- 1Canadian Forest Service, Laurentian Forestry Centre, Quebec City, QC, Canada
- 2Canadian Forest Service, Great Lakes Forestry Centre, Sault Ste. Marie, ON, Canada
- 3Canadian Forest Service, Atlantic Forestry Centre, Fredericton, NB, Canada
Surveillance for early detection of non-native, invasive pathogens requires simple, sturdy, and easy-to-use collecting devices. In this study, we compared the fungal species detected in wet collection cups of Lindgren traps vs. those detected on slides with oiled cheesecloth as aerial spore collectors. DNA was extracted and amplified from both using the primers ITS1F and gITS7, and Illumina sequencing was used for the metabarcoding of fungi present in samples. In 90 samples, there were 1,277 fungal operational taxonomic units (OTUs). For fungal OTUs only detected by one collection method, insect traps had three times the number of fungal OTUs compared to slides, and this pattern persisted when analyses were restricted to pathogens and forest pathogens. Annually, thousands of insect traps are deployed in North America and the associated trap fluids have added value to forest disease research and monitoring.
Introduction
Forest ecosystems provide a diverse number of goods (e.g., food, timber, and fuel) and services (e.g., biodiversity maintenance, carbon storage, nutrient cycling, and water and air purification) (Vira et al., 2015; Wingfield et al., 2015). With the growth in human populations, the significance of these goods and services, as well as disturbances that threaten the health of forest ecosystems, are expected to increase. Invasive insects and pathogens are one of the most significant threats to the health of forest ecosystems globally (Roy et al., 2014), and the movement of species outside of their native range is expected to continue to increase (Seebens et al., 2017).
Although the impacts of biological invasions are realized regionally, at least initially, their causes are primarily international (i.e., trade and transport pathways) and unintended (Westphal et al., 2008; Hulme, 2009). As a result, the emphasis of many national forest biosecurity programs is prevention (Allison et al., 2021). A large network of international and regional regulatory instruments has been developed to restrict the movement of species outside of their native ranges (Moore, 2005; Ormsby and Brenton-Rule, 2017). These regulatory instruments were designed to manage biosecurity threats in the area of origin, as well as in the invaded range (Ormsby and Brenton-Rule, 2017).
There is some evidence that biosecurity efforts have been successful. For example, Haack et al. (2014) estimated that from 2003 to 2009, International Standards for Phytosanitary Measures (ISPM) 15 contributed to 36 to 52% reduction in infestation rates of inspected wood packaging coming into the United States. Despite these efforts and their associated reductions in propagule pressure, levels of globalization and trade continue to increase and as a result, species continue to arrive and establish outside of their native ranges (Chapman et al., 2017; Seebens et al., 2017). For invasive species with significant ecological and economic impacts, eradication from the invaded range often provides a significant economic return on management efforts (Sharov and Liebhold, 1998; Myers et al., 2000; Liebhold and Tobin, 2008). Although eradication can be difficult to achieve, several examples of successful eradication programs exist, including against forest pests [e.g., Asian longhorned beetle (CFIA, 2020; Allison et al., 2021)].
One of the primary determinants of the success of eradication efforts is the size of the area infested and associated population densities, both of which often increase with time since establishment (Myers et al., 2000; Brockerhoff et al., 2010; Liebhold et al., 2016). The availability of sensitive, low-cost surveillance tools is critical for assessing population densities, delimitation of the exotic in the invaded range, and assessing the efficacy of management interventions. The success of containment and long-term control programs that attempt to limit the spread and population densities within the invaded range also depends on the availability of surveillance tools.
Although economic analyses indicate that the benefits of surveillance programs exceed their costs (Epanchin-Niell et al., 2014), surveillance programs are costly (Blackburn et al., 2016). In part, the large investment required for effective surveillance is driven by the fact that optimal sensitivity requires the use of multiple surveillance tactics (Yemshanov et al., 2014). This is because surveillance programs are often designed to target communities of species (the identity of which are often unknown a priori) and the performance of surveillance tools varies among taxa (e.g., Allison et al., 2014; Dodds et al., 2015; Allison and Redak, 2017). Several studies have demonstrated that intercept traps can be co-baited with lures that target different insect communities without significant reductions in efficacy for target insect taxa (e.g., Witzgall et al., 2010; Rassati et al., 2014; Chase et al., 2018; Marchioro et al., 2020). This pyramiding of attractants facilitates the use of one trap to simultaneously sample multiple species, significantly reducing labor and material costs.
Plant diseases are increasing in frequency in forest ecosystems (Desprez-Loustau et al., 2007; Santini et al., 2013). Many of these diseases are caused by exotic pathogenic fungi and oomycetes and have significant ecological and economic impacts in the invaded range (Desprez-Loustau et al., 2007; Wingfield et al., 2015; Ramsfield et al., 2016). Surveillance of plant pathogens and the diseases they cause is critical to the early detection of invasive species and changes in the population densities of naturalized and native pest species (Parnell et al., 2017). As with insect pests, once plant pathogens are established across a broad range and/or begin to experience rapid population growth, the likelihood of successful management is low. The importance of surveillance for forest pathogens is further increased by the fact that few control tactics are available to manage pathogens in forest ecosystems. Surveillance programs in forest ecosystems typically target pathogens or insects. This is primarily because the collectors for fungal spores cannot capture and retain insects; however, intercept traps used to survey insects can collect spores either through random alightment of spores into the trap collection reservoir or via the body of captured insects. This study aimed to compare the efficacy of passive spore collecting devices and insect intercept traps with wet collection cups for sampling fungal species. Secondarily, we tested the effect of site type (rural, urban) on abundance and the number of fungal species detected. We did not process the insects captured in the field trials because the use of intercept traps to survey forest insects is ubiquitous (Rabaglia et al., 2019; Allison et al., 2021) and further empirical work to promote their use in forest ecosystems is not needed.
Materials and methods
Insect traps and passive aerial spore collectors (described below) were deployed in wooded areas at one urban and one rural site located <15 km apart in each of three Canadian cities: Sault Ste. Marie, Ontario; Fredericton, New Brunswick (NB); and Halifax, Nova Scotia (NS) (Supplementary Table 1). The insect traps were black Lindgren 12-funnel traps (Synergy Semiochemical Corp., Burnaby, BC, Canada) with a surface area of 1,393 cm2 (McIntosh et al., 2001). The aerial spore collectors were developed using technology from the 1960s for their simplicity to fabricate and use by non-scientists, absence of maintenance requirements, and the need to protect them from theft and vandalism due to their low cost. Each consisted of Grade 50 double-layer cheesecloth (operational surface area 8.4 cm2) (Uline, Pleasant Prairie, WI, USA) impregnated with silicone oil #378399 (Sigma) at 60 g per m2 mounted in 35 mm projector slides. The slides were held in place vertically by an alligator clip 7 cm inside a 25.4 cm diam. airport wind indicator (Airport Windsock Corporation, Lake City, MN, USA) to protect it from rain that could dislodge captured spores, a problem highlighted in a study by Aguayo et al. (2018). The slides were positioned 2 m above the ground.
Within each city, urban sites were located relatively close (<2 km) to industrial parks, whereas rural sites were located 6 to 15 km distant from these areas. At each of the six sites, we set up two insect traps and two aerial spore collectors in a paired design with traps and aerial spore collectors <5 m apart within pairs and replicate pairs separated by about 30 m. Within each site, both the traps and the aerial spore collectors were deployed on the same dates and exposed for a total of 4 weeks. Preservative fluids and slides were collected weekly or biweekly (3–4 times) from 1 to 28 August 2018 in New Brunswick and Nova Scotia, and weekly from 16 August to 13 September 2018 in Ontario. The samples were shipped with ice packs and kept frozen at −20°C until extraction. A total of 46 slides and 44 trap preservative fluids were collected.
Funnel traps were suspended from a rope tied between two trees such that the trap was at least 1 m from the nearest tree and the collection cup was 30 to 50 cm above the ground, and remained up for the entire 4-week duration of the experiment. Traps were treated with 50% Fluon diluted in water to increase the efficiency of capturing wood-boring beetles (Allison et al., 2016). The collecting cups contained commercial RV antifreeze as preservative fluid (WinterProof Water System Antifreeze, 10 to 30% ethanol, 1 to 5% propylene glycol, Recochem Inc., Montreal, QC, Canada) with a drop or two of liquid dish detergent to reduce surface tension in the NB and NS sites. Each funnel trap was baited with four semiochemical lures known to increase captures of several species of bark and wood-boring beetles in the families Cerambycidae and Curculionidae (subfamily Scolytinae), that is, monochamol, ipsenol, alpha pinene, and ethanol (Allison et al., 2003; Miller et al., 2016; Boone et al., 2019; Flaherty et al., 2019).
Preservative fluids from each funnel trap collection (approximatively 250 ml) from Ontario were filtered on 25 mm diam. 2.7 μm pore size Glass Microfiber GF-D (Whatman, Buckinghamshire, UK) using vacuum, whereas Nova Scotia and New Brunswick fluids were filtered on 90 mm diam. Whatman #1 qualitative filter papers 11 μm pore size (Whatman, Maidstone, UK). Funnel trap filters and passive trap cheesecloths were sampled using a 6-mm paper punch. One 6-mm disk per filter and cheesecloth slide was ground using a Christison M3 Mixermill with a tungsten bead, two times for 2 min at 30 hertz with extraction buffer. Extraction was done with the Plant DNeasy mini kit (Qiagen Inc., Valencia, CA, USA) according to manufacturer instructions. One microliter of the eluate was used as a genomic DNA (gDNA) template for Polymerase Chain Reaction (PCR).
To obtain a comprehensive data set of all fungal DNA present in our samples, a High Throughput Sequencing (HTS) method based on the Illumina Miseq sequencing system was used. DNA amplification, primer constructs, purification, and sequencing were done as described in Bérubé et al. (2018). The eluted DNA (gDNA) was used as a template for polymerase chain reaction (PCR). ITS regions of the ribosomal DNA fragment (ITS1-5.8S) were first amplified in PCR-1 using the Illumina Fusion Primers containing the forward ITS1f primer which is fungal specific and the universal reverse gITS7 (Ihrmark et al., 2012) located in the 5.6s ribosomal ITS region. These target-specific primers were chosen because they amplify amplicons of approximately 300 bp, an ideal size for the Illumina paired-end technology. ITS is the recognized barcode gene for fungi (Schoch et al., 2012), despite its limitation to discriminating species in some genera. Primers flanking the ribosomal gene can be universal, kingdom, or phylum specific, allowing amplification of a vast number of species simultaneously, from diverse taxonomic levels. The amplicons obtained were then re-amplified in PCR-2 with Illumina Universal Indexed Primers which contained an index sequence for tagging every sequence to a sample. Fifteen forward-indexed sequences were used in combination with 15 reverse-indexed sequences to provide 225 indexed combinations.
