- 1State Key Laboratory of Water Environment Simulation, School of Environment, Beijing Normal University, Beijing, China
- 2Shandong Provincial Key Laboratory of Applied Microbiology, Ecology Institute, Qilu University of Technology (Shandong Academy of Sciences), Jinan, China
- 3Research and Development Center for Watershed Environmental Eco-Engineering, Beijing Normal University, Zhuhai, China
- 4Henan Key Laboratory of Ecological Environment Protection and Restoration of Yellow River Basin, Yellow River Institute of Hydraulic Research, Zhengzhou, China
The depositional flux of coastal wetlands and the deposition rate of biogenic elements greatly affect the carbon sink storage. Ecological stoichiometry is an important ecological indicator, which can simply and intuitively indicate the biogeochemical cycle process of the region. This study investigated the soil deposition flux, stocks, and ecological stoichiometric ratios of C, N, P, and S under different water and salt conditions based on 137Cs dating technology in the Yellow River Delta (YRD) of China. The results showed that the deposition fluxes were 0.38 cm/year for PV wetlands, 1.08 cm/yr for PA wetlands, and 1.06 cm/yr for SS wetlands. Similarly, PA wetlands showed higher deposition fluxes of C, N, and S compared with SS and PV wetlands. PA wetlands had higher stocks of C (5.86 kg/m2), N (0.36 kg/m2) and S (0.36 kg/m2) in the top 1-m soil layer compared with PV and SS wetlands. However, the highest deposition rate of P (9.82 g/yr/m2) was observed in SS wetlands among the three wetlands. Three accumulative hotspots of C, N, and S in soil profiles of PA and SS wetlands were observed at soil depths of 0–10, 40–60, and 90–100 cm, whereas one accumulative hotspot of P was at the soil depth of 10–12 cm in SS wetlands and 80–82 cm in PA wetlands. PV wetlands showed higher accumulations of C, P, and S in the top 10 cm soil layer and N at the soil depth of 90–100 cm. The higher top concentration factors in these three wetlands indicated that the dominant input of plant residues was the main reason. The ratios of C/N and C/N/P of each sampling site were higher in the surface soils and decreased with depth. The ratios of C/P and N/P were larger in the surface layer (0–20 cm), the middle layer (40–60 cm), and the deep layer (90–100 cm). The ratios of N/P and C/N/P were relatively lower, indicating that these studied wetlands were N-limited ecosystems. The results implied that the coastal wetlands in the YRD have huge storage potential of biogenic elements as blue carbon ecosystems.
Introduction
Coastal wetlands, sea-land interlaced belts and buffer zones are important areas for the strong exchange of materials and energy between the ocean and land. They play a vital role in regulating global environmental changes and serving as sources and sinks of carbon (C), nitrogen (N), phosphorus (P), and sulfur (S). C, N, P, and S are important constituent elements in the soil. Their contents and stocks can directly affect the soil quality, nutrient cycling, and ecological functions of the coastal wetland ecosystems (Bai et al., 2012; Feng et al., 2017; Lu et al., 2018).
The regulation of biological elements with different soil profiles in the coastal wetland is not obvious because coastal wetlands are subject to the combined effects of erosion and sedimentation under the two-way regulation of tidal current and runoff. Taking soil organic carbon (SOC) as an example, the SOC pool is composed of the active pool and the inert pool, which leads to different stability in the soil profile due to high spatial heterogeneity. In addition, soil animals and microorganisms could decompose SOC and nitrogen into CO2, CH4 or N2O, which would exacerbate the heterogeneity of soil carbon or nitrogen pools (Wu et al., 2013, 2015).
Many studies have shown that SOC is mainly distributed in the upper soil layer of the soil profile and it tends to gradually decrease from the surface to the bottom (Wang et al., 2010). However, the current research on the SOC storage of coastal wetlands is mainly concentrated on the surface soil (0–30 cm) of the Yellow River Delta (YRD) region, and there are few studies on the contribution rate of the deep SOC storage to the carbon pool (Luo et al., 2020). Therefore, it is not enough to accurately reflect the soil carbon pool of coastal wetlands by studying the biogenic elements in surface soils. For the study of the distribution form of soil biogenic elements and ecological stoichiometry in the soil, a deeper soil profile should be selected.
The ecological stoichiometric ratio provides important insights for studying the energy and material cycles in estuarine ecosystems (Coynel et al., 2016). In addition, each ecological stoichiometric ratio is of great significance to the biogeochemical cycle of C, N, P, and S in the soil. For example, the soil C/N ratio is a sensitive indicator used to measure soil quality (Elser et al., 2003); the C/P ratio and C/S ratio are considered to be symbols of the mineralization ability of phosphorus and sulfur in the soil. Previous studies have focused on the stoichiometric ratios of soil and the effects of various environmental factors, such as vegetation types and rainfall, on the ecological stoichiometric ratios of soil at different soil depths in forests, karst, and grasslands (Yang et al., 2014; Fan et al., 2015; Wang et al., 2018). Some researchers have presented the impacts of the ecological stoichiometric ratios on various ecological functions in the ecosystems and the interactions between microbes and the ecological stoichiometric ratios in the abovementioned ecosystems. Although the stoichiometric ratios in coastal wetlands are more important due to the impacts of historical sedimentation and strong exchange between marine and terrestrial materials of coastal wetlands, only few studies have been conducted on the relations between sedimentation and ecological stoichiometry in coastal wetlands.
The Yellow River is one of the rivers with the highest sediment content in the world, and the relationship between its sedimentation flux and the stocks of soil biogenic elements is also a problem that needs to be revealed. As the most fragile and sensitive new delta in the world, the YRD has extremely precious ecological value. In recent years, governance along the Yellow River has also received great attention from the Chinese government. Therefore, it is necessary to study the soil deposition rate of the YRD and enhance the sequestration capacity of these elements and soil quality management of the coastal wetlands. The primary objectives of this study were to: (1) analyze the depth of the distributions of the contents and stocks of C, N, P, and S along soil profiles with different water and salt conditions in the YRD; (2) investigate the deposition rates of C, N, P, and S in the soil using the 137Cs dating technique in coastal wetlands; and (3) identify the changes in ecological stoichiometric ratios of C, N, P, and S with depth along the soil profiles.
Materials and Methods
Study Area
The study area is located in the YRD (N 37°35′-38°12′, E 118°33′-119°20′), Dongying city, Shandong Province, China. It has a warm temperate semihumid monsoon climate with four distinct seasons, rain and heat at the same time, small regional climate differences, and a frost-free period of 196 days (Cui et al., 2008). The annual average sunshine hours are 2,590–2,830 h, and the annual average temperature is 12.1°C. The annual precipitation is 551.6 mm, 70% of which is concentrated in the period from June to August. The annual evaporation is 1,962 mm. The spring evaporation is strong, and the evaporation accounts for approximately 51.7% of the year. The drought index is as high as 3.56 (Gao et al., 2012). The main vegetation types are herbs, such as Pteris violata, Phragmites australis, Triarrhena sacchariflora, Suaeda salsa, Myriophyllum spicatum, and Limoninum sinense; shrubs, such as Tamarix chinensis; and trees, such as Salix matsudana (Cheng et al., 2021). There are more than 40 families, 110 genera, and 160 species. Phragmites australis, Pteris violate, and Suaeda salsa are the main dominant species in this area.
