plant and Food Research Limited, Havelock North, New Zealand
Bull’s eye rot, caused by Phlyctema vagabunda, is an important postharvest rot of apples. A combination of laboratory and field trials were conducted to examine the relationship between infection and status of apple lenticels. Fruit were hydrated and then inoculated, and climatic factors were compared with inoculations and natural infections in the field. In laboratory trials it was shown that increased lenticel density and size led to more infections. Hydration distended lenticel diameter, and dehydration contracted lenticels. A strong relationship with wind run, leaf wetness and temperature were shown to be important for laboratory inoculations of monthly harvested fruit, natural infections in trap fruit experiments, and monthly field inoculations. It was hypothesised that high wind run (> c. 240 km/day) reduced fruit susceptibility by promoting lenticel closure, and when lenticels were open, temperature limited the formation of an infection stroma. If the stroma did not form, postharvest expression of disease was negligible. Leaf wetness over 28 days was also an important factor for infection, probably because of the effect on micro-crack formation and resealing of lenticels during maturation by components of the cuticle. From these results, pre-harvest application of compounds that close lenticels could provide some control of bull’s eye rot without the need for fungicides.
1 Introduction
Bull’s eye rot (BER) is a significant postharvest disease affecting apples, which causes economic losses around the globe (Spadaro et al., 2020). This disease is caused by several closely related fungal species, of which Phlyctema vagabunda (syn. Neofabraea alba, Neofabraea vagabunda) (Chen et al., 2016) is the most common in New Zealand (Johnston et al., 2005; Everett et al., 2022). P. vagabunda is the main causal organism in continental Europe (Spadaro et al., 2020), and has also been reported from North America (Gariepy et al., 2005), Australia (Cunnington, 2004), Chile (Henriquez, 2005), Japan (Sato et al., 2021), and South Africa (Den Breeyen et al., 2020). BER is symptomless until fruit have been cold stored for c. 12 weeks (Everett et al., 2021), after which circular spots appear, originating from lenticels on the sides of the fruit and around the stem, calyx, and wounds (Spotts, 2014).
Infections occur in the field throughout the year, but most occur towards the end of the season, and conidia penetrate through the lenticels in the sides of the fruit, through microcracks, wounds, or the stem end (Spadaro et al., 2020). The infection process is not well understood, although it has been noted that rain facilitates infections by releasing conidia rather than providing humidity or wetness for spore germination (Henriquez et al., 2008). It was found that the application of simulated rain to freshly harvested fruit artificially inoculated with P. vagabunda conidia increased the number of infections (Edney et al., 1977), but the mechanism by which it did so was unclear.
For Neofabraea perennans and Neofabraea malicorticis, which are not present in New Zealand (Everett et al., 2022), Grove (1990) stated that N. malicorticis is more common in the moist western coastal regions of the Pacific Northwest of North America, whereas N. perennans is more common in the interior plains, where winters are more severe, and summers are hot and dry. Both cause cankers in the trunks of trees, but N. malicorticis is a primary pathogen that attacks healthy bark to cause cankers, which are characterised by fiddle strings, or bast fibres, which are exposed after the rest of the tissue disintegrates (Creemers, 2014). These cankers rarely grow beyond the first season and new cankers form in autumn. Sporulation takes place for several years after the cessation of canker expansion, and occurs in cool, moist conditions. In contrast, N. perennans causes perennial cankers that are eventually surrounded by annual callus growth rings (Creemers, 2014). N. perennans is a wound parasite and will penetrate host tissue through pruning wounds, frost cracks, or woolly aphid injuries. Spores are produced throughout the year, and their production peaks during autumn and winter, depending on the climate. The fungus overwinters in infected fruit remaining in the orchard after harvest and in cankers. The infection of fruit can occur 1 month after harvest, and susceptibility increases during the season. Conidia penetrate through lenticels and form appressoria, which become latent until after 3 months of cold storage, that is when the mycelium penetrates further into the fruit flesh to cause rot (Edney, 1958; Dugan et al., 1993a).
Apple lenticels are formed from stomata that are present on young fruit (Clements, 1935; Khanal et al., 2020). During expansion growth, tangential stress causes the stomata to shear, as they are unable to undergo periclinal cell divisions (Ruess and Stosser, 1993). The consequent wound response results in a periderm layer of corky cells that fill and seal the ruptured wound (Clements, 1935; Meyer, 1944; Esau, 1977; Ruess and Stosser, 1993). Thus, apple fruit lenticels are irregularly shaped, and can differ in both colour (from white to tan) and size (from minute spots to those up to 2–3 mm in diameter). Apple lenticels act as conduits for water and gas diffusion between the loosely stacked corky cells (Khanal et al., 2020).
In this study, the detached fruit were examined to explore the effect of hydration on lenticel morphology and susceptibility to BER. The influence of climatic factors on BER and P. vagabunda in the laboratory and in the field was also examined.
2 Materials and methods
2.1 Preparation of P. vagabunda conidia
P. vagabunda was grown on Difco® potato dextrose agar (PDA) for 14 days. An agar plug (with a 5-mm diameter) was then collected, comminuted on a Petri plate lid with a sterile bent glass rod, and spread onto Difco corn meal agar (CMA). After 7–10 days of growth at 20°C under lights (12 h:12 h day : night), conidia were harvested, placed in sterile deionised water, and adjusted to the desired concentration for inoculation (Everett et al., 2017).
2.2 Source of the apple fruit
The apple fruit were obtained from the Plant & Food Research (PFR) Havelock North Research Centre orchard (39°39′13.53″S, 176°52′33.71″E) or an organic apple orchard nearby (1.84 km distant). The cultivars used in this study were ‘Scired’ (marketed as Pacific Queen®), ‘Scifresh’ (marketed as Jazz®), and ‘Sciros’ (marketed as Pacific Rose®). The apple fruit were sprayed with 70% ethanol and then dried on a paper towel before laboratory experiments were conducted.
2.3 Peel inoculation
A thin slice of peel was removed from apples and washed with 70% ethanol. Peel segments measuring approximately 1 cm × 4 cm were removed with a sterile razor blade from four parts of the ‘Scired’ cultivar apple fruit obtained from an organic apple orchard near Havelock North, Hawke’s Bay, namely the sun-exposed, shady-cheeks, top, and bottom parts. The peels from six apples were placed skin-side down on 10 µL of a spore suspension (107 spores/mL) on CMA in a Petri plate. Each of the six Petri plates contained four slices taken from the sun-exposed, shady-cheeks, top, and bottom parts of a single replicate apple. The Petri plates were sealed with Parafilm™, then placed in the incubator at 20°C. A section of one slice from each apple replicate was removed after 6, 12, 24, 48, and 72 h, and samples were cut in half. Half of the sample was placed on PDA, and the other was placed in fixative for microscopic examination. Any resultant fungal colonies were recorded.
The number of lenticels per cm2 was counted for 12 replicate slices (three slices from each of the four apples) from each of the four parts of the apple (i.e., sun-exposed, shady-cheeks, top, and bottom). The experiment was conducted twice.
2.4 Spore germination on peel
Spore germination was tested on excised apple skin removed from the sun-exposed cheek of ‘Scired’ apples on an aluminium temperature gradient plate (Everett, 2003), with temperatures ranging from 0°C to 30°C. The apple skin peels (1 cm× 2 cm) were removed with a sterile razor blade and placed on glass microscope slides with the skin side facing up, after which 100 μL of 1 × 103 spores/mL of P. vagabunda was aliquoted onto them. The spore suspensions were covered with a glass cover slide, and the slide was then transferred to the gradient plate. There were two replicate apple skin portions per each of the 14 places on the gradient plate in accordance with the method described in the study by Everett (2003). The spore germination was assessed by counting four replicates of 25 spores (two from each replicate skin piece) after 6, 12, 24 and 48 h. To prevent further spore germination, 100 μL of lactophenol (20 g of phenol, 20 mL of lactic acid, and 40 mL of glycerol) containing 0.5% cotton blue was placed under each cover slide when removed from the gradient plate.
2.5 Detached fruit inoculations
A 100-µL aliquot of 106 conidia/mL was placed in the centre of a Petri plate containing CMA. The sides of whole ‘Scired’ apple fruit were secured with masking tape to the Petri plate containing inoculum, enclosed in a plastic bag with a wet paper towel, then placed in an incubator at 20°C, in accordance with the method reported by Everett et al. (2017). Six replicate apples were removed after 6, 12, 24, 48 and 72 h. Six fruit received the same treatment, except that they were placed on CMA without inoculum, and these fruit were removed after 72 h. Immediately after removal from the incubator and the Petri plates, the fruit were placed on cardboard P70 packing trays (six fruit per layer) in apple boxes and placed in the cold store at 0.5°C ± 0.5°C with the inoculated side facing up. The fruit were visually assessed for BER symptoms after 6, 12, 16, and 20 weeks. Any lenticels showing dark staining were cut in half, with half being placed in fixative and half being surface sterilised (2 min in 1 : 3 sodium hypochlorite) and placed on PDA. Any resultant fungal colonies were identified morphologically and P. vagabunda were recorded. The half placed in fixative was not able to be examined with microscopy due to complete rotting of the tissue.
2.6 Monthly detached fruit inoculations
Comparisons of detached fruit inoculations from different years without wounding were made using the methodology described in Section 2.5. The ‘Scired’ apples were placed in incubators set at 20°C. The fruit were picked from an organic apple orchard near Havelock North, Hawke’s Bay, on or near the 30th of the month from January to April in 2018 and 2019, then placed in a cold store (0.5°C ± 0.5°C) until inoculated. In 2018, the monthly samples were inoculated on 5 March, 12 March, 10 April, and 6 May, within 1 month of harvest. The processing times were delayed in 2019 and harvesting took place on 16 April, 30 April, 7 May and 13 May, or a maximum of 2.5 months after harvest. In 2021, fruit were harvested on 22 January, 19 February, 16 March, and 7 April, and were inoculated within 6 days of each harvest.
2.7 Inoculation of hydrated detached fruit
Three hydrated and three non-hydrated ‘Scired’ fruit, which had been harvested from an organic apple orchard on 28 March 2018, were inoculated with P. vagabunda by placing the fruit cheek on a 100-µL droplet containing 106 conidia/mL on Difco CMA in Petri plates for 72 h on 23 June 2020. A further three hydrated and three non-hydrated fruit were placed on CMA for 72 h without P. vagabunda (uninoculated controls). The remaining three fruit were untreated. The fruit were weighed after treatment. Three hydrated and three non-hydrated fruit were destructively sampled so that the lenticel diameter could be determined immediately after they were soaked in water for 24 h. The remaining fruit were assessed after 20 weeks in the cold store at 0.5°C ± 0.5°C.
Every month, starting on 20 January 2021, 30 ‘Scired’ apple fruit were harvested from the same row on an organic apple orchard near Havelock North, Hawke’s Bay on 17 February and 17 March, and an extra 15 fruit were harvested on 14 April 2021. The apples were treated within 1 week of harvest after courier transport to the Mt Albert Research Centre of The New Zealand Institute for Plant & Food Research Limited (PFR). Following receipt, 12 fruit were submerged in water (hydrated), and 18 were left on the bench. All treatments took place overnight (from 5 p.m. to 9 a.m. the following day).
In April, a further five fruit were placed in a laminar flow overnight (from 5 p.m. to 9 a.m. the following day) to examine the effect of dehydration on lenticel diameter. An extra five fruit were hydrated, and five were left as untreated controls. These five fruit per treatment (dehydrated, hydrated, and untreated controls) were sampled by the removal of thin skin sections (one slice from the sun-exposed cheek of each apple) with a razor blade and the sample mounted in lactophenol on a microscope slide. Lenticels were counted and measured using an eyepiece micrometer in a Leica DMLS light microscope.
2.8 Sample preparation for microscopy
Samples from the peel inoculation experiment (Section 2.3) were fixed in 2% paraformaldehyde and 2.5% glutaraldehyde in 0.1 M phosphate buffer at pH 7.2 under vacuum for 1 h. Samples were then washed in buffer, dehydrated in an ethanol series and embedded in LR White resin. One-µm-thick sections were dried onto poly-L-lysine-coated slides and stained in a solution of toluidine blue in 1% aqueous sodium carbonate (pH 4.4). Sections were viewed on an Olympus Vanox AHBT3 compound microscope.
