- 1Institute of Organic Chemistry, Technische Universität Braunschweig, Braunschweig, Germany
- 2Institute of Microbiology, Technische Universität Braunschweig, Braunschweig, Germany
Outer Membrane Vesicles (OMVs) of the Gram-negative marine bacterium Dinoroseobacter shibae, a member of the Roseobacteraceae, were investigated for the presence of volatile organic compounds (VOCs). Extracts of vesicles were analyzed by gas chromatography/mass spectrometry (GC/MS). In these analyses the short fatty acid (Z)-5-dodecenoic acid (1) and the related, more volatile aldehyde (Z)-5-dodecenal (8) were identified as VOCs of the OMVs. The aldehyde 8 has not yet been reported before from bacteria. Due to their possible function as signaling molecules, both compounds were tested for Quorum Sensing (QS) inhibition in a bioassay against the QS sensor strain Pseudomonas putida F117 (pKRC12) responsive to long-chain N-acylhomoserine lactones, the effectors of the sensor. Both compounds showed QS inhibitory activity. The potential function of VOCs in OMVs which has not been observed previously is discussed.
1. Introduction
Outer Membrane Vesicles (OMVs) are spherical vesicles secreted by Gram-negative bacteria (Beveridge, 1999; Ellis and Kuehn, 2010; Schwechheimer and Kuehn, 2015; Jan, 2017; Sartorio et al., 2021) and, less frequently, by Gram-positive bacteria (Anand and Chaudhuri, 2016) at all stages of growth (Beveridge, 1999; Ellis and Kuehn, 2010; Manning and Kuehn, 2011). Their diameter ranges from 10 to 300 nm (Ellis and Kuehn, 2010) and they are composed of a phospholipid bilayer, with the outer layer consisting of lipopolysaccharides, outer membrane proteins and receptors. Within the vesicles, there is a thin layer of peptidoglycan with periplasmic proteins and nucleic acids (McBroom and Kuehn, 2007; Cecil et al., 2019). OMVs have been reported from various bacteria such as Escherichia coli (McBroom and Kuehn, 2007; Li et al., 2022), Pseudomonas aeruginosa (Bauman and Kuehn, 2006), Shigella flexneri (Kadurugamuwa and Beveridge, 1999), Salmonella enterica (Elhenawy et al., 2016), Pectobacterium brasilense (Maphosa and Moleleki, 2021), from pathogenic and non-pathogenic strains, performing various physiological and pathological functions (Anand and Chaudhuri, 2016; Sartorio et al., 2021).
OMVs have been described to be involved in inter- and intraspecific bacterial communication (Anand and Chaudhuri, 2016; Jan, 2017; Zhao et al., 2022), promotion of virulence (Ellis and Kuehn, 2010; Mondal et al., 2016), or the transfer of DNA (Renelli et al., 2004), RNA (Ghosal et al., 2015; Celluzzi and Masotti, 2016) or toxins (Horstman and Kuehn, 2000; Lindmark et al., 2009). Moreover, the production and release of OMVs can increase in response to bacterial stress (Jan, 2017) and to biofilm formation (Kulp and Kuehn, 2010). OMVs may not only transport proteins and DNA, but also signaling molecules. For example, OMVs of the coral pathogen Vibrio shilonii AK1 contained N-acylhomoserine lactones (AHLs; Li et al., 2016). AHLs are a group of compounds mediating quorum sensing (QS), i.e., density dependent changes in gene expression of a bacterial population. For this mechanism to work, the concentration of a signaling molecule (e.g., an AHL), excreted continuously by every cell in the population, is detected by a transcriptional regulator (LuxR family of regulators) resulting in induction or repression of downstream genes (Fuqua et al., 1994).
Only recently OMVs from marine ecosystems have been detected (Biller et al., 2014) and their biogenesis and function in marine bacteria are relatively unexplored (Wang et al., 2021). The marine bacterium Dinoroseobacter shibae is a symbiont of the dinoflagellate Prorocentrum lima (Biebl et al., 2005) and serves as a model for the physiology, ecology and interactions of bacteria of the Roseobacteraceae family (Wagner-Döbler et al., 2010; Neumann et al., 2013; Patzelt et al., 2013; Wang et al., 2014). Wang et al. (2021) investigated the formation of OMVs from D. shibae and reported an average size of 20–75 nm. The OMVs carried DNA and a proteome analysis indicated their formation to be coupled to cell division, clearing the division site from small DNA fragments. These data indicate a DNA waste-disposal function for the OMVs. The fatty acid composition of the whole OMVs including bound fatty acids of the membrane phospholipids were reported to consist of the major components vaccenic acid (C18:1ω7c, 2), palmitic acid (C16:0, 3), stearic acid (C18:0, 4), (Z)-5-dodecenoic acid (C12:1ω7c, 1), and 3-hydroxydecanoic acid (10:0 3-OH, 5; Wang et al., 2021; Figure 1).
Volatile organic compounds (VOCs) are released by many bacteria and are mediating intra- and interspecific interactions leading to various physiological changes in the recipient (Schulz et al., 2010, 2020; Groenhagen et al., 2013; Schulz-Bohm et al., 2017; Tyc et al., 2017; Weisskopf et al., 2021). Although various bacteria of the Roseobacteraceae family are known to produce VOCs (Dickschat et al., 2005, 2010; Thiel et al., 2010; Riclea et al., 2012; Hahnke et al., 2013; Brock et al., 2014; Ziesche et al., 2015; Harig et al., 2017), volatiles of D. shibae have not been reported from liquid cultures. VOCs are usually small, often lipophilic compounds that can diffuse passively through membranes (Weisskopf et al., 2021). We therefore were interested whether additional mechanisms, such as release of OMVs, may exist that transport VOCs out of the bacterial cell. Indeed, here we show that OMVs transport a volatile aldehyde and a free acid, which may act as signals or active compounds in receiving organisms.
These compounds were tested for quorum-sensing inhibition using E. coli sensor strains (Riedel et al., 2001) where the downstream gene is a gfp protein, leading to fluorescence as the output signal for QS in an in vitro assay. By determining the decrease in fluorescence in the presence of an added compound, inhibition of QS can be detected. Both the aldehyde and the acid showed QS inhibitiory activity. Inhibition of QS is an exciting target for the development of next generation antibiotics which do not kill the bacteria, resulting in the undesirable selection of resistant clones, but inhibit their pathogenic traits in a density dependent way (Hentzer et al., 2002; LaSarre and Federle, 2013; O'Loughlin et al., 2013). OMVs containing QS inhibitors are potential vehicles for delivering them to pathogenic bacteria (Collins and Brown, 2021). Finally, the results show that besides passive diffusion in water or the gas phase (Weisskopf et al., 2021), extracellular transport by OMVs can be used by bacteria for dissemination of volatiles. In this study we investigated whether OMVs of D. shibae contain VOCs, indentified their structures and evaluated their activity in QS assays.
