- 1Collaborative Innovation Center of Atmospheric Environment and Equipment Technology, Jiangsu Key Laboratory of Atmospheric Environment Monitoring and Pollution Control, School of Environmental Science and Engineering, Nanjing University of Information Science and Technology, Nanjing, China
- 2Shenzhen Academy of Environmental Sciences, Shenzhen, China
- 3School of Materials and Environmental Engineering, Shenzhen Polytechnic, Shenzhen, China
The strong stability of Cu–ethylenediaminetetraacetic acid (Cu–EDTA) results in the low decomplexation efficiency by the traditional Fenton process. For breaking this limitation, a three-dimensional electro-Fenton (3D-EF) system was constructed to study the decomplexation of Cu–EDTA at different pH, and the effects of Fe2+ concentration, particle electrode dosage, current density, and coexisting ions on decomplexation performance were investigated. The results showed that 3D-EF exhibited high pollutant removal efficiency in a wide pH range compared with the traditional electro-Fenton process. The optimal conditions for the removal of Cu–EDTA were as follows: the pH was 7, Fe2+ was 1 mmol L−1, granular activated carbon was 2 g L−1, and current density was 10 mA cm−2, and the optimum Cu–EDTA removal efficiency reached 90.95%. In addition, the presence of Cl− slightly improved the decomplexation efficiency, whereas NO3− and HPO42− inhibited the removal of Cu–EDTA. The kinetics of Cu–EDTA decomplexation in all experimental groups followed the first-order kinetic equation.
Introduction
The chelator ethylenediaminetetraacetic acid (EDTA) is widely used in electroplating, metallurgical, and chemical cleaning industries to form stable complexes with heavy metals of different structures (Wang et al., 2019; Wang Y. et al., 2020). Their efficient removals remain a key challenge in the field of wastewater treatment. Cu–ethylenediaminetetraacetic acid (Cu–EDTA), a strong stability complex compound in wide pH range of wastewater, poses potential hazards to water, soil environments, and human health (Song et al., 2021), and it is difficult to remove from wastewater by chemical precipitation and other processes. Therefore, appropriate methods should be taken to overcome this problem (Hu et al., 2021; Wang and Chen, 2021; Wang and Tang, 2021).
Some promising treatment methods for removing the stable complex from wastewater have received extensive attention, such as electro-coagulation (Xie et al., 2020), nonthermal plasma oxidation (Wang et al., 2019; Liu et al., 2021), photo-electrocatalytic oxidation (He et al., 2021; Shah and Patel, 2021), ozone-based oxidation (Zhao et al., 2018a; Guan et al., 2022), and microwave-assisted Fenton reaction (Wang H. et al., 2020; Xia et al., 2021). Electrochemical advanced oxidation processes (EAOPs) are sought-after for many researchers due to their high degradation efficiency, versatility, and environmental friendliness (Wang and Zhuan, 2020; Wang and Chen, 2021; Wang and Wang, 2021). The hydroxyl radical (·OH) produced from the electro-Fenton (EF) process by the reaction of Fe2+ and H2O2 solution owns the characteristics of strong oxidation, high reactivity, easy formation, non-selectivity, and harmlessness, which has been proved to have perfect removal performance for the stable complex in wastewater (Wang and Wang, 2018; Chen and Wang, 2021). Chu et al. (2022) prepared several composite cathodes using graphite, carbon nanotube, and polytetrafluoroethylene, and the degradation efficiency of cefepime reached nearly 100% at pH 3.0. Zhang et al. (2022) synthesized a novel core-shell Fe@Fe2O3–CeO2 composite for effective degradation of the tetracycline (TC) pollutant, and TC removal efficiency reached 90.7% within 1 h at the optimum pH of 3.85. Midassi et al. (2020) used the carbon-felt cathode and boron-doped diamond anode to completely remove chloroquine at pH 3.0. Hence, three-dimensional electro-Fenton (3D-EF) is appropriate for refractory organics removal, and whether it owns the same decomplexation efficiency on Cu–EDTA needs to be further studied.
The EF reaction could produce high activity only in a narrow pH range (may be in pH 2.5–3.5), which greatly limits the application of EF technology. In order to solve the problems, the 3D-EF system has been constructed by adding particle electrodes between the anode and cathode. It was reported that the rhodamine B removal reached 91.6% in the 3D-EF system in the presence of Fe–Cu/kaolin particle electrodes (Zhang et al., 2019). Iron foam was used as the particle electrode in a 3D-EF system in the study of Zheng et al. (2019) and found that the chemical oxygen demand (COD) and total nitrogen (TN) removal percentage in folic acid wastewater reached 43.5 and 70.4%, respectively, under the following optimal conditions: the initial COD concentration of 10 g L−1, applied current of 0.3 A, initial pH of 3, and particle electrode of 4 g. It is noteworthy that the Long’s team reported that the diclofenac removal rate reached 96.3% in the presence of manganese slag–loaded active substance as a particle electrode (Long et al., 2019). Among them, it can be noted the work of Li M. et al. (2021), who prepared Cu–Fe bimetallic aerogel-like carbon as a catalytic particle electrode based on sodium alginate to remove 81.1% of fulvic acids at pH 5.4, voltage 2.5, and catalyst dosage of 4 g L−1.
In the 3D-EF system, the electrode plate, pH, Fe2+, particle electrodes, current density, and coexisting ions could affect the efficiency of pollutant removal. Among them, the electrode material plays a decisive role in the generation of OH, which is a key factor in the wastewater treatment by EF (Ou et al., 2019). According to the reports, graphite electrode plates (carbon materials) have been widely examined and regarded as the most efficient electrode plates (Zhang et al., 2019; Zhang B. et al., 2019; Song et al., 2020). Graphite material plates showed better removal effect for some refractory organics, but less research was carried out on Cu–EDTA using graphite polar plates. Due to the unique functional group structure on the surface of graphite (carbon materials), organics decompose through free radical–induced pathways (Duan et al., 2018). Compared with other electrodes, graphite electrodes are easier to obtain, low-cost, nontoxic, and own a high specific surface area, which will be cost-effective in electrochemical analysis (Le et al., 2017; Zhao K. et al., 2018). In addition, the excellent electrical conductivity of graphite makes it an ideal candidate for electrodes (Pan et al., 2020; Tasić et al., 2021).
In this study, a 3D-EF system with granular-activated carbon (GAC) as the particle electrode was used to remove Cu–EDTA. The effects of main parameters (the pH value of initial solution, Fe2+ concentration, particle electrode dosage, current density, and coexisting ions) on the decomplexation performance of Cu–EDTA were optimized. In addition, the reaction kinetics for all optimization processes were fitted. This study provides a new idea for the decomplexation of Cu–EDTA and other complexes, and provides a suitable way for the treatment of complex heavy metal complexes.