Three sampling sites were selected along one sampling belt perpendicular to the riverbed in the coastal wetlands on the north bank of the Yellow River (Figure 1), including Pteris violata wetlands (PV), Phragmites australis wetlands (PA), and Suaeda salsa wetlands (SS). Among them, PA wetlands can be affected by underground seawater and freshwater when the water and sediment regulation project of the YRD is implemented in July. Comparatively, SS wetlands are dominantly affected by tidal water.
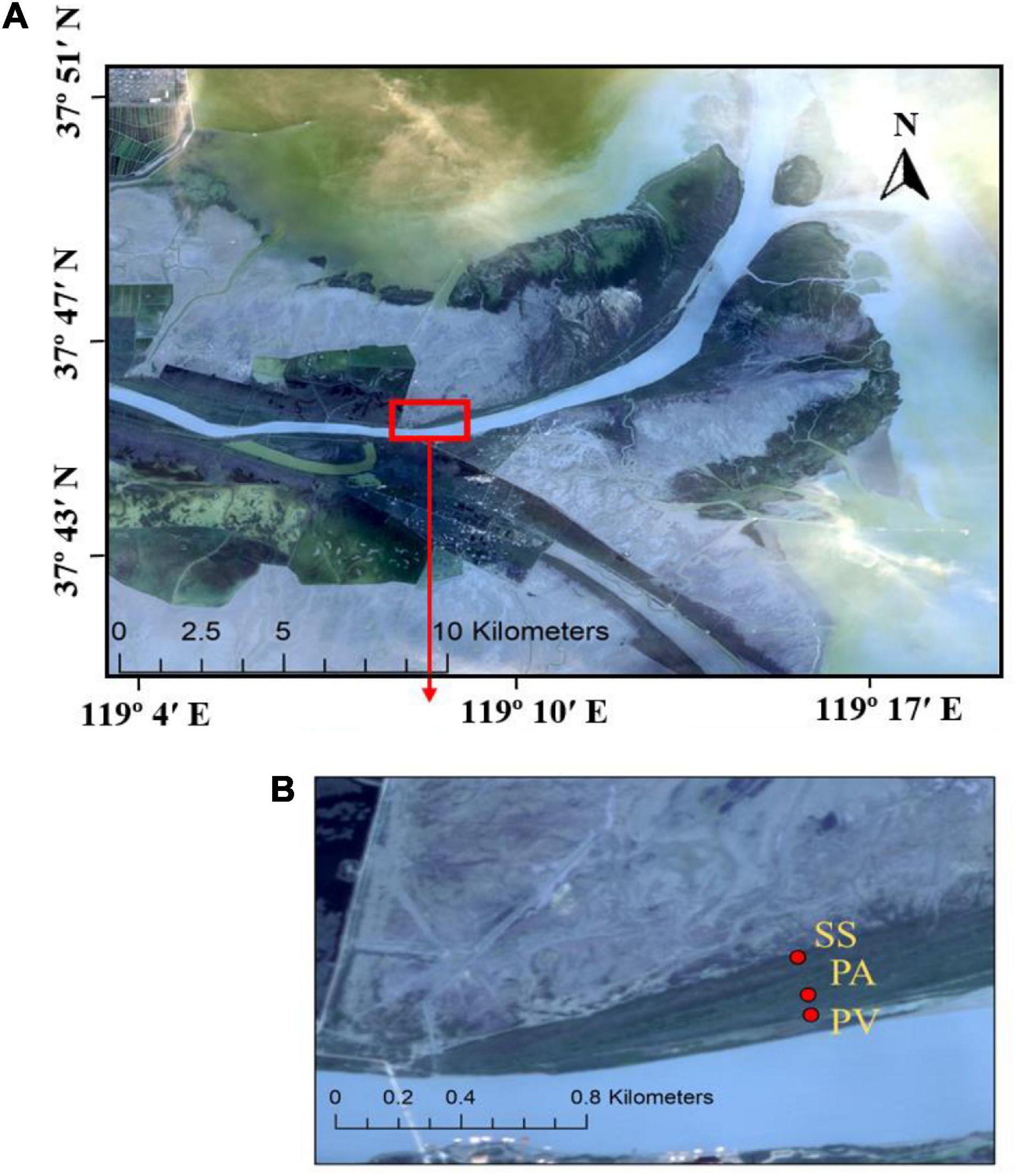
Figure 1. Location map of sampling sites in the YRD. (A) The location of sampling belt in the YRD, (B) the location of sampling sites in the sampling belt.
Sample Collection and Analysis
The soil samples were collected by excavating the soil profiles at a depth of 0–100 cm at intervals of 2 cm. After removing visible plant residues and stones, the soil samples were air-dried for 2 or 3 weeks. One replicate was sampled at each point for analyzing relevant indicators. One part of the air-dried samples were ground through sieving using a 20-mesh for the determination of 137Cs labeling. Another part of the sample was further ground to pass through a 100-mesh sieve for determining soil properties. SOC was measured by the potassium dichromate dilution thermal-colorimetric method. Total nitrogen (N) was measured on an elemental analyzer (CHNOS elemental analyzer, Vario EL, Germany). Total phosphorus (P) and total sulfur (S) were determined by inductively coupled plasma atomic emission spectroscopy (ICP/AES) after digestion in an HClO4-HNO3-HF mixture in Teflon tubes. Quality assurance and quality control were assessed using duplicates, method blanks, and standard reference materials (GBW07401) from the Chinese Academy of Measurement Sciences with each batch of samples (1 blank and 1 standard for every 10 samples). The recoveries of samples spiked with standards ranging from 95 to 106%. 137Cs value in the soil was determined using a high-purity germanium gamma spectrometer in the laboratory of the Geography Department, Beijing Normal University.
Stocks and Topsoil Concentration Factors
The stocks of C, N, P, and S were calculated as follows:
where CS, NS, PS, and SS are the stocks of C, N, P, and S per unit area (kg/m2), respectively; Ci, Ni, Pi, and Si are the average contents of C, N, P and S in the soil layer i (i = 1, 2, 3, 4, and 5); and T is the thickness of soil layer i (cm). The topsoil concentration factor is calculated by the stocks in the top 10 cm and the stocks in the top 100 cm.
Statistical Analysis and Graphing
One-way ANOVA was used to identify the significant difference in C, N, P, and S contents and stocks between different wetlands. The difference was considered to be significant if P < 0.05. Data processing was performed using Excel 2019 software package software, and graphs were created by Origin 2017 software package.
