- 1Institute for Plant Nutrition and Soil Science, Christian-Albrechts-University Kiel, Kiel, Germany
- 2Leibniz Centre for Agricultural Landscape Research (ZALF) e. V., Müncheberg, Germany
- 3Department Soil System Science Helmholtz Centre for Environmental Research—UFZ, Halle, Germany
The mechanical strength of agricultural soils depends on many soil properties and functions. The database, “soil strength and consequences for sustainable land use and soil management SOILMECHDAT-Kiel”, originates from the “Horn Research Group” includes analyses of undisturbed soil samples taken from more than 460 profiles in and is developed in collaboration with BONARES, a funding initiative of the German Federal Ministry for Education and Research that focuses on the sustainable use of soils. For over 40 years, over 42 different authors recorded 59 physical and 29 chemical parameters for complete soil profiles. In order to the aim of the initial analyses of this data is to determine the influence of bulk density (BD) organic matter (OM) and time (year) on precompression stress (Pc) and saturated hydraulic conductivity (ks) as a function of Pc. Three main textural groups sand, loam, and silt for both topsoils and subsoils (SS) were studied. In loamy and silty subsoils BD and OM are not related to Pc (R2 = 0.17 and R2 = 0.25). OM and bulk density are more related to Pc in sandy soils (R2 0.55–0.59). The link between ks and Pc showed that sandy soils have a significantly higher Pc (>150 kPa) and conductivities did not change much. In loamy soils, with a Pc > 90 kPa, 50% of the ks fell below the critical value of 10 cm d−1. For silty soils, at a Pc of 60 kPa, 50% of the data fall below the critical value of ks. These findings suggest that the stability of loamy and silty soils not only depends on OM and BD, but requires further data to explain the variation in the measurements. With respect to ks, the results show that fertile silty soils are more sensitive than formerly defined.
Introduction
Soil compaction, especially subsoil compaction, is one of the major threats of soil degradation globally (Bridges and Oldeman, 1999). The European Soil Framework Directive (2006) stated that soil compaction, along with erosion by water and wind, is one of the main physical processes that severely threatens soil and causing degradation. It is estimated that 32% of the subsoils (SS) in Europe are highly degraded and 18% are moderately vulnerable to compaction and soil degradation, caused by too heavy agricultural and forestry machinery. The machinery is responsible for the degradation of an area of 330,000 km2 (Soane and van Ouwerkerk, 1995; Fraters, 1996), and the affected area increases continuously (Keller et al., 2017, 2019).
The main reasons for the increase in compaction, arable or forest soils are the ever-increasing weight of the machines and the increased frequency of wheeling under unfavorable (high moisture) soil conditions. This statement is repeatedly mentioned by Horn (1983), Håkansson et al. (1987), Lebert (1989), Alakukku (1996), Kühner (1997), Wiermann et al. (2000), Arvidsson et al. (2001), Horn and Fleige (2003); Horn and Fleige, (2009), Keller et al. (2004), Horn and Smucker, 2005, Zink et al. (2010), Riggert (2015), Keller et al. (2019), and Horn (2021). But the consequences are most often underestimated although well defined (Arvidsson and Hakansson, 1996; Zink et al., 2010). Although many farmers do care on these threats, visible and measurable effects (e.g., yield decline or increased probability of flooding even during the growing season) have often resulted in minor changes in land management. However, soil degradation occurs generally in all soils, even if the intensity and the consequences differ depending on the internal soil properties as well as the kind and frequency of stress application. The latter has increased over the last decades (Arvidsson and Hakansson, 1996; Zink, 2009; Duttmann et al., 2014; Hartge and Horn, 2016; Horn et al., 2017; Keller et al., 2019). The consequences of land management and tillage, needs to be analyzed, because saturated hydraulic conductivity (ks) also depends on shear- and vibration-induced soil deformation interactions. These interactions enhance the degradation of soil properties, especially if the soil water content is high and the internal soil strength is low (Huang et al., 2021a, b). Furthermore, intense animal trampling often in combination with induced overgrazing of moist to wet pastures, causes soil compression and leads to a reduction of coarse pores with smaller continuity. Even if soil horizons are still structured after stress application, the trampling will lead to a compacted platy structure in the topsoil and changes down to deep depths (Fazekas and Horn, 2005).