Materials and Methods
Chemicals
All the reagents used in this study were of analytical grade. Ethylenediaminetetraacetic acid disodium salt (C10H14N2Na2O8·2H2O) was purchased from Shanghai Macklin Biochemical Co., Ltd. (Shanghai, China). Copper sulfate (CuSO4·5H2O), sodium chloride (NaCl), sodium nitrate (Na2NO3), disodium hydrogen phosphate (Na2HPO4·12H2O), and sodium sulfate (Na2SO4) were purchased from Tianjin Damao Chemical Reagent Factory. (Tianjin, China). Sodium hydroxide (NaOH) was purchased from Shantou Xilong Scientific Co., Ltd. (Shantou, China). Sulfuric acid (H2SO4) was purchased from Hengyang Kaixin Chemical Reagent Co., Ltd. (Hengyang, China). GAC was obtained from Shanghai Aladdin Inc. (Shanghai, China). The particle size of GAC is approximately 0.6–2 mm. Solutions were prepared with Milli-Q water.
Experimental Setup
In this study, the experimental device consists of a 5 A, -30 V DC-regulated power supply (HXD-305DM), 500-ml beaker, and magnetic stirrer (IKA). The polar plates were dual graphite electrode plates (100 × 50 × 5 mm) with a 70-mm plate spacing and GAC particle electrode. The graphite electrode plate and GAC were boiled in dilute acid and alkali for 30 min, ultrasonic cleaned for 20 min, and dried in an oven at 105°C for 10 h.
Experimental Procedure
The Cu–EDTA reserve solution of 50 mg L−1 was added into a 500-ml breaker with a volume of 300 ml solution for each experiment. Besides, 0.2 mol L−1 NaOH and H2SO4 were used to adjust the initial pH, and 0.047 mol L−1 Na2SO4 was used as the electrolyte. The reaction time was 120 min, and the samples were taken three times at 10, 30, 60, 90, and 120 min. After that, sample’s pH were adjusted to 11 with H2SO4 or NaOH to form hydroxide precipitates of heavy metal ions to remove from the supernatant and diluted 500 times for further detection. The concentration of the remaining heavy metal complex in samples was determined by inductively coupled plasma mass spectrometry (ICP-MS) after being filtered by a 0.22-µm membrane.
Cu–EDTA Degradation by 3D-EF
In order to prove the advantage of the 3D-EF system for the decomplexation of Cu–EDTA, a GAC system (pH = 3 and Fe2+ = 1 mmol L−1), EF system (pH = 3, Fe2+ = 1 mmol L−1, and current density 4 mA cm−2), and 3D-EF system (pH = 3, Fe2+ = 1 mmol L−1, GAC 2 g L−1, and current density 4 mA cm−2) were set up. Three samples were taken at 10, 30, 60, 90 and 120 min to detect the Cu concentration.
Parameter Optimization of 3D-EF Degradation
The relevant factors include pH, Fe2+, particle electrodes, and current density to assess the efficiency in removing Cu–EDTA via the 3D-EF. For investigating the effect of pH on the decomplexation of Cu–EDTA, the changes of Cu–EDTA removal efficiency in different pH (pH = 3, 5, 7, and 9) were investigated. Different amounts of Fe2+ (0.5, 1.0, 1.5, and 2.0 mmol L−1 Fe2+) were put into the solution to study the effect of Fe2+ dosages on Cu–EDTA decomplexation. This was followed up by using the most suitable pH solution obtained from the previous experiment. Subsequently, different doses of the particle electrode (doses of 1, 2, 3, 4, 5, and 6 g L−1) were added to discuss the effect of the particle electrode on the decomplexation of Cu–EDTA. The most suitable conditions such as pH, Fe2+ dosages, and particle electrode were prepared. A certain current density (2, 4, 6, 8, 10, and 12 mA cm−2) was added to study its effect on Cu–EDTA decomplexation.
Effect of Coexisting Ions on Cu–EDTA Removal
The effects of coexisting ions (Cl−, NO3−, and HPO42−) were studied in terms of the most suitable conditions such as solution pH of 7, 1 mmol L−1 Fe2+, 4 g L−1 GAC, and current density of 10 mA cm−2.
Chemical Analytical Methods
The solution pH was measured by a pH meter (PB-10, Sartorius). During the electrolysis, samples were collected at specific time intervals for copper ion determination. If not noted otherwise, each experiment was repeated three times to ensure the repeatability of experimental results. The concentrations of copper ions were determined by inductively coupled plasma mass spectrometry (ICP-MS, NexlON 2000). The conditions for the detection of copper ions were as follows: a radio frequency power of 1500 W, plasma gas flow of 15 L min−1, and auxiliary gas flow of 1.0 L min−1 and nebulization gas flow of 1.0 L min−1.
Statistical Analysis
Three replicates were conducted for each sample, and average values were calculated and shown in charts. Origin 8.6 software was used for drawing figures.
The Cu–EDTA removal rate was calculated by Eq. 1.
where R is the removal efficiency at time (t), C0 is the initial concentration, and Ct is the copper ion concentration at time (t).
The term removal rate refers to the kinetic process; the Cu–EDTA decay is followed by the first-order reaction kinetics with reaction rate constants (Kobs) determined in min−1 according to Eq. 2.
where t is the electrolysis time (min).
Results and Discussion
Removal of Cu–EDTA by 3D-EF
Three different reaction systems, i.e., GAC adsorption system, EF reaction system, and 3D-EF reaction system were studied to prove the advantage of the 3D-EF process for the decomplexation of Cu–EDTA, and the results were demonstrated in Figure 1. The decomplexation efficiency of Cu–EDTA increased with the reaction time extended in all experience groups (Figure 1A), and the removal rates of Cu–EDTA were 11.37, 75.46, and 86.81% in three groups, respectively. The 3D-EF reaction system owned higher removal efficiency than that of GAC adsorption and EF processes. From Figure 1B; Table 1, it is noted that the degradation of Cu–EDTA in all systems conformed to the first-order kinetic model, and the reaction rate constant Kobs of the 3D-EF, GAC, and EF were 0.0175, 0.0126, and 0.0012, respectively, which indicated that the 3D-EF reaction system owns a good application prospect for Cu–EDTA decomplexation. Hu et al. (2021) prepared the Ni/GO-PAC particle electrode, and Cu–EDTA degradation reached 99% at 1 mmol L−1 Cu–EDTA, current density of 1.6 mA cm−2, and pH = 3.15. Also, Song et al. (2021) used RuO2–IrO2/Ti and Al electrodes to treat Cu–EDTA wastewater, and the Cu removal efficiency reached 99.85%. Zhao et al. (2018b) explored the application of the EF technique by using iron-sacrificing electrodes, and the effluent could meet discharge standard (0.3 mg L−1 for Cu in China) under the initial pH of 2.0, H2O2 dosage of 6 ml L−1 h−1, current density of 20 mA cm−2, and sulfate electrolyte concentration of 2 g L−1.
