- 1School of Chemical Engineering and Technology, Tianjin University, Tianjin, China
- 2School of Chemical Engineering, Hebei University of Technology, Tianjin, China
- 3Zhejiang Institute of Tianjin University, Shaoxing, Zhejiang, China
Rapid industrial development has led to excessive levels of various contaminants in natural water, which poses a challenge to the innovation of environmental remediation technology. In recent years, iron sulfide and its modified materials have attracted extensive attention in environmental remediation due to their high activity in advanced oxidation processes and widespread existence in anoxic environment. This paper reviewed the latest advances of the synthesis methods for iron sulfide and modified FeS. In addition, the application of persulfate activation by iron sulfide materials (FeS, FeSx, S−ZVI, FeS@Carbon materials and MFexSy) for contaminants remediation is also reviewed, and the enhancement of this system by photo irradiation, ultrasound, and microwave have also been concluded. Additionally, the interaction mechanism of iron sulfide and persulfate with contaminants was reviewed. Based on the above contents, we concluded that the long−term stability of iron sulfide, the toxicity to organisms of iron sulfide materials in the treated water, and the combination of FeS/PS with other assisted technologies should be focused in future.
1 Introduction
As one of the global environmental problems, water pollution caused by various organic pollutants (such as drugs and metabolites, endocrine disrupting chemicals (EDCs), dyes, plastic additives, pesticides, antibiotics, etc.) is increasingly serious (Petrie, et al., 2015; Pan, et al., 2018; Li, et al., 2019a; Liu, et al., 2019; Bhatt, et al., 2021; Wang, et al., 2021). Advanced oxidation processes based on persulfates (PS−AOPs) can degrade refractory contaminants effectively via radical or non−radical routes, the related reactive oxidation species (ROSs) include SO4•−, •OH, O2•−, 1O2, high valent metals, and electron−transfer process.
Various methods such as ultrasonic (Hao, et al., 2014), thermal (Qi, et al., 2014; Wang and Wang, 2018), electric (Silveira, et al., 2017), photo irradiation (Lin and Wu, 2014) and transition metal (Anipsitakis and Dionysiou, 2004; Wu, et al., 2022) activation have been developed to activate persulfates for pollutant degradation, among which Fe−based catalysts have wider application due to their high reactivity, stability, low cost, environmentally friendly, and simple synthesis (Li, et al., 2021a; Hou, et al., 2021; Liu, et al., 2022a).
As a rate−limiting step in Fe−mediated PS−AOPs, the low regeneration rate from Fe (III) to Fe (II) (0.002–0.01 M−1 s−1) decrease the efficiency of the catalytic reaction (Wang, et al., 2014). Therefore, many strategies have been proposed to accelerate Fe (III)/Fe (II) cycling, such as combining bimetals or adding some reductants (Hou, et al., 2016; Luo, et al., 2020; Xiang, et al., 2020; Zhang, et al., 2022). However, the synthesis of those composite materials is complex and expensive. Therefore, catalyst with auto−enhanced effect may be more promising (Cai, et al., 2022a).
Iron sulfide (FeS), also known as mackinawite, is a kind of tetragonal system common non−toxic mineral (Gong, et al., 2016). With unique molecular structure and surface chemistry, FeS is very effective in fixing divalent metals such as Fe2+, Mn2+, Ca2+, Mg2+, Ni2+, Cd2+ and Hg2+ (Wharton et al., 2000; Mariëtte, et al., 2003). Due to the reducibility of FeS, both Fe (II) and S (−II) can act as electron donors. Therefore, both surface−bound Fe (II) and self−released dissolved Fe2+ in FeS can serve as continuous iron sources, which can be used to activate persulfate to generate free radicals (Eqs.1, 2) (Fan, et al., 2018a). In addition, the lattice S (−II) on FeS surface can also provide electrons to Fe (III), which can effectively alleviate the excessive consumption of Fe2+ and accumulation of Fe3+ to maintain the Fe (III)/Fe (II) cycle on the catalyst surface (Eq. 3) (Hou, et al., 2022). Therefore, FeS have been used in recent years to activate PS for the degradation of organic pollutants (Sühnholz, et al., 2021; Xiang, et al., 2022).
The purpose of this paper is to focus on the synthesis, modification, and application of iron sulfide to activate PS for pollutants degradation in recent years. Specifically, this review aims to: 1) review the synthesis methods of iron sulfide particles; 2) Clarify the activation mechanism of iron sulfides for PMS to degrade organic pollutants; and 3) Put forward the existing knowledge gap in exist research and the prospect of future research.
2 Synthesis methods of iron sulfide
2.1 The synthesis of FeS and other FeSx
FeS particles are usually synthesized by physicochemical and biochemical methods. Physicochemical method is the co−precipitation of different types of iron salts and sulfide salts in the aqueous solution under the condition of hypoxia (Eq. 4). Common source of Fe2+ include ferrous chloride (FeCl2), ferric sulfate heptahydrate (FeSO4·7H2O), and ammonium ferrous sulfate (Fe (NH4)2·(SO4)2·6H2O). Sodium sulfide (Na2S) is usually used as the source of S2− (Chen, et al., 2019).
Biosynthetic FeS has much attracted attention due to its environmental friendliness. In general, ferroreductive bacteria (FRBS) and sulfate−reducing bacteria (SRBS) reduce Fe3+ and sulfur substances (including sulfate, thiosulfate and elemental sulfur) to S2− and Fe2+ respectively, and subsequently to produce FeS nanoparticles (Xie, et al., 2013; Zhou, et al., 2017).
However, FeS particles prepared by traditional methods, especially co−precipitation, tend to rapidly aggregate, which greatly reduces their specific surface area and leads to significant reduction in pollutant removal efficiency (Gong, et al., 2012). Therefore, the preparation of dispersible FeS nanoparticles with small particle size, large specific surface area and high reactivity has attracted extensive attention. Previous studies have explored various techniques to prepare FeS nanoparticles with controllable particle morphology and size distribution, such as reverse micelles (Ajay, et al., 1996), SRB−assisted production (Watson, et al., 2001), high−energy mechanical grinding (Soori, et al., 2016), wet chemical synthesis (Paknikar, et al., 2005), etc.
Recent studies have shown that the presence of polymer stabilizers and surfactants during the synthesis of FeS particles can effectively control the nucleation and growth of nanoparticles through simultaneous electrostatic repulsion and steric hindrance, thus effectively promoting the size control of FeS in aqueous solution. This can not only reduce the aggregation of nanostructured materials, but provide a large number of functional groups to degrade pollutants (Shao, et al., 2016). For example, polyelectrolyte stabilizer carboxymethyl cellulose (CMC) is widely used to modify nanoparticles to enhance the stability of nano FeS due to a large number of carboxylic and hydroxyl groups in its macromolecular chains (Xiong, et al., 2009; Gong, et al., 2012; Gong, et al., 2014; Van Koetsem, 2016). Additionally, other macromolecular biomaterials with similar physical and chemical properties, such as starch, cyclodextrin (CD), chitosan, polysaccharide sodium alginate (SA), etc., have also been used as stabilizers to synthesize nano FeS particles (Shao, et al., 2016; Wu, et al., 2017; Sun, et al., 2018a; Sun, et al., 2018b). The mechanism and processes of synthesis of iron sulfide are exhibited in Figures 1A, B.
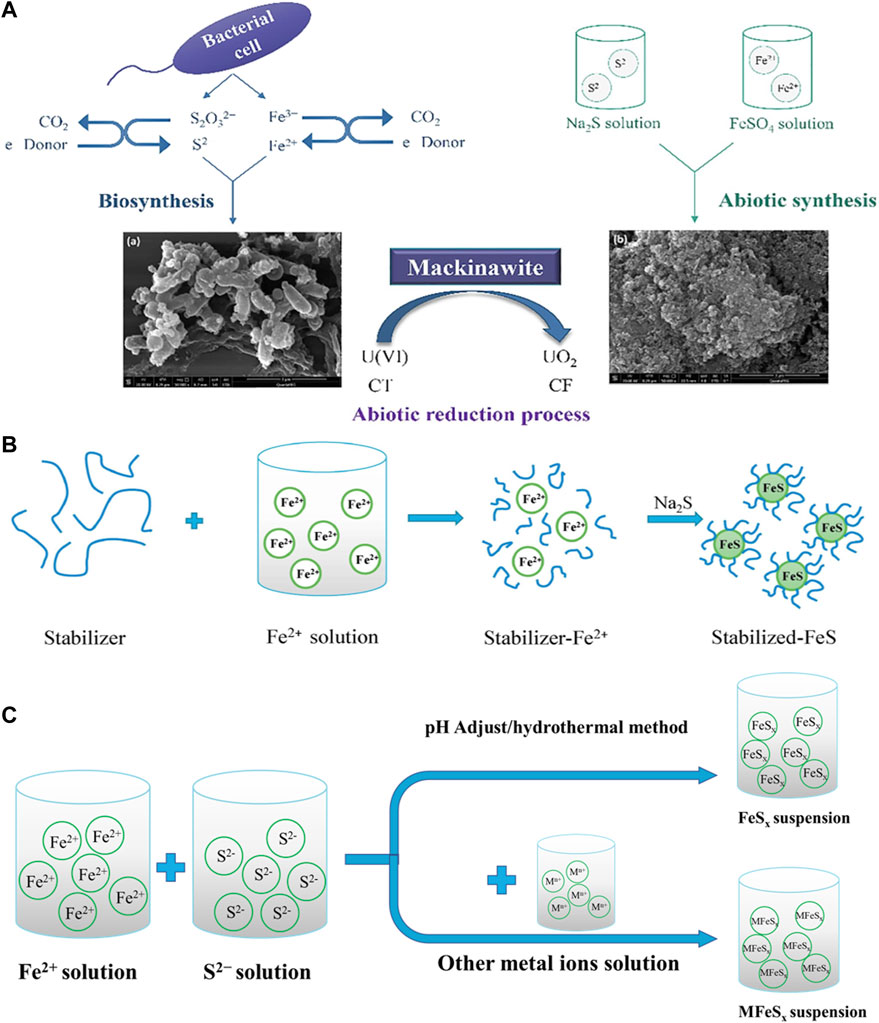
FIGURE 1. (A) The mechanism of biological and abiotic synthesis of FeS. (B) The modified methods of nano−sized FeS particles by coating stabilizers. Adapted with permission from (Chen et al., 2019). Copyright 2019 Elsevier. (C) The mechanism of synthesis of FeSx and multicomponent iron sulfide.
In aqueous solution, the reducibility of ferrous ions will decrease with the pH value decreasing, which means acidic environment can inhibit the oxidation of ferrous ions to ferric ions (Liu, et al., 2022b). Therefore, the elemental valence state of the synthesized iron sulfide catalysts can also be adjusted. Liu et al. (Liu, et al., 2022a) obtained three kinds of iron sulfides by controlling the pH of the co−precipitation system of iron and sulfide salt to synthesize FeS2, Fe7S8, Fe3S4 at
Because of the synergistic effect between zero−valent iron (ZVI) and FexSy (FeS and FeS2), S−ZVI has high reactivity and selectivity for PS activation to degrade refractory contaminants (Li, et al., 2017a; Wei, et al., 2022). S−ZVI can be synthesized by the “one−pot” process, where ferrous ions react with borohydrides and sulfides. While in the two−step process, sulfur compounds (sodium sulfide and thiosulfate) are added to the synthesized nZVI to form S−ZVI (Li, et al., 2017b). For S−ZVI prepared by one−pot process, FeSx are distributed both inside and on the surface of ZVI particles, while prepared by two−step method, FeSx are only formed on the surface of ZVI to form a core−shell structure (Su, et al., 2018; Xu, et al., 2019). In addition, vulcanized micro−scale S−ZVI can be prepared by ball−milling S and ZVI powders under dry conditions (Gu, et al., 2017; He, et al., 2022). The structure, physicochemical properties and performance in PS based Fenton−like reactions of S−ZVI produced by various synthesis methods are mainly affected by the molar ratio of S/Fe (Fan, et al., 2017; Zhang, et al., 2020). Kim et al. (Kim, et al., 2011) reported that the degradation rate of TCE by S−nZVI increased linearly with increasing molar ratio of S/Fe, but decreased with increasing molar ratio of S/Fe when the concentration of Na2S2O4 increased to 2.0 g/L (S/Fe molar ratio was 0.33). However, Han et al. (Han and Yan, 2016) reported that when S/Fe ratio is smaller than 0.025, the degradation rate of TCE becomes faster with the increase of S/Fe molar ratio, and then becomes stable when the molar ratio of S/Fe molar ratio exceeds 0.025. Rajajayavel et al. (Rajajayavel and Ghoshal, 2015) showed that the reducing ability of S−nZVI on TCE was strongly dependent on the S/Fe molar ratio, which provided the highest TCE dechlorination rate in the range of 0.04–0.083. Since the reaction conditions (including synthesis methods, reaction conditions and the properties of the target stain, etc.) used by different researchers to obtain the optimal S/Fe molar ratio are very different, the optimal S/Fe molar ratio values in different studies are not comparable. Based on the above examples, higher S/Fe molar ratio results in more production of FeSx and a larger surface area of the synthesized S−ZVI, thus facilitates the pollutants degradation (Gu, et al., 2017; Huang, et al., 2017). However, excess S content blocks the active Fe site on the surface, which will decrease their activity for PS activation. In addition, the conversion of Fe0 to ferric/ferrous (hydrogen) oxides may be intensified at high S/Fe ratio, leading to waste of ZVI with strong reducing capacity (Li, et al., 2017a). Therefore, it is essential to find the optimal S/Fe molar ratio in different reaction systems. The mechanism and processes of synthesis of S−ZVI are exhibited in Figure 2A.
2.2 The synthesis of carbon modified FeS
The large surface area of carbon material can greatly reduce the agglomeration of iron−based nanoparticles, thus improving the activity of catalyst. Additionally, strong electron transfer capacity of carbon material can greatly enhance the electron transfer rate of iron−based catalyst (Li, et al., 2018; Ma, et al., 2021). Due to environmentally friendly, good surface physical and chemical properties, and rich oxygen−containing functional groups. Biochar (BC), graphene/graphene oxide (GO)/reductive graphene oxide (rGO), carbon nanotubes (CNTs), graphite carbon nitride (g−C3N4), etc., are commonly selected as supports to load FeS. They have been proven to significantly reduce the aggregation of FeS, resulting in better catalytic activity (Ma, et al., 2015; Sun, et al., 2020; Lyu, et al., 2018a; Zhang, et al., 2019a; Bin, et al., 2020; Zhuang, et al., 2020; Han, et al., 2022; Xu, et al., 2022). Generally, the composite of FeS and carbon materials can be obtained by loading iron salt on carbon materials firstly followed with sulfidation process, and freeze−drying, ultrasound or mechanical stirring are widely used loading methods (Sun, et al., 2020; Han, et al., 2022; Xu, et al., 2022). Specifically, these hybrids can be synthesized by mixing carbon materials with ferrous salt and sulfide and then reacted at high temperature under hypoxia condition (Ma, et al., 2015; Lyu, et al., 2018b; Hong, et al., 2021), or carbon materials mixed with pre−synthesized iron sulfide and then treated by hydrothermal method (Li, et al., 2022a). In addition, ball−milling can also achieve the combination of FeS with some carbon materials (Lyu, et al., 2018b; He, et al., 2021; Xia, et al., 2022). The mechanism and processes of synthesis of FeS@Carbon materials are exhibited in Figure 2B.