The reduction of pore space and especially of macropores, along with the conversion of three-dimensionally uniform pore systems to completely horizontal anisotropic conditions in platy structured soil horizons by compaction must be regarded as harmful. Thus, compaction may cause severe consequences for hydraulic, gas, and heat transport, as well as for nutrient storage and uptake by plants, if the stresses exceed the internal soil strength (Horn et al., 2019). By 2050, food production must increase by + 70% to nourish the nine billion people on earth (Horn and Blum, 2020). In addition, climate change will affect precipitation frequency and intensity and the temperature. Consequently, soil structure changes need to be studied to gain insight into basic soil physical and mechanical properties and how they change over time. These interactions are seldom published for whole soil profiles but for only single soil horizons as well as their time dependent changes.
The “Horn Research Group” has studied for over 40 years the topic of soil strength for soil profiles with different geological substrates, climatic boundary conditions, and different land uses and the relation to sustainable land use and soil management. The compiled a database: SOILMECHDAT-Kiel, which includes these physical and mechanical properties. With coordinates available, this database can be used to develop, at various scales (farm level to country to continent), soil maps showing strength or stress distribution based on wheeling experiments and consecutive analyses of stress distribution patterns at given mechanical soil properties.
The precompression stress (= internal soil strength, Pc) is a sensitive and scale-spanning parameter that defines the rigidity of the soil structure. It indicates the current state of compaction, as a result of all previous physical, chemical, or biological compressive and stabilizing processes as well as natural decompression (loosening such as bioturbation). It is derived from stress-strain curves and is the transition from the recompression to the virgin compression range. It depends on the matric potential, as well as former pedo- and anthropogenic processes (Mordhorst et al., 2020). The higher the soil strength, the lower is the likelihood for additional mechanical stress and long-term degradation of soil structure (Horn and Dexter 1989; Horn et al., 1991a; Kirby 1991b; Van den Akker et al., 1999; Trautner et al., 2003; Horn and Fleige, 2009; Keller et al., 2019). The values for Pc also document the range of resilience (recompression range) while in the virgin compression stress range it declines due to plastic deformation (Horn and Blum 2020).
The Pc value is often related to soil conditions in early spring at matric potential values of pF 1.8 (−60 hPa) or when the soil is drying due to evapotranspiration and the soil water content is reduced to values such as pF 2.5 (−300 hPa) matric potential. The drier the soil is, the more negative is the pore water pressure and the soil structure solidifies. Relationships between matric potential and internal soil strength can be described by the effective stress equation (Bishop 1959) which enable to quantify the effective stresses between particles or aggregates and the stabilizing or weakening effects of the pore water pressure. Besides the soil strength change with matric potential can also the impact of the chi factor at given total stresses for the effective stresses be quantified. Besides landuse and soil management can also geogenic impacts for given soil types be quantified in order to classify mechanical properties of soils from farm to country or continental scale or with respect to tentative effects for given land use managements. In order to optimize the analysis purpose, the following properties that affect soil strength are included in the database: texture, structure, clay mineralogy, organic matter (OM), bulk density (BD), chemical composition, and negative pore water pressure (= matric potential) (for more details see also Larson et al., 1980; Horn, 1981; McBride, 1989; Soane,1990; O'Sullivan, 1992; Watts and Dexter, 1997; Zhang et al., 1997; Paz and Guérif, 2000; Keller et al., 2011; Baumgartl and Horn, 2013).