2. Materials and methods
2.1. Strains and culture conditions
Dinoroseobacter shibae DSM 16493T was cultivated as described in Wang et al. (2021). Briefly, liquid cultures were obtained in defined saltwater medium (SWM) using 20 mM succinate as a carbon source at 30°C in the dark on a platform shaker with 160 rpm. Cultures were harvested at an OD600 of approximately 2.5, which represents the late-exponential growth phase.
OMVs were isolated as described (Wang et al., 2021). Briefly, bacterial cells were removed by centrifugation (10,900 g, 15 min, 4°C). The supernatant was filtered through 0.45 mm (Nalgene, Thermo Scientific) and 0.22 mm (Millipore) bottle-top filters. The filtrate was concentrated using a tangential flow filtration system (Vivaflow 200; Sartorius) with a 100-kDa-molecular-mass cutoff. The concentrate was ultracentrifuged at 100,000 g for 2 h at 4°C. The pellets were resuspended in 2 ml 45% OptiPrep (in buffer containing 3.6% [wt/vol] NaCl and 10 mM HEPES, pH 8). Samples were loaded into the bottom of a 13.2-ml ultracentrifuge tube and overlaid with 1 ml of 40, 35, 30, 25, 20, 15, 10, 5, and 0% OptiPrep. The gradient was centrifuged at 280,000 g for 3 to 12 h at 4°C. The brownish fraction containing pure vesicles was collected, diluted with buffer (10 mM HEPES, 3.6% NaCl) to 30 ml, and pelleted at 100,000 g for 2 h at 4°C. The supernatant was discarded, and the final pellets were frozen at −70°C until further analysis. Two OMV pellets were thus prepared. Details on microscopy, size, abundance, protein content and DNA content of the OMVs can be found in Wang et al. (2021).
2.2. Quorum sensing inhibition tests
Two compounds from D. shibae OMVs were tested for their ability to inhibit QS: (Z)-5-dodecenal (8), mw 182.3 g/mol, and (Z)-5-dodecenoic acid (1), mw 198.3 g/mol. Inhibition of QS induced by three different long-chain N-acylhomoserine lactones (AHLs) was tested: (1) N-3-oxododecanoylhomoserine lactone (3-oxo-C12-HSL), (2) N-3-oxotetradecanoylhomoserine lactone (3-oxo-C14-HSL), and (3) N-(Z)-9-hexadecenoylhomoserine lactone (C16:1-HSL).
The Pseudomonas putida F117 (pKRC12) sensor strain for long-chain AHLs (Riedel et al., 2001) was used for the QS inhibition assay. The sensor strain was grown overnight in Luria-Bertani (LB) medium with addition of gentamycin (20 μg/ml) at 30°C with shaking (160 rpm). The culture was then diluted 20-fold with fresh medium and grown until an optical density at 600 nm (OD600) of 1.0 was reached. Compounds used for the bioassay were dissolved in methanol and added to 96-well microtiter plates at a final concentration of 1, 10, 50, and 100 μg/ml. Ten μL of the test compound were pipetted into the wells. After evaporation of methanol, 100 μl of fresh medium and 100 μl of culture were pipetted into the wells. The microtiter plates were incubated at 30°C for 24 h. Every hour for the first 10 h and after 24 h the green fluorescence was determined using the microtiter plate reader Victor 1,420 Multilabel Counter (Perkin-Elmer) with an excitation wavelength of 485 nm and a detection wavelength of 535 nm. OD620 was also measured at the same time points. DMSO and methanol added to the culture was used as negative control (no QS activity), and the synthetic AHLs 3-oxo-C12-HSL, 3-oxo-C14-HSL and C16:1-HSL, dissolved in DMSO were used as positive controls at the final concentrations of 100 nM for oxo-C12-HSL and 3-oxo-C14-HSL, and 1 μM for C16:1-HSL. Test compounds were assayed in triplicate. Fold induction of fluorescence was calculated by dividing the specific fluorescence (gfp535/OD620) of the test compound by the specific fluorescence of the negative control. The % inhibition was calculated using the following formula:
2.3. Experimental procedures
2.3.1. General experimental procedures
Chemicals were purchased from Sigma Aldrich (Germany), TCI (Germany) or from abcr GmbH (Germany) and used without further purification. Solvents were purified by distillation and dried according to usual standard laboratory methods. Reactions with air- and moisture-sensitive compounds were carried out in vacuum-heated flasks under a nitrogen atmosphere. Solutions at 0°C were obtained with the aid of an ice-water bath. Thin-layer chromatography (TLC) was carried out on silica gel coated films Polygram® SIL G/UV254 (Macherey-Nagel, layer thickness 0.2 mm). In addition to UV detection (254 nm), common staining reagents such as molybdophosphoric acid or potassium permanganate were used. Flash column chromatography was carried out on silica gel 60 Å (grain size 35–70 μm) from Fisher Scientific. NMR spectra were recorded with an Avance III 400 spectrometer (400 MHz for 1H, 100 MHz for 13C) from Bruker at room temperature. Tetramethylsilane served as the internal standard. The chemical shifts are given in ppm relative to the standard. The coupling constants J are given in Hertz (Hz). Full synthetic details of the synthesized compounds are given in the Supporting information.
2.3.2. Gas chromatographic/mass spectrometric analyses
Gas chromatographic/mass spectrometric (GC/MS) analyses of synthetic samples were performed on an Agilent 8,860 gas chromatograph coupled to an Agilent 5977B mass selective detector. The measurements were carried out in a pulsed split mode with the following temperature program: 50°C (5 min. isothermal) start temperature, 20°C/min heating rate, 320°C (5 min isothermal) final temperature. The analyses of extracts of the OMVs were performed on an Agilent 7890A gas chromatograph with a MSD 5975 mass selective detector. The measurements were carried out with the following temperature program: 50°C (5 min. isothermal) start temperature, 5°C/min heating rate, 320°C (10 min isothermal) final temperature. Gas chromatographic separation was performed on fused-silica capillary columns HP-5MS (30 m × 0.25 mm ID × 0.25 μm film, Agilent Technologies). Helium was used as carrier gas with a volume flow of 1.2 ml/min and electron impact ionization was carried out at 70 eV for both instruments. Linear gas chromatographic retention indices (RI) were determined from a homologous series of n-alkanes (C8–C40).
For VOC analysis, 50.0 mg of each of the two OMVs pellets were freeze-dried. Then, 2.0 mg of each of pellet were extracted six times with 50 μl dichloromethane by shaking. The solvent was evaporated at room temperature and the residue was dissolved in 90 μl dichloromethane. The extracts were derivatized with MSTFA for a better chromatographic separation of polar components such as acids or alcohols, and analyzed by GC/MS. Controls of the work-up and extraction processes were prepared and analyzed by GC/MS.