Results
Contents and Stocks of C, N, P and S
The highest average contents of C, N, and S in the PA wetland were 3.91, 0.24, and 0.24 g/kg, respectively, and the PV wetland had the highest average contents of P (0.65 g/kg) (Figure 2). SOC content in PA wetlands was significantly higher than that in PV wetlands (P < 0.05). Three layers with high concentrations of C, N, and S in soil profiles were observed in PA and SS wetlands at soil depths of 0–10 cm, 40–60 cm, and 90–100 cm. In PV wetlands, higher concentrations of C (5.29 g/kg) and S (0.36 g/kg) appeared in surface soils (0–10 cm) and N (0.48 g/kg) at soil depths of 90–100 cm, respectively. However, P contents showed different depth distributions in three wetlands, with an accumulative peak at the soil depth of 4–6 cm (3.70 g/kg) in PV wetlands, 10–12 cm (2.58 g/kg) in SS wetlands, and 80–82 cm (3.36 g/kg) in PA wetlands.
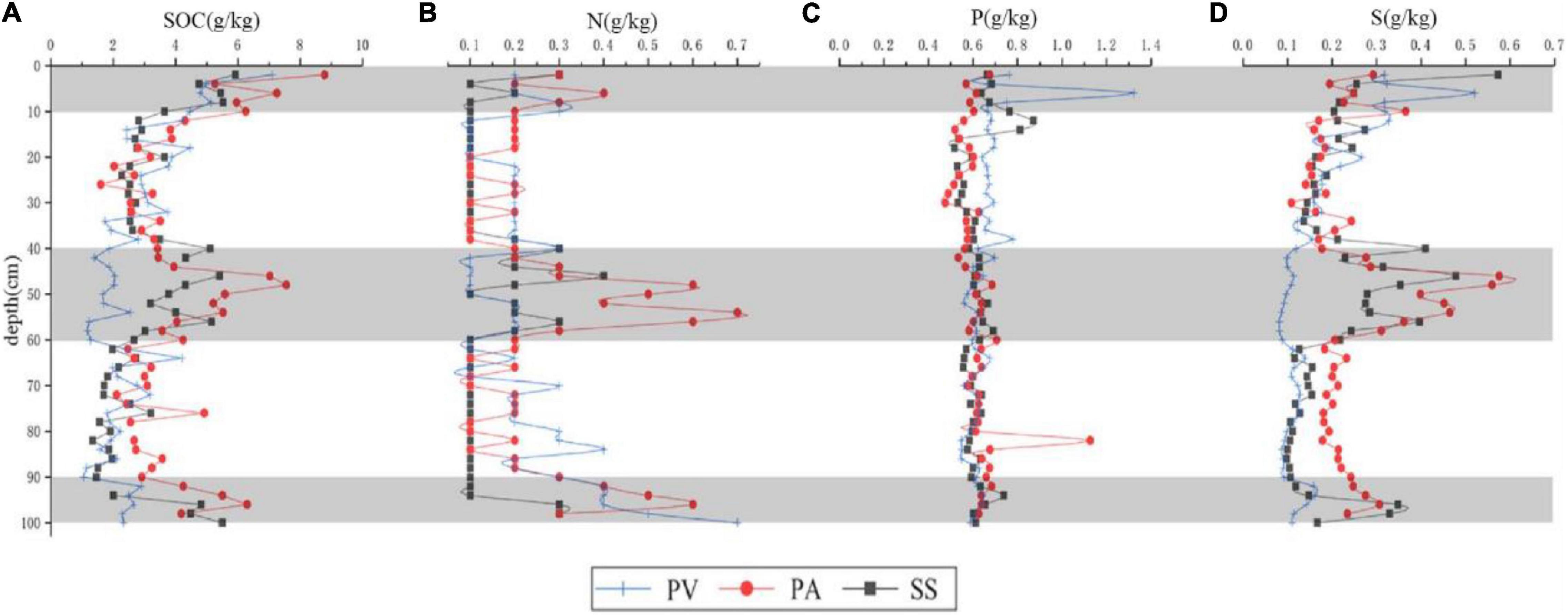
Figure 2. Changes in the contents of C (A), N (B), P (C), and S (D) along different soil profiles in PV, PA, and SS wetlands.
SOC stocks in 1-m depth reached approximately 6 kg/m2 in PA wetlands, slightly higher than that in the PV (3.55 kg/m2) and SS (5 kg/m2) wetlands (Figure 3). In contrast, low soil N stocks (0.36 kg/m2, PA; 0.22 kg/m2, SS; and 0.32 kg/m2, PV) were observed in the YRD. Unlike C and N, three wetlands showed similar soil P stocks (0.92 kg/m2) in 1-m depth. Similar to N, PA wetlands exhibited the largest soil S stocks (0.36 kg/m2), followed by SS wetlands (0.31 kg/m2), while the lowest soil S stocks (0.23 kg/m2) in PV wetlands were observed. SOC stocks in three accumulative hotspots in PA wetlands were 97.79 g/m2 in top 10 cm soils, 148.25 g/m2 for the 40–60 cm soil depth, and 66.32 g/m2 for the 90–100 cm soil depth, also 64.67, 113.86, and 59.25 g/m2 in SS wetlands, respectively. Soil TN stocks in three accumulative hotspots were 4.09 g/m2 (0–10 cm), 12.14 g/m2 (40–60 cm), and 5.36 g/m2 (90–100 cm) in PA wetlands, with TS stocks of 3.87, 11.50, and 3.65 g/m2, respectively. In SS wetlands, TN and TS stocks were 2.05 and 5.03 g/m2 in the top 10 cm, 5.88 and 2.81 g/m2 for the 40–60 cm soil depth, and 3.52 and 1.99 g/m2 for the 90–100 cm soil depth. Soil P stocks in the accumulative hotspot were 9.85 g/m2 for the 10–20 cm soil depth in SS wetland and 11.25 g/m2 for the 80–90 cm soil depth in the PA wetland. In contrast, the accumulative hotspot of C, P, and S stocks appeared in top 10 cm soils of PV wetlands (with the levels of 74.06, 11.67, and 3.83 g/m2, respectively), whereas the accumulative hotspot of N appeared in the 90–100 cm soil depth (6.96 g/m2).