Shear strength, determines the binding forces between particles (texture) or the ability of soil aggregates to withstand the rearrangement (= strain) against smearing (also defined as slip). The shear parameters defined by the Mohr Coulomb equation are the angle of internal friction and cohesion, and they define the slope and the intercept of the linear regression equation between shear resistance as dependent variable and normal stress for a given matric potential, of a structured soil horizon.
Soil physical properties affected by soil deformation are the ks and air permeability (kl), which show a dependency on the internal soil strength. Both the ks and unsaturated hydraulic conductivity (ku) as well as kl as a function of matric potential represent the functional quality of soil structure and pore continuity as they define the air and water fluxes within the soil. (Arthur et al., 2012; Berisso et al., 2012). but the rigidity of the structured pore system is limited to the recompression stress range while within the virgin compression stress range decrease these values intensely and cause a decrease of continuity of the pore network (Hamamoto et al., 2011; Horn 2021). The same would be in addition true for gas diffusivity, which, however, was not always analysed during the research activities. If pores lose their continuity (blocked pores) and diameter fluxes are reduced and in the worst case also the liquefaction increase with more intense impacts or an increased rigidity change (Huang et al., 2021a). Blocked pores are affected by soil management including shearing (Dörner and Horn 2006) and can be related to soil deformation processes if the history as well as the registered management of the analysed soils are regarded.
Organic matter (OM) does not only increase the water storage in soils, but also the hydraulic or pneumatic functions which helps to define structure properties (Piccolo et al. (1997); IPCC, 2003; Janssens et al., 2005; Jones et al., 2005; Smith et al., 2005; Stolbovoy et al., 2007; Saha et al., 2011). Feller and Beare (1997) substantiated the physical interactions between clay particles and OM and showed that, with increasing humus content, the structural stability of the soil improved. Specifically, the clay particles and OM should be in proportion to each other to form organic carbon complexes (Dexter et al., 2008; Horn and Blum, 2020).
The objectives of this first paper are the following:
• In cooperation with BONARES, a funding initiative of the German Federal Ministry for Education and Research, we want to create a database to document and statistically evaluate the soil strength of structured and pre-drained soils under agricultural use.
• To determine the link between the Pc and OM, BD and ks for the three soil classes (sand, loam, and silt) and time depending changes separated in top- and subsoils.
• The aim of this project is to elaborate scenarios how to at least maintain for the present soil structural properties as a sustainable soil management, according to an initiative of the German Federal Ministry of Education and Research (BMBF).
Materials and Methods
The origin database is composed of three Excel tables (not shown in this paper), which inform about the soil profiles that were studied using the three German Soil Mapping Instructions (AG Bodenkunde, 1982; AG Boden, 1994; Ad-hoc-AG Boden, 2005) over the last 40 years. Each table is divided into metadata and physical/chemical parameters. The measured values are assigned to their respective parameter sheets. These include parameters of “Chemical properties”, “Water retention”, “Saturated hydraulic conductivity”, “Air conductivity”, “Pc” and “Shear resistance”. The complete description of the database will be part of the finalizing PhD thesis while at present some general information about parameters needed to detect and to quantify soil properties and functions will be given.
Origin Data
Mechanical data originate from master’s and PhD publications and other projects from the Institute of Soil Science of CAU in Kiel, Germany. More than 70% of the soil samples analysed originate from Germany (Figure 1) and will be completely available for further analyses within the BONARES database following the finished PhD Thesis in 2022.of R. Schroeder.