2.3.3. Derivatization with N-methyl-N-(trimethylsilyl)trifluoroacetamide
An synthetic acid (0.1 mg) or a natural sample (10 μl) was dissolved in 300 μl dichloromethane (DCM), mixed with 50 μl N-methyl-N-(trimethylsilyl)trifluoroacetamide (MSTFA) and the mixture was heated for 60 min at 60°C in a heating block in a 2 ml screwcap vial. Subsequently, the solvent and excess MSTFA reagent were removed in a stream of nitrogen, and the residue was taken up in dichloromethane, the volume reduced by evaporation at room temperature to 10 μl and finally analyzed by GC/MS.
3. Results
3.1. GC/MS analysis of the OMVs
Initial experiments showed the presence of a suspecteted fatty acid in the extract of the OMVs. Therefore, parts of the extracts were trimethylsilylated with MSTFA for better peak shape of polar compounds, but only the acid was derivatized. The total ion chromatograms (TICs) of the GC/MS analyses of the two OMVs pellet extracts and the control measurement are shown in Figure 2. Due to the low amount of material and the isolation process, various compounds present as contaminants were detected. Nevertheless, two compounds A and B were detected in larger amounts, not present in the control, and no additional compounds were detected in the silylated samples.
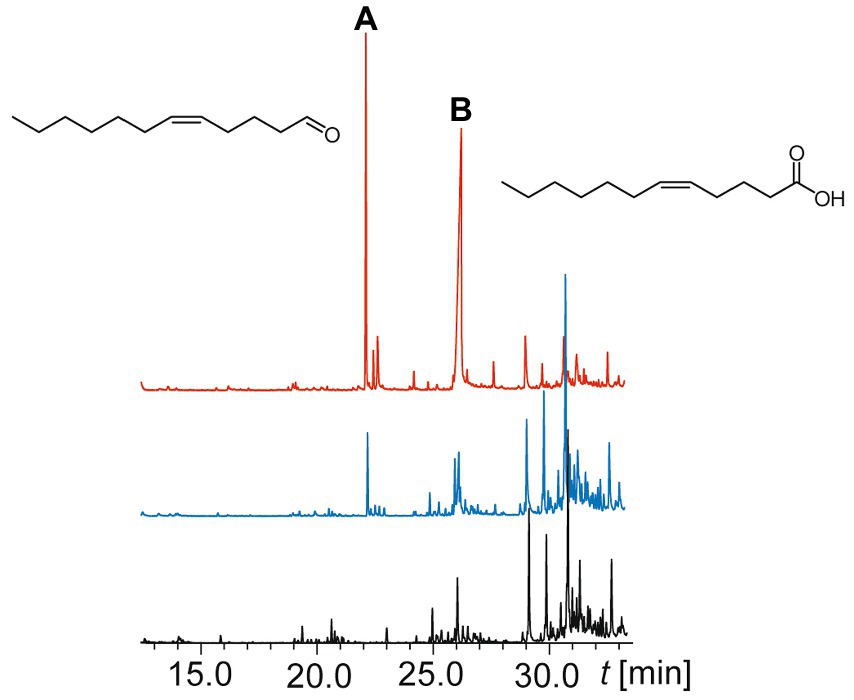
Figure 2. Total ion chromatograms of a GC/MS analyses of two outer membrane vesicle extracts (blue and red upper traces) and a control sample of the medium (black lower trace). Compounds A and B and their structures are indicated.
Comparison of the spectrum of compound A (linear gas chromatographic retention index (RI) 1,389) with the NIST 17 mass spectral library (2017) revealed (Z)-5-dodecenal (8) as the closest match. The RI was identical to the value reported by Marques et al. (2000), which reported the RIs of all possible positional isomers of dodecenal on an apolar DB-5 phase. Interestingly, all other dodecenals showed higher RI values, (Z)-4-dodecenal with RI 1393 being the closest isomer. Compound B (RI 1555) showed a mass spectrum that was similar to that of (Z)-5-dodecenoic acid (1), an acid found earlier in combined bound and unbound fatty acid analysis of D. shibae OMVs by Wang et al. (2021). None of the other reported OMV acids were identified. The structural proposals for compounds A and B were then confirmed by synthesis of reference compounds. Earlier experiments had shown that bacterial cultures of D. shibae did not release volatile A or other specific volatiles.
3.2. Syntheses of reference compounds
The unsaturated acid 1 was commercially available and thus used for verification. Derivatization with MSTFA gave trimethylsilyl (Z)-5-dodecenoate (6) that proved to be identical with the silylated natural acid, thus confirming the identity of compound B as (Z)-5-dodecenoic acid. Acid 1 was also reduced with LiAlH4 in quantitative yield to give (Z)-5-dodecen-1-ol (7), followed by a Parikh-Doering oxidation (Tojo, 2006) to arrive at the desired unsaturated aldehyde (Z)-5-dodecenal (8) with a yield of 84% (Figure 3). GC/MS confirmed the identity of 8 and compound A.