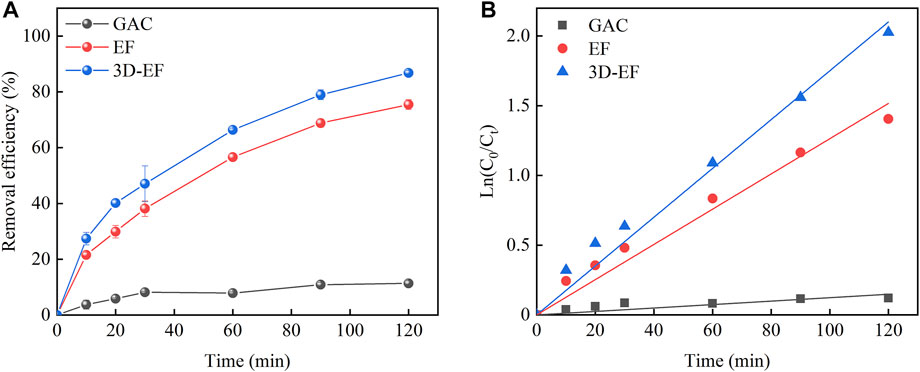
FIGURE 1. (A) Removal efficiency of Cu–EDTA in different reaction systems and (B) kinetics fitting of Cu–EDTA removal in different reaction systems. (pH = 3 and Fe2+ = 1 mmol L−1).
In the 3D-EF process, the additional particle electrode adsorbed pollutants, and the contaminated materials underwent electrochemical degradation on the surface of the particle electrode to achieve the efficient removal. The lower current was able to enhance the removal efficiency of pollutants, which could greatly improve the chemical reaction rate and treatment effect. Therefore, the 3D-EF reaction system exhibited better performance than the conventional EF reaction system. Theoretically, using activated carbon as the particle electrode in the EF reaction system was able to enhance Cu–EDTA removal, which was related to the particle electrode increasing the electrode specific surface area, increasing the utilization of the whole space, and improving the mass transfer effect (Zhang et al., 2018; Li H. et al., 2021), and a synergistic strengthening effect should appear with the combination of the activated carbon and electrochemical process. However, this synergy was not obvious in this study. It might because that the adsorption of Cu–EDTA by activated carbon was presented and occupied the active sites of the particle electrodes, and with the reaction extending, the Fe2+ and ∙OH on the surface of the particle electrode formed the Fenton reaction, whose products (Fe3+ and Cu2+) took the place of these sites to inhibit the synergistic strengthening performance.
pH Application Window for Cu–EDTA Decomplexation by 3D-EF
Different initial pH conditions could affect the degradation efficiency of Cu–EDTA and is illustrated in Figure 2A. The removal efficiency of Cu–EDTA reached more than 80% at different initial pH conditions, and the removal rates of Cu–EDTA were 84.22, 88.04, 90.19, and 87.74%, when the pH ranged from 3 to 9. Among them, the Cu–EDTA removal rate reached the maximum when the pH was 7. The experimental results proved that using GAC particles as the particle electrode in a 3D-EF system broke a limitation of pH conditions, which enabled the 3D-EF reaction system to better treat various types of water quality and greatly broadened the application window of pH. The kinetics of Cu–EDTA removal at different pH conditions were fitted as shown in Figure 2B. The reaction rate constant Kobs of Cu–EDTA reaches maximum at pH 7 and reaction time 120 min. The reaction rate constant Kobs showed a tendency of increasing at first and then decreasing with the increase of the initial pH, which was the same as the removal effect of Cu–EDTA (Table 2).
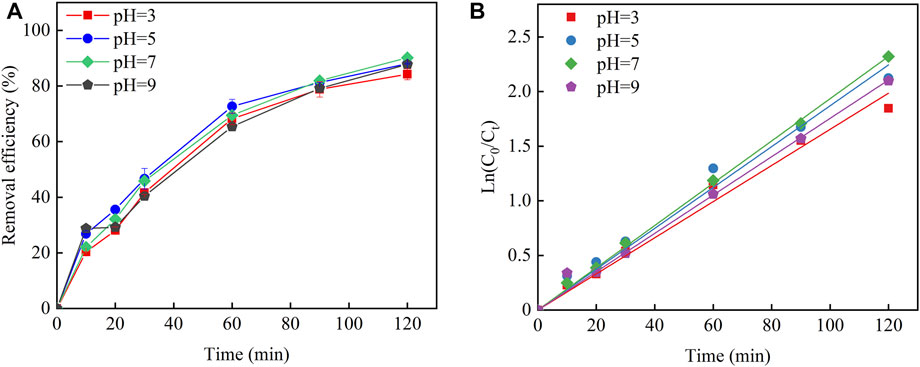
FIGURE 2. (A) Effects of pH application on the Cu–EDTA complex-breaking removal effect of Cu–EDTA and (B) kinetic fitting of Cu–EDTA removal effect.
The lower pH values tend to have higher pollutant removal rates in many EF studies. Shi et al. (2021) demonstrated that the removal rate of ibuprofen could reach 90.2% in 120 min at pH 3.0 and current density of 10 mA cm−2. Also, for aniline, ciprofloxacin, and cefazolin, their removal efficiencies were more than 99, 91.30, and 95.8% under acid condition, respectively (Ma et al., 2021; Yao et al., 2021; Ghasemi et al., 2020). GAC particles, particle electrodes used in a 3D-EF system, made this system remove Cu–EDTA better under neutral conditions. The reason could be explained as follows: low pH value contributed to higher oxygen evolution potential and direct oxidation played the dominant role. However, there would be a certain side reaction of hydrogen forming in the negative area, which inevitably occupied some of the active spots (Eq. 3) (Guan et al., 2018). Under acidic conditions, the Fenton reaction occurred between Fe2+ and ∙OH on the electrode surface of GAC particles, which could improve the oxidation ability of the whole reaction system and enhance the removal effect of Cu–EDTA. On the contrary, with the pH increase, the side effect of oxygen forming would occur in the positive area. The decomposition of H2O2 into H2O and O2 under alkaline conditions was also occurred in a certain scavenging reaction with ∙OH. In turn, the ∙OH on the particle electrode surface decreased and the oxidation capacity was somewhat diminished, so that the treatment efficacy decreased at high pH values (Eqs 4–6) (Zheng et al., 2019; Zhang M.-h. et al., 2019). Therefore, the operation of the 3D-EF system with GAC particles at neutral or near neutral pH reduced the chemical requirements for pH regulation and reduced operating costs to achieve optimal treatment performance.