2.9 Field inoculations
The ‘Scifresh’ cultivar apple trees at PFR, Havelock North orchard were inoculated in the field in accordance with the method described in the study by Everett et al. (2018), except that some fruit were not wounded. In 2015, five trees within a row were selected. From each of these trees, five fruit were treated. In 2016, 10 trees within a row were selected and three fruit per tree were treated. Immediately prior to treatment, half of the apple fruit were wounded with a pin sterilised with 95% ethanol to a depth of 5 mm, and half were not wounded. Half of the wounded and half of the unwounded apple fruit were spray inoculated using a Devibiliss® sprayer (0.25 mL/fruit) containing 105 conidia/mL of P. vagabunda strain KE 171 in 2014–2015, and with an equal mixture of KE 127, 171, 183 and 892 in 2015–2016. Water controls, both of those that had been subjected and not subjected to wounding treatments, were also included. The treatments were applied monthly (on 20 November and 23 December 2014; 16 January, 20 February, 27 March, 28 November, and 24 December 2015; and 27 January, 29 February, and 30 March 2016). At each inoculation time, treated apple fruit were enclosed in a sealed plastic bag and covered with aluminium foil. Both were removed after 48 h. The fruit were harvested 4 days after the final treatment.
2.10 Inoculum exclusion
Fifteen trees within a row on an organic orchard near PFR Havelock North were selected. From each of these trees, seven ‘Sciros’ cultivar fruit were treated. Except for the unbagged controls, Chinese fruit bags (150 mm × 180 mm; 100x Pulp Paper Bags Fruits Protection Pouch Orchard Against Insect Bird Hot, Fruugo NZ1) were placed on all treated fruit on 20 December 2014 after the fruit set was complete. Apples were unbagged at monthly intervals to allow natural infections and then re-bagged for a 1-month window of exposure. One set of fruit was bagged throughout the season, and one set was not. The fruit were unbagged between 20 December 2014 and 19 January 2015, 19 January 2015 and 23 February 2015, 23 February 2015 and 23 March 2015, and 23 March 2015 and 14 April 2015 (Table 1). The fruit were harvested on 14 April 2015 and placed on cardboard Friday trays (containing pockets for individual apple fruit to prevent damage during transport and storage) in apple boxes. The incidence of BER was visually assessed after 24 weeks of cold storage at 0.5°C ± 0.5°C.
2.11 Statistical analysis
Data were analysed using Minitab® 18.1. Statistical software (Minitab Ltd. Coventry CV3 2TE, United Kingdom) and R 4.2.2 2022 (R Development Core team) was used for the analysis of spore germination. Before analysis, an angular transformation was applied to the percentage incidence data. The raw means are reported in the figures. Mixed-effect models for analysis of variance (ANOVA), followed by Fisher’s least significant difference test (α = 0.05), were used to determine the statistically significant differences between the means. The multivariate regression and best-fits regression functions of Minitab were used to determine the most important climatic factors. The means and totals, where appropriate, of 1, 3, 7, 14, 21, and 28 days were examined. The factors considered were leaf wetness, rainfall, wind run, mean daily temperature, evapotranspiration, and relative humidity. Daily weather data were downloaded from the Havelock North site on MetWatch®2.
Data presented in graphical form were modelled using the statistical fitting function of the graphics package Origin®2022b (OriginLab Corporation, Northampton, MA, USA). A mixed-effects model was used to predict the effect of changes in temperature on the spore germination of P. vagabunda using the R package glmmTMB (Brooks et al., 2017). The slides nested within each time period were included as a random effect because the slides had four samples in each time period. The time since experiment inception and temperature were considered fixed effects. Fixed effects were scaled to a mean of zero and standard deviation of one, so that the regression coefficients for time and temperature could be compared. A beta-regression error term was specified in the model because data were bounded by 0 and 25. Spore counts were transformed into proportions of 25 prior to analysis. Residual diagnostics were carried out using the R package DHARMa (Hartig, 2022), and showed no significant departure from expectations for deviation, dispersion, or outliers.
3 Results
3.1 Skin peel inoculations
3.1.1 The effect of temperature on spore germination
The spore germination models (Figure 1, Table 2) showed that the optimal temperature was 20°C. After 72 h, almost all spores had germinated at all tested temperatures that were above 6°C (Figure 1). After 24, 48, and 72 h, some germination occurred at 0°C.
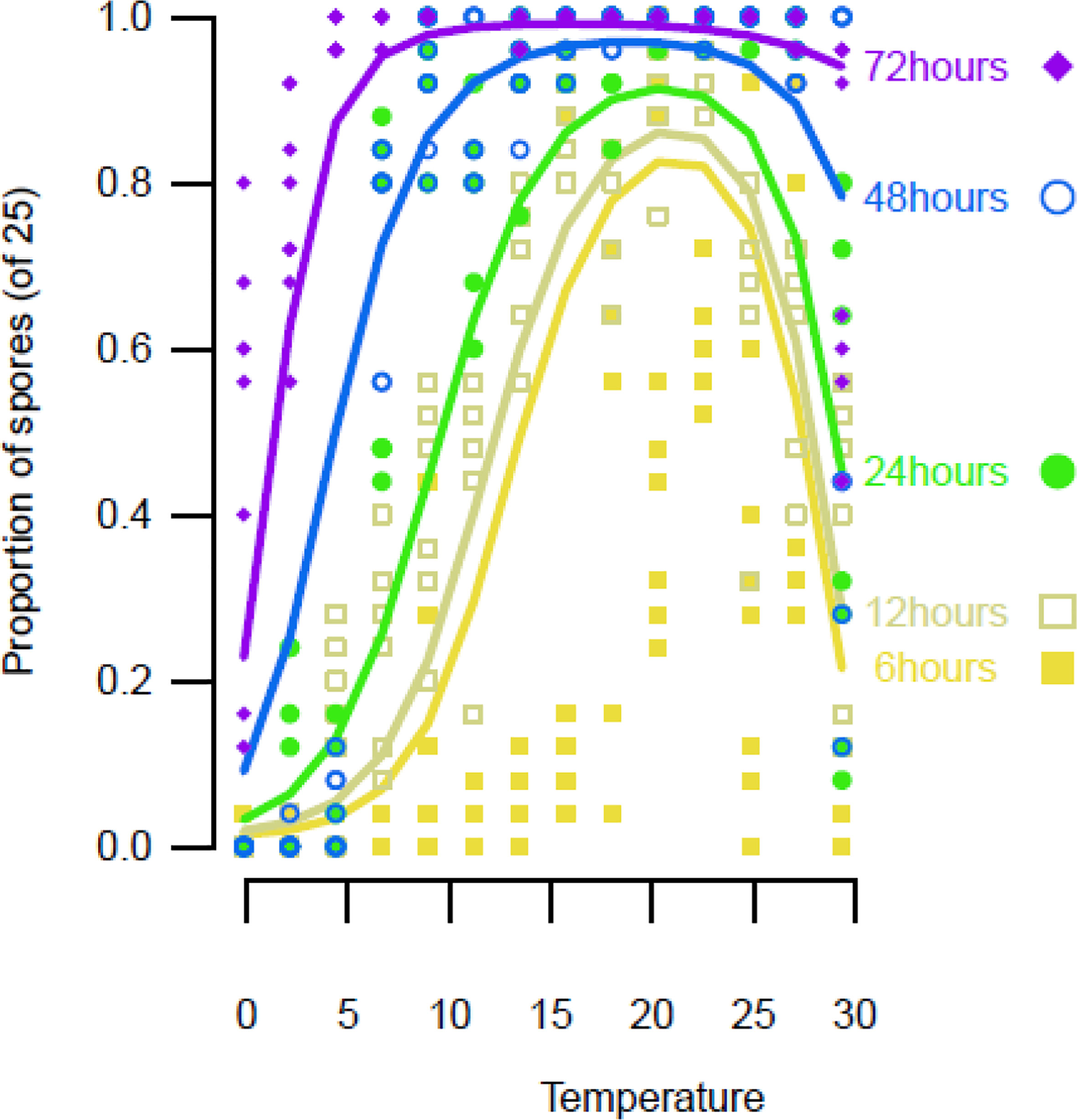
Figure 1 Spore germination on ‘Scired’ apple skins following the placement of a 100-µL aliquot of a suspension of 106 conidia/mL on each end of a strip of excised apple skin placed on a glass microscope slide, after which each drop was covered with a glass cover slip and placed on a temperature gradient plate. The results are the average of three separate experiments on two isolates (KE121, KE171). A mixed-effects third-degree polynomial, with the factors temperature and time, was used to model the data points. Lines are predictions from the mixed-effects beta regression. Points are the observed proportions of spores counted out of a maximum of 25.
3.1.2 Lenticel density and susceptibility
The re-isolations of P. vagabunda from inoculated apple slices were fewest in those removed from the top, next-fewest in those from the shady-cheeks parts, followed by those from the sun-exposed sides, and most numerous from the bottom of parts of the fruit (Figure 2A). Lenticels were fewest from the tissue immediately adjacent to the stem (top), then increased in density in the skin from the shady-cheeks parts, followed by the sun-exposed sides, and were most dense from the skin that was adjacent to the calyx at the bottom of the fruit (Figure 2B). This was a similar pattern to that for lenticel density, in which most re-isolations were found in the peel from the bottom of the fruit (which was also the region with the highest lenticel density), and the fewest re-isolations were observed in peel from the top of the fruit, where lenticel density was the lowest (Figures 2A, B).
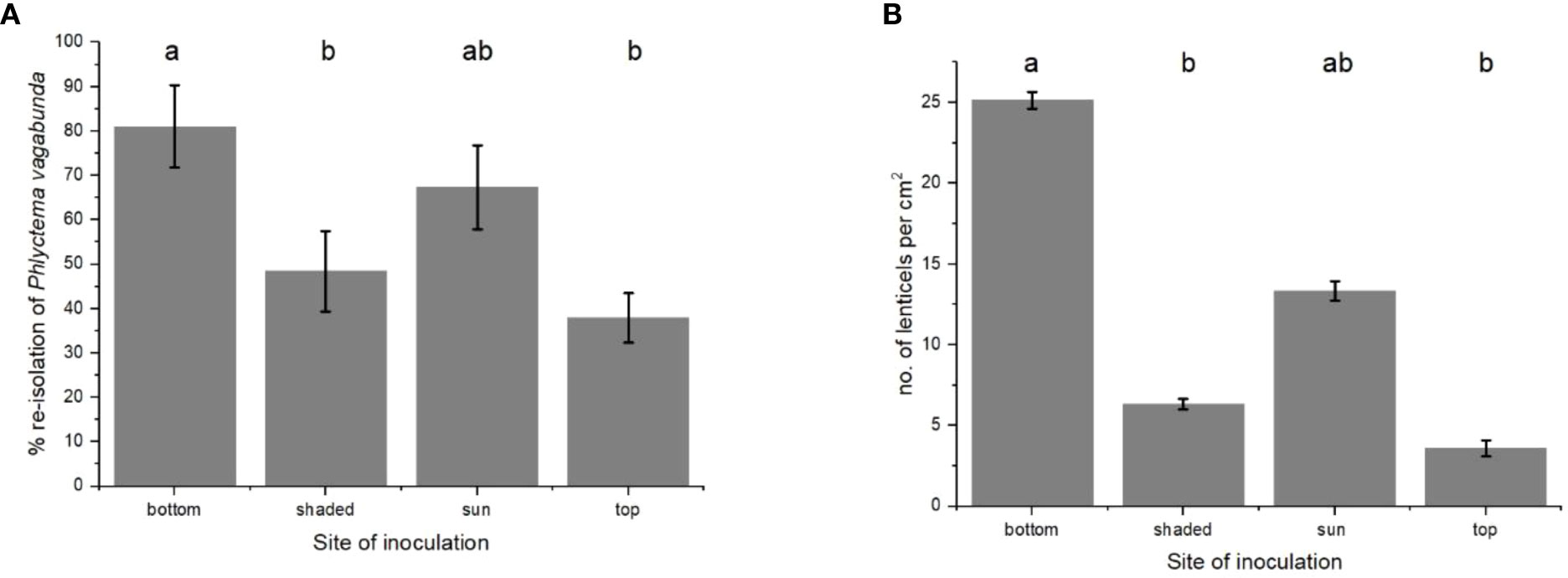
Figure 2 (A) Re-isolations of Phlyctema vagabunda from thin slices of ‘Scired’ apple skin from the bottom, shady-cheeks, sun-exposed, and top sides of six replicate apple fruit and (B) the mean number of lenticels per cm2 on four replicate apples for each type of skin. The skin was inoculated with 106 spores/mL and incubated for 72 h at 20°C. There was no evidence to suggest differences between the means followed by the same letter (LSD, α = 0.05). Values are means ± standard errors. LSD, least significant difference.