Further sampling took place in Switzerland, Estonia, Norway, Finland, Great Britain, Brazil, Chile, and China. The database includes up to 59 physical/mechanical and 29 chemical parameters and consider amongst others texture, structure, BD, OM pore size distribution, soil strength parameters and hydraulic as well as pneumatic functions as well as pH etc. The analyses recorded are from a period between 1979 and 2019, with over 460 profiles, 588 Topsoil-horizons (TS), 974 Subsoil-horizons (SS), and 225 underground horizons quantified and available for statistical analysis. TS horizons are defined as all mineral horizons with the main symbol “A” and with their TS horizon limit between 0 and 30 cm. The SS horizons are identified according to the German classification system: B, P, T, S, G, M, E, R, and Y with an upper horizon limit of >0 cm und mineral surface. Subsurface mineral horizons (not/less weathered) (n = 225) are defined by the symbol “C”/“-C” as parent material. The variable number of the three horizon groups is due to different sampling depths and sampling strategies. The SS horizons include also those with OM > 1%, which result from former deeper ploughing strategies (35—40 cm) from early 1970th/80th. Because the later ploughing was limited to 25—30 cm, these deeper parts of A-horizons are defined as “buried” and SS horizons.
Documentation of Soil Use
The database allows a categorization of the investigated areas with respect to their use, properties, and functions. Different types of land use were included and categorized amongst others as “peatland”, “grassland”, “forest”, and “arable land".
The peatland area share (4.3%) includes areas that are difficult or impossible to use and are not under agricultural use. This proportion includes bog areas (Histosols) with more than 30 cm organic cover (>30% OM) and “subhydric areas”. Bog areas also include fallow areas (share: < 1%). The data share for grassland is 28% and includes (mowed) pastures, all types of grasslands, parks, and cultivated moors. “Forest” with 10% includes woodlands, as well as mixed coniferous and deciduous forests. 62% of the profiles are under conventional (86%) or conservation (14%) agricultural use. Conventionally farmed areas are characterized by regular ploughing (p) operations of the TS (Ap-horizon), with tillage depth varying between 25—30 cm. Areas with conservation tillage are not ploughed or have minor or no tillage.
Database Structure and Processing
For each soil mapping manual: AG Bodenkunde (1982) AG Boden (1994) and Ad-hoc-AG Boden (2005) an Excel spreadsheet (Excel 2016) was created. The spreadsheets for each Excel spreadsheet are divided into the sections: profile, horizon, chemical properties, water retention, hydraulic conductivity, precompression stress and shearing (Table 1). This allows a systematic transfer of the profile data from the publications and other sources into the database. The entries are made by means of free text or a predefined drop-down menu. The parameters of the profile and horizon listed represent elementary basic information of the sample sites, which are considered as a minimum requirement for inclusion in the database. Table 1 give an overview about the main sections of the construction of the data basis and the given parameter.
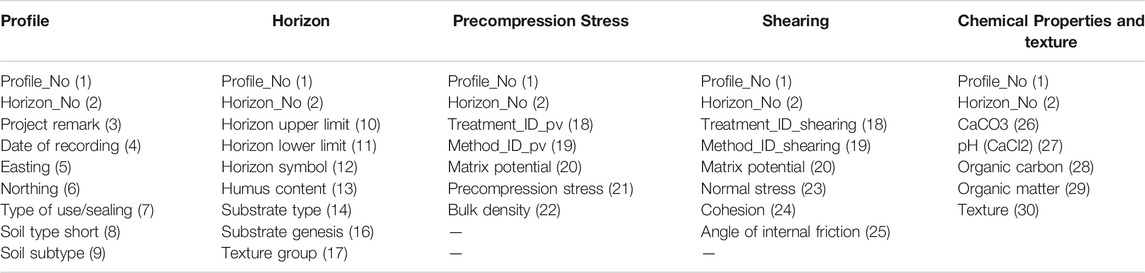
TABLE 1. Structure of each Excel spreadsheet of the soil data base with the main sections: Profile, Horizon, precompression stress (Pc), shearing, chemical properties and texture with several given parameters (0–30).
Section Profile
The profile number 1) is generated individually for each profile in the database. It is used as a reference for the chemical and physical parameters, and it ensures an exact assignment of the laboratory analyses to the respective profile metadata. It is composed of the four initial letters of the author and the number of the profile as a digit.