2.3 The synthesis of multicomponent iron sulfide
Multicomponent iron sulfides exhibit better electrochemical and catalytic properties due to the synergistic effect between metal ions and redox reactions. It can be synthesized by improved hydrothermal methods. Li et al. (Li, et al., 2020) mixed FeCl2, Co (NO3)2, NH4F and g−C3N4 powders at 25°C for 30 min, then heated the mixture in hydrothermal reactor at 140°C for 9 h. Put the Fe−Co precursor and thionoacetamide solution in hydrothermal reactor at 160°C for 6 h, FeCo2S4−CN composite can then be obtained. Nie et al. (Nie, et al., 2019) mixed CuCl, FeCl3·6H2O and (NH4)2S for 30 min, and heated at 200°C for 10 h in hydrothermal reactor to obtain CuFeS2 NPs. Yan et al. (Yan, et al., 2020) loaded ferric nitrate, cobalt nitrate hexahydrate, ammonium fluoride, urea solution, and reduced graphene oxide film (RGOF) in a hydrothermal kettle at 120°C for 8 h, and then placed the obtained Fe−Co precursor and sodium sulfide solution in the hydrothermal reactor at 160°C for 8 h to obtain FeCo2S4/RGOF composite material. The mechanism and processes of synthesis of multicomponent iron sulfide are exhibited in Figure 1C.
3 Applications and mechanism of iron sulfides in AOPs
3.1 Bare FeS
Among various iron sulfides, FeS and FeS2 are the most commonly used materials for persulfate activation. Since FeS is widely distributed in anoxic environment, and has strong reducibility and high reactivity to organic pollutants, it has been widely used as PS activators for water remediation. As shown in Figure 3, Xu et al. (Xu and Sheng, 2021) used FeS/PMS system to degrade chloramphenicol (CAP), sulfoxamycin (TAP), ciprofloxacin (CIP) and norfloxacin (NOR), and 100% NOR, 100% CIP, 93.5% CAP and 98.5% TAP was degraded within 2 h. Fan et al. (Fan, et al., 2018b) used FeS/PDS system to degrade PCA, and PCA degradation reached nearly 100% in acidic conditions within 240 min. And Sarah et al. used FeS as PDS activator and reached nearly complete removal of TCE within 20 min. These results showed that the FeS/PS system can effectively remediate the organic pollutants containing wastewater. In addition, the catalytic performance of iron−based catalysts in PS based Fenton−like reactions is summarized in Table 1.
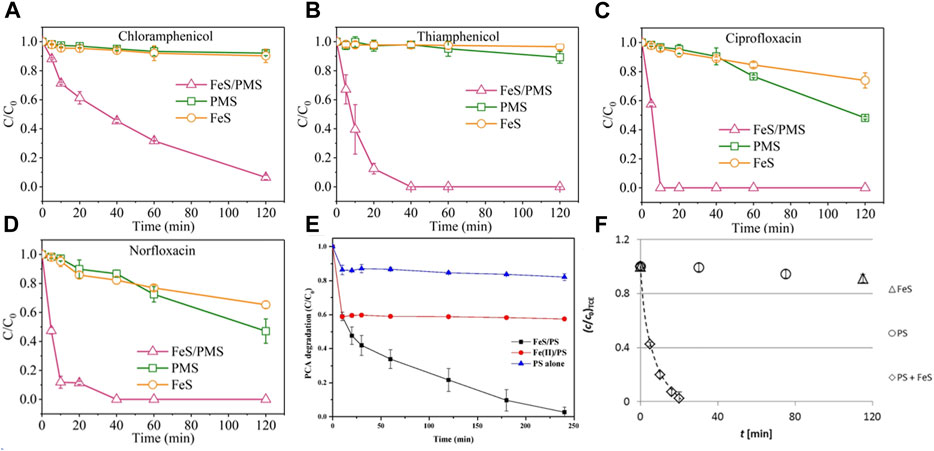
FIGURE 3. Degradation of four antibiotics in the FeS/PMS, PMS and FeS systems (A) CAP (B) TAP (C) CIP (D) NOR. Conditions [Contaminants]0 = 30 μM [PMS]0 = 6 mM [FeS]0 = 0.6 g/L, initial
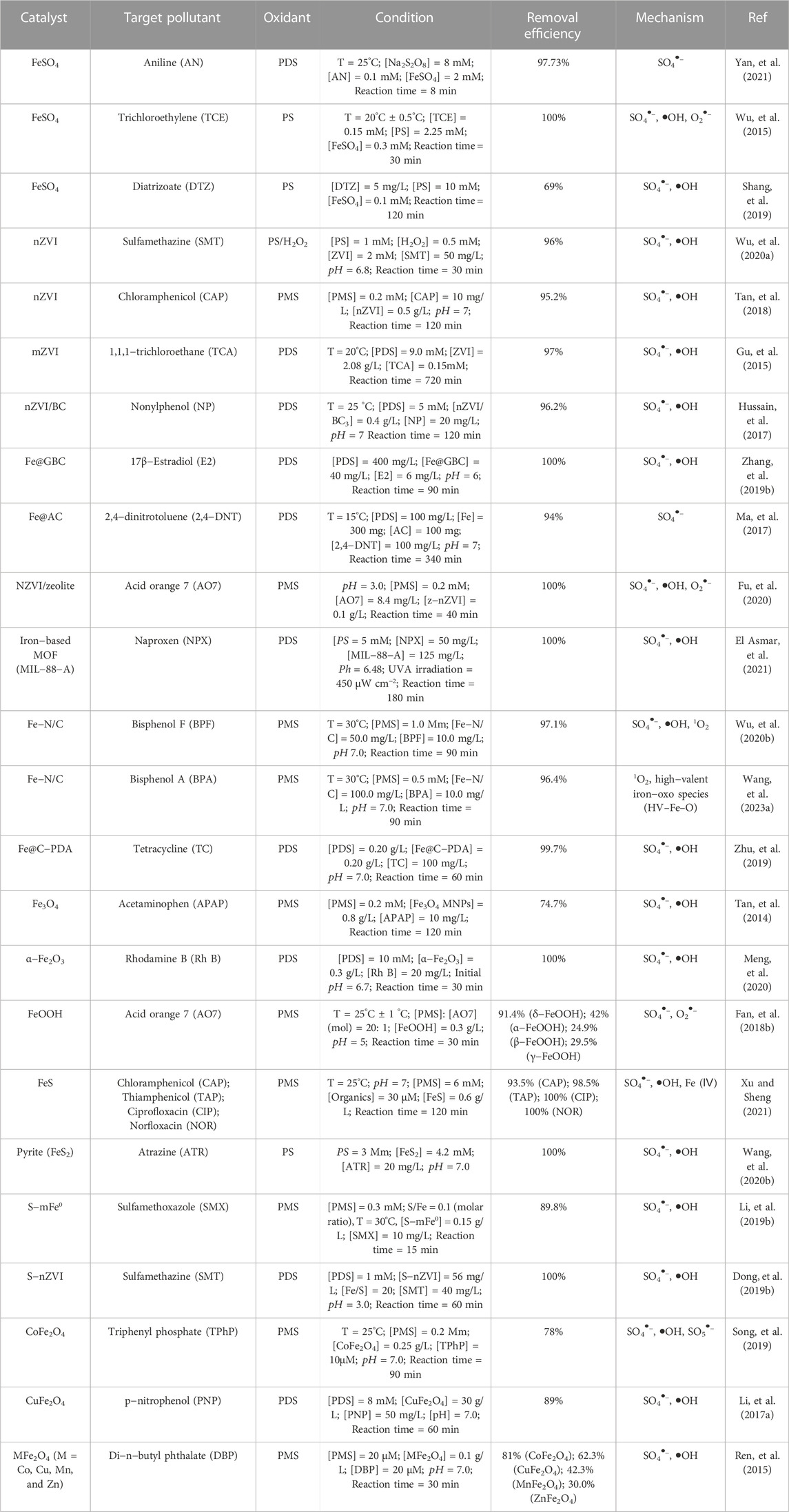
TABLE 1. Summary of the reported work on the activation of persulfates by Fe−based catalysts for the removal of target pollutant.
So far, the mechanism of PS activation through FeS has been considered to be homogeneous and heterogeneous activation. Homogeneous activation refers to the continuous release of dissolved Fe2+ by FeS for persulfate activation, and the slow release of Fe2+ by FeS can effectively inhibit the self−quenching effect on SO4•− to promote the degradation of pollutants (Eqs. 5, 6). Heterogeneous activation refers to the surface of FeS combines Fe (II) or structural ≡Fe (II) for persulfate activation (Yuan, et al., 2015; Chen, et al., 2017; Fan, et al., 2018a; Sühnholz, et al., 2022). Fan et al. (Fan, et al., 2018b) found the presence of binding free radicals (≡SO4•−) on the surface of the catalyst through radical quenching experiments, indicating the activation of persulfate by structural Fe (Ⅱ). In addition, it was found that Saq2− ion itself could not activate PS to produce oxidation radicals (Eq. 7) (Oh, et al., 2011), but SO42−, S0, polysulfide (Sn2−) and S− were detected on the surface of FeS after PS activation by X−ray photoelectron spectroscopy (XPS) and FT−IR analysis (Eq. 3 and Eqs. 8, 9), indicating that S (−Ⅱ) in FeS can indirectly provide electrons to PS by facilitating the reduction of Fe (III) to Fe (II). Once S2− is exhausted, Fe (II) regeneration via PS reduction will dominate, since S2− is non−renewable in FeS/PS systems (Eq. 10). In addition, Xu et al. (Xu and Sheng, 2021) also demonstrated that Fe (Ⅳ) was generated in the FeS/PS system, but its contribution to pollutant degradation as reactive species is not significant.
Therefore, the mechanism of FeS for persulfate activation can be proposed in Figures 4A, B, which is also explained as follows:
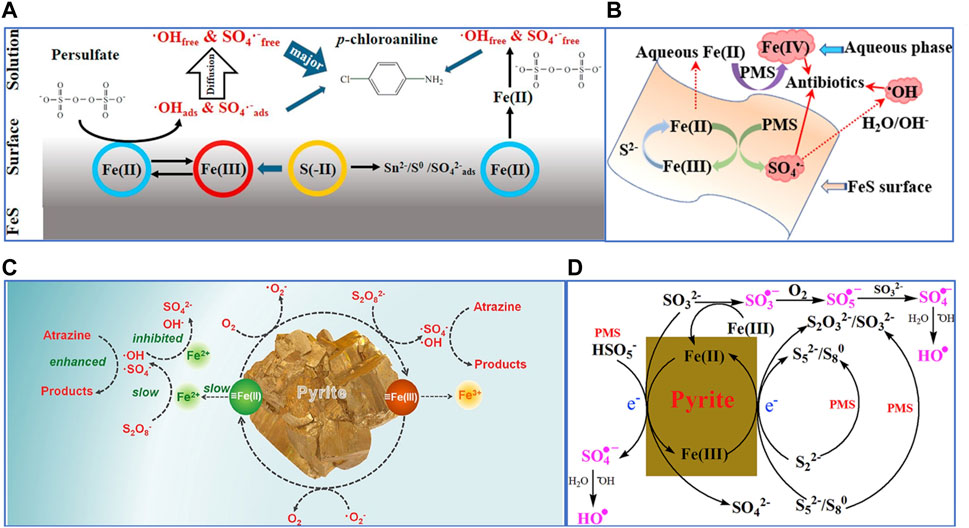
FIGURE 4. (A) The catalytic mechanism of mackinawite/PDS system. Adapted with permission from (Fan, et al., 2018a). Copyright 2018 Elsevier. (B) The possible mechanism of reactive species generation in the FeS/PMS system. Adapted with permission from (Xu and Sheng, 2021). Copyright 2021 Elsevier. (C) Degradation mechanism of atrazine by pyrite/PS. Adapted with permission from (Wang, et al., 2020c). Copyright 2020 Elsevier. (D) Proposed pathways of PMS activation by pyrite. Adapted with permission from (Zhou, et al., 2018). Copyright 2018 Elsevier.
Homogeneous activation process: Firstly, FeS release Fe2+ ions (Eqs. 11, 12), which can activate PS to form SO4•− (Eqs. 1, 2). The Fe3+ can be reduced to Fe2+ by reacting with FeS or S2− (Eq. 3, 13). Finally, the regenerated Fe2+ continue to maintain PS activation for pollutant degradation.
Heterogeneous activation process: ≡Fe (II) in FeS activates PS as electron donor to produce SO4•−(Eqs. 1, 2), then ≡S2− and HS− adsorbed on the surface of FeS can also give electrons to ≡Fe (III) and reduce it to ≡Fe (II), ensuring that the heterogeneous activation process can be continued (Eqs. 14, 15) (Yang, et al., 2022).
In addition, it is worth mentioning that solution pH is one of the most significant influencing factors in the remediation of contaminants by nano−sized FeS, which not only plays an important role in the decomposition of oxidants, but affects the surface charge of FeS−based catalysts and the speciation of substrates to be transformed (Chen, et al., 2019; Li, et al., 2021b). In general, when the solution pH is greater than the pH value at point of zero charge (pHpzc), the surface of the catalyst is negatively charged, otherwise positively charged (Li, et al., 2020). Under strongly alkaline or strongly acidic conditions, the adsorption capacity is significantly reduced, which not only destroys the active sites on surface and accelerates the corrosion of FeS nanoparticles, but promotes the hydrolysis of bio−modifiers, thus decrease the stability and dispersion of modified nano−FeS. In addition, under strongly alkaline conditions, Fe (II) species will be reduced due to the precipitation of ferric hydroxide in reaction system and produce passivation layer on the surface of FeS. Additionally, negatively charged hydroxide ions compete for absorption with other negatively charged contaminants, which will influence the degradation of contaminants by FeS. Therefore, neutral conditions can provide optimal degradation of pollutants with FeS materials as catalysts for PS.