3.1.3 Microscopic examination
The microscopic examination of the lenticels on inoculated peels failed to show any penetration into the apple flesh (Figures 3A, B) even after a 72-h incubation period. Mycelium penetrated the space beneath the lenticellular cuticle after 24 h (Figure 3B, grey arrow). A mycelial stroma formed after 72 h (Figure 3B, grey arrow). In the laboratory conditions used in the present study, at 20°C, contact with spores on agar for 72 h was required for spores to germinate, penetrate the lenticel, and form an infection stroma.
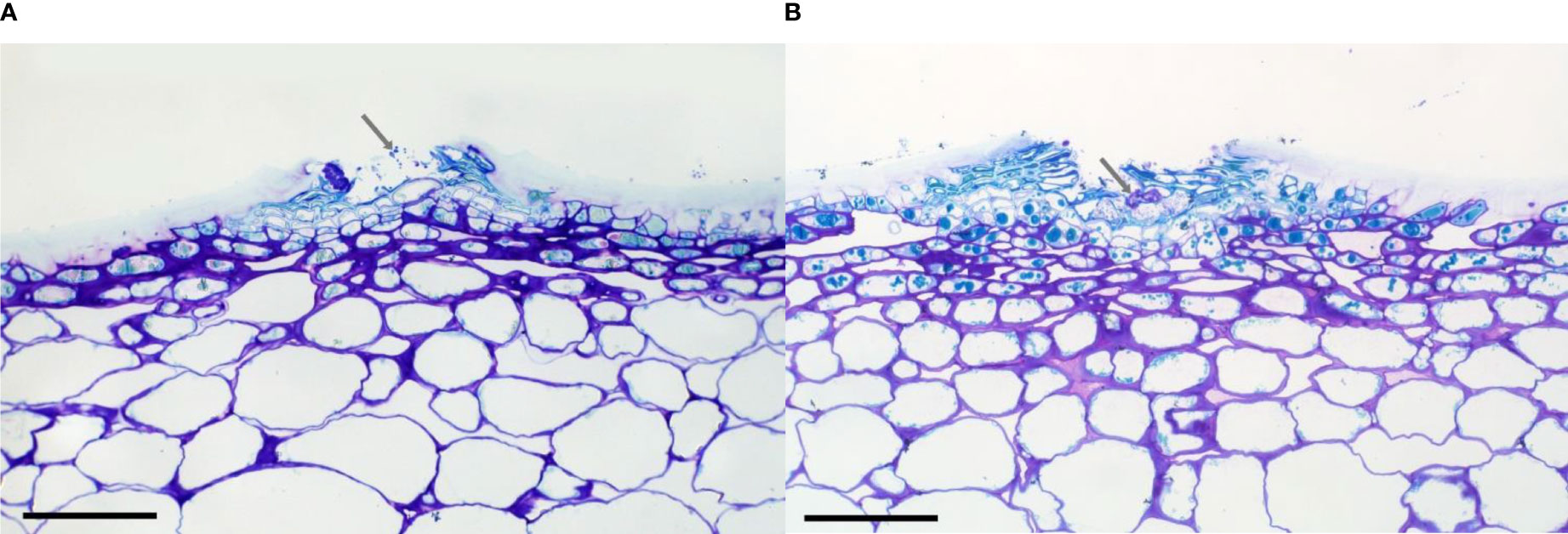
Figure 3 Cross-section through lenticels showing brown staining following (A) 24 h and (B) 72 h of incubation following the inoculation of ‘Scired’ apple skin peels with 106 spores/mL of Phlyctema vagabunda. Arrow shows mycelial strands (A) and a putative infection structure, or stroma (B). Scale bar = 0.1 mm.
3.2 Detached fruit inoculations
3.2.1 Effect of temperature on the infection of detached fruit
The inoculation of ‘Scired’ fruit was carried out by placing fruit slices on a suspension of P. vagabunda conidia (methods in Section 2.5). These showed that the optimal temperature was approximately 21.8°C (Figure 4), which was similar to the 20°C temperature optima for spore germination (Section 3.1.1). Although the model suggested a minimum temperature of 5°C was required, a few infections occurred at 5°C and below, including at 0°C. Lower temperatures were not tested. A few infections occurred at 30°C, and the upper limit for infections predicted from the model (see Equation 1) was 32°C.
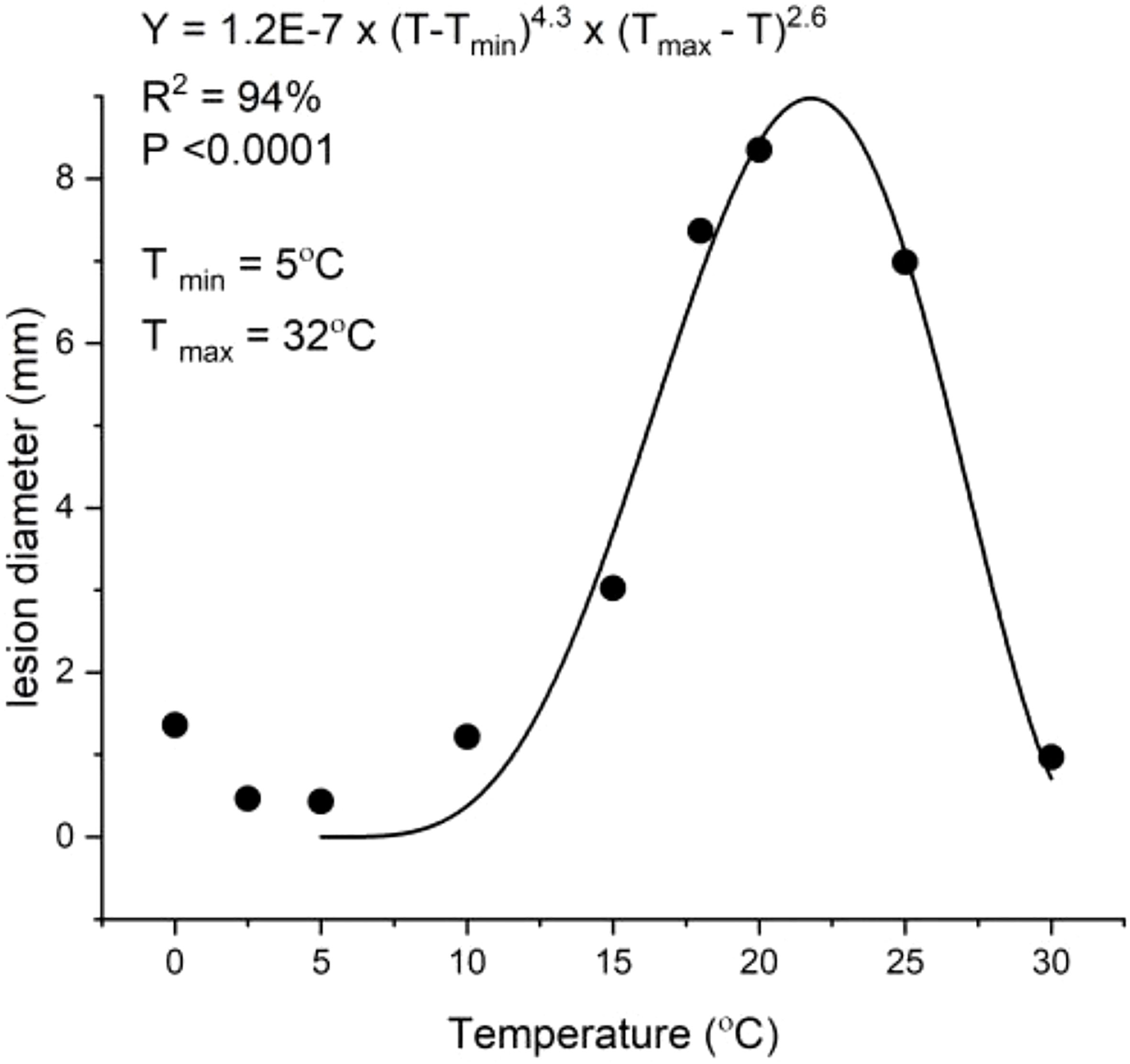
Figure 4 Inoculation of ‘Scired’ apple fruit without wounding by placing on a 100-μL droplet of 106 spores/mL of Phlyctema vagabunda on Difco corn meal agar (CMA) for 72 h showing a temperature (T) optimum of 21.8°C. The experiment was conducted twice. An asymmetric model (Hau et al., 1985) was fitted to the data points. Bull’s eye rot lesions were measured after 20 weeks of cold storage at 0.5°C ± 0.5°C.
where T = Temperature (°C)).
3.2.2 Effect of the incubation time on the infection of detached fruit
Infection did not occur after contact for 6 and 12 h with P. vagabunda conidia. A small amount of infection occurred after contact for 24 and 48 h, and the most successful infections required contact for 72 h (Figure 5). Because the fruit were placed into the cold store immediately after the incubation period concluded, these results suggest that infections for the fruit inoculated by 6 and 12 h of contact with spores were not able to occur in the cold store, probably because spores remaining on the surface of the apple fruit would have become desiccated. Spores were able to penetrate into lenticels after 24 h (Figure 3A), which enhanced their ability to survive desiccation after removal from the highly humid agar surface. However, 72 h was required for a stroma to form at 20°C (3.1.3). Low infections for fruit inoculated by 24 and 48 h contact with spores indicates that formation of the stroma was very slow during cold storage at 0°C.
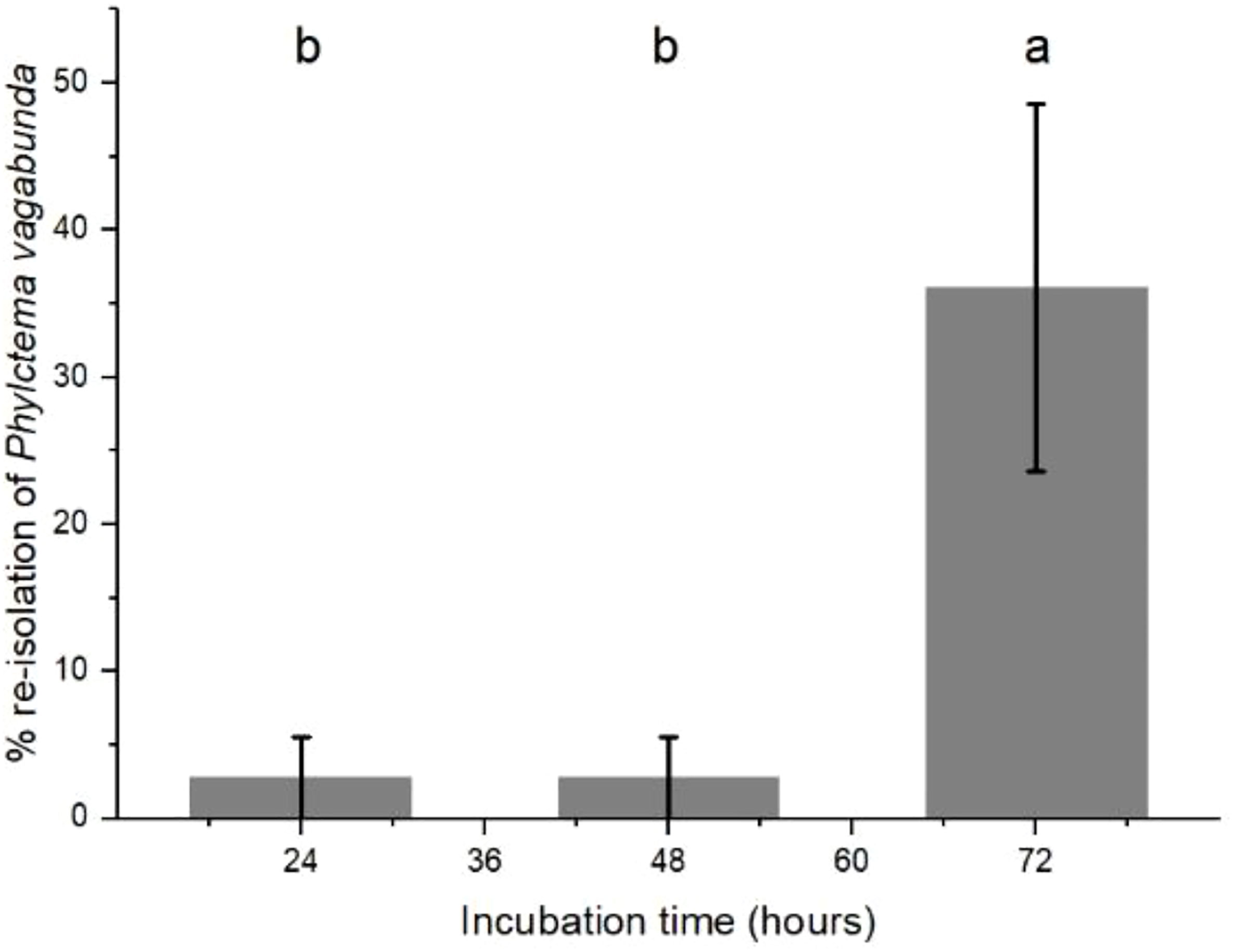
Figure 5 Re-isolation of Phlyctema vagabunda from lesions on inoculated ‘Scired’ apple fruit that had been placed on a 100-μL droplet of 106 spores/mL on Difco corn meal agar (CMA) for 24, 48, and 72 h, then cold stored at 0.5°C ± 0.5°C for 20 weeks. There were no lesions on the fruit that had been inoculated then removed after 6 and 12 h. There was no evidence to suggest differences between means followed by the same letter (LSD, α = 0.05). Values are means ± standard errors, n = 6. LSD, least significant difference.