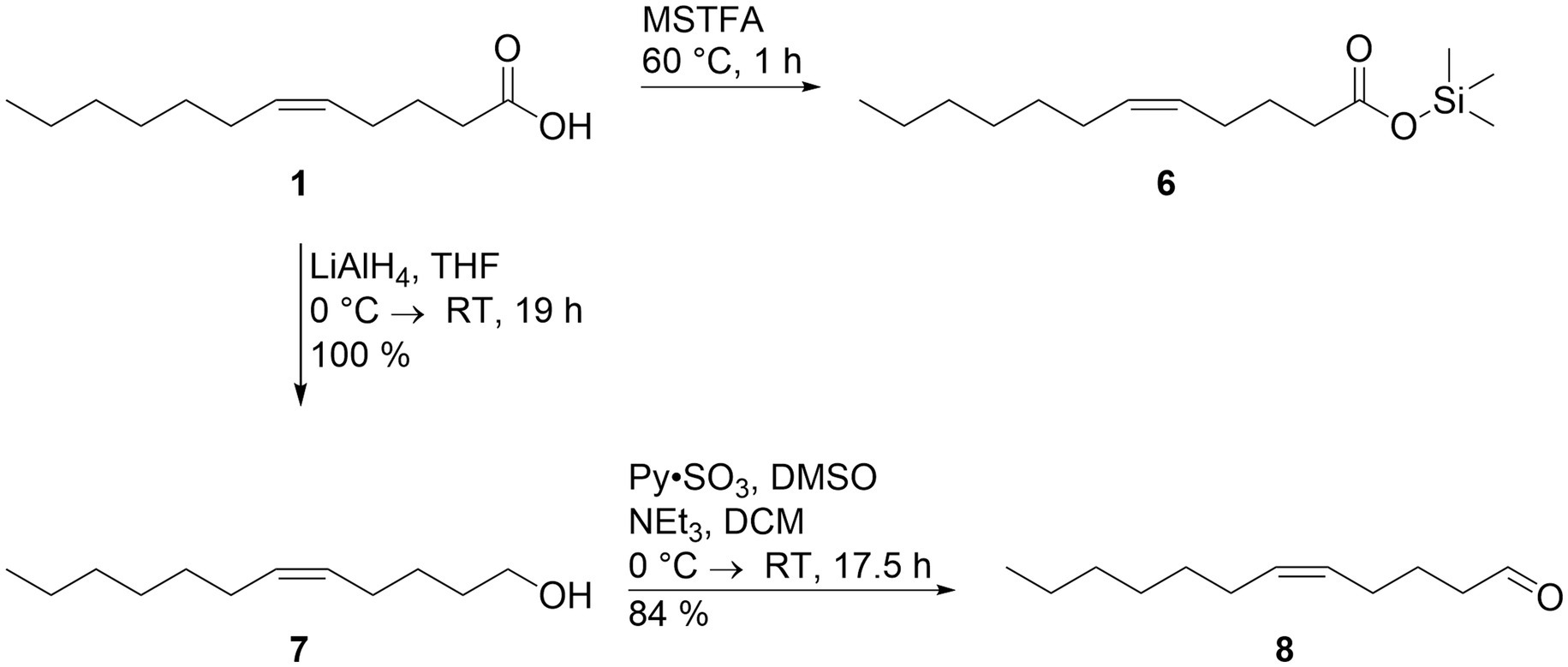
Figure 3. Syntheses of reference compounds trimethylsilyl (Z)-5-dodecenoate (6) and (Z)-5-dodecenal (8). The synthesis is described in detail in the text. The trimethylsilyl ester of natural compound A proved to be identical to compound 6, confirming the identity of (Z)-5-dodecenoic acid (1), and natural compound B.
3.3. Inhibition of QS by (Z)-5-dodecenal and (Z)-5-dodecenoic acid
(Z)-5-Dodecenal (8) and the corresponding acid (Z)-5-dodecenoic acid (1) were tested for their ability to inhibit QS mediated by acylated homoserine lactones (AHLs). A sensor strain whose QS mechanism is responsive to short-chain AHLs and a sensor strain sensitive to long-chain AHLs (Riedel et al., 2001) were used. Since no activity was found against the short-chain sensor strain, these data are not reported here. To induce QS in the assay strain P. putida F117 (pKR12), three long-chain AHLs with different chain lengths and substitutions were used, namely C12-oxo-HSL, C14-oxo-HSL, and C16:1-HSL. They were applied at the lowest concentrations that resulted in maximum QS, namely 100 nM for C12-oxo-HSL and C14-oxo-HSL and 1 μM for C16:1-HSL. The test compounds 1 and 8 were applied at concentrations between 1 and 100 μg/ml. The complete experiment was conducted twice.
Figure 4 shows that both compounds had similar QS inhibiting activity. The AHLs with different chain-lengths were inhibited at different concentrations. Inhibition of C12-oxo-HSL was the weakest, with only 22.5 and 23.3% at 100 μg/ml of 8, and a slightly higher inhibition for 1 (29.4 and 38.4%). At lower concentrations the inhibition was below 10%.
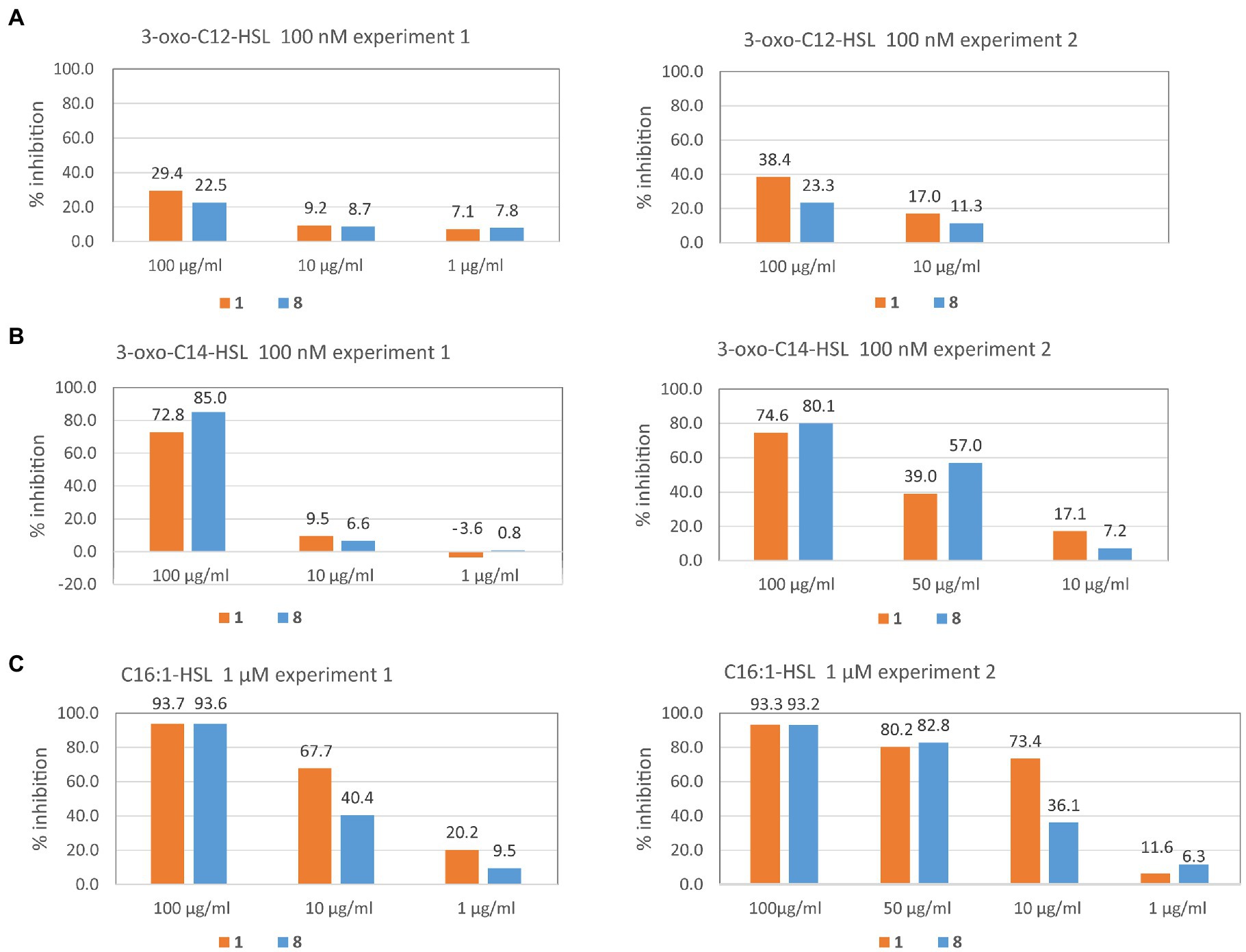
Figure 4. Inhibition of QS by volatiles from OMVs of Dinoroseobacter shibae. QS was induced in the sensor strain Pseudomonas putida F117 (pKRC12) by 3-oxo-C12-HSL (A), 3-oxo-C14-HSL (B), or C16:1-HSL (C) and inhibition by (Z)-5-dodecenal (8) and (Z)-5-dodecenoic acid (1) is shown in % of control without inhibitor. The experiment for each QS inducer was performed twice (experiment 1 and experiment 2) and the inhibitor concentrations tested in the second experiment were sometimes modified based on the results found in the first experiment to provide a finer resolution. For example, for inducer 3-oxo-C14-HSL (B) we did not test 1 μg/ml of inhibitor in the second experiment, because no activity was found in the first experiment, but tested 50 μg/ml instead. Each QS test was conducted by measuring green fluorescence of the test strain P. putida F117 pKRC12 in 10 parallel wells under the respective condition every hour (except at night) for 24 h. For each timepoint, mean and standard deviation of inhibition from 10 replicate wells are provided in Supplementary Table S1 for both experiments. Here we show % inhibition after 24 h. Standard deviation is below 0.1% and therefore would not be visible on the graph. Inhibition of 100 nM 3-oxo-C12-HSL by 1 μg/ml of inhibitors was not measured. See methods for further details.