3.2 FeSx
FeS2 can also effectively activate persulfate to degrade pollutants, and the good persulfate activation performance is attributed to the low−valent Fe and S (Fe2+ and S−1) (Hou, et al., 2021). By being fully oxidized to SO42− and Fe3+, FeS2 can provide 15 electrons. Therefore, FeS2 can slowly and sustainably releases dissolved Fe2+, which activates persulfates to produce reactive free radicals to degrade pollutants (Eqs. 1, 2). Fe2+ comes from the water corrosion process of FeS2 (Oh, et al., 2011), and Fe2+ will activate persulfate to be consumed, which will accelerate the water corrosion reaction of FeS2 (Eq. 10 and Eqs. 16–18). ≡S−1 could also give electrons to persulfate or Fe (III), which would cause Fe2+ to continue to form (Liang, et al., 2010). In addition, surface−bound ≡Fe (Ⅱ) can activate molecular oxygen to produce O2•− through single electron transfer pathway (Liu, et al., 2015), while surface−bound ≡Fe (Ⅱ) is also reduced, allowing the degradation to continue. The mechanism of FeS2 for persulfate activation can be proposed in Figures 4C, D.
S−ZVI has been widely used in activating persulfate to degrade refractory organic pollutants, such as tetracycline (Dong, et al., 2019a), bisphenol S (Cai and Zhang, 2022), tetrabromobisphenol A (Quoc, et al., 2021), trichloroethylene (Zhou, et al., 2021a), sulfadiazine (SDZ) (Guo, et al., 2020), etc., indicating that S−ZVI/PS system can effectively treat organic wastewater.
S−ZVI has high electron utilization efficiency, 10–50 times larger than that of unsulfide ZVI. Its sulfide layer can significantly enhance the activity of ZVI and promote to release ferrous ions into the environment (Fan, et al., 2016; Fan, et al., 2018b). This is because there are delocalized electrons in the FeS layer, which has good electrical conductivity and facilitates the transfer of electrons from Fe0, thus accelerating the ferrous ions formation (Kim, et al., 2011; Song, et al., 2017). According to electrochemical test, the results of Tafel curve and electrochemical impedance spectroscopy (EIS) also confirm that S−ZVI supports better electron transfer (Turcio−Ortega, et al., 2012; Wang, et al., 2019). Hence, the sulfide layer mainly acts as conductor of electrons to promote the release of Fe2+ during the reaction process, rather than source of Fe2+ production, which can be used to activate persulfate.
In addition to promoting the release of ferrous ions, the sulfur compounds in the sulfide layer have strong reducing capacity, which can reduce Fe3+ to Fe2+ (Eq. 3), and these ferrous species will be subsequently used to activate persulfate based on the electron transfer capacity of the sulfide layer (Fan, et al., 2018a; Li, et al., 2019a). Fe0 in S−ZVI can also reduce Fe (III) to Fe (Ⅱ) (Eq. 19), and a small amount of FeS2 in S−ZVI can react with water to produce Fe2+ to further enhancing the iron cycle (Eq. 20). The cycle of iron species enables the degradation reaction to be carried out contumely and efficiently (Liu, et al., 2015). The mechanism of S−ZVI for persulfate activation can be proposed in Figure 5.
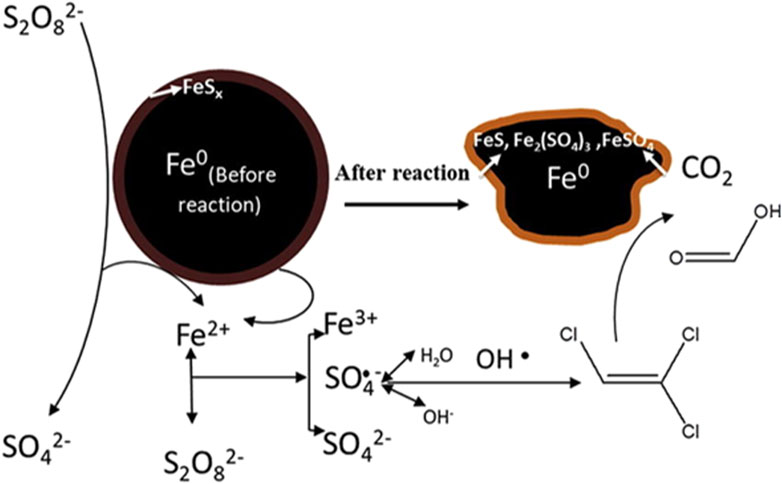
FIGURE 5. Possible degradation mechanism of TCE in S−ZVI/PS system. Adapted with permission from (Dong, et al., 2019a). Copyright 2019 Elsevier.
3.3 Carbon modified FeS
FeS@Caobon materials has been widely used to activate persulfate for degradation of organic pollutants, such as petroleum hydrocarbons (Xia, et al., 2022), 2, 4−dichlorophenoxyacetic acid (Hong, et al., 2021), tetracycline (He, et al., 2021), sulfamethazine (Jin, et al., 2022), etc., indicating the potential of FeS@Caobon/PMS system in wastewater remediation.
It has been reported that in heterogeneous activation systems, radicals are first produced near the surface of the activator and then diffused into the solution to degrade pollutants (Liu, et al., 2014), but it has also been reported that both activation and degradation processes may occur near the surface of the carbon−based activator (He, et al., 2019). By measuring the levels of dissolved ion (dissolved Fe2+ and total Fe) in the reaction system, and using hydrophobic phenol and 1, 10−phenanthroline to chelate with surface−bound Fe (II) and dissolved Fe2+ as quenchers, confirming that the active radicals were mainly generated on the Fe@Carbon surface (He, et al., 2021; Han, et al., 2022). According to the XPS results of catalysts before and after the reaction, the ratio of Fe2+ to S2− decreased significantly after the reaction, while that of other sulfur species such as Sn2− and SO42− increased, indicating that Fe (II) and S (−II) species were involved in the reaction process, and S (−II) contribute to the conversion of Fe (III) and Fe (II), which will further enhance the activation of PS (Xia, et al., 2022; Yu, et al., 2022). All of these results suggest that surface−bound Fe (II) plays an important role in the PS activation process and generates ROSs on the FeS@Carbon surface. In addition, a small amount of Fe2+ dissolved in solution can also directly activate persulfate to produce free radicals (He, et al., 2021).
During the process of contaminants degradation in FeS@Carbon/PS system, carbon materials can prevent the agglomeration of FeS particles to make FeS particles evenly dispersed, which increase the chance of catalyst contact with solution and further increase the concentration of sustainably released Fe2+ (Wang, et al., 2017). Besides, the adsorption capacity of carbon materials can make contaminants adsorbed on the surface or inside of composite materials, which make the free radicals produced easier to contact with contaminants (Qu, et al., 2022). Additionally, carbon material itself can act as intermediary of electron transport to accelerate the electron transfer process and improve the degradation efficiency (Qiu, et al., 2021), CNTs and BC has strong electron donor groups on surface, such as hydrogen peroxide (−OOH) and hydroxyl (−OH), which can also activate persulfate to produce more radicals (Eqs. 21−24) (Zhou, et al., 2020; Qiu, et al., 2022). In the composite of FeS and graphene, the bond length between FeS and graphene layer is relatively long, which indicates the weaker bonding, resulting in the higher activity of S to react with SO52− during PMS activation. With long bond length, greater electron localization could be facilitated on the S sites to reduce the barrier of pollutant bonding and accelerate the regeneration of metal species (Zhuang, et al., 2020).
Therefore, there are three possible mechanisms of FeS@Carbon to activate persulfate: 1) the adsorption of contaminants by FeS@Carbon; 2) The active sites on catalyst surface, such as oxygen−containing functional groups, Fe2+, S2−, etc. act as electron donors in reaction process to activate PS and then produce ROSs for pollutants degradation (Eqs. 21−30). In addition, S2− also participates in the reduction of Fe3+, enabling the continuous generation of Fe2+, which can be further used for the activation of PS (Eqs. 1−3); 3) Carbon materials can accelerate the electron transfer process, which promote the electron transfer from pollutants to PS and further improve the generation rate of radicals on catalyst surface. The mechanism of FeS@Carbon for persulfate activation can be proposed in Figure 6.
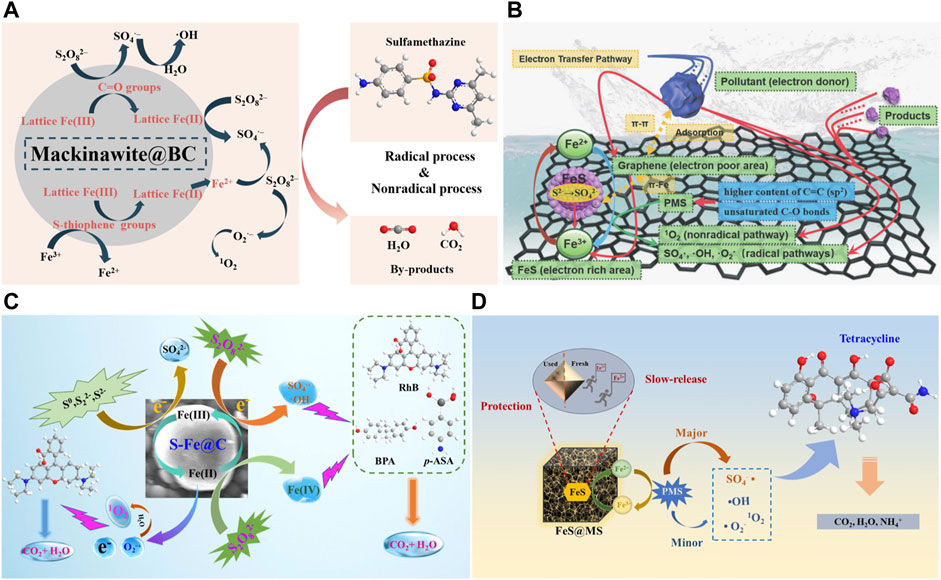
FIGURE 6. (A) Possible degradation mechanism of sulfadimethacil (SMT) in FeS@BC/PS system. Adapted with permission from (Jin, et al., 2022). Copyright 2022 Elsevier. (B) Mechanism of tetracycline removal by an FeS/graphene−based catalyst (DMG). Adapted with permission from (Zhuang, et al., 2020). Copyright 2020 Royal Society of Chemistry. (C) Proposed mechanism for various contaminants degradation in the S−Fe@C/PDS system. Adapted with permission from (Yu, et al., 2022). Copyright 2022 Elsevier. (D) Possible mechanism of peroxymonosulfate activation by FeS@MS for TC degradation. Adapted with permission from (Han, et al., 2022). Copyright 2022 Elsevier.
3.4 Multicomponent iron sulfide
Compared with single−component sulfide, multi−component metal sulfide exhibits better catalytic performance due to its richer redox reactions and synergistic effects between metals (Li, et al., 2019c). Moreover, as an electron donor, the low electronegativity of S2− can promote the redox cycle of metal ions, making multi−component metal sulfide an effective catalyst for the activation of PMS. CuFeS2 (Nie, et al., 2019), NiFe2S4 (Fan, et al., 2022), Cu2FeSnS4 (CFTS) (Li, et al., 2022a), CoFe2S4 (Li, et al., 2022b) etc., have been widely used in the field of persulfate activation to degrade organic pollutants, showing good pollutant removal effect.
During the process of persulfate activation by polymetallic sulfide, Cu+, Co2+, Fe2+, Ni2+ act as active sites to accelerate the generation of radicals such as SO4•−, •OH, O2•− by destroying the O−O bond of PS, and they are themselves oxidized into Ni3+, Fe2+, Ni3+, Co3+. (Eqs. 31, 32). Due to the strong reducibility of sulfur species such as S2− and S22−, the high valent metal ions formed can be reduced to low valent states (Eqs. 33−35). The reduction of Fe3+ by Cu+ and Ni2+ is easy to achieve, which is thermodynamically advantageous (Eqs. 36, 37) (Nie, et al., 2019; Fan, et al., 2022). Therefore, the synergistic interaction between these metals on the catalyst surface facilitates interfacial electron transfer. In addition, ROSs produced by hydrolysis of persulfate can also oxidize high−valent metals to low−valent metals (Eq. 10), and the regenerated active sites such as Cu+, Ni2+ and Fe2+ on the surface can again participate in the continuous generation of ROSs induced by persulfate activation. In the CFTS/PMS system, there is S→M σ bond ((M = Cu, Fe, and Sn) which is favorable for electron transfer and contribute to form ≡Fe (II)*. It has been reported that Sn(Ⅱ) can also activate persulfate to produce active free radicals due to the synergy between ≡Fe (II)* and Sn (Eqs. 38−40) (Kong, et al., 2019). The mechanism of multicomponent iron sulfide for persulfate activation can be proposed in Figures 7A−C.
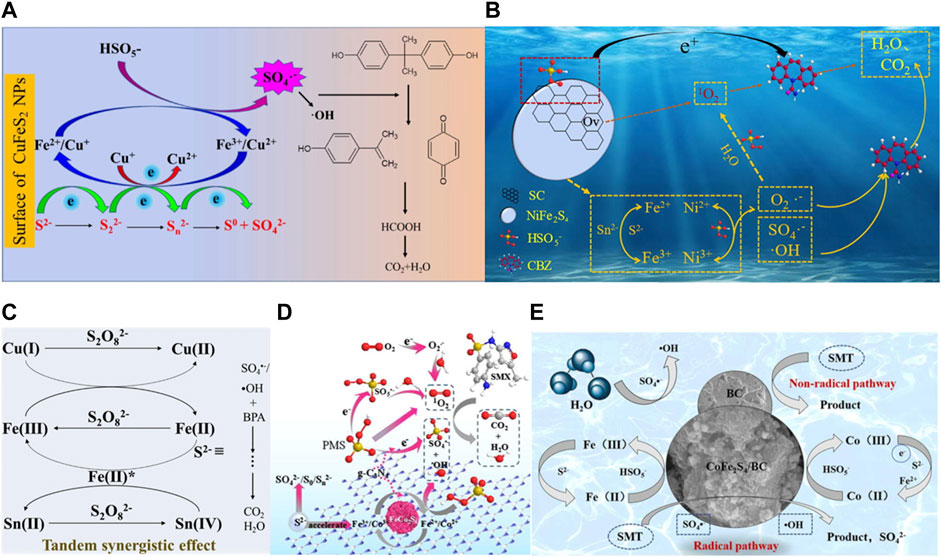
FIGURE 7. (A) Proposed catalytic mechanism for PMS activation and BPA degradation by CuFeS2. Adapted with permission from (Nie, et al., 2019). Copyright 2019 Elsevier. (B) The mechanism of SC/NiFe2S4 activating PMS to degrade CBZ, Adapted with permission from (Fan, et al., 2022). Copyright 2022 Elsevier. (C) The proposed oxidation mechanism in the Cu2FeSnS4/PMS process, Adapted with permission from (Kong, et al., 2019). Copyright 2019 Elsevier. (D) The mechanism of CoFe2S4/BC activating PMS to degrade SMT. Adapted with permission from. Copyright 2020 Elsevier. (E) Proposed mechanism for the catalytic SMT oxidation over the CoFe2S4/BC/PMS system. Adapted with permission from (Li, et al., 2022c). Copyright 2022 Elsevier.