3.2.3 Monthly detached fruit inoculations
Inoculations of detached ‘Scired’ apple fruit harvested monthly without wounding showed inconsistent patterns (Figure 6A). During 2018 and 2019, the maximum lesion size occurred after inoculation in March, and in 2021 in April. The minimum lesion size occurred in January 2018, in February 2019, and in January 2021. When data for all 3 years were compared with wind run, it was apparent that there was a putative threshold at an average of 240 km/day during the 28 days before harvest (Figure 6B), which is similar to the results of the trap fruit experiment (Figure 7).
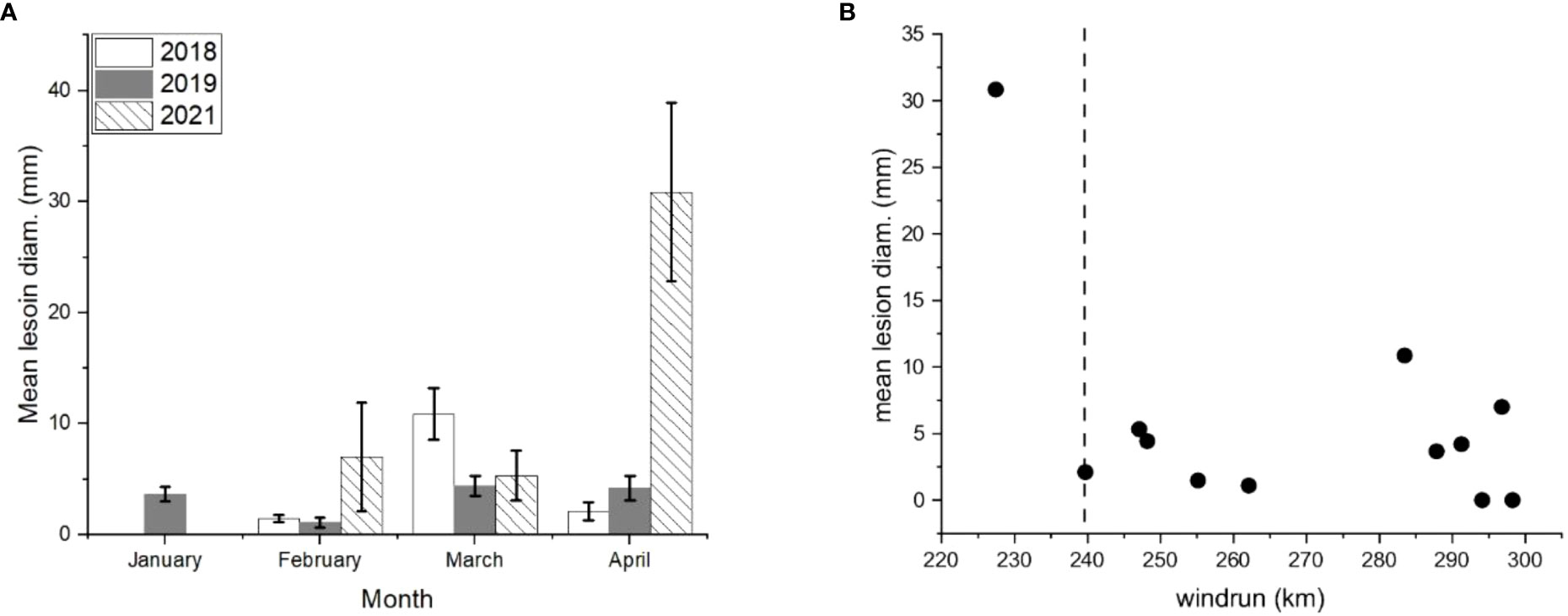
Figure 6 (A) Detached ‘Scired’ apple fruit harvested at monthly intervals in 2018, 2019, and 2020 and inoculated without wounding by placing on a 100-µL aliquot of 106 spores/mL of Phlyctema vagabunda on Difco corn meal agar (CMA) for 72 h The fruit were assessed after 20 weeks of cold storage at 0.5°C ± 0.5°C. The values are means ± standard errors, n = 6. Uninoculated controls remained symptomless except for small (3.3 ± 3.3 mm) lesions in April 2021. (B) The mean lesion diameter for these same detached ‘Scired’ apple fruit versus average wind run (km/day) for 28 days before harvesting in January, February, March, and April. The dotted vertical line represents a putative threshold beyond which fruit become less susceptible to infection by BER.
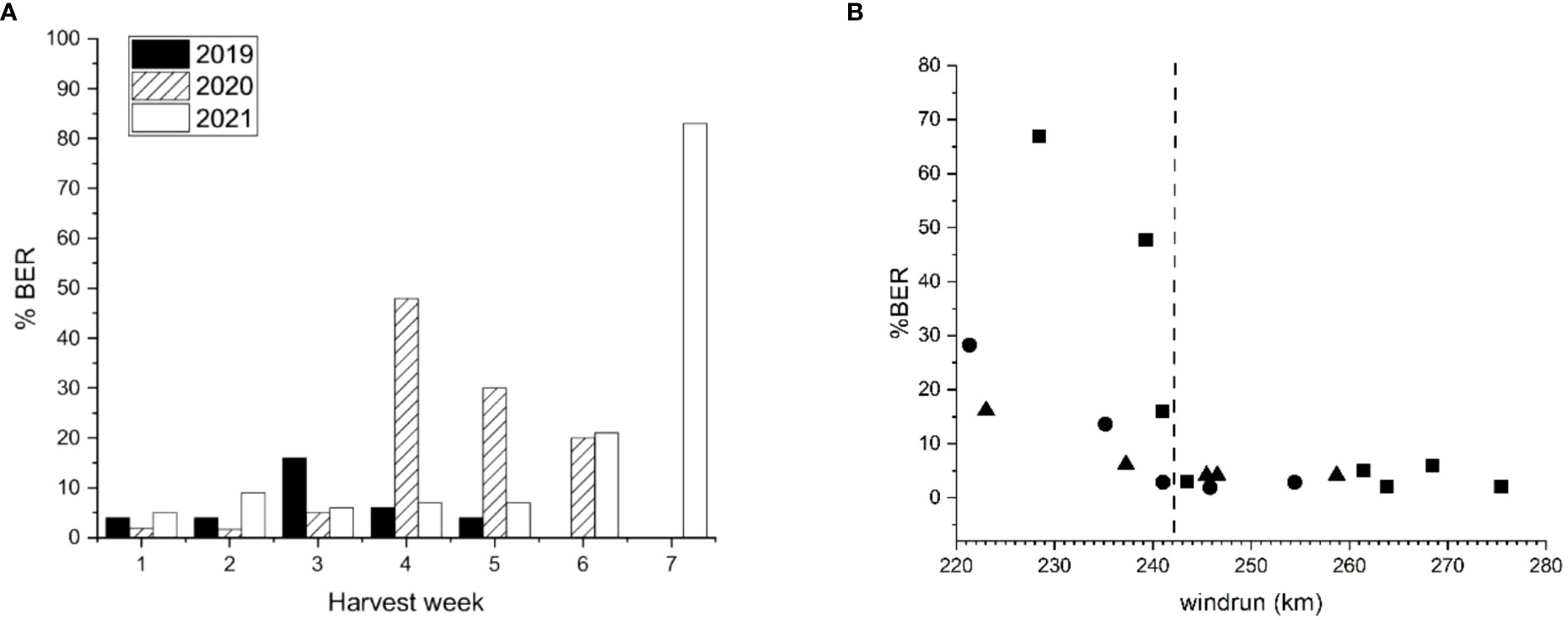
Figure 7 (A) ‘Scired’ apple fruit harvested from the same trees at weekly intervals for 5–7 weeks before final harvest showing the amount of bull’s eye rot symptoms (% BER) after 20 weeks of cold storage (0.5°C ± 0.5°C). In 2019, the fruit were harvested on 14 March, 21 March, 28 March, 4 April, 10 April, and 17 April; in 2020, the fruit were harvested on 26 February, 11 March, 25 March, 3 April, 1 May, and 6 May; and in 2021, 100 fruit were harvested on 1 March, 8 March, 15 March, 22 March, 30 March, 7 April, and 14 April. (B) The amount of bull’s eye rot (% BER) that developed in the same ‘Scired’ apple fruit versus the average for 28 days wind run (km/day). Each data point represents 50 (for 2019 ▲) or 100 (for 2020● and 2021■) fruit harvested at weekly intervals for 5–7 weeks at the end of the season. The dotted vertical line represents a putative threshold beyond which fruit become less susceptible to infection by BER.
3.3 Detached hydrated fruit
The size of the lenticels increased noticeably when ‘Scired’ fruit were soaked in water overnight, from averages of 30 µm to 58 µm (Figure 8A), with a concomitant increase in average fruit weight of 0.62 g (Figure 8B). When ‘Scired’ fruit soaked in water overnight were inoculated without wounding by being placed on a suspension of P. vagabunda conidia for 72 h (method described in Section 2.5), hydrated fruit were more susceptible to infection than control fruit (Figure 8C). The mean lesion diameter was an average of 0.5 mm for control fruit and 4.2 mm for hydrated fruit when fruit were treated after 3 months in the cold store (Figure 8C), and 11 mm for control fruit and 23 mm for hydrated fruit when fruit were treated within 1 week of harvest (Figure 8D). Statistically significant differences were apparent after analysis with mixed-effects ANOVA (p< 0.05) followed by Fisher’s least significant difference (LSD) test (α = 0.05) between control and hydrated fruit for lenticel diameter (Figure 8A), fruit weight (Figure 8B), and infection (Figure 8D).