Influencing Factors of Cu–EDTA Removal by 3D-EF
Fe2+ Dosage
The Fe2+ dosage has certain influence on Cu–EDTA removal in a 3D-EF process. As shown in Figure 3A, the removal rate of Cu–EDTA presented an increasing trend first and then decreased with the increase of Fe2+ dosage. The highest removal efficiency could reach up to 90.95%, which was achieved at a Fe2+ dosage of 1 mmol L−1 after 120 min reaction. Kinetic fitting of Fe2+ dosage for Cu–EDTA removal is shown in Figure 3B with the increase of Fe2+ concentration. The reaction rate constant Kobs also showed a trend of increasing first and then decreasing, and the reaction rate constant Kobs peaked at the Fe2+ dosage of 1 mmol L−1. The reaction rate constant Kobs reached a maximum of 0.0215 at 1 mmol L−1 Fe2+ (Table 3).
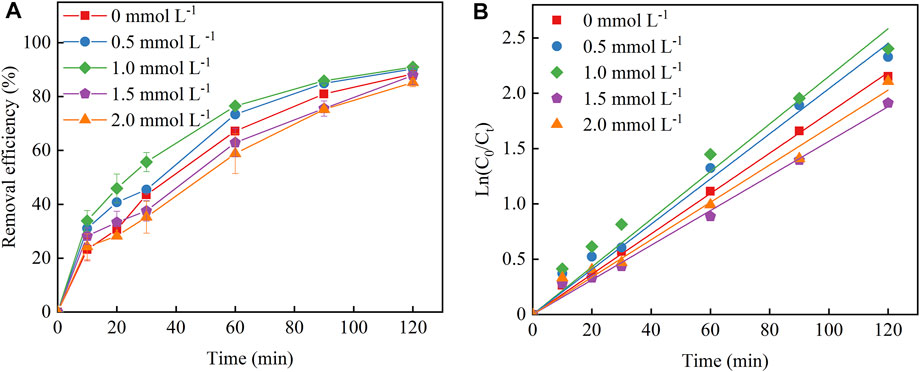
FIGURE 3. (A) Effects of Fe2+ dosage on the removal effect of Cu–EDTA and (B) kinetic fitting of removal effect of Cu–EDTA.
Fe2+ was the core of the 3D-EF reaction system. The removal of Cu–EDTA depended mainly on the reaction of the plate and the particles without Fe2+ adding into this reaction system. The activity of microelectrolysis was weak with low-dosage Fe2+ addition, and the Cu–EDTA removal rate gradually increased with increasing Fe2+ dosage to generate more ∙OH through the system reaction (Eq. 7) (Feng et al., 2010; Chu et al., 2021). Too high Fe2+ concentration increased the contact probability of Fe2+ and H2O2 and also subsequently increased the ∙OH production. However, too much of Fe2+ and ·OH produced side effects and formed Fe3+, which consumed a large amount of ·OH, resulting in the decline of the Cu–EDTA removal rate in the 3D-EF reaction system (Eq. 8) (Huang et al., 2020; Zhang et al., 2020).
Particle Electrode Dosage
Particle electrode loading was also another key factor affecting the 3D-EF reaction system to treat Cu–EDTA. As seen in the inset of Figure 4A, the removal rate of Cu–EDTA was similar with that of the Fe2+ dosage experiment group, and the highest removal rate of Cu–EDTA was 87.71% after 120 min of reaction when the particle electrode was 4 g L−1 Figure 4B; Table 4 showed that the removal efficiency of the Cu–EDTA reaction rate constant Kobs reached a maximum value of 0.0190 at the particle electrode dosage of 4 g L−1.
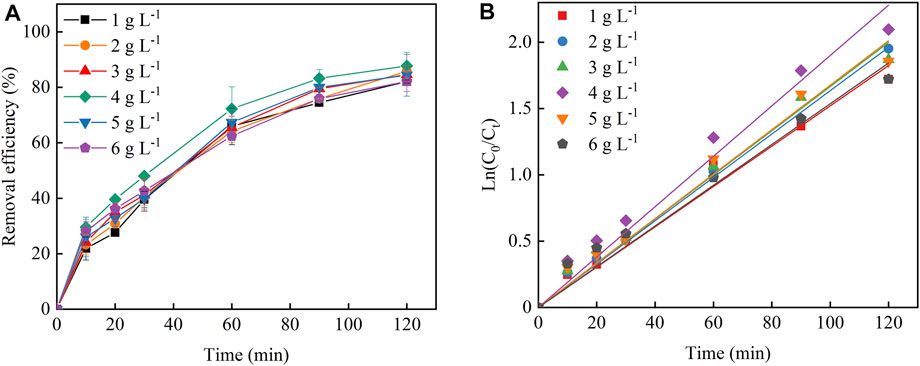
FIGURE 4. (A) Effects of particle electrode dosage on the Cu–EDTA removal effect and (B) kinetic fitting of Cu–EDTA removal effect.
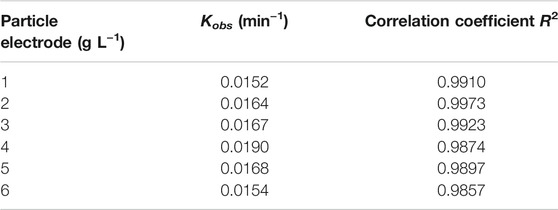
TABLE 4. Pseudo-first-order kinetics parameters of Cu–EDTA removal with different particle electrode dosages.
The particle electrode was distributed throughout the reaction system and formed microelectrode particles under the action of an electric field, which increased the specific surface area of the electrode, shortened the electrode spacing, greatly improved the mass transfer rate and current utilization efficiency, and improved the treatment capacity of pollutants (Dargahi et al., 2021). As the particle electrode increased, the surface area and the adsorption sites on the electrode increased, and then the Cu–EDTA removal efficiency increased accordingly (Wang et al., 2021). With the continuous increase of the particle electrode, the excess particle electrode occupied more space in the reaction system, which made the reaction system overloaded. In turn, the current and mass efficiencies will also be affected, such as leading to the short circuiting of the particle electrode and inhibiting the removal of contaminants (Zheng et al., 2016; Ghanbarlou et al., 2020).
Current Density
Figure 5A shows the removal effect of Cu–EDTA at different current densities. The worst removal effect of Cu–EDTA was 71.52% after 120 min at a current density of 2 mA cm−2. With the increase of current density to 10 mA cm−2, the best removal efficiency of Cu–EDTA was obtained with the removal rate of 87.26%. However, Cu–EDTA removal efficiency showed a decreasing trend as the current density continued to increase to 12 mA cm−2 within the reaction system. In Figure 5B, the reaction rate constants Kobs at a current density of 10 mA cm−2 were much larger than those at other current densities. From Table 5, the reaction rate constant Kobs reached a maximum of 0.0180 at a current density of 10 mA cm−2.