Considering the poor dispersion of metal sulfides and large amount of metal ions leaching, the researchers further modified these catalysts. For example, Li. et al. (Li, et al., 2020) used FeCo2S4 modified g−C3N4 (FeCo2S4−CN) composite for PMS activation to degrade sulfamethoxazole (SMX). As shown in Figure 7D, in this system, there is a synergistic effect between FeCo2S4 and g−C3N4, thus the removal rate of SMX is higher than that of FeCo2S4/PMS, g−C3N4/PMS and PMS alone. Moreover, due to the synergistic effect between metal ions and g−C3N4, iron and cobalt ions, excessive leaching of metal ions is avoided. Li. et al. (Li, et al., 2022a) synthesized CoFe2S4/BC catalyst by a two−step hydrothermal method and combined it with PMS for the degradation of sodium sulfadimethacil (SMT). As shown in Figure 7E, in this system, in addition to the free radical pathway induced by the metal active site, there is also a non−free radical pathway. On the one hand, electrons can be transferred directly from the contaminants to the PMS through the active center of BC, leading to the direct decomposition of the pollutant. On the other hand, BC can activate the O−O bond in the PMS and directly oxidize the target compound.
4 Hybrid activation systems
4.1 Photoassisted systems
FeS and its derivatives are considered promising candidates for photocatalytic water treatment due to their ability to absorb visible and/or ultraviolet light, narrow optical band gap, and charge transport properties (Ayodhya and Veerabhadram, 2018; Li, et al., 2021a). Bibhutibhushan et al. (Show, et al., 2017) synthesized FeS nanospheres by a simple electrochemical route, which act as photocatalysts to successfully degrade alizarin red S (ARS), methylene blue (MB), rose red (RB) and phenol, and no degradation of these dyes was observed in the dark and very slow degradation was observed in the absence of FeS but the presence of light, which indicates that FeS has a strong synergistic effect when working together with photoassisted technologies.
During the reaction process, when FeS are exposed to visible light, illumination causes the excitation of valence band electrons in the conduction band, and this charge separation leads to the formation of electron−hole pairs (Suroshe, et al., 2018). Photogenerated electron (e−)−hole pairs (h+) can react with adsorbed surface species such as O2, H2O, and OH− to form ROSs such as O2− and •OH, for pollutants degradation (Eqs. 41−45) (Nair, et al., 2011; Chabri, et al., 2016). In addition, the Fe2+ and S2− in FeS can react as persulfate activator for pollutants degradation. Additionally, visible light will accelerate the cyclic conversion between Fe3+ and Fe2+ (Eq. 46) (Chen, et al., 2021), further improving the continuous degradation. Hence, possible light−induced reactions can be proposed as Eqs. 1−3 and Eqs. 41–46:
4.2 Electro−assisted systems
Electrochemical advanced oxidation processes (EAOPs) have attracted increasing attention due to their environmental compatibility, ease of scaling up and high efficiency in degrading refractory contaminants compared to conventional advanced oxidation processes (Luo, et al., 2020). Iron sulfide shows good electrocatalytic performance due to its excellent electrical conductivity, hybrid d orbital, and general redox properties (Li, et al., 2021b), which has great potential in the field of wastewater treatment when combined with EAOPs.
Ammar. et al. (Ammar, et al., 2015) used pyrite as a heterogeneous source of Fe2+ catalyst to degrade tyrosol (TY) in an electro−assisted process, which possesses superior performance due to the self−regulation of Fe2+ content in the medium. As shown in Figure 8A, (Labiadh, et al., 2015),used pyrite/EF system to generate H2O2 in situ and regenerate Fe2+ to completely remove azo dye (4−amino−3−hydroxy−2−p−toluene −naphthalene−1−sulfonic acid) (AHPS) from water, the mineralization rate of pyrite/EF system was superior to that of EF system alone under the same conditions, specifically, more than 90% TOC was removed in pyrite/EF within 300 min, whereas in the same reaction time only 70% TOC was removed with the conventional EF process. This is due to the self−regulation effect of pyrite on pH and soluble Fe2+ without additional acidification. As shown in Figure 8C, Ye et al. (Ye, et al., 2020) used FeS2/C nanocomposites as highly active, stable and recyclable catalysts to treat fluoxetine in polyphase EF system, which achieved an impressive 90% TOC removal, while conventional EF processes produce a maximum TOC removal of 60%. The good performance of pyrite/EF system was attributed to the large amount of •OH produced by the pyrite induced Fenton reaction, which mainly attributed to the following aspects: (1) The mass transfer restriction of FeS2 is very small, and it can act as the transfer intermediate of Fe2+ to participate in the homogeneous reaction to produce •OH. Meanwhile, pyrite can provide rich active sites, and ≡Fe (II) can activate H2O2 to produce •OH by Fe−S bond. (2) the ≡Fe (II) on the surface of FeS2 can promote the activation of molecule O2, thus accelerating the formation of O2•− (Liu, et al., 2015). In addition, the boron−doped diamond (BDD) anode used in the electro−assisted system also contributes to the generation of physical adsorption •OH in pyrite/EF system. The heterogeneous mechanism of the system is dominant because the concentration of dissolved iron is relatively low. The mechanism of electro−assisted pyrite/persulfate system for contaminants degradation can be proposed in Figures 8B, D.
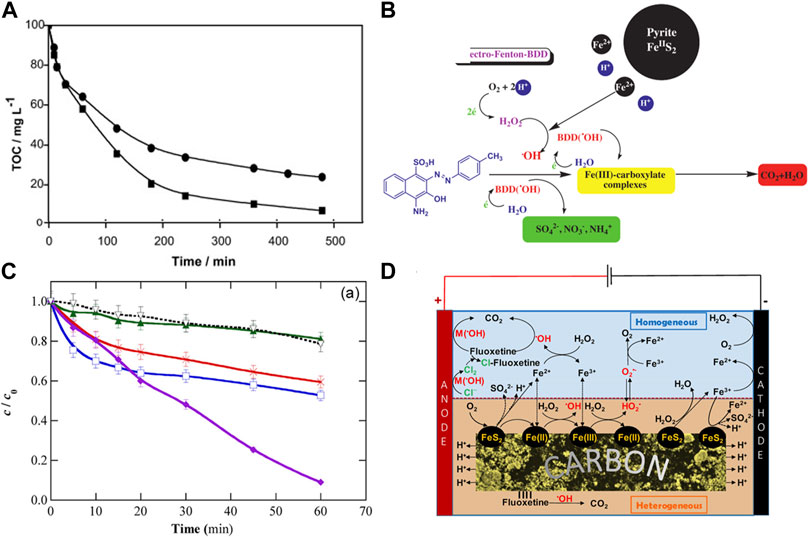
FIGURE 8. (A) Comparison between pyrite/EF system and classical EF system for evolution of TOC removal, and (B) The mechanism of FeS2/EF system for AHPS degradation. Adapted with permission from (Labiadh, et al., 2015). Copyright 2015 Elsevier. (C) Normalized concentration decay of fluoxetine during the EF process (solutions containing 0.049 mM drug), and (D) the mechanism of FeS2/C−catalyzed heterogeneous EF treatment at mild pH. Adapted with permission from (Ye, et al., 2020). Copyright 2020 American Chemical Society.
4.3 Ultrasonic−assisted technology
Increasing attention has been paid to the application of ultrasonic (US) combined with advanced oxidation technology in water treatment. On the one hand, US has strong mechanical effect, which can enhance the mass transfer between interfaces, remove the passivation film on the metal surface and make the surface regenerate continuously. These properties can overcome the heterogeneous mass transfer barrier in the process of degrading organic matter, and achieve good contaminants removal effect (Xiang, et al., 2022). On the other hand, US lead to form cavitation effect, and its local high temperature and high pressure can produce •OH, O2•− and other ROSs (Chi, et al., 2022; Savun−Hekimoğlu, 2020; Wei, et al., 2017).
Chi et al. (Chi, et al., 2022) used US to enhance ferrous sulfide (FeS) to activate persulfate (PDS) for 2’−deoxycoformycin (DCF) degradation. US/FeS/PDS system with excellent activity presented an optimal DCF degradation efficiency (98.9%), which was 56.7, 5.81, 1.48 times than that of US, US/PDS and FeS/PDS systems (Figure 9B). Wei. et al. (Xiang, et al., 2022) demonstrated good degradation effect of carbamazepine (CBZ) by using ultrasonic−enhanced FeS/PDS system, which can remove 94.2% CBZ in 60 min, while FeS/PDS system can only remove 71.2% CBZ under same conditions (Figure 9A), confirming the synergistic enhancement effect of US and FeS contributed to the degradation of contaminants.
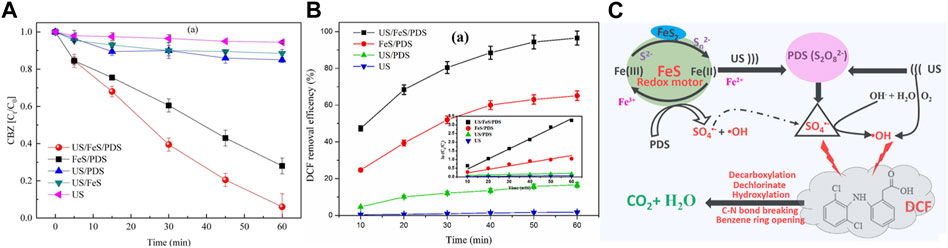
FIGURE 9. (A) Comparison of CBZ degradation during five systems. Adapted with permission from (Xiang, et al., 2022). Copyright 2022 Elsevier. (B)The DCF removal efficiency and kinetic constant, and (C) The reaction mechanism of DCF degradation in the US/FeS/PDS system. Adapted with permission from (Chi, et al., 2022). Copyright 2022 Elsevier.
In ultrasonic enhanced FeS/persulfate system, US can promote the interfacial sulfur−iron electron transfer and the disintegration of passivation layer, which avoid the passivation and deactivation of FeS, and provide redox energy between S2−/Sx2− and Fe2+/Fe3+ to promote continuous production of Fe2+. Additionally, US can directly activate PDS, H2O and dissolved oxygen to form SO4•− and •OH. In a word, US greatly promotes both heterogeneous and homogeneous iron cycles in the system. Hence, possible US−assisted reactions can be summarized as Eqs.1−3, Eqs. 47, 48, and the mechanism of US−assisted FeS/persulfate system for contaminants degradation can be proposed in Figure 9C
4.4 Microwave assisted technology
Microwave (MW) activation of persulfate has been widely studied. It has been proved to be superior to conventional thermal activation in terms of accelerating reaction rate, increasing yield and selectively activating or inhibiting reaction pathways, thus leading to higher degradation rate and significant savings in energy consumption and treatment time (Qi, et al., 2014). Wang et al. (Wang, et al., 2020a) used microwave radiation combined with FeS to activate persulfate to treat dinitrodiazophenol in explosive production wastewater. The Chemical Oxygen Demand (COD) removal efficiency of the MW–FeS/PS process reached 76.16%, which was 2.62, 2.57, and 1.42 times than that of MW/FeS, FeS/PS and MW/PS systems, indicating the strong synergistic effect between FeS and MW.
In MW–FeS/PS system, in addition to the persulfate activation performance of FeS, MW radiation can not only activate PS to produce SO4•− by its thermal effect, but make some functional groups and structures on organic pollutants vulnerable. Furthermore, the MW and FeS have strong synergic effect. Therefore, microwave−assisted FeS activation of persulfate can show higher treatment efficiency and higher PS utilization efficiency (Wang, et al., 2020b).
5 Reusability and stability
The reusability and stability of FeS−based catalysts are very important for their practical application. In general, FeS−based catalysts can maintain satisfactory catalytic performance in multiple continuous cycles due to the internal Fe (Ⅲ)/Fe (Ⅱ) cycle, which is shown in Eqs 49, 50:
As shown in Figure 10, the Cu2FeSnS4/PS system degraded more than 80% of BPA within 45 min after three reuse cycles (Yangju et al., 2019). In S−Fe@C/PDS system, no evident decline on Rh B degradation suggested the catalyst could be at least reused for five times (Yu, et al., 2022). In FeS2/C−EF system, a slight but progressive performance decrease was observed after five cycles, with only 61% fluoxetine removal achieved at 60 min (Ye, et al., 2020). Fortunately, the activity of FeS−based catalysts can be restored by various treatments, such as washing with organic solvents (Ye, et al., 2020), ultrasonic treatment (Zhao, et al., 2020), and pickling (Peng, et al., 2020).
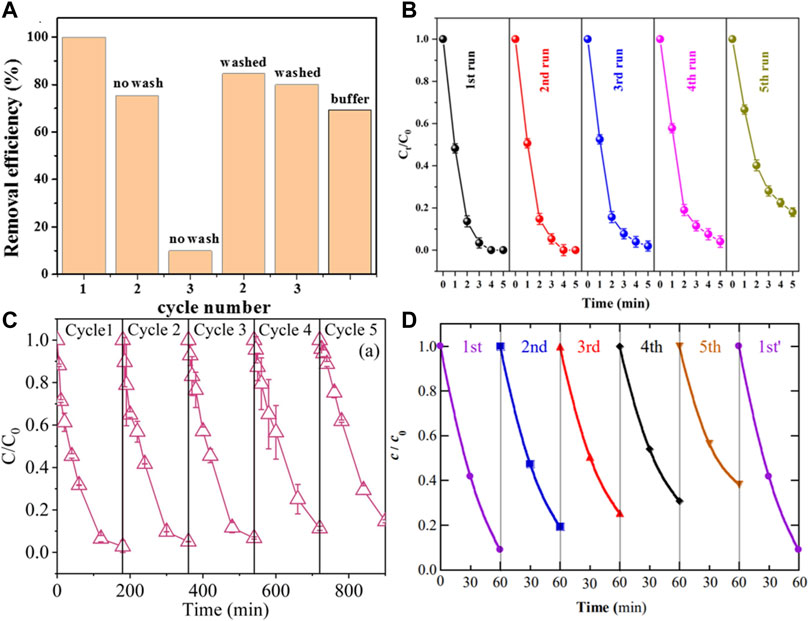
FIGURE 10. (A) Degradation of BPA by the PS/CFTS system during different activation cycles and phosphate buffer. Adapted with permission from (Kong, et al., 2019). Copyright 2019 Elsevier. (B) Evaluation of the stability and recyclability of S−Fe@C. Adapted with permission from (Yu, et al., 2022). Copyright 2022 Elsevier. (C) Catalytic activity of FeS for repeated use. Adapted with permission from (Xu and Sheng, 2021). Copyright 2021 Elsevier. (D) The fluoxetine concentration during the heterogeneous EF treatment with FeS2/C nano catalyst, Cycle first′ was made once the catalyst regeneration was performed after the fifth cycle. Adapted with permission from (Ye, et al., 2020). Copyright 2020 Elsevier.