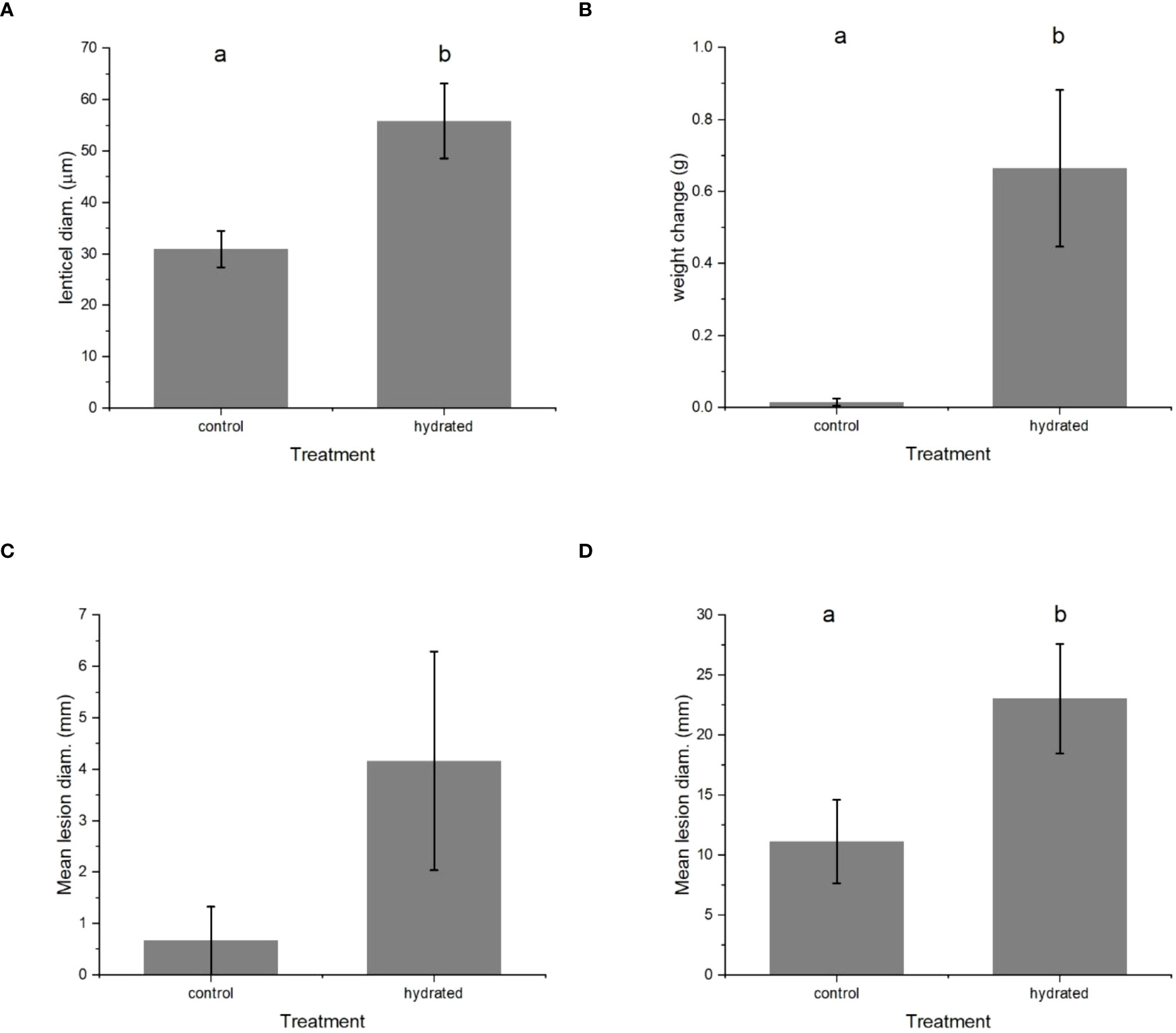
Figure 8 The effect of soaking ‘Scired’ apple fruit in water overnight on (A) lenticel size, (B) weight, and (C) and (D) susceptibility to infection by Phlyctema vagabunda (C, D). Sample sizes were n = 3 for (A–C), and n = 24 for (D). (D) is the repeat experiment conducted at monthly intervals. There was no evidence to suggest differences between the means followed by the same letter (LSD, α = 0.05). The values are means ± standard errors. LSD, least significant difference.
3.3.1 The effect of simulated wind on lenticel size
The lenticels on ‘Scired’ apple fruit that were soaked in distilled water overnight were significantly larger than those on apple fruit that were placed in the airstream of a laminar flow cabinet overnight (Figure 9). Lenticel size increased from 41 µm for the fruit remaining on the laboratory bench (controls) to an average of 52.5 µm when they were soaked in water overnight and decreased to 34.6 µm when they were placed in the airstream of the laminar flow cabinet overnight. Statistically significant differences were apparent after analysis with mixed-effects ANOVA (p< 0.05) followed by Fisher’s LSD test (α = 0.05) (Figure 9).
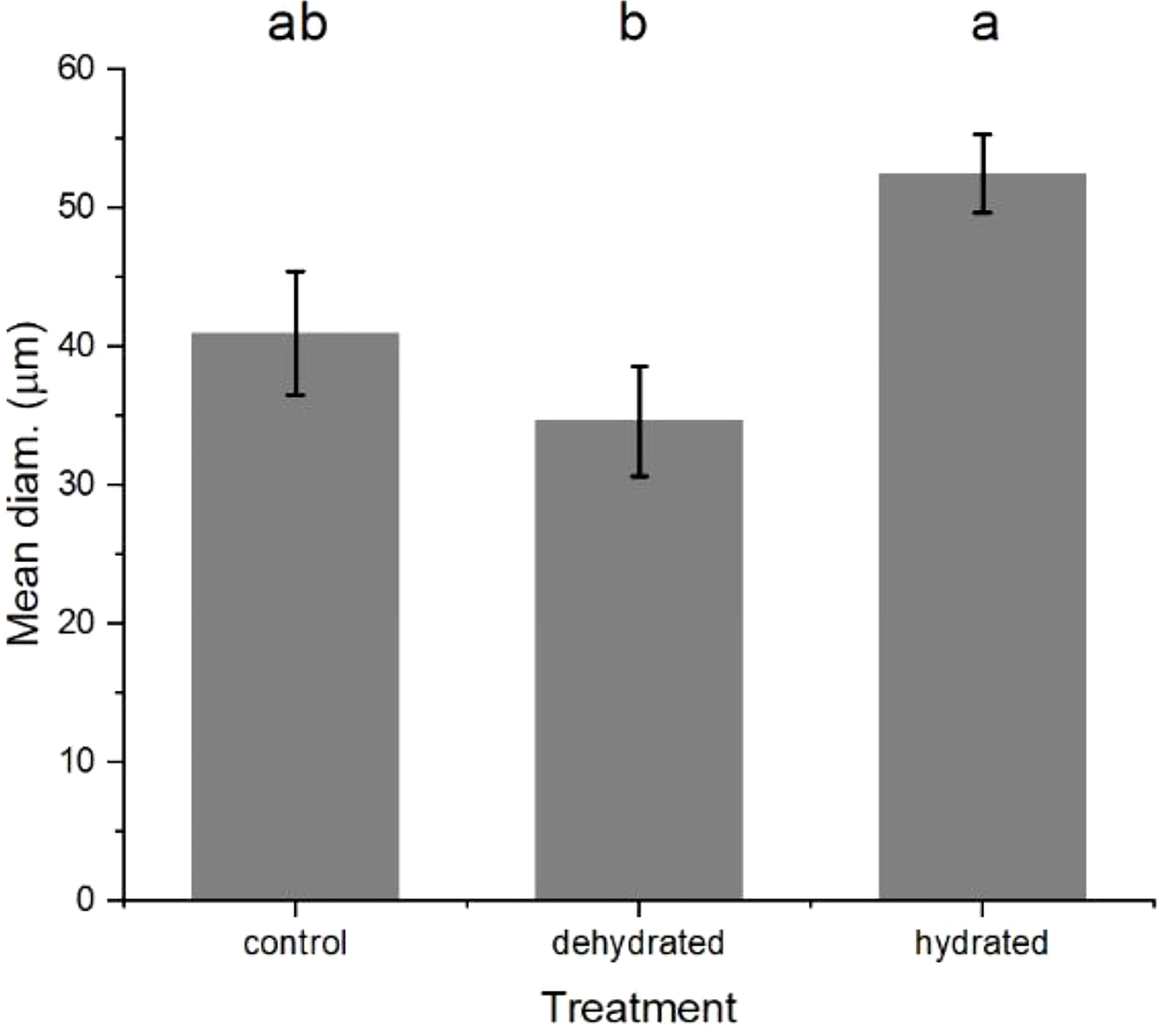
Figure 9 The mean diameter of the lenticels on apple fruit that were soaked in distilled water overnight (hydrated), placed in the airstream of a laminar flow cabinet overnight (dehydrated) and untreated controls (control). There was no evidence to suggest differences between the means followed by the same letter (LSD, α = 0.05). The values are means ± standard errors, n = 5. LSD, least significant difference.
3.4 Orchard experiments
3.4.1 Trap fruit
In 2019, the percentage of ‘Scired’ fruit affected by BER was highest in week 3, in 2020, it was highest in week 4, and in 2021, it was highest in week 7 (Figure 7A). Examination of weather data showed that there was a consistent relationship over these three seasons with wind run (Figure 7B). The fruit that were harvested when wind run was less than approximately 240 km/day for 28 days before harvest showed a BER ranging from 6.1% to 67%. In contrast, fruit that were harvested when the 28-day average for wind run was greater than approximately 240 km/day showed 6% or fewer rots (Figure 7B).
The data points below a threshold of 242 km/day for 28 days were examined. There was a significant relationship (R2 = 76.4%, p = 0.00127; Figure 10) between BER incidence and average daily temperature for 7 days before harvest (7D T) and average leaf wetness for 28 days before harvest (28D LW) (Equation 2):
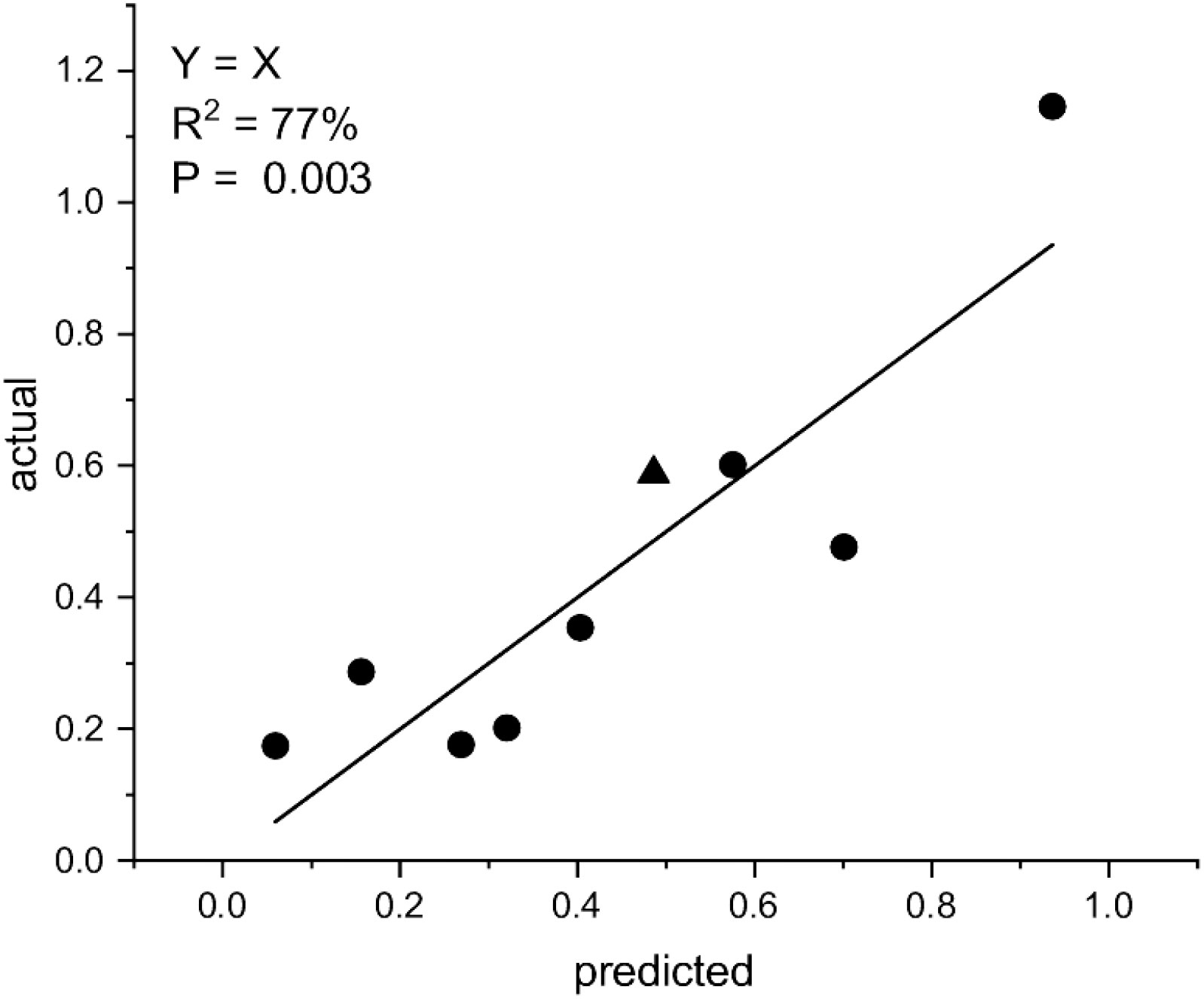
Figure 10 The relationship between the square root arcsine transformed bull’s eye rot (BER) incidence, the mean of daily temperatures for 7 days before harvest (7D T), and the mean of leaf wetness for 28 days before harvest (28D LW), where asin BER = −0.860 + 0.0793 7D T + 0.000433 28D LW. The data include values from the trap fruit from below a wind run threshold of 240 km/day. The triangle indicates the data point from below a wind run threshold of 240 km/day from Figure 6B.