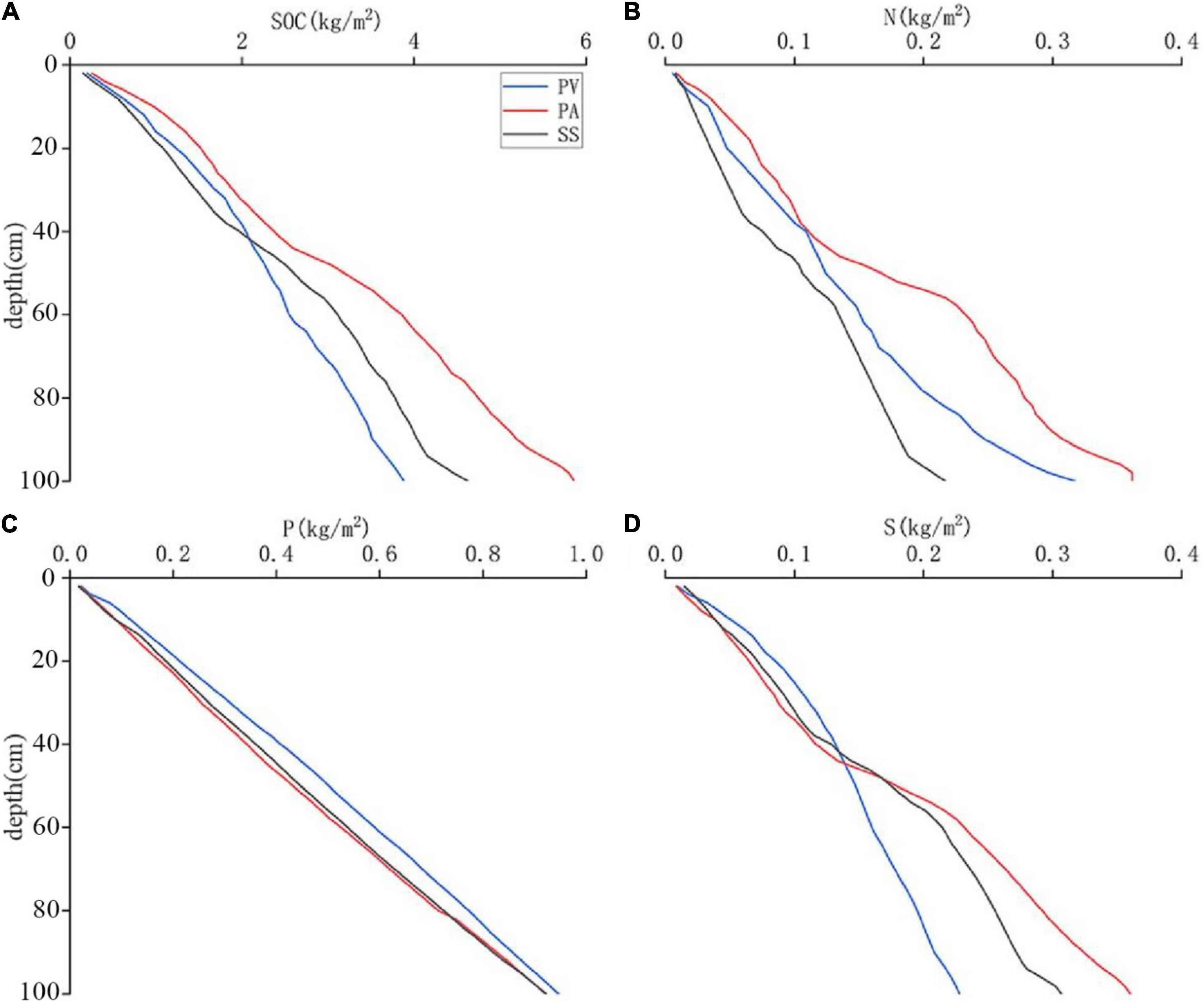
Figure 3. Accumulative distributions of the stocks of C (A), N (B), P (C), and S (D) along with different soil profiles in PV, PA, and SS wetlands.
Soil Concentration Factors and Deposition Rates
The top concentration factors of C, N, P, and S in each wetland were all more than 0.10, which indicated that the accumulation of these elements was mainly affected by plant secretions (Table 1). Among them, the topsoil concentration factor of C fell within the scope of 0.16–0.22, following the order PV > PA > SS. As for soil N, their topsoil concentration factors in the three wetlands were relatively low. The followed order of topsoil concentration factors of both P and S was PV > SS > PA. The topsoil concentration factors of C, N, P, and S in the PA wetlands were higher than that of PV and SS wetlands. After 137Cs isotope dating, the depth of the soil layer represented in the year 1963 was identified at the 20-cm soil depth in PV wetland, 56-cm soil depths in PA, and 58-cm soil depths in SS wetlands. As shown in Figure 4, the deposition rate of sediments in PV wetland was 0.38 cm/yr, 1.08 cm/yr in PA wetland, and 1.06 cm/yr in SS wetland.
Deposition Fluxes of C, N, P and S
Different deposition rates of C, N, P, and S in three wetlands were also observed. PA wetlands exhibited the highest deposition rate of C (71.17 g/yr/m2), N (4.35 g/yr/m2), and S (4.34 g/yr/m2), while the lowest deposition rate appeared in PV wetlands (Figure 5). In contrast, the highest deposition rate of P followed the order SS wetlands (9.82 g/yr/m2) > PA wetlands (9.70 g/yr/m2) > PV wetlands (4.08 g/yr/m2). The contents of four elements in three wetlands showed a similar pattern with depth along soil profiles (Figure 2). Except for N, the contents of C, P, and S in the top 15-cm soils were relatively high, then declined significantly at approximately 20-cm soil depth and kept stable. However, at soil depth of approximately 40 cm, the contents of C, N, and S in the soil increased significantly again, but the change of P content was not obvious at this depth. At approximately 60-cm soil depth, the contents of these four elements again fell to a relatively stable level, to the soil layer of approximately 90–100 cm.