6 Reactive oxidation species
In the PS based Fenton−like reactions activated by FeS−based materials, organics were normally degraded through two oxidation pathways involving free radical (e.g., •OH, SO4•− and O2•−) and non−radical (e.g., 1O2 and Fe (IV) = O) reactive oxidation species (Li, et al., 2021b).
In general, SO4•− and •OH are most frequently detected radicals during PS activation. Fe (II) in the FeS−based catalysts reacts with PS and split O−O bond to form SO4•− (Eqs 1, 2). Fan et al. (Fan, et al., 2018a) proposed that surface−bound radicals (e.g., •OHads and SO4•−ads) produced by the combination of surface Fe (II) and PS can diffuse from the catalyst surface and convert into free radicals (e.g., •OHfree and SO4•−free) to degrade contaminants. In PS activation process, •OH may be generated by two main pathways, one is produced by the reaction of SO4•− with H2O (Eq. 25), and the other is formed by radical conversion reaction of SO4•− with OH− under neutral or alkaline conditions (Eq. 26) (Zhao, et al., 2016). It was found that the main ROSs in the activation process of PS is severely pH−dependent, with SO4•− under acidic and •OH at alkaline conditions, respectively (Feng, et al., 2015). Usually, •OH always transform into O2•− under alkaline conditions through a complex series of radical chain steps (Li, et al., 2016; Ma, et al., 2019). Additionally, some FeS−based materials can also react directly with PS to produce O2•− (Jin, et al., 2022).
Non−radical reactive oxidation species such as 1O2 and Fe (IV) = O can also be produced in the PS activation process by FeS−based materials. 1O2 can be formed by the self−decomposition of PMS at alkaline condition (Eq. 53) (Zhou, et al., 2021a). Carbon modified FeS materials, such as FeS@GO and FeS@BC, can also form 1O2 in PS activation, in which carbonaceous materials play a key role in the generation of 1O2 (Wang, et al., 2023b). Furthermore, 1O2 can result from the conversion of O2•− and H2O (Eqs. 54, 55) (Zhou, et al., 2021b; Cai, et al., 2022b; Li, et al., 2022b). High−valent iron−oxo species (e.g., ≡Fe (Ⅳ) = O and ≡FeV = O) is a burgeoning ROS produced in PS activation (Li, et al., 2021a). In the activation of PS by FeS−based catalysts, the aqueous Fe (II) released from the FeS surface reacts with the PS and contribute to the formation of Fe (IV) (Eqs. 56, 57), which then effectively degrades the contaminants through non− radical pathways (Xu and Sheng, 2021).
7 Toxicity assessment
To ensure safe environmental applications of FeS−based catalysts, it is necessary to conduct toxicity assessments of the catalysts and their degradation intermediates to understand the potential environmental risks to ecosystems and human health. Bare Iron sulfide (FeS) nanoparticles were reported to bind with DNA, limiting the ability of DNA to interact with other nucleic acids and amino acids (Hatton and Rickard, 2008). Rickard, D. et al. (Rickard, et al., 2011) proposed that when the concentration of FeS nanoparticles is lower than its solubility limit, it will cause incision in DNA molecules, and pose genotoxicity by reacting with polynucleic acids when above solubility limit. Furthermore, FeS particles will inhibit the growth of microorganisms and plants. For instance, in the presence of FeS nanoparticles of 2 × 10−5 M to 5 × 10−3 M, the growth rate of E. coli is reduced under anaerobic conditions. FeS particles may impede nutrients uptake and will decrease seed yield and viability when deposited on the roots of wild rice plants (Pastor, et al., 2017). Zheng et al. (Zheng, et al., 2018) illustrated that exposure to CMC−FeS nanoparticles significantly damaged DNA and proteins due to nanoparticle induced oxidative stress. At present, although the biotoxicity of FeS−based catalysts in advanced oxidation processes have been gradually carried out, the evaluation of catalyst toxicity to water environment and the targeted regulation of highly toxic intermediates to harmless transformation are still insufficient.
8 Conclusion and future research expected
In the past few decades, increasing researchers have focused on the application of iron sulfides and related modified materials on water pollution. This paper reviews the synthesis of iron sulfides materials and the application of them in PS−AOPs for organic contaminant removal, and the related mechanisms were also reviewed. Although iron sulfide materials have been widely used in water pollutant restoration, there are still some knowledge gaps and challenges in its application as follows.
1) Although the modification of FeS greatly improves the activity and stability for PS activation, FeS is still easy to be oxidized in the presence of oxygen. Therefore, the long−term stability and oxidation resistance of FeS materials should be further studied to avoid the loss of reactivity of FeS, which will seriously limit its practical application in water restoration.
2) There is limited information of catalyst toxicity to water environment and the targeted regulation of highly toxic intermediates to harmless transformation, therefore, fully study the potential environmental toxicity of iron sulfide materials is essential.
3) It is necessary to combine iron sulfide with other auxiliary technologies to activate persulfate for contaminants degradation. Only a few literatures have reported the application of iron sulfide materials/PS with photo irradiation, ultrasonic, electrocatalysis, and microwave for water remediation.
4) The influence of iron sulfides on the water should be taken into account to avoid changing the physical and chemical properties and functions of the water due to the addition of iron sulfide materials.
5) A detailed and comprehensive study is needed on the whereabouts of iron sulfide materials after injection into water, especially large−scale water treatment, to ensure that there will be no secondary pollution in the process of water restoration.
Author contributions
YS: Conceptualization, Methodology, Investigation, Writing—original draft. XF: Writing—review and editing. YL: Writing—review and editing. JL: Writing—review and editing. WP: Resources, Conceptualization, Writing—review and editing, Supervision, Data curation. All authors contributed to the article and approved the submitted version.
Funding
This research was supported by the project of No. U20A20153 from the National Natural Science Foundation of China and No. 20YFZCSN00610 from the Tianjin Science and Technology Support Plan Key Projects.
Conflict of interest
The authors declare that the research was conducted in the absence of any commercial or financial relationships that could be construed as a potential conflict of interest.
Publisher’s note
All claims expressed in this article are solely those of the authors and do not necessarily represent those of their affiliated organizations, or those of the publisher, the editors and the reviewers. Any product that may be evaluated in this article, or claim that may be made by its manufacturer, is not guaranteed or endorsed by the publisher.
References
Ajay, C., Ramesh, K. S., and Charter, D. S. A., Dady, B. D. (1996). Iron sulfide catalysts for coal liquefaction prepared using a micellar technique. Ind. Eng. Chem. Res., 35, 2916, 2919. doi:10.1021/ie950694y9)
Ammar, S., Oturan, M. A., Labiadh, L., Guersalli, A., Abdelhedi, R., Oturan, N., et al. (2015). Degradation of tyrosol by a novel electro−Fenton process using pyrite as heterogeneous source of iron catalyst. Water Res. 74, 77–87. doi:10.1016/j.watres.2015.02.006
Anipsitakis, G. P., and Dionysiou, D. D. (2004). Radical generation by the interaction of transition metals with common oxidants. Environ. Sci. Technol. 38 (13), 3705–3712. doi:10.1021/es035121o
Ayodhya, D., and Veerabhadram, G. (2018). A review on recent advances in photodegradation of dyes using doped and heterojunction based semiconductor metal sulfide nanostructures for environmental protection. Mater Today Energy 9, 83–113. doi:10.1016/j.mtener.2018.05.007
Bhatt, P., Gangola, S., Bhandari, G., Zhang, W., Maithani, D., Mishra, S., et al. (2021). New insights into the degradation of synthetic pollutants in contaminated environments. Chemosphere 268, 128827. doi:10.1016/j.chemosphere.2020.128827
Bin, Q., Lin, B., Zhu, K., Shen, Y., Man, Y., Wang, B., et al. (2020). Superior trichloroethylene removal from water by sulfide−modified nanoscale zero−valent iron/graphene aerogel composite. J. Environ. Sci. 88, 90–102. doi:10.1016/j.jes.2019.08.011
Cai, H., Zou, J., Lin, J., Li, J., Huang, Y., Zhang, S., et al. (2022a). Sodium hydroxide−enhanced acetaminophen elimination in heat/peroxymonosulfate system: Production of singlet oxygen and hydroxyl radical. Chem. Eng. J. 429 (2), 132438. doi:10.1016/j.cej.2021.132438
Cai, J., and Zhang, Y. (2022). Enhanced degradation of bisphenol S by persulfate activated with sulfide−modified nanoscale zero−valent iron. Environ. Sci. Pollut. R. 29 (6), 8281–8293. doi:10.1007/s11356-021-16156-8
Cai, Y., Fan, J., and Liu, Z. (2022b). Enhanced degradation of tetracycline over FeS−based Fenton−like process: Autocatalytic decomposition of H2O2 and reduction of Fe(III). J. Hazard Mater 436, 129092. doi:10.1016/j.jhazmat.2022.129092
Chabri, S., Dhara, A., Show, B., Adak, D., Sinha, A., and Mukherjee, N. (2016). Mesoporous CuO–ZnO p–n heterojunction based nanocomposites with high specific surface area for enhanced photocatalysis and electrochemical sensing. Catal. Sci. Technol. 6 (9), 3238–3252. doi:10.1039/c5cy01573a
Chen, G., Yu, Y., Liang, L., Duan, X., Li, R., Lu, X., et al. (2021). Remediation of antibiotic wastewater by coupled photocatalytic and persulfate oxidation system: A critical review. J. Hazard Mater 408, 124461. doi:10.1016/j.jhazmat.2020.124461
Chen, H., Zhang, Z., Feng, M., Liu, W., Wang, W., Yang, Q., et al. (2017). Degradation of 2,4−dichlorophenoxyacetic acid in water by persulfate activated with FeS (mackinawite). Chem. Eng. J. 313, 498–507. doi:10.1016/j.cej.2016.12.075
Chen, Y., Liang, W., Li, Y., Wu, Y., Chen, Y., Xiao, W., et al. (2019). Modification, application and reaction mechanisms of nano−sized iron sulfide particles for pollutant removal from soil and water: A review. Chem. Eng. J. 362, 144–159. doi:10.1016/j.cej.2018.12.175
Chi, N., Liu, J., Feng, L., Guo, Z., Chen, Y., Pan, T., et al. (2022). FeS redox power motor for PDS continuous generation of active radicals on efficient degradation and removal of diclofenac: Role of ultrasonic. Chemosphere 300, 134574. doi:10.1016/j.chemosphere.2022.134574
Dong, H., Hou, K., Qiao, W., Cheng, Y., Zhang, L., Wang, B., et al. (2019a). Insights into enhanced removal of TCE utilizing sulfide−modified nanoscale zero−valent iron activated persulfate. Chem. Eng. J. 359, 1046–1055. doi:10.1016/j.cej.2018.11.080
Dong, H., Wang, B., Li, L., Wang, Y., Ning, Q., Tian, R., et al. (2019b). Activation of persulfate and hydrogen peroxide by using sulfide−modified nanoscale zero−valent iron for oxidative degradation of sulfamethazine: A comparative study. Sep. Purif. Technol. 218, 113–119. doi:10.1016/j.seppur.2019.02.052
El Asmar, R., Baalbaki, A., Abou Khalil, Z., Naim, S., Bejjani, A., and Ghauch, A. (2021). Iron−based metal organic framework MIL−88−A for the degradation of naproxen in water through persulfate activation. Chem. Eng. J. 405, 126701. doi:10.1016/j.cej.2020.126701
Fan, D., Lan, Y., Tratnyek, P. G., Johnson, R. L., Filip, J., O Carroll, D. M., et al. (2017). Sulfidation of Iron−Based materials: A review of processes and implications for water treatment and remediation. Environ. Sci. Technol. 51 (22), 13070–13085. doi:10.1021/acs.est.7b04177
Fan, D., O'Brien, J. G., Tratnyek, P. G., and Johnson, R. L. (2016). Sulfidation of nano zerovalent iron (nZVI) for improved selectivity during In−Situ chemical reduction (ISCR). Environ. Sci. Technol. 50 (17), 9558–9565. doi:10.1021/acs.est.6b02170
Fan, J., Gu, L., Wu, D., and Liu, Z. (2018a). Mackinawite (FeS) activation of persulfate for the degradation of p−chloroaniline: Surface reaction mechanism and sulfur−mediated cycling of iron species. Chem. Eng. J. 333, 657–664. doi:10.1016/j.cej.2017.09.175
Fan, J., Zhao, Z., Ding, Z., and Liu, J. (2018b). Synthesis of different crystallographic FeOOH catalysts for peroxymonosulfate activation towards organic matter degradation. Rsc Adv. 8 (13), 7269–7279. doi:10.1039/c7ra12615h