When the model asin BER = −0.860 + 0.0793 7D T + 0.000433 28D LW was applied to the data point below this threshold in Section 3.2.3 (Figure 6B), it aligned with the other eight data points from the trap fruit experiment.
3.4.2 Field inoculations
When ‘Scifresh’ fruit were wound inoculated, there was an increase in the number of infected fruit, which reached a maximum value in March for both seasons (Figures 11A, B). The fruit inoculated without wounding showed no symptoms in 2014–2015 (Figure 11A), and a maximum of 1 month before the final harvest in February in 2015–2016 (Figure 11B). When wind run was examined, the 28-day average was below approximately 240 km/day for both seasons. When Equation 1 was used to predict percent infection, there was no relationship with the amount of BER following wound inoculations, but for the 2015–2016 season there was a significant (p< 0.024) linear regression fitting 81% of the variability in BER following inoculations without wounding.
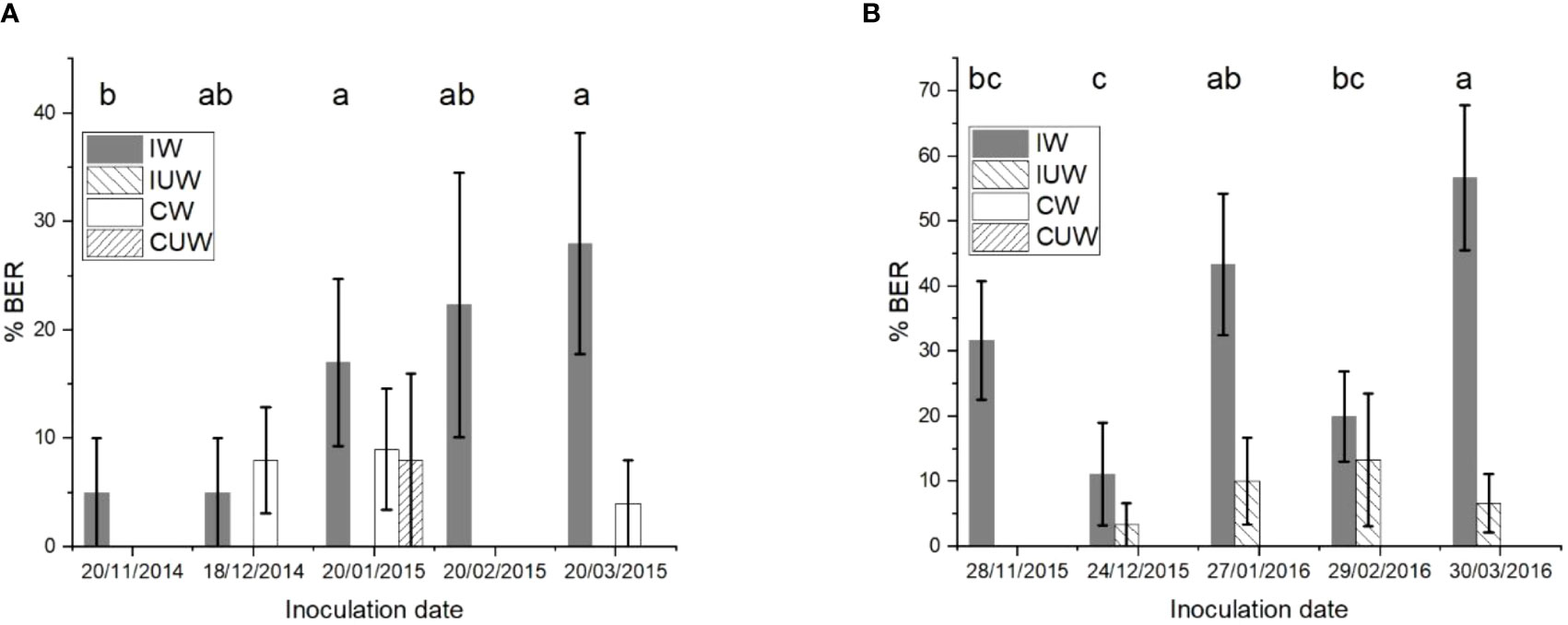
Figure 11 The amount of bull’s eye rot symptoms (% BER) of field inoculated cultivar ‘Scifresh’ apples (marketed as Jazz®) by Phlyctema vagabunda visually assessed after 28 weeks of cold storage at 0.5°C ± 0.5°C. The apples were spray inoculated with a Devibiliss® sprayer (0.25 mL/fruit) containing 105 conidia/mL of P. vagabunda strain KE 171 in 2014–2015 (A), and an equal mixture of strains KE 127, 171, 183, and 829 in 2015–2016 (B), with and without wounding. Uninoculated controls were also sprayed with water with and without wounding. IW = inoculated wounded; IUW = inoculated unwounded; CW = uninoculated control wounded; CUW = uninoculated control unwounded. There was no evidence to suggest differences between means followed by the same letter (LSD, α = 0.05) when the inoculation date was compared. The values are means ± standard errors, n = 25 for 2014–2015, and n = 30 for 2015–2016. LSD, least significant difference.
3.4.3 Inoculum exclusion
The use of the Chinese fruit bags was effective in covering the fruit from fruit set to harvest, albeit problematic due to bags being blown off. The orchards were checked twice weekly for the first month, then weekly, for bag integrity, and any loose or fallen bags were immediately replaced.
The results after 24 weeks of cold storage showed that ‘Sciros’ fruit were most infected by P. vagabunda when the bags were removed from 21 March to 14 April (Figure 12). The rot on the fruit that was not bagged were almost half as common as on those that were unbagged late in the season (Treatment 7). Treatments that resulted in significantly (p< 0.05) fewer rots to unbagged controls were bagged from 20 December 2014, between 20 December 2014 and 19 January 2015, and between 23 February 2015 and 23 March 2015. Either the fruit were not susceptible during the times that these fruit were exposed, or there was no inoculum. The fruit that were unbagged between 19 January 2015 and 23 February 2015 and 23 March 2015 and 14 April 2015 had significantly (p< 0.05) more rots than the fruit that were bagged all season, possibly because fruit were susceptible during those times and/or inoculum was available. Wind run data were not available for this time period, but the effect of the bags would have reduced the influence of wind run on the closure of lenticels.
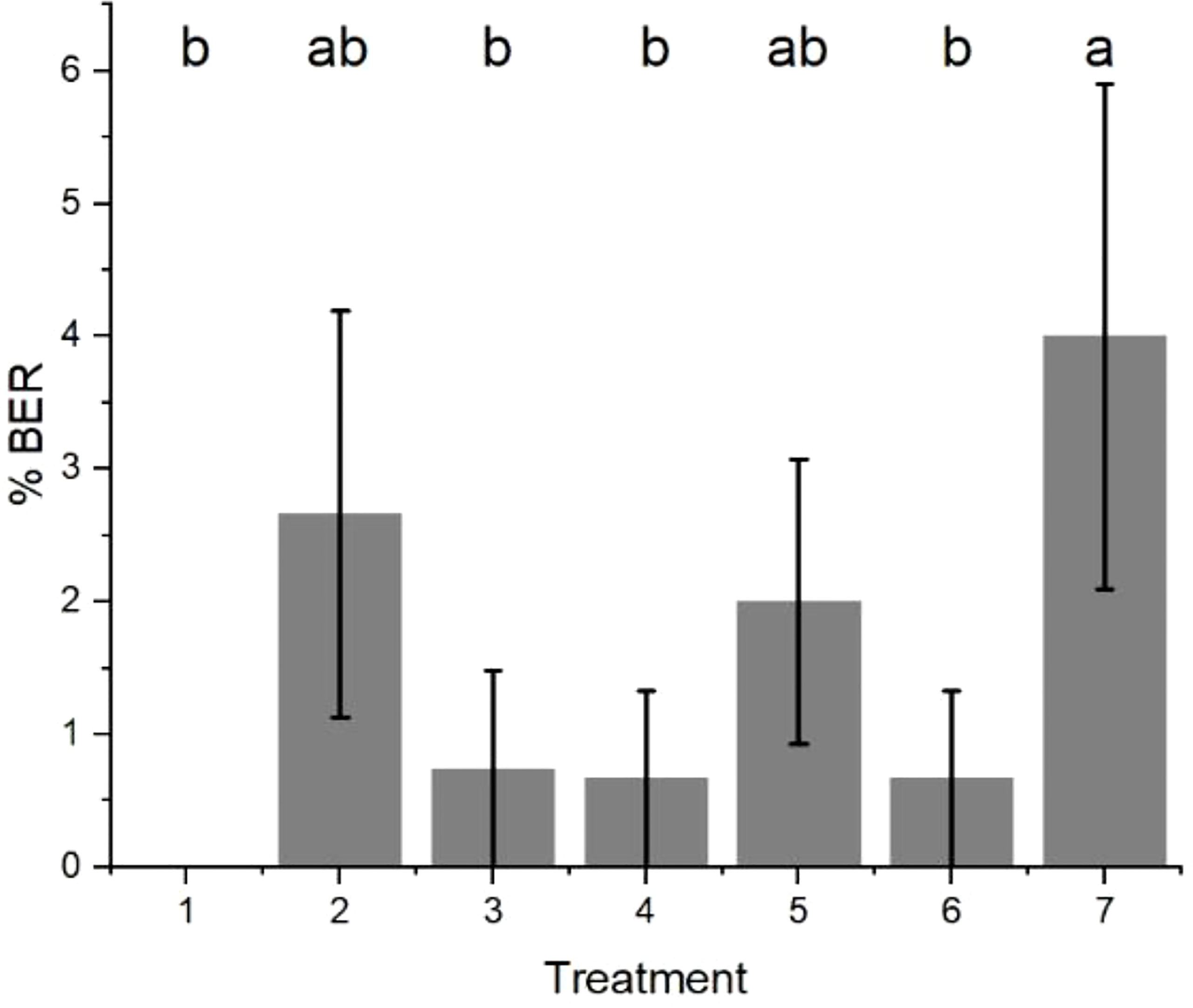
Figure 12 The amount of bull’s eye rot symptoms (% BER) of cultivar ‘Sciros’ apples from the bagging experiment. Treatments were (1) bagged all season (starting just after fruit set on 20 November 2014); (2) unbagged all season; (3) bagged from 20 December 2014; (4) unbagged between 20 December 2014 and 19 January 2015; (5) unbagged between 19 January and 23 February 2015; (6) unbagged between 23 February and 23 March 2015; and (7) unbagged between 23 March and 14 April 2015. Seven fruit (one per treatment) per each of 15 trees were treated, giving a total of 105 fruit. There was no evidence to suggest differences between means followed by the same letter (LSD, α = 0.05). The fruit were harvested on 14 April 2014 and visually assessed after cold storage at 0.5°C ± 0.5°C for 24 weeks. The values are means ± standard errors, n = 15. LSD, least significant difference.
4 Discussion
Our results showed for the first time that hydration increased the size of lenticels, and that this in turn increased the susceptibility of hydrated apple fruit to infection by P. vagabunda. Dehydration resulted in a concomitant decrease in lenticel size.
In agreement with our results, Edney (1956) showed that lenticels that are closed or partially closed are less susceptible to infection by P. vagabunda. Later, Edney et al. (1977) showed that the susceptibility of freshly harvested apple fruit to infection by P. vagabunda was increased by sustained simulated rain. This effect was also found when the fruit were immersed in running tap water for 2 h. Although the mechanism was not known at the time, based on our results, the probable cause was the expansion of lenticels following hydration that then increased the susceptibility to infection by P. vagabunda.
Apple lenticels are filled with suberised cells that have intercellular spaces for gas and water vapour exchange (Esau, 1977). These cells expand and more completely fill this space when the fruit are hydrated, and contract when they are dehydrated (Lendzian, 2006; Everett et al., 2008). Oxygen permeance was also reduced for dehydrated Betula potaninii lenticels, because of a reduction in intercellular spaces due to shrinkage of the filling cells (Lendzian, 2006). These responses to water stress result in closed lenticels that reduce water loss during drought (Lendzian, 2006). In the present study, the expansion of filling tissue following hydration led to an increased diameter of hydrated lenticels.