For C14-oxo-HSL, we found high inhibition at 100 μg/ml, namely 85.0 and 80.1% for 8, and slightly lower inhibition for 1 (72.8 and 74.6%). At 50 μg/ml, the aldehyde 8 still showed 57% inhibition of QS, and the acid 1 39%. At lower concentrations of the test compounds, inhibition was below 10%, except for 1 at 10 μg/ml with 17.1% inhibition.
For C16:1-HSL the highest QS inhibition activity found was higher for 1 compared to 8. At 100 μg/ml of the test compounds, QS was inhibited by 93% by both test compounds. At 50 μg/ml of the test compounds, QS was still inhibited by 82.8% (8) and 80.2% (1). At 10 μM of 8, inhibition was still high (40.4 and 36.1%), and even higher for 1 (67.7 and 74.4%). At 1 μM of test compounds, inhibition by 8 was below 10% for both compounds, except for 1 in one experiment (20.2%).
To summarize, the QS inhibitory activity of both compounds increased with the chain-length of the AHL that was antagonized and was highest against C16:1-AHL. At a concentration of 10 μg/ml the aldehyde (Z)-5-dodecenal (8) inhibited the QS activity induced by this AHL on average by 38.2%, and the corresponding acid (Z)-5-dodecenoic acid (1) inhibited QS by 70.5% on average.
4. Discussion
(Z)-5-Dodecenal (8) and (Z)-5-dodecenoic acid (1) were the only compounds identified by GC/MS analysis of OMVs. As described, 1 has been reported as a constituent of the total fatty acid content of the OMVs (Wang et al., 2021), but no other acids were detected. This indicates that 1 indeed occurs as the sole free acid in the OMVs, in contrast to the other acids reported by Wang et al. that obviously are present in bound form, presumably mostly in the membrane phospholipids. Acid 1 has already been described from Rhodobacter capsulatus (Mayer et al., 1996), Escherichia coli (Feng and Cronan, 2009) and other bacteria (Moss and Daneshvar, 1992). The volatile aldehyde 8 has not been reported from bacteria so far, but is known as a sex pheromone of moths, e.g., Gastropacha quercifolia (Bestmann et al., 1993) and Dendrolimus punctatus (Zhao et al., 2004) and in the anal gland of the European rabbit (Goodrich et al., 1978). Its biosynthesis very likely starts from acid 1, either as free acid or conjugated to e.g., coenzyme A. Direct reduction would lead to 8, or alternatively first to the alcohol 7 that could be oxidized again. The latter pathway has been described in the moth G. quercifolia (Bestmann et al., 1993). Unsaturated aldehydes are known as defense compounds of diatom algae, e.g., Thalassosira rotula, where they are formed differently by lipoxygenases (Pohnert, 2005). The aldehyde group is highly reactive, being able to react easily with e.g., N-nucleophiles and is easyly oxidizable, in case of 8 back to acid 1.
We report here for the first time the presence of volatile compounds in OMVs. The OMV release seems to be a second mechanism by which bacteria can release VOCs, next to direct release into the gas phase or water phase (Weisskopf et al., 2021). The diffusion of volatiles both in the gas phase or water is usually fast. Such a fast dissipation of compounds is reduced by OMV, because they transport a locally high concentration of compounds within them. When OMVs open, maybe after integration into another cell or next to a target, a higher local concentration of their enclosed compounds is obtained, compared to undirected diffusion. Furthermore, the lipid surface may also be used for defined recognition processes, mediated by the OMV wall, which cannot be the case for freely diffusing VOCs.
The function of 8, and of the acid 1, for D. shibae is unclear, but several options exist. The inherent reactivity may suggest a defensive function against competitors, e.g., by interference with quorum-sensing systems of other bacteria or with other traits (Pepi et al., 2017). It may thus secure the symbiotic relationship with its host P. lima, reducing algal surface population by other bacteria (Rolland et al., 2016). Defensive aldehydes related to those of T. rotula have recently been reported from the dinoflagellate P. cordatum, related to the D. shibae host P. lima (Koteska et al., 2022). Therefore, release of the lipoxygenase generated dinoflagellate aldehydes and the fatty acid derived bacterial aldehydes may increase efficacy of bacteria control. On the other hand, 8 may also function as chemical signal transported by the OMVs to their target location, protected from degradation by the lipid layer. Known targets of bacterial VOCs can be the cell membrane, altering their fluidity and permeability, porin channels or directly the DNA, leading to DNA damage and altered gene expression (Weisskopf et al., 2021).
A different possibility is that 8 acts as oxidation inhibitor, securing the DNA content of the OMVs against oxidative degradation by reaction with oxygen, leading to acid 1. It would be interesting to see whether a reduction of 1 to 8 can take place within the OMVs. This would then be a circular protection system as long as enough energy is present within the OMVs.