Fan, S., Shang, J., Kulan, S., He, X., Wang, X., Nasen, B., et al. (2022). Enhanced degradation of carbamazepine in water over SC−modified NiFe2S4 nanocomposites by peroxymonosulfate activation. Chem. Eng. J. 450, 138190. doi:10.1016/j.cej.2022.138190
Feng, M., Qu, R., Zhang, X., Sun, P., Sui, Y., Wang, L., et al. (2015). Degradation of flumequine in aqueous solution by persulfate activated with common methods and polyhydroquinone−coated magnetite/multi−walled carbon nanotubes catalysts. Water Res. 85, 1–10. doi:10.1016/j.watres.2015.08.011
Fu, X., Zhang, J., Zhao, H., Zhang, S., Nie, T., Zhang, Y., et al. (2020). Enhanced peroxymonosulfate activation by coupling zeolite−supported nano−zero−valent iron with weak magnetic field. Sep. Purif. Technol. 230, 115886. doi:10.1016/j.seppur.2019.115886
Gong, Y., Liu, Y., Xiong, Z., Kaback, D., and Zhao, D. (2012). Immobilization of mercury in field soil and sediment using carboxymethyl cellulose stabilized iron sulfide nanoparticles. Nanotechnology 23 (29), 294007. doi:10.1088/0957-4484/23/29/294007
Gong, Y., Liu, Y., Xiong, Z., and Zhao, D. (2014). Immobilization of mercury by carboxymethyl cellulose stabilized iron sulfide nanoparticles: Reaction mechanisms and effects of stabilizer and water chemistry. Environ. Sci. Technol. 48 (7), 3986–3994. doi:10.1021/es404418a
Gong, Y., Tang, J., and Zhao, D. (2016). Application of iron sulfide particles for groundwater and soil remediation: A review. Water Res. 89, 309–320. doi:10.1016/j.watres.2015.11.063
Gu, X., Lu, S., Guo, X., Sima, J., Qiu, Z., and Sui, Q. (2015). Oxidation and reduction performance of 1,1,1−trichloroethane in aqueous solution by means of a combination of persulfate and zero−valent iron. Rsc Adv. 5 (75), 60849–60856. doi:10.1039/c5ra07655b
Gu, Y., Wang, B., He, F., Bradley, M. J., and Tratnyek, P. G. (2017). Mechanochemically sulfidated microscale zero valent iron: Pathways, kinetics, mechanism, and efficiency of trichloroethylene dechlorination. Environ. Sci. Technol. 51 (21), 12653–12662. doi:10.1021/acs.est.7b03604
Guo, W., Zhao, Q., Du, J., Wang, H., Li, X., and Ren, N. (2020). Enhanced removal of sulfadiazine by sulfidated ZVI activated persulfate process: Performance, mechanisms and degradation pathways. Chem. Eng. J. 388, 124303. doi:10.1016/j.cej.2020.124303
Han, M., Liang, L., Wang, Y., Deng, Y., Zhang, Q., Ye, Y., et al. (2022). Peroxymonosulfate activation by sponge−based FeS material for efficient degradation of tetracycline: The critical role of sponge. J. Water Process Eng. 46, 102605. doi:10.1016/j.jwpe.2022.102605
Han, Y., and Yan, W. (2016). Reductive dechlorination of trichloroethene by Zero−valent iron nanoparticles: Reactivity enhancement through sulfidation treatment. Environ. Sci. Technol. 50 (23), 12992–13001. doi:10.1021/acs.est.6b03997
Hao, F., Guo, W., Wang, A., Leng, Y., and Li, H. (2014). Intensification of sonochemical degradation of ammonium perfluorooctanoate by persulfate oxidant. Ultrason. Sonochem 21 (2), 554–558. doi:10.1016/j.ultsonch.2013.09.016
Hatton, B., and Rickard, D. (2008). Nucleic acids bind to nanoparticulate iron (II) monosulphide in aqueous solutions. Orig. Life Evol. Biosphere 38 (3), 257–270. doi:10.1007/s11084-008-9132-7
He, J., Tang, J., Zhang, Z., Wang, L., Liu, Q., and Liu, X. (2021). Magnetic ball−milled FeS@biochar as persulfate activator for degradation of tetracycline. Chem. Eng. J. 404, 126997. doi:10.1016/j.cej.2020.126997
He, J., Xiao, Y., Tang, J., Chen, H., and Sun, H. (2019). Persulfate activation with sawdust biochar in aqueous solution by enhanced electron donor−transfer effect. Sci. Total Environ. 690, 768–777. doi:10.1016/j.scitotenv.2019.07.043
He, M., Li, W., Xie, Z., Yang, S., He, C., Xiong, Z., et al. (2022). Peracetic acid activation by mechanochemically sulfidated zero valent iron for micropollutants degradation: Enhancement mechanism and strategy for extending applicability. Water Res. 222, 118887. doi:10.1016/j.watres.2022.118887
Hong, Q., Liu, C., Wang, Z., Li, R., Liang, X., Wang, Y., et al. (2021). Electron transfer enhancing Fe(II)/Fe(III) cycle by sulfur and biochar in magnetic FeS@biochar to active peroxymonosulfate for 2,4−dichlorophenoxyacetic acid degradation. Chem. Eng. J. 417, 129238. doi:10.1016/j.cej.2021.129238
Hou, K., Pi, Z., Chen, F., He, L., Yao, F., Chen, S., et al. (2022). Peroxymonosulfate (PMS) activation by mackinawite for the degradation of organic pollutants: Underappreciated role of dissolved sulfur derivatives. Sci. Total Environ. 811, 151421. doi:10.1016/j.scitotenv.2021.151421
Hou, K., Pi, Z., Yao, F., Wu, B., He, L., Li, X., et al. (2021). A critical review on the mechanisms of persulfate activation by iron−based materials: Clarifying some ambiguity and controversies. Chem. Eng. J. 407, 127078. doi:10.1016/j.cej.2020.127078
Hou, X., Huang, X., Ai, Z., Zhao, J., and Zhang, L. (2016). Ascorbic acid/Fe@Fe2O3: A highly efficient combined fenton reagent to remove organic contaminants. J. Hazard Mater 310, 170–178. doi:10.1016/j.jhazmat.2016.01.020
Huang, D., He, J., Gu, Y., and He, F. (2017). Mechanochemically sulfidated zero valent iron as an efficient Fenton−like catalyst for degradation of organic contaminants. Acta Chim. Sin. 75 (9), 866. doi:10.6023/a17020060
Hussain, I., Li, M., Zhang, Y., Li, Y., Huang, S., Du, X., et al. (2017). Insights into the mechanism of persulfate activation with nZVI/BC nanocomposite for the degradation of nonylphenol. Chem. Eng. J. 311, 163–172. doi:10.1016/j.cej.2016.11.085
Jin, Z., Li, Y., Dong, H., Xiao, S., Xiao, J., Chu, D., et al. (2022). A comparative study on the activation of persulfate by mackinawite@biochar and pyrite@biochar for sulfamethazine degradation: The role of different natural iron−sulfur minerals doping. Chem. Eng. J. 448, 137620. doi:10.1016/j.cej.2022.137620
Kim, E., Kim, J., Azad, A., and Chang, Y. (2011). Facile synthesis and characterization of Fe/FeS nanoparticles for environmental applications. Acs Appl. Mater Inter 3 (5), 1457–1462. doi:10.1021/am200016v
Kong, L., Fang, G., Chen, Y., Xie, M., Zhu, F., Ma, L., et al. (2019). Efficient activation of persulfate decomposition by Cu2FeSnS4 nanomaterial for bisphenol A degradation: Kinetics, performance and mechanism studies. Appl. Catal. B Environ. 253, 278–285. doi:10.1016/j.apcatb.2019.04.069
Labiadh, L., Oturan, M. A., Panizza, M., Hamadi, N. B., and Ammar, S. (2015). Complete removal of AHPS synthetic dye from water using new electro−fenton oxidation catalyzed by natural pyrite as heterogeneous catalyst. J. Hazard Mater 297, 34–41. doi:10.1016/j.jhazmat.2015.04.062
Li, H., Wan, J., Ma, Y., and Wang, Y. (2016). Reaction pathway and oxidation mechanisms of dibutyl phthalate by persulfate activated with zero−valent iron. Sci. Total Environ. 562, 889–897. doi:10.1016/j.scitotenv.2016.04.093
Li, J., Liu, Q., Gou, G., Kang, S., Tan, X., Tan, B., et al. (2022c). New insight into the mechanism of peroxymonosulfate activation by Fe3S4: Radical and non−radical oxidation. Sep. Purif. Technol. 286, 120471. doi:10.1016/j.seppur.2022.120471
Li, J., Ren, Y., Ji, F., and Lai, B. (2017a). Heterogeneous catalytic oxidation for the degradation of p−nitrophenol in aqueous solution by persulfate activated with CuFe2O4 magnetic nano−particles. Chem. Eng. J. 324, 63–73. doi:10.1016/j.cej.2017.04.104
Li, J., Wan, Y., Li, Y., Yao, G., and Lai, B. (2019a). Surface Fe(III)/Fe(II) cycle promoted the degradation of atrazine by peroxymonosulfate activation in the presence of hydroxylamine. Appl. Catal. B Environ. 256, 117782. doi:10.1016/j.apcatb.2019.117782
Li, J., Yang, L., Lai, B., Liu, C., He, Y., Yao, G., et al. (2021a). Recent progress on heterogeneous Fe−based materials induced persulfate activation for organics removal. Chem. Eng. J. 414, 128674. doi:10.1016/j.cej.2021.128674
Li, J., Zhang, X., Sun, Y., Liang, L., Pan, B., Zhang, W., et al. (2017b). Advances in sulfidation of zerovalent iron for water decontamination. Environ. Sci. Technol. 51 (23), 13533–13544. doi:10.1021/acs.est.7b02695
Li, J., Zou, J., Zhang, S., Cai, H., Huang, Y., Lin, J., et al. (2022b). Sodium tetraborate simultaneously enhances the degradation of acetaminophen and reduces the formation potential of chlorinated by−products with heat−activated peroxymonosulfate oxidation. Water Res. 224, 119095. doi:10.1016/j.watres.2022.119095
Li, X., Liu, X., Lin, C., Zhang, H., Zhou, Z., Fan, G., et al. (2019b). Activation of peroxymonosulfate by magnetic catalysts derived from drinking water treatment residuals for the degradation of atrazine. J. Hazard Mater 366, 402–412. doi:10.1016/j.jhazmat.2018.12.016
Li, X., Zhou, M., and Pan, Y. (2018). Enhanced degradation of 2,4−dichlorophenoxyacetic acid by pre−magnetization Fe−C activated persulfate: Influential factors, mechanism and degradation pathway. J. Hazard Mater 353, 454–465. doi:10.1016/j.jhazmat.2018.04.035
Li, Y., Dong, H., Li, L., Tang, L., Tian, R., Li, R., et al. (2021b). Recent advances in waste water treatment through transition metal sulfides−based advanced oxidation processes. Water Res. 192, 116850. doi:10.1016/j.watres.2021.116850
Li, Y., Li, J., Pan, Y., Xiong, Z., Yao, G., Xie, R., et al. (2020). Peroxymonosulfate activation on FeCo2S4 modified g−C3N4 (FeCo2S4−CN): Mechanism of singlet oxygen evolution for nonradical efficient degradation of sulfamethoxazole. Chem. Eng. J. 384, 123361. doi:10.1016/j.cej.2019.123361
Li, Y., Zhao, X., Yan, Y., Yan, J., Pan, Y., Zhang, Y., et al. (2019c). Enhanced sulfamethoxazole degradation by peroxymonosulfate activation with sulfide−modified microscale zero−valent iron (S−mFe0): Performance, mechanisms, and the role of sulfur species. Chem. Eng. J. 376, 121302. doi:10.1016/j.cej.2019.03.178
Li, Y., Zhou, Y., Ni, R., Shang, J., and Cheng, X. (2022a). Degradation of sulfamethazine sodium salt by peroxymonosulfate activated by biochar supported CoFe2S4: Performance, mechanism and response surface method optimization. J. Environ. Chem. Eng. 10 (5), 108059. doi:10.1016/j.jece.2022.108059
Liang, C., Guo, Y., Chien, Y., and Wu, Y. (2010). Oxidative degradation of MTBE by Pyrite−Activated persulfate: Proposed reaction pathways. Ind. Eng. Chem. Res. 49 (18), 8858–8864. doi:10.1021/ie100740d
Lin, C., and Wu, M. (2014). UV/S2O82− process for degrading polyvinyl alcohol in aqueous solutions. Chem. Eng. Process. Process Intensif. 85, 209–215. doi:10.1016/j.cep.2014.08.012
Liu, H., Yao, J., Wang, L., Wang, X., Qu, R., and Wang, Z. (2019). Effective degradation of fenitrothion by zero−valent iron powder (Fe0) activated persulfate in aqueous solution: Kinetic study and product identification. Chem. Eng. J. 358, 1479–1488. doi:10.1016/j.cej.2018.10.153
Liu, J., Peng, C., and Shi, X. (2022a). Preparation, characterization, and applications of Fe−based catalysts in advanced oxidation processes for organics removal: A review. Environ. Pollut. 293, 118565. doi:10.1016/j.envpol.2021.118565
Liu, W., Ai, Z., Cao, M., and Zhang, L. (2014). Ferrous ions promoted aerobic simazine degradation with Fe@Fe2O3 core–shell nanowires. Appl. Catal. B Environ. 150−151, 1–11. doi:10.1016/j.apcatb.2013.11.034
Liu, W., Wang, Y., Ai, Z., and Zhang, L. (2015). Hydrothermal synthesis of FeS2 as a High−Efficiency fenton reagent to degrade alachlor via Superoxide−Mediated Fe(II)/Fe(III) cycle. Acs Appl. Mater Inter 7 (51), 28534–28544. doi:10.1021/acsami.5b09919
Liu, X., Li, H., Gao, S., Bai, Z., and Tian, J. (2022b). Peroxymonosulfate activation by different iron sulfides for bisphenol−A degradation: Performance and mechanism. Sep. Purif. Technol. 289, 120751. doi:10.1016/j.seppur.2022.120751
Luo, T., Feng, H., Tang, L., Lu, Y., Tang, W., Chen, S., et al. (2020). Efficient degradation of tetracycline by heterogeneous electro−Fenton process using Cu−doped Fe@Fe2O3: Mechanism and degradation pathway. Chem. Eng. J. 382, 122970. doi:10.1016/j.cej.2019.122970
Lyu, H., Gao, B., He, F., Zimmerman, A. R., Ding, C., Tang, J., et al. (2018a). Experimental and modeling investigations of ball−milled biochar for the removal of aqueous methylene blue. Chem. Eng. J. 335, 110–119. doi:10.1016/j.cej.2017.10.130