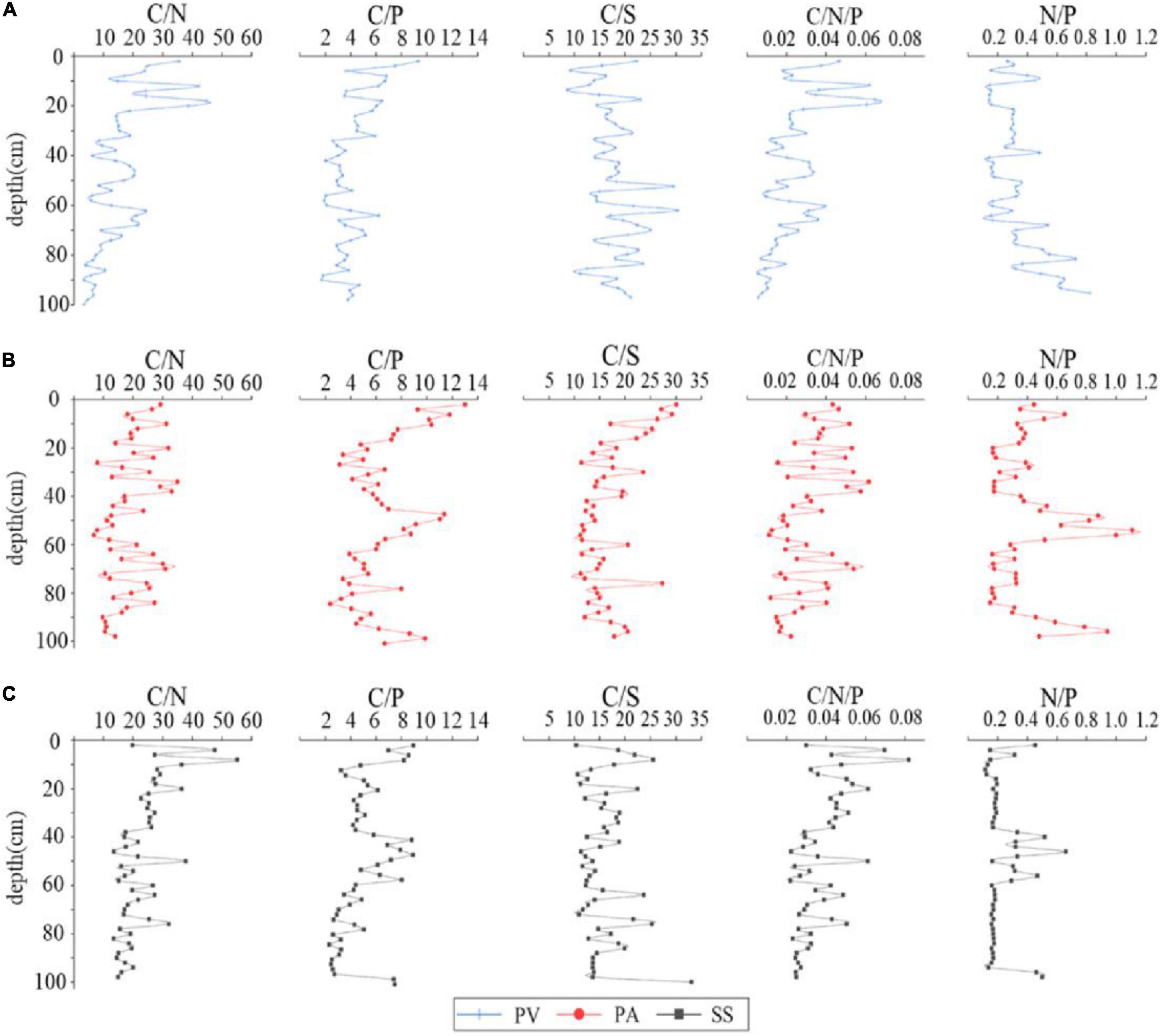
Figure 5. Depth distributions of the ecological stoichiometric ratios along soil profiles under different water and salt conditions. PV (A), PA (B), SS (C).
Ecological Stoichiometric Ratios
The soil C/N ratios in the three wetlands remained relatively stable (Figure 4). PV wetlands showed a higher range of the C/N ratios than PA and SS wetlands. This indicated that the C/N ratios at higher ranges in low-salinity wetlands were lower than that in middle-salinity and high-salinity wetlands. The highest C/P ratios were observed in PA wetlands, followed by those in SS wetlands, while the lowest value appeared in PV wetlands. Similarly, both C/N and C/P ratios were higher in surface soils of the three wetlands, and higher ratios also appeared at soil depths of 40–60 and 90–100 cm in PA and SS wetlands. However, this was not the case in PV wetlands. PA wetlands showed higher C/S ratios in upper soils than deeper soils, while a fluctuating change was observed along soil profiles in PV and SS wetlands. The C/N/P of SS wetlands was significantly higher than that of PA wetlands; the C/N/P of PA wetlands was significantly higher than that of PV wetlands (P < 0.05). N/P ratios decreased along soil profiles in PV wetlands, and a fluctuation was observed in PA and SS wetlands with higher ratios at the soil depths of 40–60 and 90–100 cm.
Discussion
Depth Distributions of the Contents of C, N, P and S Along Soil Profiles
Higher contents of C, N, P, and S in surface soils (0–10 cm) of the three wetlands were generally observed, which was associated with exogenous inputs of plant litters, sedimentation by freshwater, and seawater input (Saintilan et al., 2013). The contents of C and N in the surface soils were higher than that in the deep soils in coastal wetlands (Wang et al., 2016). The reason might be that the surface soils are more easily affected by environmental changes (Salome et al., 2010). In addition, higher contents of C, N, and S in the three wetlands were also observed at soil depths of 40–60 and 90–100 cm, which was associated with historical input and sedimentation. Over the past years, historical plant death and sedimentation due to flooding or Yellow River runoff might contribute to the elemental accumulation in deep soils. According to the annual Yellow River Yearbook, the Yellow River was diverted in 1964, which may have caused a large number of plants to be buried underground at this stage and converted into biogenic elements, such as C, N, P, and S. Another reason might be related to the high content of silt and clay in these layers since soil C content has a linear relationship with the composition content of clay + silt in the YRD (Zhao et al., 2020). Additionally, microbial activity and leaching could also cause C, N, and S in the surface soil to migrate downward (Wang et al., 2010).
Soil Deposition and Stocks of C, N, P and S in Soil Profiles
Compared with PA and SS wetlands, PV wetlands showed lower soil deposition at the rate of 0.38 cm/yr, which could be ascribed to the strong erosion by river water in PV wetland and higher sediment deposition in PA (1.08 cm/yr) and SS (1.06 cm/yr) wetlands affected by the flow-sediment regulation in July and sediment input by tidal flow (Bai et al., 2020). Our results fell within the range by DeLaune et al. (2003) that the deposition rate of Louisiana estuarine wetlands affected by freshwater input was 0.10–1.11 cm/yr. Ding et al. (2016) also reported that the deposition rate of tidal flats in the YRD was 0.58 cm/yr, which was lower than that of PA and SS wetlands and higher than that of PV wetland observed in this study. This indicated that tidal flats underwent much stronger hydraulic erosion by tidal flow than coastal salt marshes (Ding et al., 2016). Moreover, river flow might have a much stronger erosion than tidal flow in the study area.
In general, the stocks of C, N, P, and S in PA wetlands were higher than those of PV and SS wetlands. This might be associated with less erosion by runoff or tidal flow in PA wetlands. Moreover, higher plant biomass of Phragmites australis and high litter inputs in PA wetlands than PV and SS wetlands could explain higher levels of these elements (Lu et al., 2018). Additionally, higher salinity in SS wetland may inhibit the accumulation of SOC (Zhao et al., 2017). The SOC stocks in this study were 1.47–1.89 kg/m2 at the top 30 cm soils, which was within the range of the previous results (1.17–2.14 kg/m2) (Yu et al., 2013). Compared with other Chinese coastal wetlands, the SOC storage in the YRD was lower than that of the Chongming Dongtan coastal wetland (2.32 kg/m2) (Jiang et al., 2015), but higher than that in the Shanghai tidal flats (1.38 kg/m2) (Shi et al., 2010; Table 2). The possible explanation was that the YRD is a newly formed wetland, vegetation types and different runoff or tidal flow conditions would also lead to different SOC storage in different regions. The organic carbon storage in the YRD is close to that of the new wetlands, but much lower than the mature wetlands on the eastern coast of the United States (Krull and Craft, 2009). This was because the vegetation of mature wetlands had gradually evolved into more advanced large plants, and more SOC was imported into the soil. This also indicated that wetland protection and restoration will help to improve the YRD’s carbon storage.