As our two spore collecting devices also caught an array of heterogeneous material, such as plant debris, dust, and pollen, often in greater amounts than fungal spores, DNA quantification was done only after the two PCR steps. Each of the 90 samples was tagged with differing indices, PCR amplified separately and then tagged amplicons were pooled in equimolar amounts of 4 ng of DNA per sample. Final quantification, primer dimer removal, and amplicon quality check were done with an Agilent 2100 BioAnalyzer (Agilent Technologies, Santa Clara, CA, USA). Pooled DNA samples were sent to the Next-Generation Sequencing Platform, Genomics Center, CHU de Québec-Université Laval Research Center, Quebec City, QC, Canada, which performed paired-end 300 bp sequencing using MiSeq Reagent Kit v3 (600 cycles) through an Illumina MiSeq system.
A bioinformatics treatment of HTS DNA sequences was executed to create Operational Taxonomic Units (OTUs), our proxies for fungal species (Huse et al., 2010; Kunin et al., 2010). Sequence analysis was done as described in Bérubé et al. (2018). To minimize the loss of rare and targeted fungal species, standard bioinformatic tools, such as QIIME2 and DADA2, were not used. Instead, a custom bioinformatic pipeline that minimizes losses of singletons and rare OTUs was used (Gagné et al., 2020). In brief, sequence assembly was done using PANDASeq version 2.7 (Masella et al., 2012). Sequences were then filtered and trimmed with Illumicut (Gagné and Bérubé, 2017a), sequences with homopolymer >9 bp were removed with HomopRemover (Gagné and Bérubé, 2017b), and reads <120 bp were discarded. Dereplication on the full length of the set of sequences was performed before the construction of clusters with MOTHUR v.1.28.0 (Schloss et al., 2009). While not recommended by some authors (Nilsson et al., 2008; Lindahl et al., 2013), all singletons passing the quality control step were kept for analysis because the assignment of singletons as a sequencing artifact is not always justified after reads processing (Lentendu et al., 2011; Penton et al., 2013) and could lead to a loss from the data set of new emerging pathogens found at very low frequencies. The set of sequences was then organized into clusters with USEARCH 64-bit version 8.0.1623 (Edgar, 2010), with a sequence similarity threshold of 97%, to agglomerate reads and form the OTUs, with the most abundant sequence type serving as cluster seeds. Representative sequences, which are the most frequent sequence in each OTU, were extracted and then screened against Genbank nr/nt database using BLAST to identify rare and potentially new emerging invasive fungal species not found in curated databases. Output Excel files were then organized alphabetically by Latin names and parsed for plant pathogens of interest and those on the quarantine species list of Canada and other industrialized countries.
Cross-talk is a phenomenon that occurs when a DNA read is assigned to an incorrect sample (Edgar, 2018). When dealing with experiments attempting to identify emerging pests, incorrect assignment of DNA reads can lead to troublesome conclusions about its presence and distribution in a region or country where it is not yet present. To alleviate this problem, positive controls of targeted pests were not used in this trial to avoid cross-talk contaminations. One index in our study for which no amplicons were produced was also sent as a sequencing blank during the sequencing output processing to quantify cross-talk.
Statistical analysis
The primary output Excel file was compressed according to the Genbank accession number to fuse redundant OTUs created by our bioinformatic method. OTUs that were ≥97% similar were pooled. Data for a weekly sample with less than 10 reads were converted to zero for all OTUs. We then generated manually three groupings of OTUs for analysis based on Tedersoo et al. (2014): (1) forest pathogens, (2) plant pathogens (which included forest pathogens as well as non-forest plant pathogens); and (3) all fungal OTUs (including plant pathogens). DNA read counts in the weekly samples were summed over the entire 4-week sampling period to yield a balanced data set with one cumulative count of each OTU for each collection method, site type, site and replicate, for a total of 24 aggregate samples for statistical analysis (Supplementary Table 1).
Because the pore diameter of filter papers used to filter trap fluids was larger in New Brunswick and Nova Scotia (11 μm) than in Ontario (2.7 μm), some fungal species (i.e., with spores <11 μm diameter) present in trap fluids in New Brunswick and Nova Scotia may have been missed. If that were true, we would expect the relative performance of trap fluids vs. aerial spore collectors to be greater in Ontario than the other two sites. To test this, we ran separate 2 × 2 contingency table analyses on the proportions of fungal species detected by trap fluids vs. aerial spore collectors at each of the three sites, as well as on data pooled from all sites, and then tested for differences in performance among sites using the Chi-square heterogeneity test (Zar, 1999, pp. 500–502). We ran separate analyses on each of our three groups of fungi, that is, all fungi, plant pathogens, and forest pathogens.
We then ran generalized linear mixed-effect models (PROC GLIMMIX) in SAS 9.4 for Windows (version 6.2.9200; ©2002–2012, SAS Institute Inc., Cary, NC, USA) to test the effects of the collection method (aerial spore collectors vs. insect trapping solution), site type (forest vs. urban), collection method x site type interaction, and site (Sault Ste. Marie, Fredericton, Halifax) on species richness and abundance of forest pathogens, plant pathogens, and all fungal species, and abundance of the more common individual forest pathogens. Collection method and site type were considered fixed effects and sites were considered random effects. We ran the models with Gaussian (on both raw and log (y+1) transformed data), Poisson, and negative binomial distributions and report results from the model with the best fit (negative binomial in all cases) as determined by the lowest value of the corrected Akaike Information Criterion (AICc). Least-square means were compared using the Tukey-Kramer method with experiment-wise error controlled at α = 0.05, but means and standard errors are reported on raw (species richness) or log(y+1) transformed data (abundance). Of the total 101 forest pathogens, only 25 of the most abundant in our samples were analyzed by generalized linear mixed models to test for effects of collection method and site type on their abundance; high numbers of zero counts in the remaining OTUs made them inappropriate for analysis by generalized linear models.
We used non-metric multidimensional scaling (NMS) with the Sorensen-Bray Curtis (city block) distance measure (PC-ORD for Windows; McCune and Mefford, 2016) to compare the relative abundance of fungal OTUs collected in aerial spore collectors vs. Lindgren funnel trap solution. We also used NMS to compare the communities between site types (urban vs. rural) and among the three sites. We ran three separate analyses for all fungi, plant pathogens, and forest pathogens. Before analysis, we deleted singletons and doubletons and transformed the data by log(y+1) to reduce skewness, resulting in totals of 809, 157, and 70 OTUs (i.e., columns) and 24 samples in the matrices for all fungi, plant pathogens, and forest pathogens, respectively. We first ran NMS on “autopilot” with 499 iterations to determine the optimum number of dimensions (i.e., stress level vs. number of axes); we then re-ran NMS using the recommended number of axes and 250 runs to obtain the final stress level and results from the randomization test. The latter tests the null hypothesis of no redundant pattern of covariance in the data; p < 0.05 indicates a real pattern in the data. The initial NMS runs and stress tests recommended two dimensions (axes) for all fungi and three dimensions for plant pathogens and forest pathogens (Supplementary Figures 1A–C). We also used PERMANOVA (PC-ORD for Windows; McCune and Mefford, 2016) to test the effect of collection method (fixed) and site (random) on the relative abundance of forest pathogens, plant pathogens, and all fungi detected, and indicator species analysis (Dufrêne and Legendre, 1997) to identify species of plant pathogens that were more abundant and consistently associated with either trap solutions or aerial spore collectors.
Results
We detected a total of 1,277 fungal OTUs, 220 of which were plant pathogens, and 101 of these were forest pathogens (Table 1). Trap fluids detected 90% of all fungi collected by both methods including 476 OTUs that were not detected by aerial spore collectors (Table 1; Figure 1A). Aerial spore collectors detected 63% of total fungi collected, including 132 OTUs not detected in trap fluids; 669 OTUs were detected by both methods (Table 1; Figure 1A). Sixty-two of 220 plant pathogens were detected exclusively by trap fluids compared to only 15 plant pathogens detected exclusively by aerial spore collectors (Figure 1B). Of the 101 forest pathogens, 27 were detected exclusively by trap fluids and 6 exclusively by aerial spore collectors (Figure 1C).
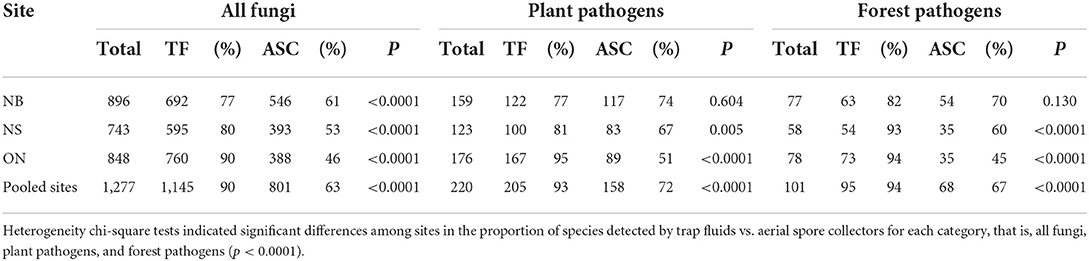
Table 1. Number (%) of OTUs of all fungi, plant pathogens, and forest pathogens detected at each site by trap fluids (TF) vs. aerial spore collectors (ASC).