Lyu, H., Tang, J., Shen, B., and Siddique, T. (2018b). Development of a novel chem−bio hybrid process using biochar supported nanoscale iron sulfide composite and Corynebacterium variabile HRJ4 for enhanced trichloroethylene dechlorination. Water Res. 147, 132–141. doi:10.1016/j.watres.2018.09.038
Ma, J., Yang, M., Yu, F., and Chen, J. (2015). Easy solid−phase synthesis of pH−insensitive heterogeneous CNTs/FeS Fenton−like catalyst for the removal of antibiotics from aqueous solution. J. Colloid Interf. Sci. 444, 24–32. doi:10.1016/j.jcis.2014.12.027
Ma, W., Wang, N., Du, Y., Tong, T., Zhang, L., Andrew Lin, K., et al. (2019). One−step synthesis of novel Fe3C@nitrogen−doped carbon nanotubes/graphene nanosheets for catalytic degradation of Bisphenol A in the presence of peroxymonosulfate. Chem. Eng. J. 356, 1022–1031. doi:10.1016/j.cej.2018.09.093
Ma, Y., Gu, Y., Jiang, D., Mao, X., and Wang, D. (2021). Degradation of 2,4−DCP using persulfate and iron/E−carbon micro−electrolysis coupling system. J. Hazard Mater 413, 125381. doi:10.1016/j.jhazmat.2021.125381
Ma, Z., Yang, Y., Jiang, Y., Xi, B., Yang, T., Peng, X., et al. (2017). Enhanced degradation of 2,4−dinitrotoluene in groundwater by persulfate activated using iron–carbon micro−electrolysis. Chem. Eng. J. 311, 183–190. doi:10.1016/j.cej.2016.11.083
Mariëtte, W., Sjierk, J. V. D. G., and David, R. (2003). The structure of disordered mackinawite. Am. Mineral. 88 (11−12), 2007–2015. doi:10.2138/am-2003-11-1245
Meng, F., Song, M., Song, B., Wei, Y., Cao, Q., and Cao, Y. (2020). Enhanced degradation of Rhodamine B via α−Fe2O3 microspheres induced persulfate to generate reactive oxidizing species. Chemosphere 243, 125322. doi:10.1016/j.chemosphere.2019.125322
Mohamed, M. M., Abdelmonem, E. E., and El−Sayed, G. O. (2023). Graphene foam mediated FeS2/α−Fe2O3 composites for chloramphenicol photodegradation using persulfate activation under visible light irradiation. J. Water Process Eng. 53, 103633. doi:10.1016/j.jwpe.2023.103633
Nair, M. G., Nirmala, M., Rekha, K., and Anukaliani, A. (2011). Structural, optical, photo catalytic and antibacterial activity of ZnO and Co doped ZnO nanoparticles. Mater Lett. 65 (12), 1797–1800. doi:10.1016/j.matlet.2011.03.079
Nie, W., Mao, Q., Ding, Y., Hu, Y., and Tang, H. (2019). Highly efficient catalysis of chalcopyrite with surface bonded ferrous species for activation of peroxymonosulfate toward degradation of bisphenol A: A mechanism study. J. Hazard Mater 364, 59–68. doi:10.1016/j.jhazmat.2018.09.078
Oh, S., Kang, S., Kim, D., and Chiu, P. C. (2011). Degradation of 2,4−dinitrotoluene by persulfate activated with iron sulfides. Chem. Eng. J. 172 (2−3), 641–646. doi:10.1016/j.cej.2011.06.023
Paknikar, K. M., Nagpal, V., Pethkar, A. V., and Rajwade, J. M. (2005). Degradation of lindane from aqueous solutions using iron sulfide nanoparticles stabilized by biopolymers. Sci. Technol. Adv. Mat. 6 (3−4), 370–374. doi:10.1016/j.stam.2005.02.016
Pan, Y., Zhou, M., Zhang, Y., Cai, J., Li, B., and Sheng, X. (2018). Enhanced degradation of Rhodamine B by pre−magnetized Fe0/PS process: Parameters optimization, mechanism and interferences of ions. Sep. Purif. Technol. 203, 66–74. doi:10.1016/j.seppur.2018.03.039
Pastor, J., Dewey, B., Johnson, N. W., Swain, E. B., Monson, P., Peters, E. B., et al. (2017). Effects of sulfate and sulfide on the life cycle ofZizania palustris in hydroponic and mesocosm experiments. Ecol. Appl. 27 (1), 321–336. doi:10.1002/eap.1452
Peng, J., Zhou, H., Liu, W., Ao, Z., Ji, H., Liu, Y., et al. (2020). Insights into heterogeneous catalytic activation of peroxymonosulfate by natural chalcopyrite: pH−dependent radical generation, degradation pathway and mechanism. Chem. Eng. J. 397, 125387. doi:10.1016/j.cej.2020.125387
Petrie, B., Barden, R., and Kasprzyk−Hordern, B. (2015). A review on emerging contaminants in wastewaters and the environment: Current knowledge, understudied areas and recommendations for future monitoring. Water Res. 72, 3–27. doi:10.1016/j.watres.2014.08.053
Qi, C., Liu, X., Lin, C., Zhang, X., Ma, J., Tan, H., et al. (2014). Degradation of sulfamethoxazole by microwave−activated persulfate: Kinetics, mechanism and acute toxicity. Chem. Eng. J. 249, 6–14. doi:10.1016/j.cej.2014.03.086
Qiu, M., Hu, B., Chen, Z., Yang, H., Zhuang, L., and Wang, X. (2021). Challenges of organic pollutant photocatalysis by biochar−based catalysts. Biochar 3 (2), 117–123. doi:10.1007/s42773-021-00098-y
Qiu, M., Liu, L., Ling, Q., Cai, Y., Yu, S., Wang, S., et al. (2022). Biochar for the removal of contaminants from soil and water: A review. Biochar 4 (1), 19. doi:10.1007/s42773-022-00146-1
Qu, J., Xu, Y., Zhang, X., Sun, M., Tao, Y., Zhang, X., et al. (2022). Ball milling−assisted preparation of N−doped biochar loaded with ferrous sulfide as persulfate activator for phenol degradation: Multiple active sites−triggered radical/non−radical mechanism. Appl. Catal. B Environ. 316, 121639. doi:10.1016/j.apcatb.2022.121639
Quoc, T. N., Nguyen, K. H., Thuy, H. N. T., Tien, N. T. H., Minh, C. T. T., Dao, V., et al. (2021). Feasibility of using sequential sulfurized nanoscale zerovalent Iron−Persulfate process to degrade tetrabromobisphenol A. J. Nanomater, 2021: 1–8. doi:10.1155/2021/8053120
Rajajayavel, S. R. C., and Ghoshal, S. (2015). Enhanced reductive dechlorination of trichloroethylene by sulfidated nanoscale zerovalent iron. Water Res. 78, 144–153. doi:10.1016/j.watres.2015.04.009
Ren, Y., Lin, L., Ma, J., Yang, J., Feng, J., and Fan, Z. (2015). Sulfate radicals induced from peroxymonosulfate by magnetic ferrospinel MFe2O4 (M = Co, Cu, Mn, and Zn) as heterogeneous catalysts in the water. Appl. Catal. B Environ. 165, 572–578. doi:10.1016/j.apcatb.2014.10.051
Rickard, D., Hatton, B., Murphy, D. M., Butler, I. B., Oldroyd, A., and Hann, A. (2011). FeS−Induced radical formation and its effect on plasmid DNA. Aquat. Geochem 17 (4−5), 545–566. doi:10.1007/s10498-010-9116-x
Savun−Hekimoğlu, B. (2020). A review on sonochemistry and its environmental applications. Acoustics−Basel 2 (4), 766–775. doi:10.3390/acoustics2040042
Shang, W., Dong, Z., Li, M., Song, X., Zhang, M., Jiang, C., et al. (2019). Degradation of diatrizoate in water by Fe(II)−activated persulfate oxidation. Chem. Eng. J. 361, 1333–1344. doi:10.1016/j.cej.2018.12.139
Shao, D., Ren, X., Wen, J., Hu, S., Xiong, J., Jiang, T., et al. (2016). Immobilization of uranium by biomaterial stabilized FeS nanoparticles: Effects of stabilizer and enrichment mechanism. J. Hazard Mater 302, 1–9. doi:10.1016/j.jhazmat.2015.09.043
Shi, Y., Wang, X., Liu, X., Ling, C., Shen, W., and Zhang, L. (2020). Visible light promoted Fe3S4 Fenton oxidation of atrazine. Appl. Catal. B Environ. 277, 119229. doi:10.1016/j.apcatb.2020.119229
Show, B., Mukherjee, N., and Mondal, A. (2017). Reusable iron sulfide nanospheres towards promoted photocatalytic and electrocatalytic activities. New J. Chem. 41 (18), 10083–10095. doi:10.1039/c7nj02018j
Silveira, J. E., Garcia−Costa, A. L., Cardoso, T. O., Zazo, J. A., and Casas, J. A. (2017). Indirect decolorization of azo dye Disperse Blue 3 by electro−activated persulfate. Electrochim Acta 258, 927–932. doi:10.1016/j.electacta.2017.11.143
Song, Q., Feng, Y., Wang, Z., Liu, G., and Lv, W. (2019). Degradation of triphenyl phosphate (TPhP) by CoFe2O4−activated peroxymonosulfate oxidation process: Kinetics, pathways, and mechanisms. Sci. Total Environ. 681, 331–338. doi:10.1016/j.scitotenv.2019.05.105
Song, S., Su, Y., Adeleye, A. S., Zhang, Y., and Zhou, X. (2017). Optimal design and characterization of sulfide−modified nanoscale zerovalent iron for diclofenac removal. Appl. Catal. B Environ. 201, 211–220. doi:10.1016/j.apcatb.2016.07.055
Soori, M., Zarezadeh, K., Sheibani, S., and Rashchi, F. (2016). Mechano−chemical processing and characterization of nano−structured FeS powder. Adv. Powder Technol. 27 (2), 557–563. doi:10.1016/j.apt.2016.02.004
Su, Y., Jassby, D., Song, S., Zhou, X., Zhao, H., Filip, J., et al. (2018). Enhanced oxidative and adsorptive removal of diclofenac in heterogeneous Fenton−like reaction with sulfide modified nanoscale zerovalent iron. Environ. Sci. Technol. 52 (11), 6466–6475. doi:10.1021/acs.est.8b00231
Sühnholz, S., Gawel, A., Kopinke, F., and Mackenzie, K. (2021). Evidence of heterogeneous degradation of PFOA by activated persulfate – FeS as adsorber and activator. Chem. Eng. J. 423, 130102. doi:10.1016/j.cej.2021.130102
Sühnholz, S., Kopinke, F., and Mackenzie, K. (2022). Heterogeneous activation of persulfate by FeS – surface influence on selectivity. Chem. Eng. J. 450, 138192. doi:10.1016/j.cej.2022.138192
Sühnholz, S., Kopinke, F., and Mackenzie, K. (2020). Reagent or catalyst? – FeS as activator for persulfate in water. Chem. Eng. J. 387, 123804. doi:10.1016/j.cej.2019.123804
Sun, M., Cheng, G., Ge, X., Chen, M., Wang, C., Lou, L., et al. (2018a). Aqueous Hg(II) immobilization by chitosan stabilized magnetic iron sulfide nanoparticles. Sci. Total Environ. 621, 1074–1083. doi:10.1016/j.scitotenv.2017.10.119
Sun, Y., Gu, M., Lyu, S., Brusseau, M. L., Li, M., Lyu, Y., et al. (2020). Efficient removal of trichloroethene in oxidative environment by anchoring nano FeS on reduced graphene oxide supported nZVI catalyst: The role of FeS on oxidant decomposition and iron leakage. J. Hazard Mater 392, 122328. doi:10.1016/j.jhazmat.2020.122328
Sun, Y., Liu, Y., Lou, Z., Yang, K., Lv, D., Zhou, J., et al. (2018b). Enhanced performance for Hg(II) removal using biomaterial (CMC/gelatin/starch) stabilized FeS nanoparticles: Stabilization effects and removal mechanism. Chem. Eng. J. 344, 616–624. doi:10.1016/j.cej.2018.03.126
Suroshe, J. S., Mlowe, S., Garje, S. S., and Revaprasadu, N. (2018). Preparation of iron sulfide nanomaterials from iron(II) thiosemicarbazone complexes and their application in photodegradation of methylene blue. J. Inorg. Organomet. P 28 (3), 603–611. doi:10.1007/s10904-018-0816-9
Tan, C., Dong, Y., Fu, D., Gao, N., Ma, J., and Liu, X. (2018). Chloramphenicol removal by zero valent iron activated peroxymonosulfate system: Kinetics and mechanism of radical generation. Chem. Eng. J. 334, 1006–1015. doi:10.1016/j.cej.2017.10.020
Tan, C., Gao, N., Deng, Y., Deng, J., Zhou, S., Li, J., et al. (2014). Radical induced degradation of acetaminophen with Fe3O4 magnetic nanoparticles as heterogeneous activator of peroxymonosulfate. J. Hazard Mater 276, 452–460. doi:10.1016/j.jhazmat.2014.05.068
Turcio−Ortega, D., Fan, D., Tratnyek, P. G., Kim, E., and Chang, Y. (2012). Reactivity of Fe/FeS nanoparticles: Electrolyte composition effects on corrosion electrochemistry. Environ. Sci. Technol. 46 (22), 12484–12492. doi:10.1021/es303422w
Van Koetsem, F., Van Havere, L., and Du Laing, G. (2016). Impact of carboxymethyl cellulose coating on iron sulphide nanoparticles stability, transport, and mobilization potential of trace metals present in soils and sediment. J. Environ. Manage 168, 210–218. doi:10.1016/j.jenvman.2015.10.047
Wang, G., Yang, Y., Xu, X., Zhang, S., Yang, Z., Cheng, Z., et al. (2023b). Rape straw supported FeS nanoparticles with encapsulated structure as peroxymonosulfate and hydrogen peroxide activators for enhanced oxytetracycline degradation. Molecules 28 (6), 2771. doi:10.3390/molecules28062771
Wang, J., Liao, Z., Ifthikar, J., Shi, L., Chen, Z., and Chen, Z. (2017). One−step preparation and application of magnetic sludge−derived biochar on acid orange 7 removal via both adsorption and persulfate based oxidation. Rsc Adv. 7 (30), 18696–18706. doi:10.1039/c7ra01425b
Wang, J., and Wang, S. (2018). Activation of persulfate (PS) and peroxymonosulfate (PMS) and application for the degradation of emerging contaminants. Chem. Eng. J. 334, 1502–1517. doi:10.1016/j.cej.2017.11.059
Wang, J., Xiong, B., Miao, L., Wang, S., Xie, P., Wang, Z., et al. (2021). Applying a novel advanced oxidation process of activated peracetic acid by CoFe2O4 to efficiently degrade sulfamethoxazole. Appl. Catal. B Environ. 280, 119422. doi:10.1016/j.apcatb.2020.119422
Wang, L., Huang, X., Han, M., Lyu, L., Li, T., Gao, Y., et al. (2019). Efficient inhibition of photogenerated electron−hole recombination through persulfate activation and dual−pathway degradation of micropollutants over iron molybdate. Appl. Catal. B Environ. 257, 117904. doi:10.1016/j.apcatb.2019.117904