5. Summary/Conclusion
In this study the major small molecule components of OMVs of D. shibae were identified as (Z)-5-dodecenoic acid (1) and the volatile (Z)-5-dodecenal (8). This aldehyde has not been described as a bacterial natural product so far and is the first volatile detected in OMVs. An additonal pathway for extracellular transport of bacterial volatiles has been detected, enhancing the commonly observed passive diffusion of VOCs in water or the gas phase. Both compounds showed QS inhibition in a in vitro assays against quorum sensing sensor strains. OMVs may be involved in the transport of VOCs through the cell membrane to their target location.
Data availability statement
The original contributions presented in the study are included in the article/Supplementary material, further inquiries can be directed to the corresponding author.
Author contributions
DK, IW-D, and SS conceived and designed the study. DK and HW performed all laboratory experiments, analyzed the data and prepared the figures. DK wrote the first draft of the manuscript. All authors contributed to the article and approved the submitted version.
Funding
This work was funded by the Deutsche Forschungsgemeinschaft (TRR/SFB 51 Roseobacter).
Acknowledgments
We thank Vanessa Stiller for technical assistance.
Conflict of interest
The authors declare that the research was conducted in the absence of any commercial or financial relationships that could be construed as a potential conflict of interest.
Publisher’s note
All claims expressed in this article are solely those of the authors and do not necessarily represent those of their affiliated organizations, or those of the publisher, the editors and the reviewers. Any product that may be evaluated in this article, or claim that may be made by its manufacturer, is not guaranteed or endorsed by the publisher.
Supplementary material
The Supplementary material for this article can be found online at: https://www.frontiersin.org/articles/10.3389/fevo.2023.1102159/full#supplementary-material
References
Anand, D., and Chaudhuri, A. (2016). Bacterial outer membrane vesicles: new insights and applications. Mol. Membr. Biol. 33, 125–137. doi: 10.1080/09687688.2017.1400602
Bauman, S. J., and Kuehn, M. J. (2006). Purification of outer membrane vesicles from Pseudomonas aeruginosa and their activation of an IL-8 response. Microbes Infect. 8, 2400–2408. doi: 10.1016/j.micinf.2006.05.001
Bestmann, H. J., Attygalle, A. B., Garbe, W., Kern, F., Martichonok, V., Schäfer, D., et al. (1993). Chemical structure and final steps of biosynthesis of the female sex pheromone of Gastropacha quercifolia (Lepidoptera: Lasiocampidae). Insect Biochem. Mol. Biol. 23, 793–799. doi: 10.1016/0965-1748(93)90067-3
Beveridge, T. J. (1999). Structures of gram-negative cell walls and their derived membrane vesicles. J. Bacteriol. 181:4733. doi: 10.1128/JB.181.16.4725-4733.1999
Biebl, H., Allgaier, M., Tindall, B. J., Koblizek, M., Lünsdorf, H., Pukall, R., et al. (2005). Dinoroseobacter shibae gen. nov., sp. nov., a new aerobic phototrophic bacterium isolated from dinoflagellates. Int. J. Syst. Evol. Microbiol. 55, 1089–1096. doi: 10.1099/ijs.0.63511-0
Biller, S. J., Schubotz, F., Roggensack, S. E., Thompson, A. W., Summons, R. E., and Chisholm, S. W. (2014). Bacterial vesicles in marine ecosystems. Science 343, 183–186. doi: 10.1126/science.1243457
Brock, N. L., Menke, M., Klapschinski, T. A., and Dickschat, J. S. (2014). Marine bacteria from the Roseobacter clade produce sulfur volatiles via amino acid and dimethylsulfoniopropionate catabolism. Org. Biomol. Chem. 12, 4318–4323. doi: 10.1039/c4ob00719k
Cecil, J. D., Sirisaengtaksin, N., O'Brien-Simpson, N. M., and Krachler, A. M. (2019). Outer membrane vesicle-host cell interactions. Microbiol. Spectrum 7:1. doi: 10.1128/microbiolspec.PSIB-0001-2018
Celluzzi, A., and Masotti, A. (2016). How our other genome controls our epi-genome. Trends Microbiol. 24, 777–787. doi: 10.1016/j.tim.2016.05.005
Collins, S. M., and Brown, A. C. (2021). Bacterial outer membrane vesicles as antibiotic delivery vehicles. Front. Immunol. 12:733064. doi: 10.3389/fimmu.2021.733064
Dickschat, J. S., Wagner-Döbler, I., and Schulz, S. (2005). The chafer pheromone buibuilactone and ant pyrazines are also produced by marine bacteria. J. Chem. Ecol. 31, 925–947. doi: 10.1007/s10886-005-3553-9
Dickschat, J. S., Zell, C., and Brock, N. L. (2010). Pathways and substrate specificity of DMSP catabolism in marine bacteria of the Roseobacter clade. Chembiochem 11, 417–425. doi: 10.1002/cbic.200900668
Elhenawy, W., Bording-Jorgensen, M., Valguarnera, E., Haurat, M. F., Wine, E., and Feldman, M. F. (2016). LPS remodeling triggers formation of outer membrane vesicles in Salmonella. MBio 7:1. doi: 10.1128/mBio.00940-16
Ellis, T. N., and Kuehn, M. J. (2010). Virulence and immunomodulatory roles of bacterial outer membrane vesicles. Microbiol. Mol. Biol. Rev. 74, 81–94. doi: 10.1128/MMBR.00031-09
Feng, Y., and Cronan, J. E. (2009). Escherichia coli unsaturated fatty acid synthesis: complex transcription of the fabA gene and in vivo identification of the essential reaction catalyzed by FabB. J. Biol. Chem. 284, 29526–29535. doi: 10.1074/jbc.M109.023440
Fuqua, W. C., Winans, S. C., and Greenberg, E. P. (1994). Quorum sensing in bacteria: the LuxR-LuxI family of cell density-responsive transcriptional regulators. J. Bacteriol. 176, 269–275. doi: 10.1128/jb.176.2.269-275.1994
Ghosal, A., Upadhyaya, B. B., Fritz, J. V., Heintz-Buschart, A., Desai, M. S., Yusuf, D., et al. (2015). The extracellular RNA complement of Escherichia coli. Microbiol. Open 4, 252–266. doi: 10.1002/mbo3.235
Goodrich, B. S., Hesterman, E. R., Murray, K. E., Mykytowycz, R., Stanley, G., and Sugowdz, G. (1978). Identification of behaviorally significant volatile compounds in the anal gland of the rabbit, Oryctolagus cuniculus. J. Chem. Ecol. 4, 581–594. doi: 10.1007/BF00988922
Groenhagen, U., Baumgartner, R., Bailly, A., Gardiner, A., Eberl, L., Schulz, S., et al. (2013). Production of bioactive volatiles by different Burkholderia ambifaria strains. J. Chem. Ecol. 39, 892–906. doi: 10.1007/s10886-013-0315-y
Hahnke, S., Brock, N. L., Zell, C., Simon, M., Dickschat, J. S., and Brinkhoff, T. (2013). Physiological diversity of Roseobacter clade bacteria co-occurring during a phytoplankton bloom in the North Sea. Syst. Appl. Microbiol. 36, 39–48. doi: 10.1016/j.yapm.2012.09.004