In case of further publications by an author, further consecutive profile numbers are added. For further classification, the project name (3). 3—17 are directly oriented to the title data of the respective mapping manual (AG Bodenkunde, 1982; AG Boden, 1994; Ad-hoc-AG Boden, 2005) and are further defined there.
Section Horizon
In the “Section Horizon”, the profile number 1) already described is placed in front of the soil parameters as a profile reference. Together with the horizon number 2) as horizon reference, they form the link between the profile reference and the measurement results. The information on points one0–17 is also defined according to the respective soil mapping instructions and further described there.
Section Precompression Stress
Methodologies for the physical and chemical soil parameters are noted in the appendix of the database and assigned to the corresponding parameters as numerical codes. Throughout the entire period, the physical and chemical analyses of the disturbed and undisturbed soil samples were performed with identical methods according to Schlichting et al. (1966), Schlichting et al. (1995), Hartge (1978), Hartge and Horn (1992, 2009,2016), and Blume et al. (2011). detailed information on the measurements can be found in the respective publications.
All strength data in the database is determined for undisturbed cylinder specimens (236 cm³) by means of uniaxial compression tests (oedometer), and they were calculated using the method of Casagrande (1936). Further information can be found in Hartge and Horn (2009, 2016), Scheffer and Schachtschabel (2018) and Horn (1980). For an adequate evaluation of the Pc values, additional data are necessary. The treatment_ID_pv 18) and the method_ID_pv 19) refer by numerical code to treatment measures, which were carried out during the test. Each Pc, BD and OM value 21) is classified as defined in DVWK (1997), Horn and Fleige (2003); Horn and Fleige (2009) and Ad-Hoc AG Boden 2005 (Table 2). It is also often stated that BD may have an influence on soil strength. Thus, it is used as a guiding parameter for the derivation of the Pc.

TABLE 2. Classification of the precompression stress (Pc), bulk density ρ and organic matter (OM) according to DVWK 1997, Horn/Fleige 2003 and Ad-Hoc AG Boden 2005.
Table 3 shows the limits for Pc of the main textural groups (MTG) according to DVWK (1997) and Horn and Fleige (2009).

TABLE 3. Critical values to verify harmful subsoil compaction by precompression stress (Pc) (low indication) and saturated hydraulic conductivity (ks) (high indication) for different texture groups according to (Horn and Fleige 2009) and critical ks value according to UBA, 2004a.
Section Shearing
Prediction of the Pc (= internal soil strength) and the parameters of the shear resistance need to be determined. To get values of shear resistance, the Mohr Coulomb equation (Eq. (1)) was used:
where c as the cohesion (kPa), φ as the angle of internal friction (°) and σn as vertical compression stress (kPa) results in τ as shear resistance (kPa).
The effects of soil structure, texture, OM, and water content are linked and can be applied to explain soil strength and changes in soil functions (Zhang et al., 1997; Huang et al., 2021a, b). The structure of this section follows the pattern of those described earlier. Matric potential determined pre-drainage (20) affects items 24 and 25, which relate to the shear resistance.
Section Chemical Properties and Texture
Due to the heterogeneous objectives of the publications included, the data base is diverse. The OM is calculated by a factor of 1.72 from organic carbon. All other chemical analyses in the database, as well as calculations, are based on identical methods described in Schlichting et al. (1966), Schlichting et al. (1995), and Blume et al. (2011). The classification of OM follows the recommendations of AG Bodenkunde, 1982, AG Boden, 1994, Ad-hoc-AG Boden, 2005 (Table 2).