Wang, Q., Tian, S., and Ning, P. (2014). Degradation mechanism of methylene blue in a heterogeneous Fenton−like reaction catalyzed by ferrocene. Ind. Eng. Chem. Res. 53 (2), 643–649. doi:10.1021/ie403402q
Wang, X., Wang, Y., Chen, N., Shi, Y., and Zhang, L. (2020c). Pyrite enables persulfate activation for efficient atrazine degradation. Chemosphere 244, 125568. doi:10.1016/j.chemosphere.2019.125568
Wang, Y., Gu, Z., Yang, S., and Zhang, A. (2020a). Activation of persulfate by microwave radiation combined with FeS for treatment of wastewater from explosives production. Environ. Sci. Water Res. Technol. 6 (3), 581–592. doi:10.1039/c9ew00803a
Wang, Y., Gu, Z., Yang, S., and Zhang, A. (2020b). Performance of a microwave radiation induced persulfate−hydrogen peroxide binary−oxidant process in treating dinitrodiazophenol wastewater. Sep. Purif. Technol. 236, 116253. doi:10.1016/j.seppur.2019.116253
Wang, Y., Lin, Y., Yang, C., Wu, S., Fu, X., and Li, X. (2023a). Calcination temperature regulates non−radical pathways of peroxymonosulfate activation via carbon catalysts doped by iron and nitrogen. Chem. Eng. J. 451, 138468. doi:10.1016/j.cej.2022.138468
Watson, J. H. P., Croudace, I. W., Warwick, P. E., James, P. A. B., Charnock, J. M., and Ellwood, D. C. (2001). Adsorption of radioactive metals by strongly magnetic iron sulfide nanoparticles produced by sulfate−reducing bacteria. Sep. Sci. Technol. 36 (12), 2571–2607. doi:10.1081/ss-100107214
Wei, X., Yuan, H., Li, J., Chen, T., Yuan, Y., Chen, W., et al. (2022). Reactive oxygen species generated in iron sulfide mediated advanced oxidation systems: A critical review of mechanisms and implications for geochemistry and environmental remediation. J. Environ. Chem. Eng. 10 (6), 108841. doi:10.1016/j.jece.2022.108841
Wei, Z., Villamena, F. A., and Weavers, L. K. (2017). Kinetics and mechanism of ultrasonic activation of persulfate: An in situ EPR spin trapping study. Environ. Sci. Technol. 51 (6), 3410–3417. doi:10.1021/acs.est.6b05392
Wharton, M. J., Atkins, B., Charnockab, J. M., Pattrick, R. A. D., and Collison, D. (2000). An X−ray absorption spectroscopy study of the coprecipitation of Tc and Re with mackinawite (FeS). Appl. Geochem 15 (3), 347–354. doi:10.1016/s0883-2927(99)00045-1
Wu, J., Wang, B., Cagnetta, G., Huang, J., Wang, Y., Deng, S., et al. (2020a). Nanoscale zero valent iron−activated persulfate coupled with Fenton oxidation process for typical pharmaceuticals and personal care products degradation. Sep. Purif. Technol. 239, 116534. doi:10.1016/j.seppur.2020.116534
Wu, J., Wang, X., and Zeng, R. J. (2017). Reactivity enhancement of iron sulfide nanoparticles stabilized by sodium alginate: Taking Cr (VI) removal as an example. J. Hazard Mater 333, 275–284. doi:10.1016/j.jhazmat.2017.03.023
Wu, S., Liu, H., Yang, C., Li, X., Lin, Y., Yin, K., et al. (2020b). High−performance porous carbon catalysts doped by iron and nitrogen for degradation of bisphenol F via peroxymonosulfate activation. Chem. Eng. J. 392, 123683. doi:10.1016/j.cej.2019.123683
Wu, S., Yang, C., Lin, Y., and Cheng, J. J. (2022). Efficient degradation of tetracycline by singlet oxygen−dominated peroxymonosulfate activation with magnetic nitrogen−doped porous carbon. J. Environ. Sci. 115, 330–340. doi:10.1016/j.jes.2021.08.002
Wu, X., Gu, X., Lu, S., Qiu, Z., Sui, Q., Zang, X., et al. (2015). Strong enhancement of trichloroethylene degradation in ferrous ion activated persulfate system by promoting ferric and ferrous ion cycles with hydroxylamine. Sep. Purif. Technol. 147, 186–193. doi:10.1016/j.seppur.2015.04.031
Xia, C., Liu, Q., Zhao, L., Wang, L., and Tang, J. (2022). Enhanced degradation of petroleum hydrocarbons in soil by FeS@BC activated persulfate and its mechanism. Sep. Purif. Technol. 282, 120060. doi:10.1016/j.seppur.2021.120060
Xiang, W., Chen, H., Zhong, Z., Zhang, C., Lu, X., Huang, M., et al. (2022). Efficient degradation of carbamazepine in a neutral sonochemical FeS/persulfate system based on the enhanced heterogeneous−homogeneous sulfur−iron cycle. Sep. Purif. Technol. 282, 120041. doi:10.1016/j.seppur.2021.120041
Xiang, W., Huang, M., Wang, Y., Wu, X., Zhang, F., Li, D., et al. (2020). New insight in the O2 activation by nano Fe/Cu bimetals: The synergistic role of Cu(0) and Fe(II). Chin. Chem. Lett. 31 (10), 2831–2834. doi:10.1016/j.cclet.2020.08.006
Xie, Y. F., Li, X. D., and Li, F. D. (2013). Study on application of biological iron sulfide composites in treating vanadium−extraction wastewater containing chromium (VI) and chromium reclamation. J. Environ. Biol. 34 (2), 301–305.
Xiong, Z., He, F., Zhao, D., and Barnett, M. O. (2009). Immobilization of mercury in sediment using stabilized iron sulfide nanoparticles. Water Res. 43 (20), 5171–5179. doi:10.1016/j.watres.2009.08.018
Xu, H., and Sheng, Y. (2021). New insights into the degradation of chloramphenicol and fluoroquinolone antibiotics by peroxymonosulfate activated with FeS: Performance and mechanism. Chem. Eng. J. 414, 128823. doi:10.1016/j.cej.2021.128823
Xu, J., Wang, Y., Weng, C., Bai, W., Jiao, Y., Kaegi, R., et al. (2019). Reactivity, selectivity, and Long−Term performance of sulfidized nanoscale zerovalent iron with different properties. Environ. Sci. Technol. 53 (10), 5936–5945. doi:10.1021/acs.est.9b00511
Xu, L., Li, L., Fang, P., Chang, K., Chen, C., and Liao, Q. (2022). Removal of uranium (VI) ions from aqueous solution by graphitic carbon nitride stabilized FeS nanoparticles. J. Mol. Liq. 345, 117050. doi:10.1016/j.molliq.2021.117050
Yan, S., Luo, S., Feng, J., Li, P., Guo, R., Wang, Q., et al. (2020). Rational design of flower−like FeCo2S4/reduced graphene oxide films: Novel binder−free electrodes with ultra−high conductivity flexible substrate for high−performance all−solid−state pseudocapacitor. Chem. Eng. J. 381, 122695. doi:10.1016/j.cej.2019.122695
Yan, Z., Gu, Y., Wang, X., Hu, Y., and Li, X. (2021). Degradation of aniline by ferrous ions activated persulfate: Impacts, mechanisms, and by−products. Chemosphere 268, 129237. doi:10.1016/j.chemosphere.2020.129237
Yang, S., He, C., Xie, Z., Li, L., Xiong, Z., Zhang, H., et al. (2022). Efficient activation of PAA by FeS for fast removal of pharmaceuticals: The dual role of sulfur species in regulating the reactive oxidized species. Water Res. 217, 118402. doi:10.1016/j.watres.2022.118402
Yangju, L., Xiuge, Z., Yan, Y., Jianfei, Y., Yuting, P., Yunhong, Z., et al. (2019). Enhanced sulfamethoxazole degradation by peroxymonosulfate activation with sulfide−modified microscale zero−valent iron (S−mFe0): Performance, mechanisms, and the role of sulfur species. Chem. Eng. J. 376 (C), 121302. doi:10.1016/j.cej.2019.03.178
Ye, Z., Padilla, J. A., Xuriguera, E., Beltran, J. L., Alcaide, F., Brillas, E., et al. (2020). A highly stable metal–organic Framework−Engineered FeS2/C nanocatalyst for heterogeneous Electro−Fenton treatment: Validation in wastewater at mild pH. Environ. Sci. Technol. 54 (7), 4664–4674. doi:10.1021/acs.est.9b07604
Yu, Z., Ma, J., Huang, X., Lv, Y., Liu, Y., Lin, C., et al. (2022). Insights into enhanced peroxydisulfate activation with S doped Fe@C catalyst for the rapid degradation of organic pollutants. J. Colloid Interf. Sci. 610, 24–34. doi:10.1016/j.jcis.2021.12.046
Yuan, Y., Tao, H., Fan, J., and Ma, L. (2015). Degradation of p−chloroaniline by persulfate activated with ferrous sulfide ore particles. Chem. Eng. J. 268, 38–46. doi:10.1016/j.cej.2014.12.092
Zhang, J., Qiu, S., Feng, H., Hu, T., Wu, Y., Luo, T., et al. (2022). Efficient degradation of tetracycline using core–shell Fe@Fe2O3−CeO2 composite as novel heterogeneous electro−Fenton catalyst. Chem. Eng. J. 428, 131403. doi:10.1016/j.cej.2021.131403
Zhang, P., Tan, X., Liu, S., Liu, Y., Zeng, G., Ye, S., et al. (2019a). Catalytic degradation of estrogen by persulfate activated with iron−doped graphitic biochar: Process variables effects and matrix effects. Chem. Eng. J. 378 (2), 122141. doi:10.1016/j.cej.2019.122141
Zhang, S., Lyu, H., Tang, J., Song, B., Zhen, M., and Liu, X. (2019b). A novel biochar supported CMC stabilized nano zero−valent iron composite for hexavalent chromium removal from water. Chemosphere 217, 686–694. doi:10.1016/j.chemosphere.2018.11.040
Zhang, W., Gao, J., Duan, W., Zhang, D., Jia, J., and Wang, Y. (2020). Sulfidated nanoscale zero−valent iron is an efficient material for the removal and regrowth inhibition of antibiotic resistance genes. Environ. Pollut. 263, 114508. doi:10.1016/j.envpol.2020.114508
Zhao, L., Ji, Y., Kong, D., Lu, J., Zhou, Q., and Yin, X. (2016). Simultaneous removal of bisphenol A and phosphate in zero−valent iron activated persulfate oxidation process. Chem. Eng. J. 303, 458–466. doi:10.1016/j.cej.2016.06.016
Zhao, Z., Pan, S., Ye, Y., Zhang, X., and Pan, B. (2020). FeS2/H2O2 mediated water decontamination from p−arsanilic acid via coupling oxidation,adsorption and coagulation: Performance and mechanism. Chem. Eng. J. 381 (2), 122667. doi:10.1016/j.cej.2019.122667
Zheng, M., Lu, J., and Zhao, D. (2018). Toxicity and transcriptome sequencing (RNA−seq) analyses of adult zebrafish in response to exposure carboxymethyl cellulose stabilized iron sulfide nanoparticles. Sci. Rep−Uk 8 (1), 8083. doi:10.1038/s41598-018-26499-x
Zhou, L., Liu, J., and Dong, F. (2017). Spectroscopic study on biological mackinawite (FeS) synthesized by ferric reducing bacteria (FRB) and sulfate reducing bacteria (SRB): Implications for in−situ remediation of acid mine drainage. Spectrochimica Acta Part A Mol. Biomol. Spectrosc. 173, 544–548. doi:10.1016/j.saa.2016.09.053
Zhou, X., Luo, M., Xie, C., Wang, H., Wang, J., Chen, Z., et al. (2021a). Tunable S doping from Co3O4 to Co9S8 for peroxymonosulfate activation: Distinguished Radical/Nonradical species and generation pathways. Appl. Catal. B Environ. 282, 119605. doi:10.1016/j.apcatb.2020.119605
Zhou, X., Zeng, Z., Zeng, G., Lai, C., Xiao, R., Liu, S., et al. (2020). Insight into the mechanism of persulfate activated by bone char: Unraveling the role of functional structure of biochar. Chem. Eng. J. 401, 126127. doi:10.1016/j.cej.2020.126127
Zhou, Y., Wang, X., Zhu, C., Dionysiou, D. D., Zhao, G., Fang, G., et al. (2018). New insight into the mechanism of peroxymonosulfate activation by sulfur−containing minerals: Role of sulfur conversion in sulfate radical generation. Water Res. 142, 208–216. doi:10.1016/j.watres.2018.06.002
Zhou, Z., Huang, J., Xu, Z., Ali, M., Shan, A., Fu, R., et al. (2021b). Mechanism of contaminants degradation in aqueous solution by persulfate in different Fe(II)−based synergistic activation environments: Taking chlorinated organic compounds and benzene series as the targets. Sep. Purif. Technol. 273, 118990. doi:10.1016/j.seppur.2021.118990
Zhu, K., Xu, H., Chen, C., Ren, X., Alsaedi, A., and Hayat, T. (2019). Encapsulation of Fe0−dominated Fe3O4/Fe0/Fe3C nanoparticles into carbonized polydopamine nanospheres for catalytic degradation of tetracycline via persulfate activation. Chem. Eng. J. 372, 304–311. doi:10.1016/j.cej.2019.04.157
Keywords: iron sulfide, water remediation, persulfate, catalytic mechanisms, synthesis
Citation: Sun Y, Liu J, Fan X, Li Y and Peng W (2023) Synthesis and application of iron sulfide−based materials to activate persulfates for wastewater remediation: a review. Front. Environ. Sci. 11:1212355. doi: 10.3389/fenvs.2023.1212355
Received: 28 April 2023; Accepted: 30 May 2023;
Published: 12 June 2023.
Edited by:
Xiaofei Tan, Hunan University, ChinaReviewed by:
Zacharias Frontistis, University of Western Macedonia, GreeceJing Zou, Huaqiao University, China
Shaohua Wu, Guangdong University of Petrochemical Technology, China
Copyright © 2023 Sun, Liu, Fan, Li and Peng. This is an open-access article distributed under the terms of the Creative Commons Attribution License (CC BY). The use, distribution or reproduction in other forums is permitted, provided the original author(s) and the copyright owner(s) are credited and that the original publication in this journal is cited, in accordance with accepted academic practice. No use, distribution or reproduction is permitted which does not comply with these terms.
*Correspondence: Wenchao Peng, d2VuY2hhby5wZW5nQHRqdS5lZHUuY24=