The SOC content of the three accumulative hotspots of PA wetlands accounted for 16.69% (0–10 cm), 25.30% (40–60 cm), 11.32% (90–100 cm) of the total soil profile; and 13.97% (0–10 cm), 24.60% (40–60 cm), 12.97% (90–100 cm) in the SS wetlands. The proportion of SOC in the three accumulative hotspots of PA and SS wetlands exceeded 40% (PA: 53.31%, SS: 51.36%). SOC stocks of PV wetlands in the top 10 cm soils accounted for 19.1% of the soil profiles. The SOC storage in the 0–10 cm hot zone showed PV > PA > SS, which may be related to the difference of soil salinity. In the deep hot zone (90–100 cm), it was just the opposite (PV < PA < SS). The results showed that the increase in salinity might accelerate the downward migration of SOC.
The proportion of N in the three accumulative hotspots of PA and SS wetlands exceeded 40% (PA: 59.6%, SS: 52.8%). The proportion of S in the three accumulative hotspots of PA and SS wetlands exceeded 40% (PA: 52.8%, SS: 52.0%). The distribution of P is relatively uniform in the entire section, and only a significant jump occurs in some soil layers. Similar to the Chongming Dongtan wetlands (Jiang et al., 2015), the nitrogen content of the YRD also showed a high peak at a depth of about 10 cm. In addition, according to the C/N comparison with other wetlands, the YRD is a nitrogen-restricted area (Cheng et al., 2021). Compared with PV wetlands, the stocks of C and S in both PA and SS wetlands increased significantly at soil depths below 40 cm. The possible reason was higher historical inputs and underground seawater inputs. Additionally, the root input of these wetland plants is mainly concentrated at a depth above 40 cm. The assimilation and utilization of nutrients by the root system make the soil at 0–40 cm less neutralized by C and S (Wang Z. et al., 2019).
Top concentration factors for three wetlands showed higher stocks of C, N, P, and S in surface soils (0–10 cm), indicating that plant-soil cycling is the dominant factor influencing the biogeochemical processes of nutrients in the YRD. This was in agreement with the results of Bai et al. (2016). The topsoil concentration factor of C, P, and S in PV wetlands was higher than that of PA and SS wetlands. This may be associated with the effects of freshwater and seawater in PV and SS wetlands, respectively. Compared with SS wetland impacted by tidal water, the salinity of SS wetland was higher than that of PV due to the impacts of tidal flooding in SS wetlands with worse plant growth. Appropriate water conditions and lower salinity would have a positive effect on the topsoil concentration factor since water and salinity conditions can greatly influence vegetation covers and plant growth. Therefore, SS wetlands had a lower surface enrichment factor than PV wetlands. Additionally, higher salinity in SS wetlands might enhance the leaching effect of soil nutrients (Wang X. et al., 2019).
Changes in Ecological Stoichiometric Ratios Along Soil Profiles
The C/N ratio of the soil has been proven to be an important indicator for the ability of microorganisms to decompose soil organic matter (Canfora et al., 2017). If C/N < 9 it represents the stage of accelerating the decomposition of soil organic matter; C/N ratio between 9 and 11 indicates a dynamic equilibrium stage; and C/N > 11 can indicate the process of complete humification (Thomsen et al., 2008). Parolari and Porporato (2016) also proposed that when C/N > 10, the mineralization of SOC began to be restricted. Generally, the C/N ratios in this study were within the range of 10–50, except for a few low-value points. This indicated that soil organic matter is in the process of complete humification in the YRD, and the mineralization of organic carbon in this area had been inhibited to a certain extent. Cleveland and Liptzin (2007) observed that the soil C/N ratio was relatively consistent across various ecosystem types, although the soil was characterized by high biological diversity, structural complexity, and spatial heterogeneity (Yang et al., 2010). Our results in the current study showed that the C/N ratio in the surface soils was higher than that in deeper soils in PV and SS wetlands (P < 0.05). Although there was no statistically significant value, the soil C/N ratios tended to decrease with soil depth in PV and SS wetlands. However, the soil C:N ratios were relatively unstable in the PA wetland.
The C/P ratio is usually an indicator for the mineralization ability of soil organic phosphorus, and C/S represents the effect of the microbial biomass of the site on the availability of soil S (Heinze et al., 2010). When the ratios of C/P and C/S in a certain area are high, the limits of P and S during the decomposition of soil organic matter are not conducive for the growth of plants in the area. In contrast, low C/P and C/S ratios will contribute to the release of nutrients during the decomposition of organic matter and increase the available P and S levels in the soil. In addition, Reddy and DeLaune (2008) presented that the initial S mineralization would occur when the C/S ratio is less than 200, and the initial S immobilization will occur when the C/S ratio is in access of 400. In this study, the low ratios of C/P (2–7) and C/S (10–35) indicated that the mineralization of P and S, instead of immobilization, is the main process in the YRD (Figure 4), resulting in lower accumulation in this region. Compared with other coastal wetlands in China, the soil nutrients in the YRD are relatively low (Zhang et al., 2012, 2013), indicating that the exchange of carbon and nutrients between the YRD and the external environment might be very active (Cao et al., 2015). Soil N/P and C/N/P also could be used as indicators of nutrient restriction types. In the current study, The N/P ratios in three wetlands were less than 1.2, which was similar to the results of Gao et al. (2012). Similarly, the C/N/P ratios were between 0 and 0.1. Therefore, the YRD is a nitrogen-limited area, and the accumulated SOC content in this area was relatively less than N and P.
Conclusion
The deposition and ecological stoichiometric of C, N, P, and S were investigated in coastal wetlands along a sampling belt of the YRD in this study. The results showed three accumulative hotspots of C, N, and S in soil profiles in PA and SS wetlands (0–10, 40–60, and 90–100 cm), and one accumulative hotspot of P in SS (10–20 cm) and PA (80–90 cm) wetlands. In PV wetlands, one accumulative hotspot of C, P, and S appeared in the top 10 cm, whereas N at the soil depth of 90–100 cm. Generally, due to the erosion of rivers, higher soil deposition rates and deposition fluxes of C, N, P, and S were observed in PA and SS wetlands compared with PV wetlands. Topsoil concentration factors showed that the area was mainly affected by the input of plant residues, while the leaching effect gradually strengthens with increasing salinities. The ecological stoichiometry further verified that coastal wetlands in the YRD were nitrogen-limited ecosystems and in the stage of accelerating the decomposition of soil organic matter. Improving the degree of salinization in this area, increasing the vegetation coverage of YRD, and reducing the erosion effect of rivers on soil will not only help to improve the ecological stability of this area but also increase the blue carbon storage of YRD and make better use of YRD’s carbon sink function.
Data Availability Statement
The original contributions presented in the study are included in the article/supplementary material, further inquiries can be directed to the corresponding authors.