The effect of hydration on lenticels and thus on susceptibility could explain the relationships with wind run observed here. Increased wind run has been shown to increase evaporation from soya plants (Sturrock, 1970), which had the effect of reducing yield and growth due to decreased moisture supply. On apples, higher wind speed was shown to be the climatic factor with the greatest effect on yield, and more wind equated to lower production (Li et al., 2022). In both examples, an eventual response to dehydration would have ensued, resulting in a drought response of smaller lenticels. Therefore, from this current study, the observation of fewer BER fruit infections when wind run was > c. 240 km/day could be due to lenticel contraction stimulated by wind-induced dehydration. The inclusion of rainfall in the analysis did not improve the relationship, probably because leaf wetness and wind run were better indicators of fruit hydration and lenticel diameter.
The timings required for lenticel contraction and expansion in the field are not known, but in the laboratory, overnight immersion in water was sufficient to distend lenticels and increase susceptibility to infection. As mentioned previously, Edney et al. (1977) showed that a 2-h exposure to running water was also sufficient to increase susceptibility. Detailed detached fruit experiments on avocado, on which hydrated lenticels produced an observable brown discolouration following “jostling” (simulated packing damage), also showed that a 2-h hydration was required to distend lenticels. These experiments on avocado also showed that 2 h of dehydration reversed the effect (Everett et al., 2008). However, the relationship with both > 240 km/day wind run and the model for BER infection when wind run< 240 km/day included 28-day averages for wind run and for leaf wetness. This suggests that lenticel susceptibility to infection in the field may have been more dynamic than in detached fruit experiments.
Harker and Ferguson (1988) showed that lenticel number and diameter increased as the fruit developed and, in the most mature fruit, lenticels were often torn, which together contributed to a large increase in the ability of mature fruit to take up calcium through their lenticels. Lenticel permeability was also affected by wax and cuticle thickness (Harker and Ferguson, 1988), both of which increase as the fruit mature. The apparent increase in lenticel number late in development was attributed to cracks and small flaws in the cuticle. However, the fruit cuticle continues to develop and grow to accommodate fruit expansion, and cuticle components, such as waxes, can grow over lenticels, resulting in their closure (Harker and Ferguson, 1988; Curry, 2009). This in part could explain the 28-day climatic effects, which may have been affecting lenticel formation and closure.
Surface moisture has been shown to induce microcracks in apple cuticles, and it was shown that this effect was not due to the uptake of water through the roots (Knoche and Grimm, 2008). Therefore, a complex interaction between surface wetness, as measured by leaf wetness, and dehydration, as measured by wind run, during the 28 days before harvest may have had an impact on lenticel and microcrack incidence and diameter. These factors have been shown to affect the susceptibility of apple fruit to infection by P. vagabunda. Surface wetness would also have promoted spore germination.
The relationship between lenticel density and increasing susceptibility to P. vagabunda has not been previously demonstrated. The differing susceptibility of apple varieties to P. vagabunda (Di Francesco et al., 2019) and N. malicorticis (Spotts et al., 1999) has been demonstrated, but the mechanism underlying this remains undiscovered. Our results showing that lenticel density was highest at the bottom of the fruit and lowest at the top agree with those of Khanal et al. (2020). Studies have sought to identify antifungal compounds in the apple skin and flesh that reduce in concentration as apple fruit matures to explain late-season infection and varietal differences in susceptibility. Although in vitro inhibition to these compounds was shown, the concentrations of these compounds in apple fruit were not obviously related to fruit susceptibility (Hulme and Edney, 1960; Edney, 1964; Edney, 1974; Lattanzio et al., 2001; Di Francesco et al., 2019). In contrast, Guan et al. (2015) showed that variable susceptibility by different apple varieties to Botryosphaeria dothidea was related to lenticel and microcrack size. Therefore, a comparison of lenticel and microcrack size and density may at least partially explain varietal differences in susceptibility to P. vagabunda and late-season susceptibility.
Appressoria were previously described as the infection structure for BER fungi (Edney, 1958; Dugan et al., 1993a), although this was based on studies of N. perennans and N. malicorticis. The structure described here as a stroma may be the initial structure that eventually develops into the well-rounded appressorium, as illustrated in the study by Edney (1958), or the infection structure for P. vagabunda may differ in shape. From our results, a 72 h-duration of wetness and contact with spores was required for postharvest expression to occur, which suggests that the formation of an infection stroma before the fruit were placed in the cold store was necessary for disease expression. The results from the trap fruit experiment show that infection occurred within 1 week before harvest because of the lack of accumulated infections during the 5–7 weeks that the fruit were harvested at weekly intervals. The strong relationship with climatic factors (wind run, temperature, and leaf wetness) provides evidence that the peaks of infection, which varied between the three seasons, were not random.
At 15°C, 75% of N. malicorticis spores germinated after 20 h and 30 min (Edney, 1974), which was similar to our results for P. vagabunda. Only 6 h were required for 60% of the P. vagabunda spores to germinate at 15°C, and examination of the 5-year average of hourly temperatures in the 2 months of and immediately before harvest (March and April) showed that these conditions were frequently met or exceeded. Temperatures on apple fruit surfaces have been shown to be up to 10°C higher than ambient temperatures (Saudreau et al., 2011), indicating that in the field temperatures would be unlikely to limit spore germination.
Infection occurred after a 0.5 h-wetness period on pears inoculated in the field (Henriquez et al., 2008), and wetness was not considered to be a factor limiting infection. However, in our experiments, the fruit were placed in the cold store immediately after cessation of the incubation period at 20°C, possibly explaining the requirement for a 72-h duration for stroma formation, which appears to be critical to the successful establishment of infections. Once the conidia had invaded the lenticellular cavity, which was shown in the present study to be after at least 24 h, it is probable that wetness was no longer important, but instead temperature was the factor limiting stroma formation. Although 72 h at 20°C was sufficient for the formation of a stroma in the laboratory, at fluctuating field temperatures, this process may need more time. The stroma may have required 7 days to form in the field, otherwise there would have been confounding overlapping infection events obscuring relationships with the climatic factors. In support of this hypothesis, the modelling of natural infections showed that 7-day temperature was a significant factor. The impact of desiccation on spore survivability should also be considered as, Edney (1974) showed that in very dry conditions, spores lost viability in 7–10 days.
After harvest, Edney (1958) and Dugan et al. (1993b) posited that for infection of apples by N. perennans, mycelium from the stroma penetrates through the base of the lenticel into the apple flesh, a process similar to the invasion of avocado fruit tissue from Colletotrichum gloeosporioides appressoria after latency is broken during ripening (Coates et al., 1993). For P. vagabunda, 12 weeks of cold storage was required before the symptoms were expressed (Everett et al., 2021), which suggests that this length of time was required for invasion into fruit flesh from the stroma to take place.
Several studies, and our results, suggest that the most important period for infection by P. vagabunda is the late season (Spotts, 1985; Henriquez et al., 2008; Cameldi et al., 2016; Spadaro et al., 2020), and it is for this reason that the trap fruit studies targeted the final weeks before harvest. Spraying experiments, which showed that fungicides applied close to harvest provided effective control of BER (Moore and Edney, 1959; Cameldi et al., 2014), also support the hypothesis that infection occurs towards the end of the season. Bagging was shown to increase the size of lenticels (Han et al., 1999), possibly explaining the higher amounts of BER in fruit that were unbagged during late March and early April than those that had never been bagged. Thus, bagging may have increased susceptibility to infection, and results need to be interpreted carefully.
The susceptibility of unwounded fruit did not steadily increase towards the end of the season, as has been shown here by several laboratory and field experiments. Our hypothesis is that the state of the lenticels is an important factor influencing susceptibility when fruit are not wounded. Late-season fruit have more lenticels and microcracks (Harker and Fergusson 1988), which explains their increased susceptibility and the necessity of applying fungicides for good control. However, this is mitigated by fruit hydration that also influences susceptibility. When the fruit are wounded, the effect of the lenticels is negated, and underlying physiological responses, such as antifungal compounds that degrade as the fruit mature (Hulme and Edney, 1960; Edney, 1964; Edney, 1974; Lattanzio et al., 2001; Di Francesco et al., 2019), may influence their increased susceptibility as the season progresses. Indeed, both field inoculation experiments showed that wound-inoculated fruit increased in susceptibility through the season. An unexplained factor during the second year of inoculations yielded lower infections on alternate months.
Olsen (1965) showed that fruit cold stored for 2–3 months had more closed lenticels than freshly harvested fruit. When Edney et al. (1977) inoculated hydrated apples that had been stored for 5 months, infection rarely occurred. Singh et al. (2016) showed that the number of open lenticels was significantly smaller after apple fruit were cold stored for 2 months. Our detached fruit experiments in 2018 were conducted less than 1 month after the harvest, in 2019, due to an equipment breakdown; the January harvested fruit inoculation was after 2.5 months, but the rest were carried out within 2 months post-harvest. In 2020, all inoculations were conducted within 1 week of the harvest. Results were difficult to interpret until 28-day wind run was examined, and the one data point that was below 240 km/day aligned with the results of the trap fruit experiment when the model was used.
Lenticel density and diameter have been shown to be highly correlated to susceptibility to infection by P. vagabunda in vitro, and hydration is an important determinant of lenticel diameter. The relationship with wind run suggested that the state of the lenticels is also critical for infection by this fungus in the field. Further experiments that use direct measurements of lenticel diameter of fruit in the orchard and relate this to susceptibility to BER are required to confirm this hypothesis. Infections through wounds may also increase towards the end of the season as antifungal compounds break down. The preharvest application of compounds that close lenticels, such as gibberellic acid (Guan et al., 2015), could provide some control of BER without the need for fungicides.
Data availability statement
The raw data supporting the conclusions of this article will be made available by the authors, without undue reservation.
Author contributions
KE was responsible for overall conception and design of the work. PW contributed to implementation and interpretation, and co-designed the fieldwork. LH, IP, and MV conducted the bulk of the laboratory work and contributed to its design. BF contributed to the execution of the bulk of the fieldwork. PS contributed the micrographs. KR and SH provided statistical advice and conducted complex statistical analyses. All authors contributed to the article and approved the submitted version.
Funding
The authors thank the Apple Futures II Partnership Programme (PNZEA1401), which was funded by the Ministry for Business, Innovation and Employment (MBIE), and New Zealand Apples and Pears Incorporated (NZAPI) for funding. Thanks also go to PFR internal funds (Growing Futures, Simulating Orchard Ecosystems Direction, Digital Horticultural Systems Programme) for covering publication costs.
Acknowledgments
Thanks to apple growers for allowing access and providing orchards and fruit.
Conflict of interest
The authors declare that the research was conducted in the absence of any commercial or financial relationships that could be construed as a potential conflict of interest.
Publisher’s note
All claims expressed in this article are solely those of the authors and do not necessarily represent those of their affiliated organizations, or those of the publisher, the editors and the reviewers. Any product that may be evaluated in this article, or claim that may be made by its manufacturer, is not guaranteed or endorsed by the publisher.
Footnotes
- ^ 2023 Best Seller 100x Pulp Paper Bags Fruits Protection Pouch Orchard Against Insect Bird Hot | Fruugo NZ
- ^ HortPlus MetWatch
References
Brooks M. E., Kristensen K., van Benthem K. J., Magnusson A., Berg C. W., Nielsen A., et al. (2017). glmmTMB Balances speed and flixibility among packages for zero-inflated generalized linear mixed modeling. R. J. 9, 378–400. doi: 10.32614/RJ-2017-066
Cameldi I., Ceredi G., Spadoni A., Ventrucci D., Mari M. (2014). “Lenticel rot of apple fruit caused by Phlyctema vagabunda (ex Gloeosporium album): epidemiological aspects and disease management,” in Atti, Giornate Fitopatologiche, vol. 93-102. (Chianciano Terme (Siena).
Cameldi I., Neri F., Ventrucci D., Ceredi G., Muzzi E., Mari M. (2016). Influence of harvest date on bull’s eye rot of ‘Cripps pink’ Apple and control chemical strategies. Plant Dis. 100, 2287–2293. doi: 10.1094/PDIS-05-16-0615-RE
Chen C., Verkley G. J. M., Sun G. Y., Groenewald J. Z., Crous P. W. (2016). Redefining common endophytes and plant pathogens in Neofabraea, Pezicula, and related genera. Fungal Biol. 120, 1291–1322. doi: 10.1016/j.funbio.2015.09.013
Clements H. F. (1935). Morphology and physiology of the pome lenticels of Pyrus malus. Bot. Gaz. 97, 101–117. doi: 10.1086/334539
Coates L. M., Muirhead I. F., Irwin J. A. G., Gowanlock D. H. (1993). Initial infection processes by Colletotrichum gloeosporioides on avocado fruit. Mycological Res. 97, 1363–1370. doi: 10.1016/S0953-7562(09)80171-8
Creemers P. (2014). “Anthracnose canker and Perennial canker,” in Compendium of Apple and Pear Diseases and Pests, 2nd ed. (St. Paul, Minnesota: APS Press), 51–53.