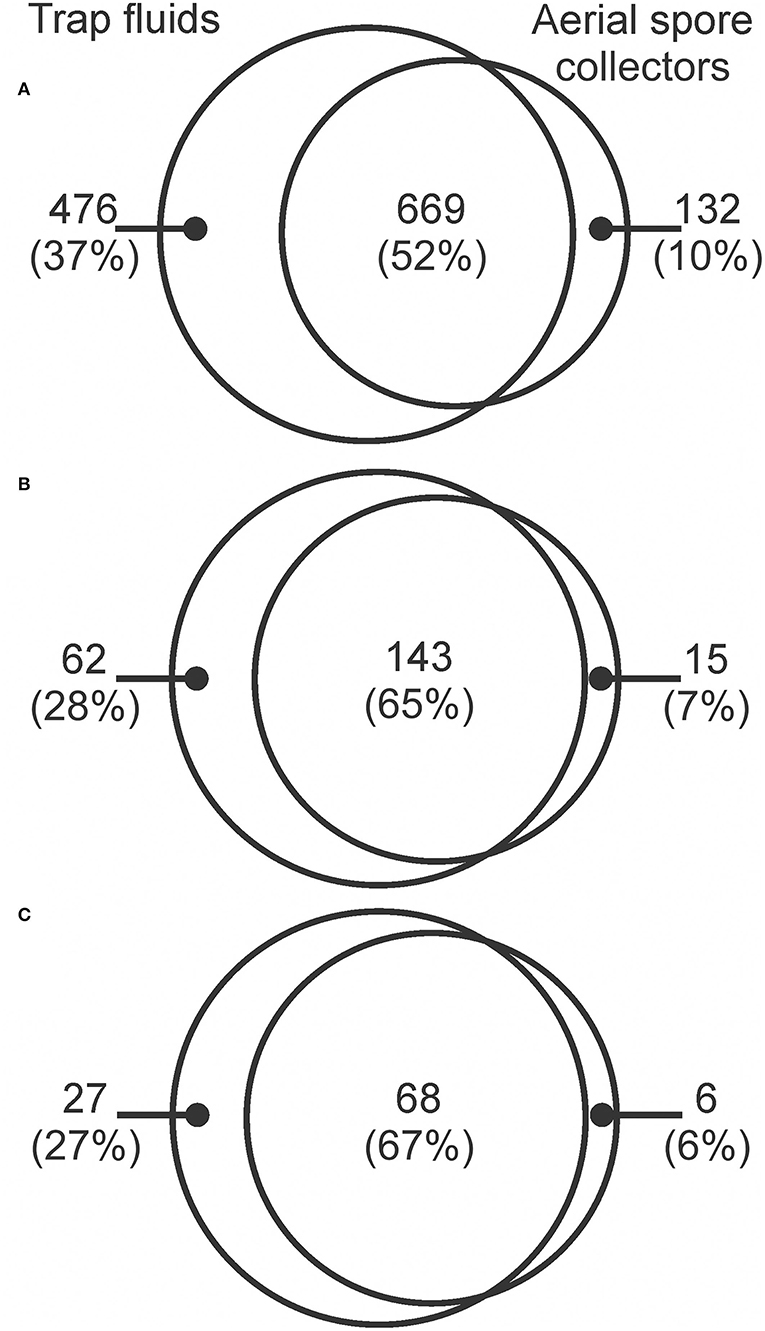
Figure 1. Venn diagrams showing the numbers of fungal OTUs detected by trap fluids and aerial spore collectors in 2018 at the forest and urban sites in New Brunswick, Nova Scotia, and Ontario (data pooled from all sites) of (A) total fungi; (B) plant pathogens; and (C) forest pathogens.
At each of the three sites, a significantly greater proportion of fungi were detected by trap fluids (77–90%) than by aerial spore collectors (46–61%) (p < 0.0001, Fisher Exact test) (Table 1). Trap fluids also outperformed aerial spore collectors in proportions of plant pathogens and forest pathogens detected in Nova Scotia and Ontario (p < 0.005) but not in New Brunswick (p ≥ 0.13) (Table 1). There was significant heterogeneity among sites in the proportion of species detected by trap fluids vs. aerial spore collectors for total fungi (χ2 = 297, p < 0.0001), plant pathogens (χ2 = 59, p < 0.0001), and forest pathogens (χ2 = 27, p < 0.0001), indicating the relative performance of collection methods varied among sites.
Species richness of fungi and pathogens
Collection method significantly affected the number of forest pathogens (F1, 18 = 21.9, p = 0.0002), plant pathogens (F1, 18 = 17.3, p = 0.0006), and total fungi (F1, 18 = 32.5, p < 0.0001) detected, with trap fluids detecting 1.6 to 1.8 as many species as aerial spore collectors (Figures 2A–C; Supplementary Table 2). Species richness of forest pathogens, plant pathogens, and total fungi were not significantly affected by site type (F1, 18 ≤ 3.25, p ≥ 0.09) nor by the interaction between site type and collection method (F2, 18 ≤ 1.51, p ≥ 0.23).
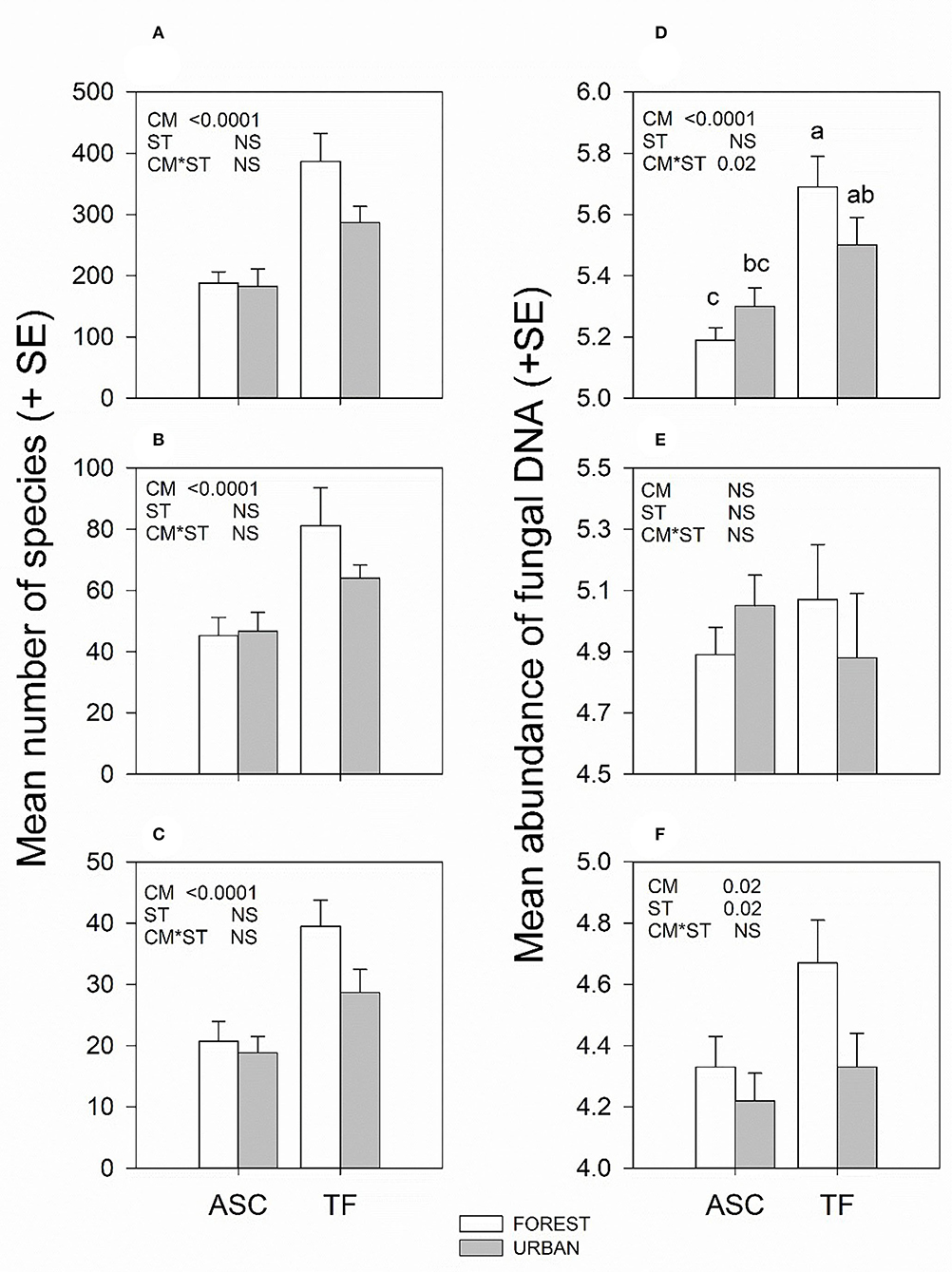
Figure 2. Mean (+SE) species richness (A–C) and mean (+SE) abundance of DNA (D–F) of all fungi (top), plant pathogens (middle), and forest pathogens (bottom) collected by aerial spore collectors (ASC) vs. trap fluids (TF) in forest and urban sites in New Brunswick, Nova Scotia, and Ontario, during a 4-week period in August to September 2018. Significance of collection method (CM), site type (ST), and their interaction (CM*ST) from generalized linear mixed models. Tukey test on least square means was performed only on mean abundance of fungi for collection method-site type combinations (D) due to the significant interaction; means followed by different letters were significantly different (p ≤ 0.05).
Abundance of fungi and pathogens
Collection method also significantly affected the abundance of forest pathogens (F1, 18 = 7.02, p < 0.016) and total fungi (F1, 18 = 34.6, p < 0.0001) with trap fluids outperforming aerial spore slide collectors (Figures 2D,F, Supplementary Table 2). However, there was a significant interaction between collection method and site type for total fungal abundance (F2, 18 = 6.48, p = 0.02); the mean abundance of fungi in trap fluids was greater than that in aerial spore collectors in rural sites but not urban sites (Figure 2F). Site type also affected the abundance of forest pathogens (F1, 18 = 7.13, p = 0.016) with more detected in rural than urban sites (Figure 2D). However, abundance of plant pathogens was not significantly affected by collection method (F1, 18 = 0.15, p = 0.70), site type (F1, 18 = 0.04, p = 0.83), or their interaction (F1, 18 = 2.17, p = 0.16) (Figure 2E).
There was a significant effect of the collection method on the abundance of 13 of 25 species of forest pathogens analyzed (F1, 18 ≥ 4.89, p ≤ 0.04) (Supplementary Table 3). Trap fluids detected more DNA than aerial spore collectors did for 11 of 13 forest pathogens, whereas the reverse was true for 2 of 13 forest pathogens (Table 2). Site type significantly affected the abundance of four forest pathogens (F1, 18 ≥ 4.43, p ≤ 0.05) and in each case, more DNA was detected in the rural site than in the urban site (Table 2).