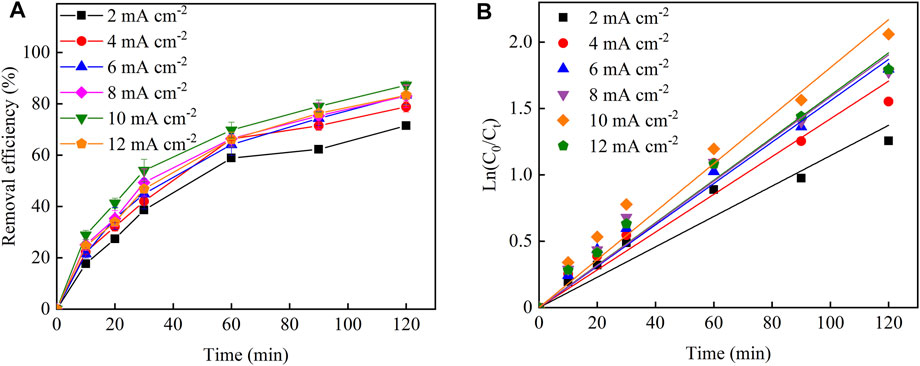
FIGURE 5. (A) Effects of current density on the removal efficiency of Cu–EDTA and (B) kinetic fitting of removal effect of Cu–EDTA.
As the current density increased within the reaction system, the electron density and H2O2 production on the electrode also gradually increased, and Fe2+ reacted with H2O2 to generate ·∙OH (Eq. 9). Moreover, the removal of Cu–EDTA gradually increased because the electron transfer between electrodes increased and accelerated the progress of its direct oxidation reaction on the electrode surface (Barhoumi et al., 2016; Moreira et al., 2019). Further increasing current density did not significantly improve the removal efficiency of Cu–EDTA because excess H2O2 was a scavenger of ·OH. The high current density would accelerate the decomposition of H2O2 and promote the occurrence of competitive side reactions such as hydrogen evolution (Eq. 3) and oxygen evolution (Eq. 10) resulting in the decrease of the concentration of ·OH (Zhang et al., 2020; Zhang et al., 2022).
Coexisting Ions
The efficiency of the 3D-EF reaction system was affected by all kinds of ions in wastewater, which could accelerate the decomposition rate of H2O2 through competitive adsorption. The images as shown in Figure 6A further supported that the coexistent Cl− could promote the removal of pollutions in the 3D-EF reaction system. After 120 min, the Cu–EDTA removal rate reached 87% in the control group, and the experiment group after adding Cl− could achieve 87.55% removal efficiency, which was slightly improved by 0.55% compared with that of the control group. Hence, it can be concluded that Cl− owned a certain promoting effect on the removal of Cu–EDTA by the 3D-EF process. However, the removal efficiency of Cu–EDTA was 85.45 and 82.90%, respectively, when NO3− and HPO42- were added into the reaction system for 120 min, which was 1.55 and 4.1% lower than that of the control group. This phenomenon reflected that NO3− and HPO42− could inhibit the removal of Cu–EDTA.
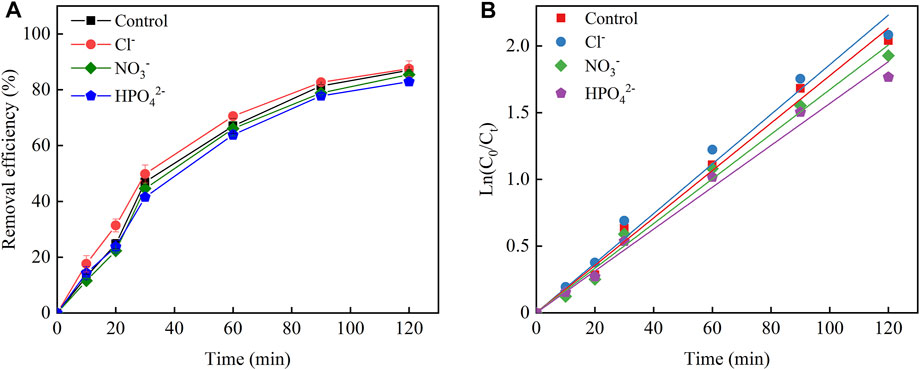
FIGURE 6. (A) Effects of coexisting ions on the removal efficiency of Cu–EDTA and (B) kinetic fitting of removal effect of Cu–EDTA.
From Figure 6B, the slope of the reaction system with the addition of the Cl− group was larger than that of the control group, while the slopes of the reaction system with the addition of NO3− and HPO42− groups were smaller than those of the control group. The reaction rate constant Kobs of 0.0186 for the addition of the Cl− group was larger than that of the control group (0.0178) and so as the addition of NO3− and HPO42− groups (0.0167 and 0.0157, respectively) (Table 6).
It was noticed that a certain promotion of Cu–EDTA removal was found after the addition of Cl−, which was related to the reactive chlorine species generated in this reaction system. Because the active species generated after the addition of Cl− exhibited a higher lifetime than ·OH, the diffusion of active species played a promoting role for Cu–EDTA removal (Rommozzi et al., 2020; Mandal et al., 2020). Cl− involved in the hydrogen evolution reaction in the anode region to form Cl2 and underwent further hydrolysis to form highly oxidized ClO− promoting the removal of Cu–EDTA (Eqs 11–13). NO3− and HPO42− would have a competitive electronic effect with Cu–EDTA, and NO3− and HPO42− were also able to scavenge a certain ·OH, which in turn generated more reactive NO3·and H2PO4·leading to a decrease in Cu–EDTA removal effect (Eqs 14, 15) (Cheng et al., 2017; Teng et al., 2021). Therefore, NO3− and HPO42− would inhibit Cu–EDTA removal in the 3D-EF reaction system.
Conclusion
In this study, the 3D-EF reaction system using GAC particles as particle electrodes could effectively achieve the goal of Cu–EDTA decomplexation. The performance of Cu–EDTA decomplexation by 3D-EF was better than that by the GAC and EF with a decomplexation efficiency of 90.95%, which broke the pH limit of the traditional EF reaction system and widened the application window of pH. Cu–EDTA was optimally treated at an initial pH of 7, 1 mmol L−1 Fe2+, 4 g L−1 GAC, and current density of 10 mA cm−2. In addition, Cl− promoted the removal of Cu–EDTA in the 3D-EF reaction system; however, NO3− and HPO42− inhibited Cu–EDTA removal.
Data Availability Statement
The original contributions presented in the study are included in the article/supplementary material, further inquiries can be directed to the corresponding authors.