Data Selection and Preparation of Analysis
In this first paper, we have chosen to document Pc as a function of the OM and the BD for various texture classes. The chemical data OM, texture as well as BD are generally available for almost all profiles. The classification into MTG, according to Ad-hoc-AG Boden, 2005, is intended to show the influence of texture, and the differences in susceptibility to over-compaction and the resulting ks (for more details: Larson et al., 1980; Horn, 1981; Hettiaratchi, 1987; Batey and McKenzie, 2006). Furthermore, the influence of OM on soil strength will be dealt with in this paper as it is often mentioned by Feller and Beare, (1997); Alekseeva and Alekseev, (1999); Alakukku et al. (2003). Furthermore, we have considered the specific pre-drainage, since, as already mentioned, the matrix potential plays a major role in soil stability.
The following pre-settings were chosen:
• Conventional management (includes Ap-horizon).
• Pre-drainage: pF 1.8
• OM≤5 %
• BD > 1 g cm−³
• Specification of texture classes (sand, silt and loam)
• Indication of Pc and/or cohesion (c).
The pre-drainage of pF 1.8 was chosen to define the weakest (most moist condition in spring) soil conditions. Only TS and SS were selected for each profile, without any further horizon differentiation of the SS. A TS horizon with a designated platy structure (pla) was added to SS, because the formation of a platy structure in the TS is rather unlikely with regular ploughing. The pre-settings resulted in 150 profiles from six countries: China (4), Estonia (2), Germany (121), Great Britain (3), Norway (17), and Switzerland (3). Terrestrial soils dominate conventionally farmed agricultural land, account for over 91%, and are represented by eleven different soil types (Table 4).
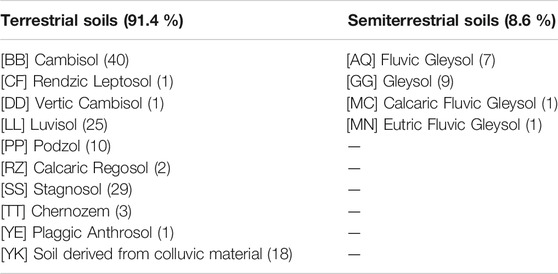
TABLE 4. Soils according to the German classification system (Ad-Hoc-AG Boden 2005, in parentheses) and WRB (2014), n = 150.
Four semi-terrestrial soil types (ground water affected soil types) are also represented and account for about 9%. Cambisols are the most represented (30%), followed by Stagnosols (22%) and soils derived from colluvic material (14%).
From soil types with corresponding specifications, there are a total of 348 analyzed soil horizons with Pc data (Table 5).
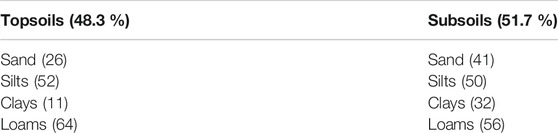
TABLE 5. Topsoils (n = 153) and subsoils (n = 179) of conventionally farmed land and their classification into main soil texture classes: sands (n = 73), silts (n = 111), clays (n = 39) and loams (n = 109), absolute values of the main texture groups (BHG) are given in parentheses (n = 348).
Overall, loam (36%) and silt (31%) are the most represented soil texture. The percentage of sandy soils is 20.1%. When considering the soil types with respect to the grain size distribution (Figure 2), a total of 25 different soil texture classes can be defined. The most common soil texture class is medium loamy sands (Sl3) with 17% and strong loamy sands (Sl4) with 15%, which corresponds to one third of the horizons. A second concentration of soil texture classes is observed in the medium clayey silt (Ut3), with 12% and in the strong clayey silt (Ut4) with 10%.