Author Contributions
SD and CW contributed to the writing of the manuscript. SD, QZ, and JJ involved in the field work and data collection. JB and QZ contributed to concept of study. JB, CW, YG, JJ, GZ, and CY provided important guidance on methods and writing. All authors contributed critically to drafts and gave approval for publication.
Funding
This study was financially supported by the Joint Funds of the National Natural Science Foundation of China (No. U2006215) and the National Natural Science Foundation of China (No. 42107490).
Conflict of Interest
The authors declare that the research was conducted in the absence of any commercial or financial relationships that could be construed as a potential conflict of interest.
Publisher’s Note
All claims expressed in this article are solely those of the authors and do not necessarily represent those of their affiliated organizations, or those of the publisher, the editors and the reviewers. Any product that may be evaluated in this article, or claim that may be made by its manufacturer, is not guaranteed or endorsed by the publisher.
Acknowledgments
We acknowledge all colleagues for their contributions to the fieldwork.
References
Bai, J., Wang, J., Yan, D., Gao, H., Xiao, R., Shao, H., et al. (2012). Spatial and temporal distributions of soil organic carbon and total nitrogen in two marsh wetlands with different flooding frequencies of the Yellow River Delta, China. CLEAN–Soil Air Water 40, 1137–1144. doi: 10.1002/clen.201200059
Bai, J., Yu, L., Du, S., Wei, Z., Liu, Y., Zhang, L., et al. (2020). Effects of flooding frequencies on soil carbon and nitrogen stocks in river marginal wetlands in a ten-year period. J. Environ. Manage. 267:110618. doi: 10.1016/j.jenvman.2020.110618
Bai, J., Zhang, G., Zhao, Q., Lu, Q., Jia, J., Cui, B., et al. (2016). Depth-distribution patterns and control of soil organic carbon in coastal salt marshes with different plant covers. Sci. Rep. 6:34835. doi: 10.1038/srep34835
Canfora, L., Salvati, L., Benedetti, A., and Francaviglia, R. (2017). Is soil microbial diversity affected by soil and groundwater salinity? Evidences from a coastal system in central Italy. Environ. Monit. Assess. 189:319. doi: 10.1007/s10661-017-6040-1
Cao, L., Song, J., Li, X., Yuan, H., Li, N., Duan, L., et al. (2015). Geochemical characteristics of soil C, N, P, and their stoichiometrical significance in the coastal wetlands of Laizhou Bay, Bohai Sea. CLEAN–Soil Air Water 43, 260–270. doi: 10.1002/clen.201300752
Cheng, Q., Chang, H., Yang, X., Wang, D., and Wang, W. (2021). Salinity and nutrient modulate soil bacterial communities in the coastal wetland of the Yellow River Delta, China. Environ. Sci. Pollut. Res. 28, 14621–14631. doi: 10.1007/s11356-020-11626-x
Cleveland, C. C., and Liptzin, D. (2007). C: N: P stoichiometry in soil: is there a “Redfield ratio” for the microbial biomass? Biogeochemistry 85, 235–252. doi: 10.1007/s10533-007-9132-0
Coynel, A., Gorse, L., Curti, C., Schafer, J., Grosbois, C., Morelli, G., et al. (2016). Spatial distribution of trace elements in the surface sediments of a major European estuary (Loire Estuary, France): Source identification and evaluation of anthropogenic contribution. J. Sea Res. 118, 77–91. doi: 10.1016/j.seares.2016.08.005
Cui, B., He, Q., and Zhao, X. (2008). Ecological thresholds of Suaeda salsa to the environmental gradients of water table depth and soil salinity. Acta Ecol. Sin. 28, 1408–1418. doi: 10.1016/s1872-2032(08)60050-5
DeLaune, R. D., Jugsujinda, A., Peterson, G. W., and Patrick, W. H. Jr. (2003). Impact of Mississippi River freshwater reintroduction on enhancing marsh accretionary processes in a Louisiana estuary. Estuar. Coast. Shelf Sci. 58, 653–662. doi: 10.1016/s0272-7714(03)00177-x
Ding, X. G., Wang, J. S., Zhao, G. M., Yuan, H. M., Wang, J., and Ye, S. Y. (2016). Accretion rate and controlling factors of carbon and nutrients during coastal wetland evolution in Yellow River Delta. Geol. Chin. 43, 319–328. doi: 10.12029/gc20160124
Elser, J. J., Acharya, K., Kyle, M., Cotner, J., Makino, W., Markow, T., et al. (2003). Growth rate–stoichiometry couplings in diverse biota. Ecol. Lett. 6, 936–943. doi: 10.1046/j.1461-0248.2003.00518.x
Fan, H., Wu, J., Liu, W., Yuan, Y., Hu, L., and Cai, Q. (2015). Linkages of plant and soil C: N: P stoichiometry and their relationships to forest growth in subtropical plantations. Plant Soil 392, 127–138. doi: 10.1007/s11104-015-2444-2
Feng, J., Zhou, J., Wang, L., Cui, X., Ning, C., Wu, H., et al. (2017). Effects of short-term invasion of Spartina alterniflora and the subsequent restoration of native mangroves on the soil organic carbon, nitrogen and phosphorus stock. Chemosphere 184, 774–783. doi: 10.1016/j.chemosphere.2017.06.060
Gao, H., Bai, J., Xiao, R., Yan, D., Huang, L., and Huang, C. (2012). Soil net nitrogen mineralization in salt marshes with different flooding periods in the Yellow River Delta, China. CLEAN–Soil Air Water 40, 1111–1117. doi: 10.1002/clen.201200031
Heinze, S., Raupp, J., and Joergensen, R. G. (2010). Effects of fertilizer and spatial heterogeneity in soil pH on microbial biomass indices in a long-term field trial of organic agriculture. Plant Soil 328, 203–215. doi: 10.1007/s11104-009-0102-2
Jiang, J., Huang, X., Li, X., Yan, Z., Li, X., and Ding, W. (2015). The Organic Carbon Reserve of Wet Beach Wetlands and Its Relationship with Soil Physical and Chemical Factors– Taking Chongming Dongtan as an Example. J. Ecol. Rural Environ. 31, 540–547.
Krull, K., and Craft, C. (2009). Ecosystem development of a sandbar emergent tidal marsh, Altamaha River Estuary, Georgia, USA. Wetlands 29, 314–322. doi: 10.1672/06-178.1
Lu, Q., Bai, J., Zhang, G., Zhao, Q., and Wu, J. (2018). Spatial and seasonal distribution of carbon, nitrogen, phosphorus, and sulfur and their ecological stoichiometry in wetland soils along a water and salt gradient in the Yellow River Delta, China. Phys. Chem. Earth Parts A/B/C 104, 9–17. doi: 10.1016/j.pce.2018.04.001
Luo, Z., Luo, Y., Wang, G., Xia, J., and Peng, C. (2020). Warming-induced global soil carbon loss attenuated by downward carbon movement. Glob. Change Biol. 26, 7242–7254. doi: 10.1111/gcb.15370
Parolari, A. J., and Porporato, A. (2016). Forest soil carbon and nitrogen cycles under biomass harvest: stability, transient response, and feedback. Ecol. Model. 329, 64–76. doi: 10.1016/j.ecolmodel.2016.03.003
Reddy, K. R., and DeLaune, R. D. (2008). Biogeochemistry of wetlands: science and applications. Boca Raton: CRC press.