Author Contributions
YM contributed to data curation, methodology, writing–original draft, and writing–review and editing; XH designed all experiments and revised and examined the manuscript; JY contributed to review and editing; FY contributed to data curation and investigation; and JZ interpreted the data and resources. All authors read and approved the final manuscript.
Funding
This work was supported by a project grant from the National Natural Science Foundation of China (421078064), Urban Smart Water Pollution Prevention and Control Technology Development Center, Project Source: Department of Education of Guangdong Province (2019GGCZX007), Project of Shenzhen Science and Technology Plan (KJYY20180206180737010), University-Level Supporting Projects by Shenzhen Polytechnic (6020320003K), Startup Foundation for Introducing Talent of NUIST, and Natural Science Fund Project of Colleges in Jiangsu Province (20KJB610010).
Conflict of Interest
The authors declare that the research was conducted in the absence of any commercial or financial relationships that could be construed as a potential conflict of interest.
Publisher’s Note
All claims expressed in this article are solely those of the authors and do not necessarily represent those of their affiliated organizations, or those of the publisher, the editors, and the reviewers. Any product that may be evaluated in this article, or claim that may be made by its manufacturer, is not guaranteed or endorsed by the publisher.
References
Barhoumi, N., Oturan, N., Olvera-Vargas, H., Brillas, E., Gadri, A., Ammar, S., et al. (2016). Pyrite as a Sustainable Catalyst in Electro-Fenton Process for Improving Oxidation of Sulfamethazine. Kinetics, Mechanism and Toxicity Assessment. Water Res. 94, 52–61. doi:10.1016/j.watres.2016.02.042
Chen, H., and Wang, J. (2021). Degradation and Mineralization of Ofloxacin by Ozonation and Peroxone (O3/H2O2) Process. Chemosphere 269, 128775. doi:10.1016/j.chemosphere.2020.128775
Cheng, T.-H., Huang, C.-P., Huang, Y.-H., and Shih, Y.-J. (2017). Kinetic Study and Optimization of Electro-Fenton Process for Dissolution and Mineralization of Ion Exchange Resins. Chem. Eng. J. 308, 954–962. doi:10.1016/j.cej.2016.09.142
Chu, Y., Su, H., Liu, C., and Zheng, X. (2022). Fabrication of sandwich-like Super-hydrophobic Cathode for the Electro-Fenton Degradation of Cefepime: H2O2 Electro-Generation, Degradation Performance, Pathway and Biodegradability Improvement. Chemosphere 286, 131669. doi:10.1016/j.chemosphere.2021.131669
Chu, Y., Su, H., Lv, R., and Zhang, X. (2021). Enhanced Electro-Reduction of Fe3+ to Fe2+ by Acidified Carbon Nanotube-Modified Graphite Cathode and its Application in a Novel Fenton Process for P-Nitrophenol Degradation. J. Water Process Eng. 40, 101912. doi:10.1016/j.jwpe.2020.101912
Dargahi, A., Hasani, K., Mokhtari, S. A., Vosoughi, M., Moradi, M., and Vaziri, Y. (2021). Highly Effective Degradation of 2,4-Dichlorophenoxyacetic Acid Herbicide in a Three-Dimensional Sono-Electro-Fenton (3D/SEF) System Using Powder Activated Carbon (PAC)/Fe3O4 as Magnetic Particle Electrode. J. Environ. Chem. Eng. 9 (5), 105889. doi:10.1016/j.jece.2021.105889
Duan, X., Sun, H., Shao, Z., and Wang, S. (2018). Nonradical Reactions in Environmental Remediation Processes: Uncertainty and Challenges. Appl. Catal. B: Environ. 224, 973–982. doi:10.1016/j.apcatb.2017.11.051
Feng, C.-H., Li, F.-B., Mai, H.-J., and Li, X.-Z. (2010). Bio-electro-Fenton Process Driven by Microbial Fuel Cell for Wastewater Treatment. Environ. Sci. Technol. 44, 1875–1880. doi:10.1021/es9032925
Ghanbarlou, H., Nasernejad, B., Nikbakht Fini, M., Simonsen, M. E., and Muff, J. (2020). Synthesis of an Iron-Graphene Based Particle Electrode for Pesticide Removal in Three-Dimensional Heterogeneous Electro-Fenton Water Treatment System. Chem. Eng. J. 395, 125025. doi:10.1016/j.cej.2020.125025
Ghasemi, M., Khataee, A., Gholami, P., Soltani, R. D. C., Hassani, A., and Orooji, Y. (2020). In-situ Electro-Generation and Activation of Hydrogen Peroxide Using a CuFeNLDH-CNTs Modified Graphite Cathode for Degradation of Cefazolin. J. Environ. Manage. 267, 110629. doi:10.1016/j.jenvman.2020.110629
Guan, W., Zhang, B., Tian, S., and Zhao, X. (2018). The Synergism between Electro-Fenton and Electrocoagulation Process to Remove Cu-EDTA. Appl. Catal. B: Environ. 227, 252–257. doi:10.1016/j.apcatb.2017.12.036
Guan, Z., Guo, Y., Huang, Z., Liao, X., Chen, S., Ou, X., et al. (2022). Simultaneous and Efficient Removal of Organic Ni and Cu Complexes from Electroless Plating Effluent Using Integrated Catalytic Ozonation and Chelating Precipitation Process in a Continuous Pilot-Scale System. Chem. Eng. J. 428, 131250. doi:10.1016/j.cej.2021.131250
He, S., Li, T., Zhang, L., Zhang, X., Liu, Z., Zhang, Y., et al. (2021). Highly Effective Photocatalytic Decomplexation of Cu-EDTA by MIL-53(Fe): Highlight the Important Roles of Fe. Chem. Eng. J. 424, 130515. doi:10.1016/j.cej.2021.130515
Hu, X., Yang, S., You, X., Zhang, W., Liu, Y., and Liang, W. (2022). Electrocatalytic Decomplexation of Cu-EDTA and Simultaneous Recovery of Cu with Ni/GO-PAC Particle Electrode. Chem. Eng. J. 428, 131468. doi:10.1016/j.cej.2021.131468
Huang, A., Zhi, D., Tang, H., Jiang, L., Luo, S., and Zhou, Y. (2020). Effect of Fe2+, Mn2+ Catalysts on the Performance of Electro-Fenton Degradation of Antibiotic Ciprofloxacin, and Expanding the Utilizing of Acid Mine Drainage. Sci. Total Environ. 720, 137560. doi:10.1016/j.scitotenv.2020.137560