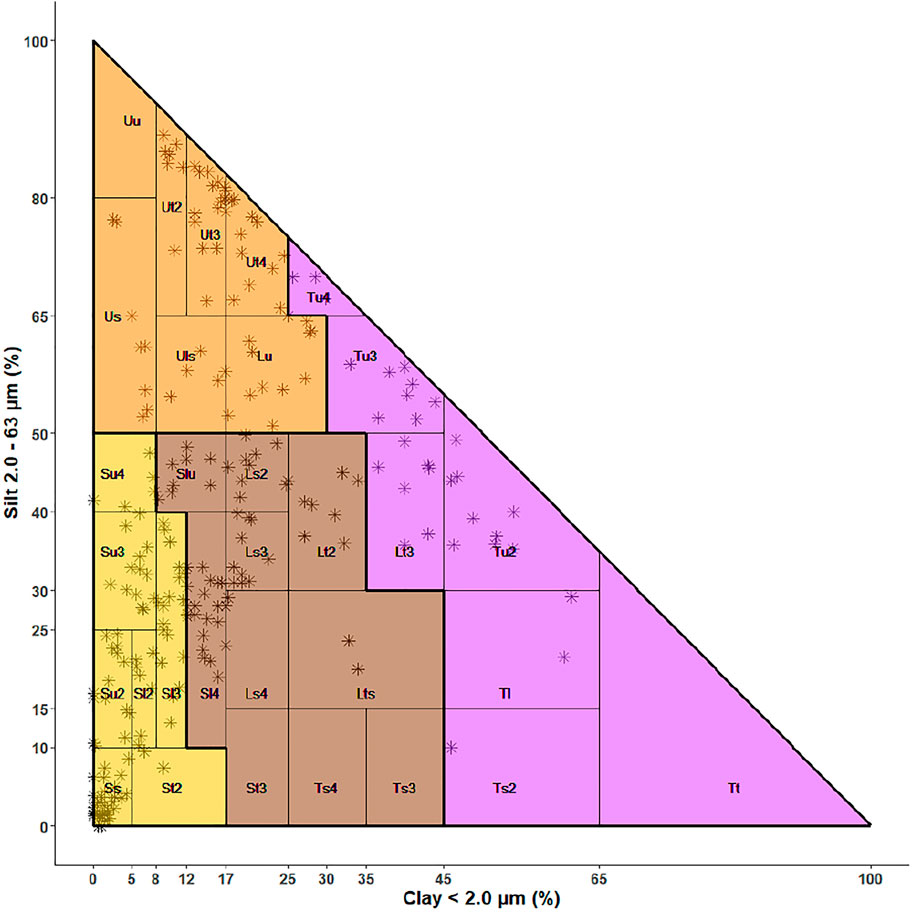
FIGURE 2. Texture classes (according to Ad-Hoc AG Boden 2005) from the selected 138 profiles with precompression (Pc) values and Saturated hydraulic conductivity (ks) values, star = topsoil (n = 142), square = subsoil (n = 147), n = 289.
Statistical Evaluation
Statistical analysis of the data was done using the statistical program R 4.0.3 (R Core Team, 2020). For processing, tables in AG Bodenkunde, 1982, AG Boden, 1994, Ad-hoc-AG Boden, 2005 are linked in R to form a main table using R package called dplyr to allow uniform statistical analyses of all profiles.
Time series have been established on 35% of the studied plots. For this reason, the “date” was considered as a parameter for sampling. Multiple linear regression analysis was performed according to Chambers (1992) and Wickham et al. (2021). In the computational model (Eq. (2)), the precompression stress (y (Pc)) is described as a dependent variable to the independent variables of organic matter (χ1(OM)), bulk density (χ2(BD)), and the year of sampling (χ3 (year)). ϵ is the level of variance in the error term or residuals of multi linear regression model.
Statistically significant differences between samples were determined by using Wilcoxon and T-tests. The results were classified as statistically significance level (***) p < 0.001 (**) p < 0.01 [*] p < 0.05, [] p > 0.05 and is given in each Equation (Eq. 3–8). Furthermore, all laboratory analyses were readjusted to the standard of Ad-hoc-AG Boden (2005), based on raw data, to create comparability among profiles or horizons.
Results
Main Soil Properties and Precompression Stress Ranges in Top- and Subsoils
The following graphs are subdivided into the MTG of sand, loam, and silt, and Pc values as a function of OM (%) and BD (g cm−³) for the TS and SS (Figure 3–5). For the linear regression equations Eq. 3–8, the unit for OM is also % and for BD is g cm−³.