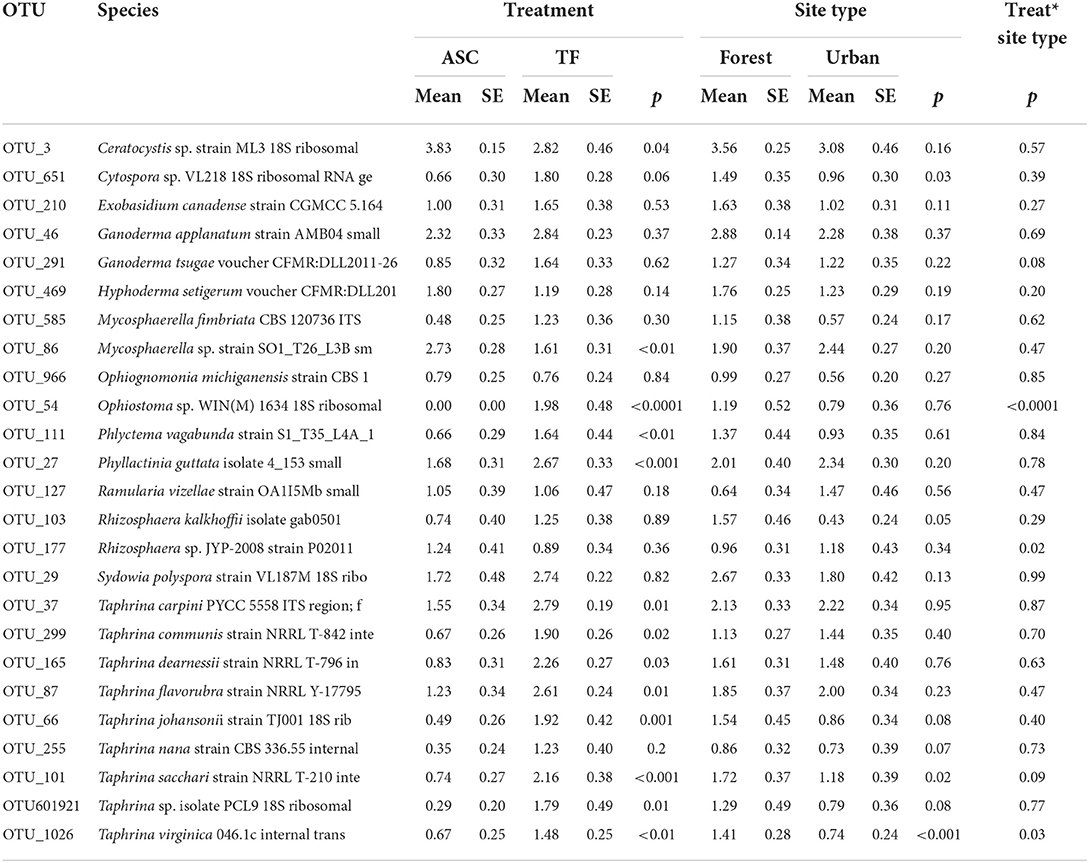
Table 2. Mean (±SE) abundance (DNA reads, log-transformed) of 25 of the 101 forest pathogens captured in aerial spore collectors (ASC) vs. Lindgren funnel trap fluids (TF), in forest vs. urban sites in New Brunswick, Nova Scotia, and Ontario, in 2018.
Effect of collection method, site type, and site on relative abundance of fungal species
The NMS analysis revealed obvious differences in fungal species composition and relative abundance in traps vs. aerial spore collectors (p < 0.01; randomization tests) for all fungi (2-dimensional solution, final stress = 13.534; R2n = 0.982), plant pathogens (3-dimensional solution, final stress = 9.961, R2n = 0.990), and forest pathogens (3-dimensional solution, final stress = 12.632; R2n = 0.984). For ease of the interpretation, we present results in two-dimensional plots (Figures 3A–C, axes 2 vs. 1; Supplementary Figures 2A–D, axes 3 vs. 1 and 3 vs. 2). Conversely, there was considerable overlap in the fungal species communities collected in urban vs. rural sites (Figures 3D–F, Supplementary Figures 3A–D). When we ran NMS with the site as the explanatory variable, the fungal community at the Nova Scotia site clearly separated from those of New Brunswick and Ontario (Supplementary Figures 4A–G). The PERMANOVA indicated significant effects of collection method and site on the communities of forest pathogens, plant pathogens, and all fungi detected (p < 0.05); the interaction between collection method and site was significant only for plant pathogens (Table 3). Indicator species analysis identified 30 species of plant pathogens that were significantly associated with trap solutions and only six species significantly associated with aerial spore collectors (Table 4).
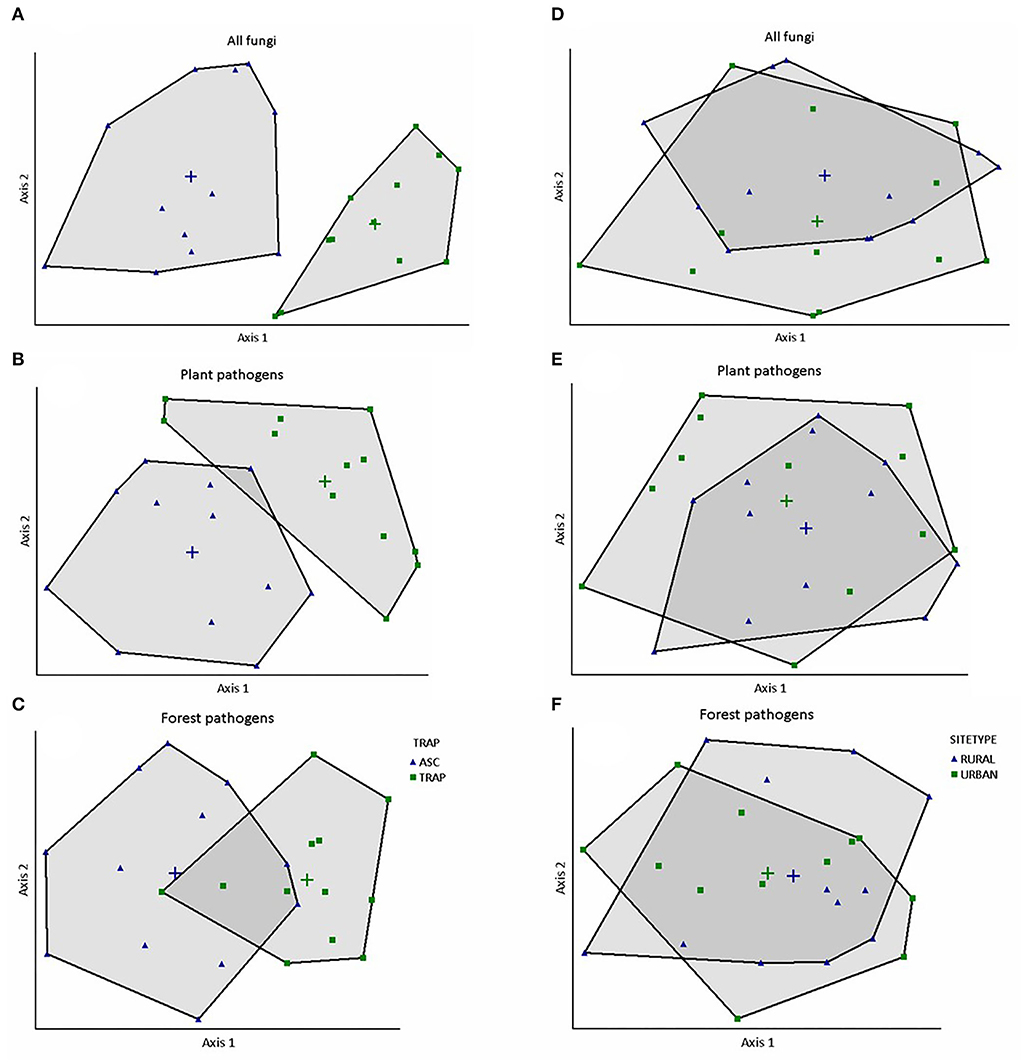
Figure 3. Nonmetric multidimensional scaling ordination shows that the relative abundance of fungal species collected in aerial spore collectors (ASC) differed (i.e., little or no overlap) from those collected in Lindgren funnel traps (TRAPS) for (A) all fungi (809 OTUs), (B) plant pathogens (157 OTUs), and (C) forest pathogens (70 OTUs). Conversely, fungal species composition in urban vs. rural sites was quite similar for (D) all fungi, (E) plant pathogens, and (F) forest pathogens. Each point in each graph represents 1 of 24 total samples.
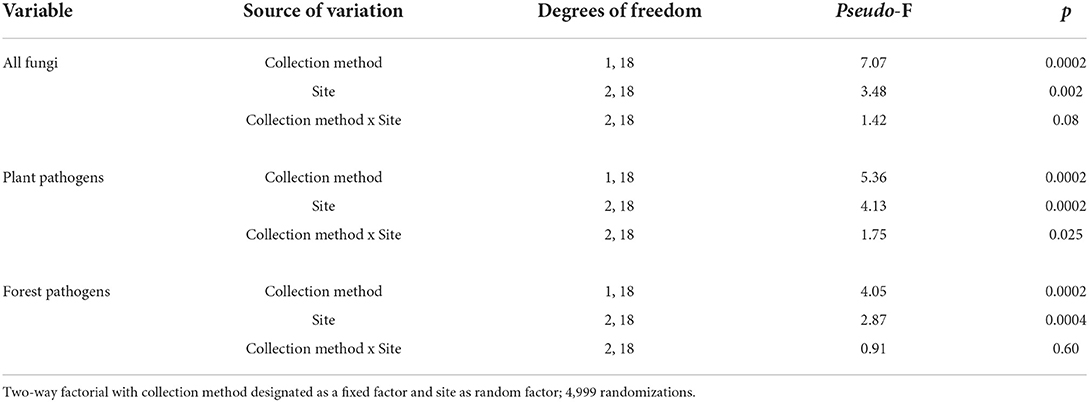
Table 3. Results of PERMANOVA (in PC-ORD) testing the effect of collection method (aerial spore collectors vs. Lindgren funnel traps) and site (New Brunswick, Nova Scotia, Ontario) on the community and relative abundance of fungal species detected.