Le, T. X. H., Bechelany, M., and Cretin, M. (2017). Carbon Felt Based-Electrodes for Energy and Environmental Applications: A Review. Carbon 122, 564–591. doi:10.1016/j.carbon.2017.06.078
Li, H., Yang, H., Cheng, J., Hu, C., Yang, Z., and Wu, C. (2021). Three-dimensional Particle Electrode System Treatment of Organic Wastewater: A General Review Based on Patents. J. Clean. Prod. 308, 127324. doi:10.1016/j.jclepro.2021.127324
Li, M., Qin, X., Cui, J., Guo, R., Guo, C., Wang, Z., et al. (2021). Three-dimensional Electro-Fenton Degradation for Fulvic Acids with Cu-Fe Bimetallic Aerogel-like Carbon as Particle Electrode and Catalyst: Electrode Preparation, Operation Parameter Optimization and Mechanism. J. Environ. Chem. Eng. 9, 105573. doi:10.1016/j.jece.2021.105573
Liu, Y., Wang, D., Xue, M., Song, R., Zhang, Y., Qu, G., et al. (2021). High-efficient Decomplexation of Cu-EDTA and Cu Removal by High-Frequency Non-thermal Plasma Oxidation/alkaline Precipitation. Sep. Purif. Tech. 257, 117885. doi:10.1016/j.seppur.2020.117885
Long, Y., Feng, Y., Li, X., Suo, N., Chen, H., Wang, Z., et al. (2019). Removal of Diclofenac by Three-Dimensional electro-Fenton-persulfate (3D electro-Fenton-PS). Chemosphere 219, 1024–1031. doi:10.1016/j.chemosphere.2018.12.054
Ma, B., Lv, W., Li, J., Yang, C., Tang, Q., and Wang, D. (2021). Promotion Removal of Aniline with Electro-Fenton Processes Utilizing Carbon Nanotube 3D Morphology Modification of an Ag-Loaded Copper Foam Cathode. J. Water Process Eng. 43, 102295. doi:10.1016/j.jwpe.2021.102295
Mandal, P., Gupta, A. K., and Dubey, B. K. (2020). Role of Inorganic Anions on the Performance of Landfill Leachate Treatment by Electrochemical Oxidation Using graphite/PbO2 Electrode. J. Water Process Eng. 33, 101119. doi:10.1016/j.jwpe.2019.101119
Midassi, S., Bedoui, A., and Bensalah, N. (2020). Efficient Degradation of Chloroquine Drug by Electro-Fenton Oxidation: Effects of Operating Conditions and Degradation Mechanism. Chemosphere 260, 127558. doi:10.1016/j.chemosphere.2020.127558
Moreira, J., Bocalon Lima, V., Athie Goulart, L., and Lanza, M. R. V. (2019). Electrosynthesis of Hydrogen Peroxide Using Modified Gas Diffusion Electrodes (MGDE) for Environmental Applications: Quinones and Azo Compounds Employed as Redox Modifiers. Appl. Catal. B: Environ. 248, 95–107. doi:10.1016/j.apcatb.2019.01.071
Ou, B., Wang, J., Wu, Y., Zhao, S., and Wang, Z. (2019). A Highly Efficient Cathode Based on Modified Graphite Felt for Aniline Degradation by Electro-Fenton. Chemosphere 235, 49–57. doi:10.1016/j.chemosphere.2019.06.144
Pan, Z., Yu, F., Li, L., Liu, M., Song, C., Yang, J., et al. (2020). Low-cost Electrochemical Filtration Carbon Membrane Prepared from Coal via Self-Bonding. Chem. Eng. J. 385, 123928. doi:10.1016/j.cej.2019.123928
Rommozzi, E., Giannakis, S., Giovannetti, R., Vione, D., and Pulgarin, C. (2020). Detrimental vs. Beneficial Influence of Ions during Solar (SODIS) and Photo-Fenton Disinfection of E. coli in Water: (Bi)carbonate, Chloride, Nitrate and Nitrite Effects. Appl. Catal. B: Environ. 270, 118877. doi:10.1016/j.apcatb.2020.118877
Shah, B. R., and Patel, U. D. (2021). Mechanistic Aspects of Photocatalytic Degradation of Lindane by TiO2 in the Presence of Oxalic Acid and EDTA as Hole-Scavengers. J. Environ. Chem. Eng. 9, 105458. doi:10.1016/j.jece.2021.105458
Shi, K., Wang, Y., Xu, A., Zhou, X., Zhu, H., Wei, K., et al. (2021). Efficient Degradation of Ibuprofen by Electro-Fenton with Microtubular Gas- Diffusion Electrodes Synthesized by Wet-Spinning Method. J. Electroanalytical Chem. 897, 115615. doi:10.1016/j.jelechem.2021.115615
Song, D., Li, J., Wang, Z., and Zhao, C. (2020). Performance of Graphite Felt as Anodes in the Electro-Fenton Oxidation Systems: Changes in Catalysis, Conductivity and Adsorption Properties. Appl. Surf. Sci. 532, 147450. doi:10.1016/j.apsusc.2020.147450
Song, P., Sun, C., Wang, J., Ai, S., Dong, S., Sun, J., et al. (2022). Efficient Removal of Cu-EDTA Complexes from Wastewater by Combined Electrooxidation and Electrocoagulation Process: Performance and Mechanism Study. Chemosphere 287, 131971. doi:10.1016/j.chemosphere.2021.131971
Tasić, Ž. Z., Petrović Mihajlović, M. B., Simonović, A. T., Radovanović, M. B., and Antonijević, M. M. (2021). Review of Applied Surface Modifications of Pencil Graphite Electrodes for Paracetamol Sensing. Results Phys. 22, 103911. doi:10.1016/j.rinp.2021.103911
Teng, X., Li, J., Wang, J., Liu, J., Ge, X., and Gu, T. (2021). Effective Degradation of Atrazine in Wastewater by Three-Dimensional Electrochemical System Using Fly Ash-Red Mud Particle Electrode: Mechanism and Pathway. Sep. Purif. Tech. 267, 118661. doi:10.1016/j.seppur.2021.118661
Wang, H., Zhao, Z., Zhang, X., Dong, W., Cao, Z., He, L., et al. (2020). Rapid Decomplexation of Ni-EDTA by Microwave-Assisted Fenton Reaction. Chem. Eng. J. 381, 122703. doi:10.1016/j.cej.2019.122703
Wang, J., and Chen, X. (2021). Removal of Antibiotic Resistance Genes (ARGs) in Various Wastewater Treatment Processes: An Overview. Crit. Rev. Environ. Sci. Tech. 1, 1–60. doi:10.1080/10643389.2020.1835124
Wang, J., and Tang, J. (2021). Fe-based Fenton-like Catalysts for Water Treatment: Preparation, Characterization and Modification. Chemosphere 276, 130177. doi:10.1016/j.chemosphere.2021.130177