Cunnington J. H. (2004). Three Neofabraea species on pome fruit in Australia. Australas. Plant Pathol. 33, 453–454. doi: 10.1071/AP04034
Curry E. A. (2009). “Growth-induced microcracking and repair mechanisms of fruit cuticles,” in SEM Annual Conference, Society for Experimental Mechanics Inc, Albuquerque, NM, USA.
Den Breeyen A., Rochefort J., Russouw A., Meitz-Hopkins J., Lennox C. L. (2020). Preharvest detection and postharvest incidence of phlyctema vagabunda on ‘Cripps pink’ Apples in South Africa. Plant Dis. 104, 841–846. doi: 10.1094/PDIS-04-19-0818-RE
Di Francesco A., Cameldi I., Neri F., Barbanti L., Folchi A., Spadoni A., et al. (2019). Effect of apple cultivars and storage periods on the virulence of Neofabraea spp. Plant Pathol. 68, 1525–1532. doi: 10.1111/ppa.13074
Dugan F. M., Grove G. G., Rogers J. D. (1993a). Comparative studies of Cryptosporiopsis curvispora and C. perennans. I. Morphology and pathogenic behavior. Mycologia 85, 551–564. doi: 10.1080/00275514.1993.12026308
Dugan F. M., Roberts R. G., Grove G. G. (1993b). Comparative studies of Cryptosporiopsis curvispora and C. perennans. II. Cytology and vegetative compatibility. Mycologia 85, 565–573. doi: 10.1080/00275514.1993.12026309
Edney K. L. (1956). The rotting of apples by Gloeosporium perennans Zeller & Childs. Ann. Appl. Biol. 44, 113–128. doi: 10.1111/j.1744-7348.1956.tb06850.x
Edney K. L. (1958). Observations on the infection of Cox’s orange pippin apples by Gloeosporium perennans Zeller & Childs. Ann. Appl. Biol. 46, 622–629. doi: 10.1111/j.1744-7348.1958.tb02245.x
Edney K. L. (1964). A comparison of the production of extra-cellular enzymes and rotting apples by Pezicula alba and P. Malicorticis. Trans. Br. Mycol. Soc. 47, 215–225. doi: 10.1016/S0007-1536(64)80055-3
Edney K. L. (1974). Pezicula Malicorticis: factors affecting spore germination and invasion of apple fruit. Trans. Br. Mycol. Soc. 62, 25–34. doi: 10.1016/S0007-1536(74)80002-1
Edney K. L., Tan A. M., Burchill R. T. (1977). Susceptibility of apples to infection by Gloeosporium album. Ann. Appl. Biol. 86, 129–132. doi: 10.1111/j.1744-7348.1977.tb01823.x
Everett K. R. (2003). The effect of low temperatures on Colletotrichum acutatum and Colletotrichum gloeosporioides causing body rots of avocados in New Zealand. Australas. Plant Pathol. 32, 441–448. doi: 10.1071/AP03050
Everett K. R., Hallett I. C., Rees-George J., Chynoweth R. W., Pak H. A. (2008). Avocado lenticel damage: The cause and the effect on fruit quality. Postharvest Biol. Technol. 48, 383–390. doi: 10.1016/j.postharvbio.2007.09.008
Everett K. R., Hasna L., Pushparajah I. P. S., Middleditch C., Ramos L., Vergara M. J., et al. (2021). Semi-commercial hot water treatments to control apple bull’s eye rot (Neofabraea alba). Acta Hort. 1325, 273–278. doi: 10.17660/ActaHortic.2021.1325.39
Everett K. R., Pushparajah I. P. S., Fisher B. M., Wood P. N. (2017). A simple method for conidial production and establishing latent infections of apples by Phlyctema vagabunda (syn: Neofabraea alba). NZ Plant Prot. 70, 106–111. doi: 10.30843/nzpp.2017.70.35
Everett K. R., Pushparajah I. P. S., Timudo-Torrevilla O. E., Ah Chee A., Scheper R. W. A., Shaw P. W., et al. (2018). Infection criteria, inoculum sources and splash dispersal of Colletotrichum acutatum causing bitter rot of apples in New Zealand. Eur. J. Plant Pathol. 152, 367–383. doi: 10.1007/s10658-018-1481-0
Everett K. R., Pushparajah I. P. S., Vergara M. J., Hasna L., Wood P. N., Fisher B. M. (2022). Phlyctema vagabunda is the main causal agent of apple bull’s eye rot in New Zealand. Australas. Plant Pathol. 51, 519–524. doi: 10.1007/s13313-022-00885-6
Gariepy T. D., Rahe J. E., Levesque C. A., Spotts R. A., Sugar D. L., Henriquez J. L. (2005). Neofabraea species associated with bull’s-eye rot and cankers of apple and pear in the Pacific Northwest. Can. J. Plant Pathol. 27, 118–124. doi: 10.1080/07060660509507202
Grove G. G. (1990). “Anthracnose and Perennial Canker,“ in Compendium of Apple and Pear Diseases (St. Paul, Minnesota: APS Press), 27, 36-38
Guan Y., Chang R., Liu G., Wang Y., Wu T., Han Z., et al. (2015). Role of lenticels and microcracks on susceptibility of apple fruit to Botryosphaeria dothidea. Eur. J. Plant Pathol. 143, 317–330. doi: 10.1007/s10658-015-0682-z
Han J., Lee H., Jang H., Hong K., Choi J., Kim K., et al. (1999). Comparison of skin characteristics between non-bagged and bagged ‘Hosui’ pear (Pyrus pyrifolia Nakai) fruits. Jour. Korean Soc. Hortic. Sci. 40, 439–442.
Harker F. R., Ferguson I. B. (1988). Transport of calcium across cuticles isolated from apple fruit. Sci. Hortic. 36, 205–217. doi: 10.1016/0304-4238(88)90055-6
Hartig F. (2022) DHARMa: Residual diagnostics for hierarchical (multi-level/mixed) regression models. Available at: https://CRAN.R-project.org/package=DHARMa (Accessed December).
Hau B., Eisenshmith S. P., Kranz J. (1985). “Construction of temporal models: II Simulation of aerial epidemics,” in Mathematical modelling of crop disease. Ed. Gilligan Ci. A. (London, New York: Academic Press), 31–65.
Henriquez J. L. (2005). First report of apple rot caused by Neofabraea alba in Chile. Plant Dis. 89, 1360. doi: 10.1094/PD-89-1360B
Henriquez J. L., Sugar D., Spotts R. A. (2008). Effects of environmental factors and cultural practices on bull’s eye rot of pear. Plant Dis. 92, 421–424. doi: 10.1094/PDIS-92-3-0421
Hulme A. C., Edney K. L. (1960). “Phenolic substances in the peel of Cox’s Orange Pippin apples with reference to infection by G. perennans,” in Phenolics in plants in health and disease (London: Pergamon Press), 87–94.
Johnston P. R., Pennycook S. R., Manning M. A. (2005). Taxonomy of fruit-rotting fungal pathogens: what’s really out there? NZ Plant Prot. 58, 42–46. doi: 10.30843/nzpp.2005.58.4252
Khanal B. P., Si Y., Knoche M. (2020). Lenticels and apple fruit transpiration. Postharvest Biol. Technol. 167, 111221. doi: 10.1016/j.postharvbio.2020.111221
Knoche M., Grimm E. (2008). Surface moisture induces microcracks in the cuticle of ‘Golden delicious’ Apple. HortScience 43, 1929–1931. doi: 10.21273/HORTSCI.43.6.1929
Lattanzio V., de Venere D., Linsalata V., Bertolini P., Ippolito A., Salerno M. (2001). Low temperature metabolism of apple phenolics and quiescence of Phlyctema vagabunda. J. Agric. Food Chem. 49, 5817–5821. doi: 10.1021/jf010255b
Lendzian K. J. (2006). Survival strategies of plants during secondary growth: barrier properties of phellems and lenticels towards water, oxygen, and carbon dioxide. J. Exp. Bot. 57, 2535–2546. doi: 10.1093/jxb/erl014
Li X., Fan B., Wei R., Yan J., Liu U., Song X. (2022). Grey correlation analysis between climate yield and meteorological factors during growth period of apple in Yantai. Jour. Xinyang Normal Uni (Natural Sci. Ed.) 35, 417–423. doi:10.3969/j.issn.1003-0972.2022.03.013
Meyer A. (1944). A study of the skin structure of Golden Delicious apples. J. Am. Soc. Hort. Sci. 45, 105–110.
Moore M. H., Edney K. L. (1959). “The timing of spray treatments for the control of storage rot of apple caused by Gloeosporium spp,” in Annual Report of the East Malling Research Station for 1958, 106–109.
Olsen K. (1965). A study of the biology of Gloeosporium album and G. perennans on apples. Meddelanden fran Statens vaxtsksyddanstalt Stockholm 13, 187–259.
Ruess F., Stosser R. (1993). Uber die dreidimensionale Rekonstruktion des Interzellularsystems von Apfelfruchten. Angew Bot. 67, 113–119.
Sato Y., Hirayama K., Toda T., Nara C., Furuya H. (2021). Previously unreported symptom of bull’s-eye rot (Kigusare-byo) on apple fruits caused by Phlyctema vagabunda Desm. Jap. J. Phytopath. 87, 133–145. doi: 10.3186/jjphytopath.87.133
Saudreau M., Marquier A., Adam B., Sinoquet H. (2011). Modelling fruit-temperature dynamics within apple tree crowns using virtual plants. Ann. Bot. 108, 1111–1120. doi: 10.1093/aob/mcr054
Singh V., Gamrasni D., Ben Arie R., Naschitz S., Friedman H. (2016). Identification of open lenticels in apples after harvest in relation to lenticel breakdown development during storage. Postharvest Biol. Technol. 121, 165–170. doi: 10.1016/j.postharvbio.2016.06.004
Spadaro D., Torres R., Errampalli D., Everett K., Ramos L., Mari M. (2020). “Chapter 2. Pome fruits,” in Postharvest Pathology of Fresh Horticultural Produce. Eds. Palou L., Smilanick J. L. (Boca Raton, Florida, USA: CRC Press), 55–110.
Spotts R. A. (1985). Environmental factors affecting conidial survival of five pear decay fungi. Plant Dis. 69, 391–392. doi: 10.1094/PD-69-391
Spotts R. A. (2014). “Postharvest diseases: bull’s-eye rot,” in Compendium of Apple and Pear Diseases, 2nd edition. Eds. Sutton T. B., Aldwinckle H. S., Agnello A. M., Walgenbach J. F. (St. Paul, MN: American Phytopathological Society), 78–79.
Spotts R. A., Cervantes L. A., Mielke E. A. (1999). Variability in postharvest decay among apple cultivars. Plant Dis. 83, 1051–1054. doi: 10.1094/PDIS.1999.83.11.1051
Keywords: Malus × domestica, Neofabraea, leaf wetness, wind run, postharvest, temperature, spore germination
Citation: Everett KR, Hasna L, Pushparajah IPS, Vergara MJ, Wood PN, Fisher BM, Sutherland PS, Richards KK and Husheer SW (2023) Lenticel hydration alters the susceptibility of apple fruit to infection by Phlyctema vagabunda. Front. Hortic. 2:1217352. doi: 10.3389/fhort.2023.1217352
Received: 05 May 2023; Accepted: 17 July 2023;
Published: 04 September 2023.
Edited by:
Marcel Wenneker, Wageningen University and Research, NetherlandsReviewed by:
Julia Christine Meitz-Hopkins, Stellenbosch University, South AfricaAlessandra Di Francesco, University of Udine, Italy
Copyright © 2023 Everett, Hasna, Pushparajah, Vergara, Wood, Fisher, Sutherland, Richards and Husheer. This is an open-access article distributed under the terms of the Creative Commons Attribution License (CC BY). The use, distribution or reproduction in other forums is permitted, provided the original author(s) and the copyright owner(s) are credited and that the original publication in this journal is cited, in accordance with accepted academic practice. No use, distribution or reproduction is permitted which does not comply with these terms.
*Correspondence: Kerry R. Everett, S2VycnkuRXZlcmV0dEBwbGFudGFuZGZvb2QuY28ubno=