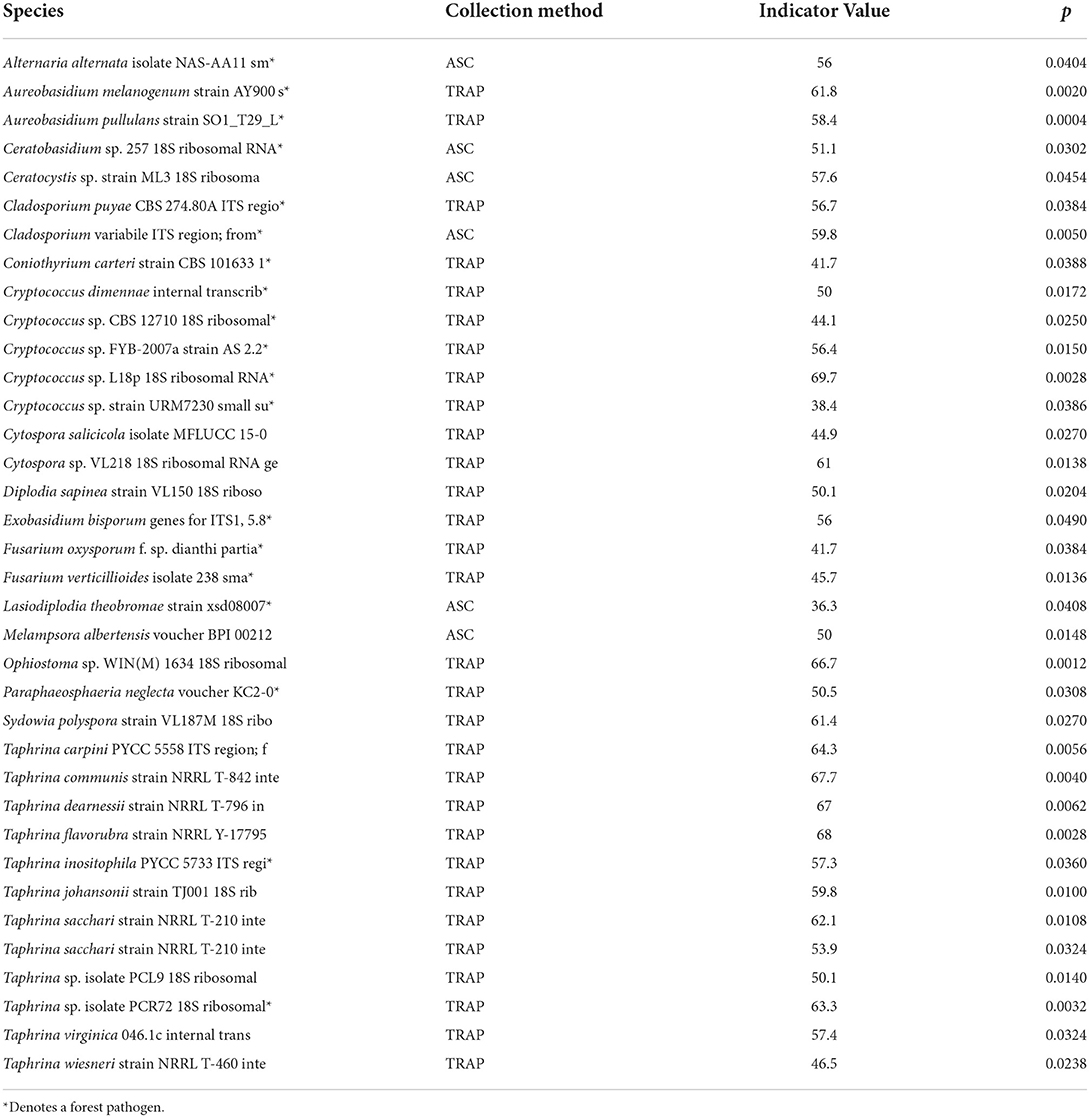
Table 4. Results of indicator species analysis show the species of plant pathogenic fungi are significantly more abundant and consistently associated with either aerial spore collectors or Lindgren funnel traps.
Discussion
Two of the biggest threats to the health of forest ecosystems are invasive species (primarily pathogens and insects) and climate change (Trumbore et al., 2015). Although the impacts of climate change on biological invasions can be difficult to predict (Ramsfield et al., 2016), it is clear that climate change has resulted in altered distributions for many taxa (Parmesan and Yohe, 2003; Chen et al., 2011; Pecl et al., 2017) and can negatively affect tree resistance (Jactel et al., 2012). As a result, increased global trade and anthropogenic effects on global climate will increase the need for biosecurity programs. Surveillance of forest pests for early detection of invasives is an important component of forest biosecurity but is costly and most programs target insects and pathogens independently. This study has demonstrated that it is possible to simultaneously sample fungal and insect communities in forest ecosystems by the complementary metabarcoding of fungal spores collected in traps primarily deployed for surveillance of non-native bark- and wood-boring insects. In fact, this study found that of the 1,277 fungal OTUs detected, approximately 50% were detected in both aerial spore detectors and insect trapping fluids, and 40% were detected only in trapping fluids compared to 10% that were detected only by spore collectors. Similar patterns were observed when comparisons were restricted to plant pathogen and forest plant pathogen species. The ability to use one trap to simultaneously sample for forest insects and pathogens without a loss in sensitivity represents a major programmatic advance. However, we do note that the fungal species assemblage collected in aerial spore collectors differed from that collected in trap solutions, so sole reliance on the latter method may be less effective at detecting certain fungal pathogens. Indeed, it is likely that some airborne fungal spores were not collected by either the aerial spore collectors or the funnel traps as no sampling method is capable of detecting all spore types present. We also emphasize that we cannot relate the fungal genera we collected on our passive aerial spore collectors or funnel traps to actual concentrations of airborne spores because we did not use a volumetric sampling device, such as Burkard spore trap (Hirst, 1952) or rotorod spore trap (Edmonds, 1972).
Many insect surveillance programs use intercept traps with wet cups (e.g., Rabaglia et al., 2019) and the preservative fluid in these traps has the potential for the surveillance of fungal spores. The idea of using preservative trap fluids for metabarcoding of invertebrates is not new (Zizka et al., 2019) and fungal species have previously been detected in insect trapping fluids (Tremblay et al., 2018). Insect trap fluids have also been used to characterize biodiversity associated with insects (e.g., bacteria, Linard et al., 2016). Similarly, other studies used crushed insect specimens to extract bacterial DNA for metabarcoding (Malacrino et al., 2017; Ravenscraft et al., 2019). Many metabarcoding studies have used classical aerial spore collecting devices, such as active rotating-arm devices (Botella et al., 2019), sticky Ionic traps (Redondo et al., 2020), Hirst-type spore traps (Dananché et al., 2017; Aguayo et al., 2021), Burkard volumetric spore traps (Nicolaisen et al., 2017), or passive collecting devices such as rainwater traps (Castaño et al., 2019; Crandall et al., 2020). This is the first comprehensive study to compare insect trap fluids and classical spore collecting devices.
There are at least two possible reasons why a greater number of fungal species were observed in trap fluids than in the slide aerial spore collectors. The first could be the difference in trapping surface area. The funnel traps had more than 200 fold the silhouette surface area (1,393 cm2) (McIntosh et al., 2001) for intercepting wind-borne spores than the slide aerial spore collectors (8.4 cm2). Second, semiochemical-baited traps actively attract many beetle species (e.g., Monochamus spp., Ips spp., Dendroctonus spp.; Allison et al., 2012; Flaherty et al., 2019), which carry fungal spores, including potentially damaging Ophiostomatales blue stain fungi (Yamaoka et al., 1997; Jacobs et al., 2003; Jankowiak et al., 2007; Kirisits, 2007; Min et al., 2009), and aerial spore collecting is a passive process. If the higher fungal diversity in trap fluids vs. aerial spore collectors is due to the insects captured, then the species more abundant on spore collectors likely do not rely on insect vectors for dispersal. Indicator species analysis suggested that 30 species of plant pathogenic fungi were relatively more abundant and consistently associated with trap fluids, whereas only six species were associated with aerial spore collectors. Of the species more common in aerial spore collectors, at least one (Ceratocystis sp.) comes from a genus known to be vectored by insects. While some of the 30 species that were more common in the trapped fluid are known to be vectored by insects (e.g., Ophiostoma sp.), some are not (Table 4), suggesting that multiple mechanisms likely explain the treatment effects observed in this study.
Site type had no effect on species richness or species assemblages of forest pathogens, plant pathogens, or total fungi and affected only the abundance of forest pathogens detected, with more detected at rural than urban sites. By contrast, Rassati et al. (2015) found more species of bark- and wood-boring beetles in traps that were placed in forests surrounding ports compared to traps placed directly inside ports and showed that species richness was positively related to the proportion of forest cover in the surrounding landscape. The minor effects of site type on fungal pathogens detected in our study may be due in part to the fact that both urban and rural sites were forested areas, so the proportion of forest cover (though not quantified) surrounding our traps was qualitatively similar at both site types.
The contingency table analysis indicated that the relative performance of trap solution vs. slide collectors for detecting species of plant pathogens was greater in Ontario than in New Brunswick and Nova Scotia. This was likely due in part to the smaller pore size of filters used to strain the trap solution in Ontario (2.7 μm) than in New Brunswick and Nova Scotia (11 μm). For example, the ellipsoidal spores of Cladosporium cladosporioides, which measure 3 to 7 μm long and 2 to 4 μm wide (Reponen et al., 2001), were detected with all aerial spore collector samples at all three sites and in all trap solution samples from Ontario but in only two of 12 and two of 16 trap solution samples from Nova Scotia and New Brunswick, respectively. The larger diameter filter paper (90 mm) used to filter trap solution in Nova Scotia and New Brunswick likely resulted in greater dilution of spores per unit surface area compared to samples from Ontario where smaller diameter (25 mm) filters were used. This may also have contributed to the greater relative performance of trap solutions vs. slide collectors in Ontario compared to New Brunswick and Nova Scotia. Our results suggest that the smaller pore size filters should be used in the future to reduce the risk of missing fungal pathogens that may be present in trap solutions. Future studies should explore this question further by straining trap solution samples sequentially through filters of decreasing pore size to determine which species are missed by the coarser filters.