Harig, T., Schlawis, C., Ziesche, L., Pohlner, M., Engelen, B., and Schulz, S. (2017). Nitrogen-containing volatiles from marine Salinispora pacifica and Roseobacter-group bacteria. J. Nat. Prod. 80, 3289–3295. doi: 10.1021/acs.jnatprod.7b00789
Hentzer, M., Riedel, K., Rasmussen, T. B., Heydorn, A., Andersen, J. B., Parsek, M. R., et al. (2002). Inhibition of quorum sensing in Pseudomonas aeruginosa biofilm bacteria by a halogenated furanone compound. Microbiology 148, 87–102. doi: 10.1099/00221287-148-1-87
Horstman, A. L., and Kuehn, M. J. (2000). Enterotoxigenic Escherichia coli secretes active heat-labile enterotoxin via outer membrane vesicles. J. Biol. Chem. 275, 12489–12496. doi: 10.1074/jbc.275.17.12489
Jan, A. T. (2017). Outer membrane vesicles (OMVs) of gram-negative bacteria: a perspective update. Front. Microbiol. 8:1053. doi: 10.3389/fmicb.2017.01053
Kadurugamuwa, J. L., and Beveridge, T. J. (1999). Membrane vesicles derived from Pseudomonas aeruginosa and Shigella flexneri can be integrated into the surfaces of other gram-negative bacteria. Microbiology 145, 2051–2060. doi: 10.1099/13500872-145-8-2051
Koteska, D., Sanchez Garcia, S., Wagner-Döbler, I., and Schulz, S. (2022). Identification of volatiles of the dinoflagellate Prorocentrum cordatum. Mar. Drugs 20:371. doi: 10.3390/md20060371
Kulp, A., and Kuehn, M. J. (2010). Biological functions and biogenesis of secreted bacterial outer membrane vesicles. Annu. Rev. Microbiol. 64, 163–184. doi: 10.1146/annurev.micro.091208.073413
LaSarre, B., and Federle, M. J. (2013). Exploiting quorum sensing to confuse bacterial pathogens. Microbiol. Mol. Biol. Rev. 77, 73–111. doi: 10.1128/MMBR.00046-12
Li, J., Azam, F., and Zhang, S. (2016). Outer membrane vesicles containing signalling molecules and active hydrolytic enzymes released by a coral pathogen Vibrio shilonii AK1. Environ. Microbiol. 18, 3850–3866. doi: 10.1111/1462-2920.13344
Li, C., Wen, R., Mu, R., Chen, X., Ma, P., Gu, K., et al. (2022). Outer membrane vesicles of avian pathogenic Escherichia coli mediate the horizontal transmission of blaCTX-M-55. Pathogens 11:481. doi: 10.3390/pathogens11040481
Lindmark, B., Rompikuntal, P. K., Vaitkevicius, K., Song, T., Mizunoe, Y., Uhlin, B. E., et al. (2009). Outer membrane vesicle-mediated release of cytolethal distending toxin (CDT) from Campylobacter jejuni. BMC Microbiol. 9:220. doi: 10.1186/1471-2180-9-220
Manning, A. J., and Kuehn, M. J. (2011). Contribution of bacterial outer membrane vesicles to innate bacterial defense. BMC Microbiol. 11:258. doi: 10.1186/1471-2180-11-258
Maphosa, S., and Moleleki, L. N. (2021). Isolation and characterization of outer membrane vesicles of Pectobacterium brasiliense 1692. Microorganisms 9:1918. doi: 10.3390/microorganisms9091918
Marques, F. D. A., McElfresh, J. S., and Millar, J. G. (2000). Kováts retention indexes of monounsaturated C12, C14, and C16 alcohols, acetates and aldehydes commonly found in lepidopteran pheromone blends. J. Braz. Chem. Soc. 11, 592–599. doi: 10.1590/S0103-50532000000600007
Mayer, H., Merkofer, T., Warth, C., and Weckesser, J. (1996). Position and configuration of double bonds of lipid A-associated monounsaturated fatty acids of Proteobacferia and Rhodobacter capsulatus 37b4. J. Endotoxin Res. 3, 345–352. doi: 10.1177/096805199600300409
McBroom, A. J., and Kuehn, M. J. (2007). Release of outer membrane vesicles by gram-negative bacteria is a novel envelope stress response. Mol. Microbiol. 63, 545–558. doi: 10.1111/j.1365-2958.2006.05522.x
Mondal, A., Tapader, R., Chatterjee, N. S., Ghosh, A., Sinha, R., Koley, H., et al. (2016). Cytotoxic and inflammatory responses induced by outer membrane vesicle-associated biologically active proteases from Vibrio cholerae. Infect. Immun. 84, 1478–1490. doi: 10.1128/IAI.01365-15
Moss, C. W., and Daneshvar, M. I. (1992). Identification of some uncommon monounsaturated fatty acids of bacteria. J. Clin. Microbiol. 30, 2511–2512. doi: 10.1128/jcm.30.9.2511-2512.1992
Neumann, A., Patzelt, D., Wagner-Döbler, I., and Schulz, S. (2013). Identification of new N-acylhomoserine lactone signalling compounds of Dinoroseobacter shibae DFL-12T by overexpression of luxI genes. Chembiochem 14, 2355–2361. doi: 10.1002/cbic.201300424
O'Loughlin, C. T., Miller, L. C., Siryaporn, A., Drescher, K., Semmelhack, M. F., and Bassler, B. L. (2013). A quorum-sensing inhibitor blocks Pseudomonas aeruginosa virulence and biofilm formation. Proc. Natl. Acad. Sci. U. S. A. 110, 17981–17986. doi: 10.1073/pnas.1316981110
Patzelt, D., Wang, H., Buchholz, I., Rohde, M., Gröbe, L., Pradella, S., et al. (2013). You are what you talk: quorum sensing induces individual morphologies and cell division modes in Dinoroseobacter shibae. ISME J. 7, 2274–2286. doi: 10.1038/ismej.2013.107
Pepi, M., Heipieper, H. J., Balestra, C., Borra, M., Biffali, E., and Casotti, R. (2017). Toxicity of diatom polyunsaturated aldehydes to marine bacterial isolates reveals their mode of action. Chemosphere 177, 258–265. doi: 10.1016/j.chemosphere.2017.03.031
Pohnert, G. (2005). Diatom/copepod interactions in plankton: the indirect chemical defense of unicellular algae. Chembiochem 6, 946–959. doi: 10.1002/cbic.200400348
Renelli, M., Matias, V., Lo, R. Y., and Beveridge, T. J. (2004). DNA-containing membrane vesicles of Pseudomonas aeruginosa PAO1 and their genetic transformation potential. Microbiology 150, 2161–2169. doi: 10.1099/mic.0.26841-0
Riclea, R., Gleitzmann, J., Bruns, H., Junker, C., Schulz, B., and Dickschat, J. S. (2012). Algicidal lactones from the marine Roseobacter clade bacterium Ruegeria pomeroyi. Beilstein J. Org. Chem. 8, 941–950. doi: 10.3762/bjoc.8.106
Riedel, K., Hentzer, M., Geisenberger, O., Huber, B., Steidle, A., Wu, H., et al. (2001). N-Acylhomoserine-lactone-mediated communication between Pseudomonas aeruginosa and Burkholderia cepacia in mixed biofilms. Microbiology 147, 3249–3262. doi: 10.1099/00221287-147-12-3249
Rolland, J. L., Stien, D., Sanchez-Ferandin, S., and Lami, R. (2016). Quorum sensing and quorum quenching in the phycosphere of phytoplankton: a case of chemical interactions in ecology. J. Chem. Ecol. 42, 1201–1211. doi: 10.1007/s10886-016-0791-y
Sartorio, M. G., Pardue, E. J., Feldman, M. F., and Haurat, M. F. (2021). Bacterial outer membrane vesicles: from discovery to applications. Annu. Rev. Microbiol. 75, 609–630. doi: 10.1146/annurev-micro-052821-031444
Schulz, S., Biwer, P., Harig, T., Koteska, D., and Schlawis, C. (2020). “Chemical ecology of bacterial volatiles” in Comprehensive natural products III. eds. H.-W. Liu and T. P. Begley (Amsterdam: Elsevier), 161–178.