Wang, J., and Wang, S. (2018). Activation of Persulfate (PS) and Peroxymonosulfate (PMS) and Application for the Degradation of Emerging Contaminants. Chem. Eng. J. 334, 1502–1517. doi:10.1016/j.cej.2017.11.059
Wang, J., and Wang, S. (2021). Effect of Inorganic Anions on the Performance of Advanced Oxidation Processes for Degradation of Organic Contaminants. Chem. Eng. J. 411, 128392. doi:10.1016/j.cej.2020.128392
Wang, J., and Zhuan, R. (2020). Degradation of Antibiotics by Advanced Oxidation Processes: An Overview. Sci. Total Environ. 701, 135023. doi:10.1016/j.scitotenv.2019.135023
Wang, Q., Yu, J., Chen, X., Du, D., Wu, R., Qu, G., et al. (2019). Non-thermal Plasma Oxidation of Cu(II)-EDTA and Simultaneous Cu(II) Elimination by Chemical Precipitation. J. Environ. Manage. 248, 109237. doi:10.1016/j.jenvman.2019.07.008
Wang, Y., Liu, Y., Wu, B., Rui, M., Liu, J., and Lu, G. (2020). Comparison of Toxicity Induced by EDTA-Cu after UV/H2O2 and UV/persulfate Treatment: Species-specific and Technology-dependent Toxicity. Chemosphere 240, 124942. doi:10.1016/j.chemosphere.2019.124942
Wang, Z., Song, B., Li, J., and Teng, X. (2021). Degradation of Norfloxacin Wastewater Using Kaolin/steel Slag Particle Electrodes: Performance, Mechanism and Pathway. Chemosphere 270, 128652. doi:10.1016/j.chemosphere.2020.128652
Xia, H., Li, C., Yang, G., Shi, Z., Jin, C., He, W., et al. (2022). A Review of Microwave-Assisted Advanced Oxidation Processes for Wastewater Treatment. Chemosphere 287, 131981. doi:10.1016/j.chemosphere.2021.131981
Xie, S., Shao, W., Zhan, H., Wang, Z., Ge, C., Li, Q., et al. (2020). Cu(II)-EDTA Removal by a Two-step Fe(0) Electrocoagulation in Near Natural Water: Sequent Transformation and Oxidation of EDTA Complexes. J. Hazard. Mater. 392, 122473. doi:10.1016/j.jhazmat.2020.122473
Yao, B., Luo, Z., Yang, J., Zhi, D., and Zhou, Y. (2021). FeIIFeIII Layered Double Hydroxide Modified Carbon Felt Cathode for Removal of Ciprofloxacin in Electro-Fenton Process. Environ. Res. 197, 111144. doi:10.1016/j.envres.2021.111144
Zhang, B., Hou, Y., Yu, Z., Liu, Y., Huang, J., Qian, L., et al. (2019). Three-dimensional Electro-Fenton Degradation of Rhodamine B with Efficient Fe-Cu/kaolin Particle Electrodes: Electrodes Optimization, Kinetics, Influencing Factors and Mechanism. Sep. Purif. Tech. 210, 60–68. doi:10.1016/j.seppur.2018.07.084
Zhang, J., Qiu, S., Feng, H., Hu, T., Wu, Y., Luo, T., et al. (2022). Efficient Degradation of Tetracycline Using Core-Shell Fe@Fe2O3-CeO2 Composite as Novel Heterogeneous Electro-Fenton Catalyst. Chem. Eng. J. 428, 131403. doi:10.1016/j.cej.2021.131403
Zhang, M.-h., Dong, H., Zhao, L., Wang, D.-x., and Meng, D. (2019). A Review on Fenton Process for Organic Wastewater Treatment Based on Optimization Perspective. Sci. Total Environ. 670, 110–121. doi:10.1016/j.scitotenv.2019.03.180
Zhang, Y., Chen, Z., Wu, P., Duan, Y., Zhou, L., Lai, Y., et al. (2020). Three-dimensional Heterogeneous Electro-Fenton System with a Novel Catalytic Particle Electrode for Bisphenol A Removal. J. Hazard. Mater. 393, 120448. doi:10.1016/j.jhazmat.2019.03.067
Zhao, K., Su, Y., Quan, X., Liu, Y., Chen, S., and Yu, H. (2018). Enhanced H2O2 Production by Selective Electrochemical Reduction of O2 on Fluorine-Doped Hierarchically Porous Carbon. J. Catal. 357, 118–126. doi:10.1016/j.jcat.2017.11.008
Zhao, Z., Dong, W., Wang, H., Chen, G., Tang, J., and Wu, Y. (2018b). Simultaneous Decomplexation in Blended Cu(II)/Ni(II)-EDTA Systems by Electro-Fenton Process Using Iron Sacrificing Electrodes. J. Hazard. Mater. 350, 128–135. doi:10.1016/j.jhazmat.2018.02.025
Zhao, Z., Liu, Z., Wang, H., Dong, W., and Wang, W. (2018a). Sequential Application of Fenton and Ozone-Based Oxidation Process for the Abatement of Ni-EDTA Containing Nickel Plating Effluents. Chemosphere 202, 238–245. doi:10.1016/j.chemosphere.2018.03.090
Zheng, T., Wang, Q., Shi, Z., Fang, Y., Shi, S., Wang, J., et al. (2016). Advanced Treatment of Wet-Spun Acrylic Fiber Manufacturing Wastewater Using Three-Dimensional Electrochemical Oxidation. J. Environ. Sci. 50, 21–31. doi:10.1016/j.jes.2016.03.020
Keywords: Cu-EDTA, three-dimensional electro-Fenton, decomplexation, parameter optimization, electrochemical
Citation: Ma Y, Huang X, Han Q, Yu J, Yu F and Zhu J (2022) Decomplexation Performance of Cu–EDTA and Parameter Optimization by Three-Dimensional Electro-Fenton. Front. Environ. Sci. 10:818142. doi: 10.3389/fenvs.2022.818142
Received: 19 November 2021; Accepted: 04 January 2022;
Published: 25 January 2022.
Edited by:
Shihai Deng, Xi’an Jiaotong University, ChinaReviewed by:
Zacharias Frontistis, University of Western Macedonia, GreeceZhirong Sun, Beijing University of Technology, China
Copyright © 2022 Ma, Huang, Han, Yu, Yu and Zhu. This is an open-access article distributed under the terms of the Creative Commons Attribution License (CC BY). The use, distribution or reproduction in other forums is permitted, provided the original author(s) and the copyright owner(s) are credited and that the original publication in this journal is cited, in accordance with accepted academic practice. No use, distribution or reproduction is permitted which does not comply with these terms.
*Correspondence: Xiao Huang, huangxiao901231@126.com; Jia Zhu, zhujia@szpt.edu.cn
†These authors have contributed equally to this work and share first authorship