Cross-talk associated with High Throughput Sequencing can be troublesome, inducing nearly 1% of DNA reads to be incorrectly assigned to a sample (Edgar, 2018). When dealing with a moving front of large populations of an invasive pest, cross-talk could lead to incorrect conclusions about actual distribution in the invaded range. However, when determining the range of a recently introduced pest with an extremely small number of individuals, the probability that cross-talk affects that species' DNA read assignment is very small, indeed less than 1% of reads from any OTUs in our studies were subjected to cross-talk. We did not use the metabarcoding pipeline DADA2 and as a result, a proportion of OTUs we detected may in fact be artifacts (Pauvert et al., 2019). However, we used the same size samples and bioinformatic methods on samples from traps and aerial spore collectors, so although the absolute number of OTUs detected by either collection device may have been overestimated, it does not change the fact that trap fluids detected relatively more fungal OTUs, plant pathogens, and forest pathogens than did the aerial slide collectors.
The results of this study suggest that insect trap fluids have excellent potential for the detection of non-native forest pathogens compared to passive aerial spore collectors. This is likely due to two factors: the common association of many forest fungal pathogens with bark- and wood-boring beetles (Krokene and Solheim, 1998; Six and Wingfield, 2011), and the diversity of beetle species captured in semiochemical-baited traps used for surveillance in biosecurity programs (Rabaglia et al., 2019). Annually, thousands of wet-cup traps are deployed in North America for monitoring and early detection of potentially invasive forest insect pests, and trap fluids are typically discarded (Rabaglia et al., 2019; John Crowe, USDA Animal and Plant Health Inspection Service, personal communication; Troy Kimoto, Canadian Food Inspection Agency, personal communication). The results of this study suggest that surveillance programs could gain additional useful information about fungal communities by screening insect trap fluids. However, further work is required to compare their performance with active high-volume air sampling devices because passive fungal spore collecting methods are less efficient than active volumetric methods (Chen et al., 2018). Future studies should also: (1) test the method at additional sites; (2) test whether the efficacy of fungal pathogen detection is affected by the type of liquid preservative used; (3) compare relative abundance and species assemblages of fungal species with those of insect target taxa (e.g., bark- and wood-boring beetles) collected in the same traps and at different heights above ground; and (4) conduct detailed comparisons of fungal communities sampled by different survey tools to determine which tools are optimal for which taxa. This research could also promote closer connections between entomologists and pathologists involved in forest health research and monitoring, as recently advocated by Jactel et al. (2020).
Data availability statement
The raw data supporting the conclusions of this article will be made available by the authors, without undue reservation. Genbank Accession number: PRJNA875096.
Author contributions
JB, JA, and JS conceived the project, secured funding, and wrote the manuscript. JA, IO, KV, CH, and JS collected and processed trap samples. JB and PG conducted molecular analyses. JS conducted statistical analyses. All authors contributed to the article and approved the final draft.
Funding
Funding for this project was provided by the Canadian Forest Service.
Acknowledgments
We thank Garrett Brodersen and Jacob Palmer for assistance in the field and Jeri Peck for helpful advice on the use of PC-ORD for community analyses.
Conflict of interest
The authors declare that the research was conducted in the absence of any commercial or financial relationships that could be construed as a potential conflict of interest.
Publisher's note
All claims expressed in this article are solely those of the authors and do not necessarily represent those of their affiliated organizations, or those of the publisher, the editors and the reviewers. Any product that may be evaluated in this article, or claim that may be made by its manufacturer, is not guaranteed or endorsed by the publisher.
Supplementary material
The Supplementary Material for this article can be found online at: https://www.frontiersin.org/articles/10.3389/ffgc.2022.953130/full#supplementary-material
References
Aguayo, J., Fourrier-Jeandel, C., Husson, C., and Ioos, R. (2018). Assessment of passive traps combined with high-throughput sequencing to study airborne fungal communities. Appl. Environ. Microbiol. 84, e02637–e02617. doi: 10.1128/AEM.02637-17
Aguayo, J., Husson, C., Chancerel, E., Fabreguettes, O., Chandelier, A., Fourrier-Jeandel, C., et al. (2021). Combining permanent aerobiological networks and molecular analyses for large-scale surveillance of forest fungal pathogens: A proof-of-concept. Plant Pathol. 70, 181–194. doi: 10.1111/ppa.13265
Allison, J. D., Bhandari, B. D., McKenney, J. L., and Millar, J. G. (2014). Design factors that influence the performance of flight intercept traps for the capture of longhorned beetles (Coleoptera: Cerambycidae) from the subfamilies Lamiinae and Cerambycinae. PLos ONE 9, e93203. doi: 10.1371/journal.pone.0093203
Allison, J. D., Graham, E. E., Poland, T. M., and Strom, B. L. (2016). Dilution of Fluon before trap surface treatment has no effect on longhorned beetle (Coleoptera: Cerambycidae) captures. J. Econ. Entomol. 109, 1215–1219. doi: 10.1093/jee/tow081
Allison, J. D., Marcotte, M., Noseworthy, M., and Ramsfield, T. (2021). Forest biosecurity in Canada – An integrated multi-agency approach. Front. For. Glob. Change 4, 700825. doi: 10.3389/ffgc.2021.700825
Allison, J. D., McKenney, J. L., Millar, J. G., McElfresh, J. S., Mitchell, R. F., and Hanks, L. M. (2012). Response of the woodborers Monochamus carolinensis and Monochamus titillator (Coleoptera: Cerambycidae) to known cerambycid pheromones in the presence and absence of the host plant volatile α-pinene. Environ. Entomol. 41, 1587–1596. doi: 10.1603/EN12185
Allison, J. D., Morewood, W. D., Borden, J. H., Hein, K. E., and Wilson, I. M. (2003). Differential bio-activity of Ips and Dendroctonus (Coleoptera: Scolytidae) pheromone components for Monochamus clamator and M. scutellatus (Coleoptera: Cerambycidae). Environ. Entomol. 32, 23–30. doi: 10.1603/0046-225X-32.1.23
Allison, J. D., and Redak, R. A. (2017). The impact of trap type and design features on survey and detection of bark and woodboring beetles and their associates: A review and meta-analysis. Ann. Rev. Entomol. 62, 127–146. doi: 10.1146/annurev-ento-010715-023516
Bérubé, J. A, Gagné, P. N, Ponchart, J. P., Tremblay, E. D., and Bilodeau, G. J. (2018). Detection of Diplodia corticola spores in Ontario and Québec based on high throughput sequencing (HTS) methods. Can. J. Plant Pathol. 40, 378–386. doi: 10.1080/07060661.2018.1498394
Blackburn, L., Epanchin-Niell, R., Thompson, A., and Liebhold, A. (2016). Predicting costs of alien species surveillance across varying transportation networks. J. Appl. Ecol. 54, 225–233. doi: 10.1111/1365-2664.12754
Boone, C. K., Sweeney, J., Silk, P., Hughes, C., Webster, R. P., Stephen, F., et al. (2019). Monochamus species from different continents can be effectively detected with the same trapping protocol. J. Pest Sci. 92, 3–11. doi: 10.1007/s10340-018-0954-4
Botella, L., Bačov,á, A, Dvorák, M., Kudláček, T., Pepori, A. L., Santini, A., et al. (2019). Detection and quantification of the air inoculum of Caliciopsis pinea in a plantation of Pinus radiata in Italy. iForest-Biogeosci. Forest. 12, 193. doi: 10.3832/ifor2866-012
Brockerhoff, E. G., Liebhold, A. M., Richardson, B., and Suckling, D. M. (2010). Eradication of invasive forest insects: concepts, methods, costs and benefits. N.Z. J. For. Sc. 40, S117–S135. Available online at: https://www.nrs.fs.fed.us/pubs/34736 (accessed May 14 2022).
Castaño, C., Bonet, J. A., Oliva, J., Farré G., de Aragón, J. M., Parladé J., et al. (2019). Rainfall homogenizes while fruiting increases diversity of spore deposition in Mediterranean conditions. Fungal Ecol. 41, 279–288. doi: 10.1016/j.funeco.2019.07.007
CFIA (2020). Asian longhorned beetle declared eradicated in the cities of Mississauga and Toronto. Available online at: https://www.canada.ca/en/food-inspection-agency/news/2020/06/asian-longhorned-beetle-declared-eradicated-in-the-cities-of-mississauga-and-toronto.html (accessed May 14, 2022).
Chapman, D., Purse, B. V., Roy, H. E., and Bullock, J. M. (2017). Global trade networks determine the distribution of invasive non-native species. Glob. Ecol. Biogeog. 26, 907–917. doi: 10.1111/geb.12599
Chase, K. D., Stringer, L. D., Butler, R. C., Liebhold, A. M., Miller, D. R., Shearer, P. W., et al. (2018). Multiple-lure surveillance trapping for Ips bark beetles, Monochamus longhorn beetles, and Halyomorpha halys (Hemiptera: Pentatomidae). J. Econ. Entomol. 111, 2255–2263. doi: 10.1093/jee/toy190
Chen, I. C., Hill, J. K., Ohlemüller, R., Roy, D. B., and Thomas, C. D. (2011). Rapid range shifts of species associated with high levels of climate warming. Science 333, 1024–1026. doi: 10.1126/science.1206432
Chen, W., Hambleton, S., Seifert, K. A., Carisse, O., Diarra, M. S., Peters, R. D., et al. (2018). Assessing performance of spore samplers in monitoring Aeromycobiota and fungal plant pathogen diversity in Canada. Appl. Environ. Microbiol. 84, e02601–e02617. doi: 10.1128/AEM.02601-17
Crandall, S. G., Saarman, N., and Gilbert, G. S. (2020). Fungal spore diversity, community structure, and traits across a vegetation mosaic. Fungal Ecol. 45, 100920. doi: 10.1016/j.funeco.2020.100920
Dananché, C, Gustin, M. P., Cassier, P., Loeffert, S. T., Thibaudon, M., Bénet, T., et al. (2017). Evaluation of hirst-type spore trap to monitor environmental fungal load in hospital. PLoS ONE. 12, e0177263. doi: 10.1371/journal.pone.0177263
Desprez-Loustau, M., Robin, C., Buée, M., Courtecuisse, R., Garbaye, J., Suffert, F., et al. (2007). The fungal dimension of biological invasions. Trends Ecol. Evol. 22, 472–480. doi: 10.1016/j.tree.2007.04.005
Dodds, K. J., Allison, J. D., Miller, D. R., Hanavan, R. P., and Sweeney, J. (2015). Considering species richness and rarity when selecting optimal survey traps: Comparisons of semiochemical baited flight intercept traps for Cerambycidae in eastern North America. Agric. For. Entomol. 17, 36–47. doi: 10.1111/afe.12078
Dufrêne, M., and Legendre, P. (1997). Species assemblages and indicator species: the need for a flexible asymmetrical approach. Ecol. Monogr. 67:345–366. doi: 10.1890/0012-9615(1997)067[0345:SAAIST]2.0.CO;2
Edgar, R. C. (2010). Search and clustering orders of magnitude faster than BLAST. Bioinformatics 26, 2460–2461. doi: 10.1093/bioinformatics/btq461
Edgar, R. C. (2018). UNCROSS2: identification of cross-talk in 16S rRNA OTU tables. BioRxiv.400762. doi: 10.1101/400762
Edmonds, R. L. (1972). Collection efficiency of rotorod samplers for sampling fungal spores in the atmosphere. Plant Dis. Rep. 56, 704–708.