Schulz, S., Dickschat, J. S., Kunze, B., Wagner-Dobler, I., Diestel, R., and Sasse, F. (2010). Biological activity of volatiles from marine and terrestrial bacteria. Mar. Drugs 8, 2976–2987. doi: 10.3390/md8122976
Schulz-Bohm, K., Martín-Sánchez, L., and Garbeva, P. (2017). Microbial volatiles: small molecules with an important role in intra- and inter-kingdom interactions. Front. Microbiol. 8:2484. doi: 10.3389/fmicb.2017.02484
Schwechheimer, C., and Kuehn, M. J. (2015). Outer-membrane vesicles from gram-negative bacteria: biogenesis and functions. Nat. Rev. Microbiol. 13, 605–619. doi: 10.1038/nrmicro3525
Thiel, V., Brinkhoff, T., Dickschat, J. S., Wickel, S., Grunenberg, J., Wagner-Döbler, I., et al. (2010). Identification and biosynthesis of tropone derivatives and sulfur volatiles produced by bacteria of the marine Roseobacter clade. Org. Biomol. Chem. 8, 234–246. doi: 10.1039/B909133E
Tojo, G. (2006). Oxidation of alcohols to aldehydes and ketones: A guide to current common practice. New York: Springer.
Tyc, O., Song, C., Dickschat, J. S., Vos, M., and Garbeva, P. (2017). The ecological role of volatile and soluble secondary metabolites produced by soil bacteria. Trends Microbiol. 25, 280–292. doi: 10.1016/j.tim.2016.12.002
Wagner-Döbler, I., Ballhausen, B., Berger, M., Brinkhoff, T., Buchholz, I., Bunk, B., et al. (2010). The complete genome sequence of the algal symbiont Dinoroseobacter shibae: a hitchhiker's guide to life in the sea. ISME J. 4, 61–77. doi: 10.1038/ismej.2009.94
Wang, H., Beier, N., Boedeker, C., Sztajer, H., Henke, P., Neumann-Schaal, M., et al. (2021). Dinoroseobacter shibae outer membrane vesicles are enriched for the chromosome dimer resolution site dif. mSystems 6:1. doi: 10.1128/mSystems.00693-20
Wang, H., Ziesche, L., Frank, O., Michael, V., Martin, M., Petersen, J., et al. (2014). The CtrA phosphorelay integrates differentiation and communication in the marine alphaproteobacterium Dinoroseobacter shibae. BMC Genomics 15:130. doi: 10.1186/1471-2164-15-130
Weisskopf, L., Schulz, S., and Garbeva, P. (2021). Microbial volatile organic compounds in intra-kingdom and inter-kingdom interactions. Nat. Rev. Microbiol. 19, 391–404. doi: 10.1038/s41579-020-00508-1
Zhao, C.-H., Adlof, R. O., and Löfstedt, C. (2004). Sex pheromone biosynthesis in the pine caterpillar moth, Dendrolimus punctatus (Lepidoptera: Lasiocampidae): pathways leading to Z5-monoene and 5,7-conjugated diene components. Insect Biochem. Mol. Biol. 34, 261–271. doi: 10.1016/j.ibmb.2003.10.005
Zhao, Z., Wang, L., Miao, J., Zhang, Z., Ruan, J., Xu, L., et al. (2022). Regulation of the formation and structure of biofilms by quorum sensing signal molecules packaged in outer membrane vesicles. Sci. Total Environ. 806:151403. doi: 10.1016/j.scitotenv.2021.151403
Ziesche, L., Bruns, H., Dogs, M., Wolter, L., Mann, F., Wagner-Döbler, I., et al. (2015). Homoserine lactones, methyl oligohydroxybutyrates, and other extracellular metabolites of macroalgae-associated bacteria of the Roseobacter clade: identification and functions. Chembiochem 16, 2094–2107. doi: 10.1002/cbic.201500189
Keywords: VOCs, GC/MS, Roseobacteraceae fam. nov., quorum sensing inhibition, aldehydes
Citation: Koteska D, Wang H, Wagner-Döbler I and Schulz S (2023) Outer membrane vesicles of Dinoroseobacter shibae transport a volatile aldehyde. Front. Ecol. Evol. 11:1102159. doi: 10.3389/fevo.2023.1102159
Edited by:
Viviane Cordovez, Netherlands Institute of Ecology (NIOO-KNAW), NetherlandsReviewed by:
Graciela Dias, Federal University of Rio de Janeiro, BrazilAnna Maria Puglia, University of Palermo, Italy
Olaf Tyc, University Hospital Frankfurt, Germany
Copyright © 2023 Koteska, Wang, Wagner-Döbler and Schulz. This is an open-access article distributed under the terms of the Creative Commons Attribution License (CC BY). The use, distribution or reproduction in other forums is permitted, provided the original author(s) and the copyright owner(s) are credited and that the original publication in this journal is cited, in accordance with accepted academic practice. No use, distribution or reproduction is permitted which does not comply with these terms.
*Correspondence: Stefan Schulz, c3RlZmFuLnNjaHVsekB0dS1icmF1bnNjaHdlaWcuZGU=