Saintilan, N., Rogers, K., Mazumder, D., and Woodroffe, C. (2013). Allochthonous and autochthonous contributions to carbon accumulation and carbon store in southeastern Australian coastal wetlands. Estuar. Coast. Shelf Sci. 128, 84–92. doi: 10.1016/j.ecss.2013.05.010
Salome, C., Nunan, N., Pouteau, V., Lerch, T. Z., and Chenu, C. (2010). Carbon dynamics in topsoil and in subsoil may be controlled by different regulatory mechanisms. Glob. Change Biol. 16, 416–426. doi: 10.1111/j.1365-2486.2009.01884.x
Shi, L., Zheng, L., Mei, X., Yu, L., and Jia, Z. (2010). Characteristics of Soil organic carbon and total nitrogen under different land use types in Shanghai. J. Appl. Ecol. 21, 2279–2287. doi: 10.13287/j.1001-9332.2010.0325
Thomsen, I. K., Petersen, B. M., Bruun, S., Jensen, L. S., and Christensen, B. T. (2008). Estimating soil C loss potentials from the C to N ratio. Soil Biol. Biochem. 40, 849–852. doi: 10.1016/j.soilbio.2007.10.002
Wang, L., Wang, P., Sheng, M., and Tian, J. (2018). Ecological stoichiometry and environmental influencing factors of soil nutrients in the karst rocky desertification ecosystem, southwest China. Glob. Ecol. Conserv. 16, e00449. doi: 10.1016/j.gecco.2018.e00449
Wang, T., Kang, F., Cheng, X., Han, H., and Ji, W. (2016). Soil organic carbon and total nitrogen stocks under different land uses in a hilly ecological restoration area of North China. Soil Till. Res. 163, 176–184. doi: 10.1016/j.still.2016.05.015
Wang, X., Jiang, Z., Li, Y., Kong, F., and Xi, M. (2019). Inorganic carbon sequestration and its mechanism of coastal saline-alkali wetlands in Jiaozhou Bay, China. Geoderma 351, 221–234. doi: 10.1016/j.geoderma.2019.05.027
Wang, Y., Li, Y., Ye, X., Chu, Y., and Wang, X. (2010). Profile storage of organic/inorganic carbon in soil: From forest to desert. Sci. Total Environ. 408, 1925–1931. doi: 10.1016/j.scitotenv.2010.01.015
Wang, Z., Zhang, H., He, C., Liu, C., Liang, X., and Chen, X. (2019). Spatiotemporal variability in soil sulfur storage is changed by exotic Spartina alterniflora in the Jiuduansha wetland, China. Ecol. Eng. 133, 160–166. doi: 10.1016/j.ecoleng.2019.04.014
Wu, H., Lu, M., Lu, X., Guan, Q., and He, X. (2015). Interactions between earthworms and mesofauna has no significant effect on emissions of CO2 and N2O from soil. Soil Biol. Biochem. 88, 294–297. doi: 10.1016/j.soilbio.2015.06.005
Wu, H., Lu, X., Wu, D., Song, L., Yan, X., and Liu, J. (2013). Ant mounds alter spatial and temporal patterns of CO2, CH4 and N2O emissions from a marsh soil. Soil Biol. Biochem. 57, 884–891. doi: 10.1016/j.soilbio.2012.10.034
Yang, Y., Chen, Y., Li, W., and Chen, Y. (2010). Distribution of soil organic carbon under different vegetation zones in the Ili River Valley, Xinjiang. J. Geogr. Sci. 20, 729–740. doi: 10.1007/s11442-010-0807-4
Yang, Y., Fang, J., Ji, C., Datta, A., Li, P., Ma, W., et al. (2014). Stoichiometric shifts in surface soils over broad geographical scales: evidence from China’s grasslands. Glob. Ecol. Biogeogr. 23, 947–955. doi: 10.1111/geb.12175
Yu, J., Wang, Y., Dong, H., Wang, X., Li, Y., Zhou, D., et al. (2013). Estimation of soil organic carbon storage in coastal wetlands of modern Yellow River Delta based on landscape pattern. Wetl. Sci. 11, 1–6. doi: 10.13248/j.cnki.wetlandsci.2013.01.006
Zhang, Z. S., Song, X. L., Lu, X. G., and Xue, Z. S. (2013). Ecological stoichiometry of carbon, nitrogen, and phosphorus in estuarine wetland soils: influences of vegetation coverage, plant communities, geomorphology, and seawalls. J. Soil. Sediment. 13, 1043–1051. doi: 10.1007/s11368-013-0693-3
Zhang, Z., Lu, X., Song, X., Guo, Y., and Xue, Z. (2012). Soil C, N and P stoichiometry of Deyeuxia angustifolia and Carex lasiocarpa wetlands in Sanjiang Plain, Northeast China. J. Soil. Sediment. 12, 1309–1315. doi: 10.1007/s11368-012-0551-8
Zhao, Q., Bai, J., Lu, Q., and Zhang, G. (2017). Effects of salinity on dynamics of soil carbon in degraded coastal wetlands: implications on wetland restoration. Phys. Chem. Earth, Parts A/B/C 97, 12–18. doi: 10.1016/j.pce.2016.08.008
Keywords: water and salt conditions, deposition flux, ecological stoichiometry, topsoil concentration factor, soil profiles
Citation: Du S, Bai J, Zhao Q, Wang C, Guan Y, Jia J, Zhang G and Yan C (2022) Deposition Flux, Stocks of C, N, P, S, and Their Ecological Stoichiometry in Coastal Wetlands With Three Plant Covers. Front. Ecol. Evol. 10:840784. doi: 10.3389/fevo.2022.840784
Received: 21 December 2021; Accepted: 18 February 2022;
Published: 21 March 2022.
Edited by:
Haitao Wu, Northeast Institute of Geography and Agroecology (CAS), ChinaReviewed by:
Xu Chen, China University of Geosciences Wuhan, ChinaWeiqi Wang, Fujian Normal University, China
Jiang Bao Xia, Binzhou University, China
Copyright © 2022 Du, Bai, Zhao, Wang, Guan, Jia, Zhang and Yan. This is an open-access article distributed under the terms of the Creative Commons Attribution License (CC BY). The use, distribution or reproduction in other forums is permitted, provided the original author(s) and the copyright owner(s) are credited and that the original publication in this journal is cited, in accordance with accepted academic practice. No use, distribution or reproduction is permitted which does not comply with these terms.
*Correspondence: Junhong Bai, anVuaG9uZ2JhaUAxNjMuY29t; Chen Wang, d2FuZ2NoZW45MEBibnUuZWR1LmNu