Epanchin-Niell, R. S., Brockerhoff, E. G., Kean, J. M., and Turner, J. (2014). Designing cost-efficient surveillance for early detection and control of multiple biological invaders. Ecol. Appl. 24, 1258–1274. doi: 10.1890/13-1331.1
Flaherty, L., Gutowski, J. M. G., Hughes, C., Mayo, P., Mokrzycki, T., Pohl, G., et al. (2019). Pheromone-enhanced lure blends and multiple trap heights improve detection of bark and wood-boring beetles potentially moved in solid wood packaging. J. Pest Sci. 92, 309–325. doi: 10.1007/s10340-018-1019-4
Gagné, P., and Bérubé, J. A. (2017a). Illumicut, a C++ program specially designed to efficiently detect and remove forward and reverse sequencing primers in paired-end reconstructed sequences. Available online at: https://github.com/Patg13/Illumicut (accessed May 14, 2022).
Gagné, P., and Bérubé, J. A. (2017b). HomopRemover, a program designed to efficiently remove sequences containing very long homopolymers. Available online at: https://github.com/Patg13/HomopRemover (accessed May 14, 2022).
Gagné, P. N., Hardy, S., and Bérubé, J. A. (2020). Élaboration d'une méthodologie d'analyses bio-informatiques pour des données de séquençage illumina dans un contexte de metabarcoding. Master's degree dissertation, Faculté des sciences et de génie. Université Laval, Quebec City, Canada, Sept 21 2020. Available online at: http://hdl.handle.net/20.500.11794/66444 (accessed May 14 2022).
Haack, R. A., Britton, K. O., Brockerhoff, E. G., Cavey, J. F., Garrett, L. J., Kimberley, M., et al. (2014). Effectiveness of the International Phytosanitary Standard ISPM No. 15 on reducing wood borer infestation rates in wood packaging material entering the United States. PLos ONE 9, e96611. doi: 10.1371/journal.pone.0096611
Hirst, J. M. (1952). An automatic volumetric spore trap. Ann. Appl. Biol. 39, 257–265. doi: 10.1111/j.1744-7348.1952.tb00904.x
Hulme, P. E. (2009). Trade, transport and trouble: managing invasive species pathways in an era of globalization. J. Appl. Ecol. 46, 10–18. doi: 10.1111/j.1365-2664.2008.01600.x
Huse, S. M., Welch, D. M., Morrison, H. G., and Sogin, M. L. (2010). Ironing out the wrinkles in the rare biosphere through improved OTU clustering. Environ. Microbiol. 12, 1889–1898. doi: 10.1111/j.1462-2920.2010.02193.x
Ihrmark, K., Bödeker, I., Cruz-Martinez, K., Friberg, H., Kubartova, A., Schenck, J., et al. (2012). New primers to amplify the fungal ITS2 region–evaluation by 454-sequencing of artificial and natural communities. FEMS Microbiol. Ecol. 82, 666–677. doi: 10.1111/j.1574-6941.2012.01437.x
Jacobs, K., Seifert, K. A., Harrison, K. J., and Kirisits, T. (2003). Identity and phylogenetic relationships of ophiostomatoid fungi associated with invasive and native Tetropium species (Coleoptera: Cerambycidae) in Atlantic Canada. Can. J. Botany 81, 316–329. doi: 10.1139/b03-025
Jactel, H., Desprez-Loustau, M. L., Battisti, A., Brockerhoff, E., Santini, A., Stenlid, J., et al. (2020). Pathologists and entomologists must join forces against forest pest and pathogen invasions. NeoBiota 58, 107–127. doi: 10.3897/neobiota.58.54389
Jactel, H., Petit, J., Desprez-Loustau, M. L., Delzon, S., Piou, D., Battisti, A., et al. (2012). Drought effects on damage by forest insects and pathogens: a meta-analysis. Glob. Change Biol. 18, 267–276. doi: 10.1111/j.1365-2486.2011.02512.x
Jankowiak, R., Rossa, R., and Bilański, P. (2007). Contributions to pathogenicity of three blue-stain fungi associated with the pine sawyer beetle (Monochamus galloprovincialis) (Coleoptera: Cerambycidae) to Scots pine in Poland. Phytopathologia polonica 46, 37–46. Available online at: https://www.researchgate.net/publication/309073568_Contribution_to_pathogenicity_of_three_blue-stain_fungi_associatedwith_the_pine_sawyer_beetle_Monochamus_galloprovincialis_Coleoptera_Cerambycidae_to_Scots_pine_in_Poland (accessed May 14 2022).
Kirisits, T. (2007). “Fungal associates of European bark beetles with special emphasis on the ophiostomatoid fungi,” In: Lieutier, F., Day, K. R., Battisti, A., Grégoire, J. C, Evans, H. F. (eds) Bark and Wood Boring Insects in Living Trees in Europe, a Synthesis. Dordrecht: Springer. p. 181–236. doi: 10.1007/978-1-4020-2241-8_10
Krokene, P., and Solheim, H. (1998). Pathogenicity of four blue-stain fungi associated with aggressive and nonaggressive bark beetles. Phytopathology 88, 39–44. doi: 10.1094/PHYTO.1998.88.1.39
Kunin, V., Engelbrekston, A., Ochman, H., and Hugenholtz, P. (2010). Wrinkles in the rare biosphere: pyrosequencing errors can lead to artificial inflation of diversity estimates. Environ. Microbiol. 12, 118–123. doi: 10.1111/j.1462-2920.2009.02051.x
Lentendu, G., Zinger, L., Manel, S., Coissac, E., Choler, P., Geremia, R. A., et al. (2011). Assessment of soil fungal diversity in different alpine tundra habitats by means of pyrosequencing. Fungal Divers. 49, 113–123. doi: 10.1007/s13225-011-0101-5
Liebhold, A. M., Berec, L., Brockerhoff, E. G., Epanchin-Niell, R. S., Hasting, A., Herms, D. A., et al. (2016). Eradication of invading insect populations: From concepts to applications. Ann. Rev. Entomol. 61, 335–352. doi: 10.1146/annurev-ento-010715-023809
Liebhold, A. M., and Tobin, P. C. (2008). Population ecology of insect invasions and their management. Ann. Rev. Entomol. 53, 387–408. doi: 10.1146/annurev.ento.52.110405.091401
Linard, B., Arribas, P., Andújar, C., Crampton-Platt, A., and Vogler, A. P. (2016). Lessons from genome skimming of arthropod-preserving ethanol. Mol. Ecol. Resour. 16, 1365–1377. doi: 10.1111/1755-0998.12539
Lindahl, B. D., Nilsson, R. H., Tedersoo, L., Abarenkov, K., Carlsen, T., Kioller, R., et al. (2013). Fungal community analysis by high throughput sequencing of amplified markers – a user's guide. New Phytol. 199, 288–299. doi: 10.1111/nph.12243
Malacrino, A., Rassati, D., Schena, L., Mehzabin, R., Battisti, A., and Palmeri, V. (2017). Fungal communities associated with bark and ambrosia beetles trapped at international harbours. Fungal. Ecol. 28, 44–52. doi: 10.1016/j.funeco.2017.04.007
Marchioro, M., Rassati, D., Massimo, F., Van Rooyen, K., Kostanowicz, C., Webster, V., et al. (2020). Maximizing bark and ambrosia beetle (Coleoptera: Curculionidae) catches in trapping surveys for longhorn and jewel beetles. J. Econ. Entomol. 113, 2745–2757. doi: 10.1093/jee/toaa181
Masella, A. P., Bartram, A. K., Truszkowski, J. M., Brown, D. G., and Neufeld, J. D. (2012). PANDAseq: paired end assembler for Illumina sequences. BMC Bioinform. 13, 31. doi: 10.1186/1471-2105-13-31
McCune, B., and Mefford, M. J. (2016). PC-ORD. Multivariate Analysis of Ecological Data. Version 7.05 MjM Software, Gleneden Beach, Oregon, U.S.A. Available online at: https://static1.squarespace.com/static/58f588c93e00be17785ced5d/t/5bcccc404785d3d9aac3e266/1540148334020/PBooklet7.pdf (accessed May 14 2022).
McIntosh, R. L., Katinic, P. J., Allison, J. D., Borden, J. H., and Downey, D. L. (2001). Comparative efficacy of five types of trap for woodborers in the Cerambycidae, Buprestidae and Siricidae. Agric. For. Entomol. 3, 113–120. doi: 10.1046/j.1461-9563.2001.00095.x
Miller, D. R., Allison, J. D., Crowe, C. M., Dickinson, D. M., Eglitis, A., Hofstetter, R. W., et al. (2016). Pine sawyers (Coleoptera: Cerambycidae) attracted to α-pinene, monochamol, and ipsenol in North America. J. Econ. Entomol. 109, 1205–1214. doi: 10.1093/jee/tow071
Min, L., Zhou, X. D., De Beer, Z. W., Wingfield, M. J., and Sun, J. H. (2009). Ophiostomatoid fungi associated with the invasive pine-infesting bark beetle, Dendroctonus valens, in China. Fungal Diversity 38, 133–145. Available online at: http://hdl.handle.net/2263/13580 (accessed May 14 2022).
Moore, B. A. (2005). Alien invasive species: impacts on forests and forestry. A review. Working Paper FBS/8E. Forest Resources Development Service, Forest Resources Division, Forestry Department, FAO, Rome, Italy. Available online at: https://www.fao.org/3/j6854e/J6854E00.htm (accessed May 14 2022).
Myers, J. H., Simberloff, D., Kuris, A. M., and Carey, J. R. (2000). Eradication revisited: dealing with exotic species. Trends Ecol. Evol. 15, 316–320. doi: 10.1016/S0169-5347(00)01914-5
Nicolaisen, M., West, J. S., Sapkota, R., Canning, G. G., Schoen, C., and Justesen, A. F. (2017). Fungal communities including plant pathogens in near surface air are similar across northwestern Europe. Front. Microbiol. 8, 1729. doi: 10.3389/fmicb.2017.01729
Nilsson, R. H., Kristiansson, E., Ryberg, M., Hallenberg, N., and Larsson, K. H. (2008). Intraspecific ITS variability in the kingdom Fungi as expressed in the international sequence databases and its implications for molecular species identification. Evol. Bioinform. 4, 193–201. doi: 10.4137/EBO.S653
Ormsby, M., and Brenton-Rule, E. (2017). A review of global instruments to combat invasive alien species in forestry. Biol. Invas. 19, 3355–3364. doi: 10.1007/s10530-017-1426-0
Parmesan, C., and Yohe, G. A. (2003). Globally coherent fingerprint of climate change impacts across natural systems. Nature 421, 37–42. doi: 10.1038/nature01286
Parnell, S., van den Bosch, F., Gottwald, T., and Gilligan, C. A. (2017). Surveillance to inform control of emerging plant diseases: an epidemiological perspective. Annu. Rev. Phytopathol. 55, 591–610. doi: 10.1146/annurev-phyto-080516-035334
Pauvert, C., Buée, M., Laval, V., Edle-Hermann, V., Faucherty, L., Gautier, A., et al. (2019). Bioinformatics matters: The accuracy of plant and soil fungal community data is highly dependent on the metabarcoding pipeline. Fung. Ecol. 41, 23–33. doi: 10.1016/j.funeco.2019.03.005
Pecl, G. T., Araújo, M. B., Bell, J. D., Blanchard, J., Bonebrake, T. C., Chen, I., et al. (2017). Biodiversity redistribution under climate change: Impacts on ecosystems and human well-being. Science 355, aai9214. doi: 10.1126/science.aai9214
Penton, C. R., St. Louis, D., Cole, J. R., Luo, Y., Wu, L., Schuur, E. A., et al. (2013). Fungal diversity in permafrost and tallgrass prairie soils under experimental warming conditions. Appl. Environ. Microbiol. 79, 7063–7072. doi: 10.1128/AEM.01702-13
Rabaglia, R. J., Cognato, A. I., Hoebeke, E. R., Johnson, C. W., LaBonte, J. R., Carter, M. E., et al. (2019). Early detection and rapid response: a 10-year summary of the USDA forest service program of surveillance for non-native bark and ambrosia beetles. Am. Entomol. 65, 29–42. doi: 10.1093/ae/tmz015
Ramsfield, T., Bentz, B. J., Faccoli, M., Jactel, H., and Brockerhoff, E. G. (2016). Forest health in a changing world: effects of globalization and climate change on forest insect and pathogen impacts. Forestry 89, 245–252. doi: 10.1093/forestry/cpw018
Rassati, D., Faccoli, M., Petrucco Toffolo, E., Battisti, A., and Marini, L. (2015). Improving the early detection of alien wood-boring beetles in ports and surrounding forests. J. Appl. Ecol. 52, 50–58. doi: 10.1111/1365-2664.12347
Rassati, D., Toffolo, E. P., Roques, A., Battisti, A., and Faccoli, M. (2014). Trapping wood boring beetles in Italian ports: a pilot study. J. Pest Sci. 87, 61–69. doi: 10.1007/s10340-013-0499-5
Ravenscraft, A., Berry, M., Hammer, T., Peay, K., and Boggs, C. (2019). Structure and function of the bacterial and fungal gut microbiota of Neotropical butterflies. Ecol. Mon. 89, e01346. doi: 10.1002/ecm.1346
Redondo, M. A., Berlin, A., Boberg, J., and Oliva, J. (2020). Vegetation type determines spore deposition within a forest–agricultural mosaic landscape. FEMS Microbiol. Ecol. 96, fiaa082. doi: 10.1093/femsec/fiaa082
Reponen, T., Grinshpun, S. A., Conwell, K. L., Wiest, J., and Anderson, M. (2001). Aerodynamic versus physical size of spores: Measurement and implication for respiratory deposition. Grana 40, 119–125. doi: 10.1080/00173130152625851
Roy, B. A., Alexander, H. M., Davidson, J., Campbell, F. T., Burdon, J. J., Sniezko, R., et al. (2014). Increasing forest loss worldwide from invasive pests requires new trade regulations. Front. Ecol. Environ. 12, 457–465. doi: 10.1890/130240
Santini, A., Ghelardini, L., De Pace, C., Desprez-Loustau, M. L., Capretti, P., Chandelier, A., et al. (2013). Biogeographical patterns and determinants of invasion by forest pathogens in Europe. New Phytol. 197, 238–250. doi: 10.1111/j.1469-8137.2012.04364.x
Schloss, P. D., Westcott, S. L., Ryabin, T., Hall, J. R., Hartmann, M., Hollister, E. B., et al. (2009). Introducing Mothur: Open-source, platform-independent, community-supported software for describing and comparing microbial communities. Appl. Environ. Microbiol. 75, 7537–7541. doi: 10.1128/AEM.01541-09
Schoch, C. L., Seifert, K. A., Huhndorf, S., Robert, V., Spouge, J. L., Lévesque, C. A., et al. (2012). Fungal Barcoding Consortium. Nuclear ribosomal internal transcribed spacer (ITS) region as a universal DNA barcode marker for Fungi. Proc. Natl. Acad. Sci. USA 109, 6241–6246. doi: 10.1073/pnas.1117018109
Seebens, H., Blackburn, T. M., Dyer, E. E., Genovesi, P., Hulme, P. E., Jeschke, J. M., et al. (2017). No saturation in the accumulation of alien species worldwide. Nat. Commun. 8, 14435. doi: 10.1038/ncomms14435
Sharov, A. A., and Liebhold, A. M. (1998). Bioeconomics of managing the spread of exotic pest species with barrier zones. Ecol. Appl. 8, 833–845. doi: 10.2307/2641270
Six, D. L., and Wingfield, M. J. (2011). The role of phytopathogenicity in bark beetle–fungus symbioses: A challenge to the classic paradigm. Ann. Rev. Entomol. 56, 255–272. doi: 10.1146/annurev-ento-120709-144839
Tedersoo, L., Bahram, M., Põlme, S., Kõljalg, U., Yorou, N. S., Wijesundera, R., et al. (2014). Global diversity and geography of soil fungi. Science 346, 1256688. doi: 10.1126/science.1256688
Tremblay, É. D., Duceppe, M. O., Bérubé, J. A, Kimoto, T., Lemieux, C., and Bilodeau, G. J. (2018). Screening for exotic forest pathogens to increase survey capacity using metagenomics. Phytopathol. 108, 1509–1521. doi: 10.1094/PHYTO-02-18-0028-R
Trumbore, S., Brando, P., and Hartmann, H. (2015). Forest health and global change. Science 349, 814–818. doi: 10.1126/science.aac6759
Vira, B., Wildburger, C., and Mansourian, S. (2015). Forests, trees and landscapes for food security and nutrition. IUFRO World Series 33, 1–172. Available online at: https://www.iufro.org/publications/series/world-series/article/2015/05/06/world-series-vol-33-forests-trees-and-landscapes-for-food-security-and-nutrition-a-global-asses/ (accessed May 14 2022).
Westphal, M. I., Browne, M., MacKinnon, K., and Noble, I. (2008). The link between international trade and the global distribution of invasive alien species. Biol. Invasions 10, 391–398. doi: 10.1007/s10530-007-9138-5
Wingfield, M. J., Brockerhoff, E. G., Wingfield, B. D., and Slippers, B. (2015). Planted forest health: The need for a global strategy. Science 349, 832–836. doi: 10.1126/science.aac6674
Witzgall, P., Kirsch, P., and Cork, A. (2010). Sex pheromones and their impact on pest management. J. Chem. Ecol. 36, 80–100. doi: 10.1007/s10886-009-9737-y
Yamaoka, Y., Wingfield, M., Takahashi, I., and Solheim, H. (1997). Ophiostomatoid fungi associated with the spruce bark beetle Ips typographus f. japonicus in Japan. Mycol. Res. 101, 1215–1227. doi: 10.1017/S0953756297003924
Yemshanov, D., Koch, F. H., Lu, B., Lyons, D. B., Prestemon, J. P., Scarr, T., et al. (2014). There is no silver bullet: the value of diversification in planning invasive species surveillance. Ecol. Econ. 104, 61–72. doi: 10.1016/j.ecolecon.2014.04.024
Keywords: pest management, survey, detection, sampling, forest biosecurity
Citation: Bérubé JA, Allison JD, Van Rooyen K, Hughes C, Gagné PN, Ochoa I and Sweeney J (2022) Comparison of intercept trap fluids and aerial spore collectors to survey fungal spores. Front. For. Glob. Change 5:953130. doi: 10.3389/ffgc.2022.953130
Received: 25 May 2022; Accepted: 30 August 2022;
Published: 30 September 2022.
Edited by:
Alberto Santini, National Research Council (CNR), ItalyReviewed by:
Benoit Marçais, INRA Centre Nancy-Lorraine, FranceJonathan Spencer West, Rothamsted Research, United Kingdom
Copyright © 2022 Bérubé, Allison, Van Rooyen, Hughes, Gagné, Ochoa and Sweeney. This is an open-access article distributed under the terms of the Creative Commons Attribution License (CC BY). The use, distribution or reproduction in other forums is permitted, provided the original author(s) and the copyright owner(s) are credited and that the original publication in this journal is cited, in accordance with accepted academic practice. No use, distribution or reproduction is permitted which does not comply with these terms.
*Correspondence: Jean A. Bérubé, amVhbi5iZXJ1YmUmI3gwMDA0MDtOUkNhbi1STkNhbi5nYy5jYQ==