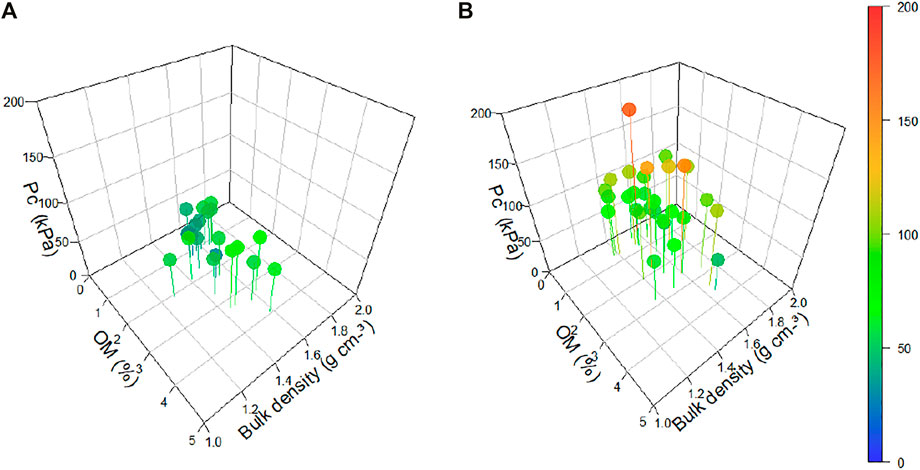
FIGURE 3. (A) Precompression stress (Pc) as a function of the organic matter (OM) and bulk density (BD) of sandy topsoils for conventionally managed arable soilsat a pre-drainage of pF 1.8, n = 26. Figure 3 (B) Precompression stress (Pv) as a function of the organic matter (OM) and bulk density of sandy subsoils for conventionally managed arable soils at a pre-drainage of pF 1.8, n = 41. The colour scale shows the value of Pc.
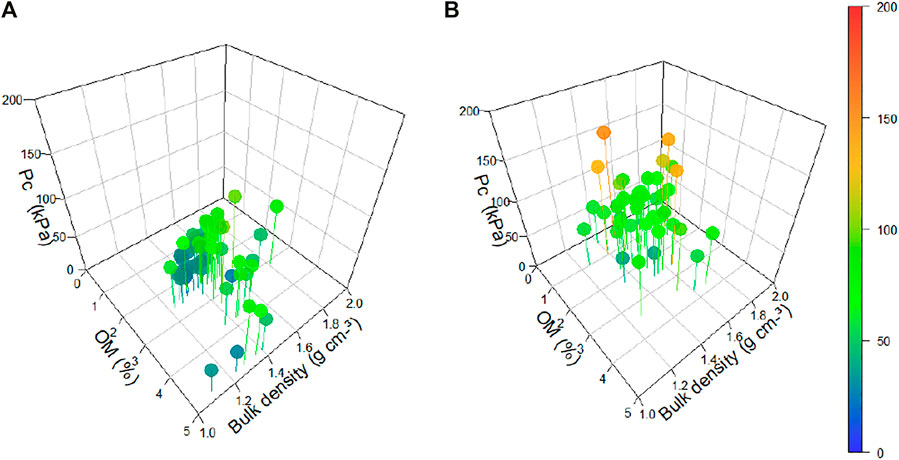
FIGURE 4. (A) Precompression stress (Pc) as a function of organic matter (OM) and bulk density of loamy topsoils of conventionally managed arable soils at a pre-drainage of pF 1.8, n = 64. Figure 4 (B) Pc as a function of organic matter (OM) and bulk density (BD) of loamy subsoils of conventionally managed arable at a pre-drainage of pF 1.8, n = 56. The colour scale shows the value of Pc.
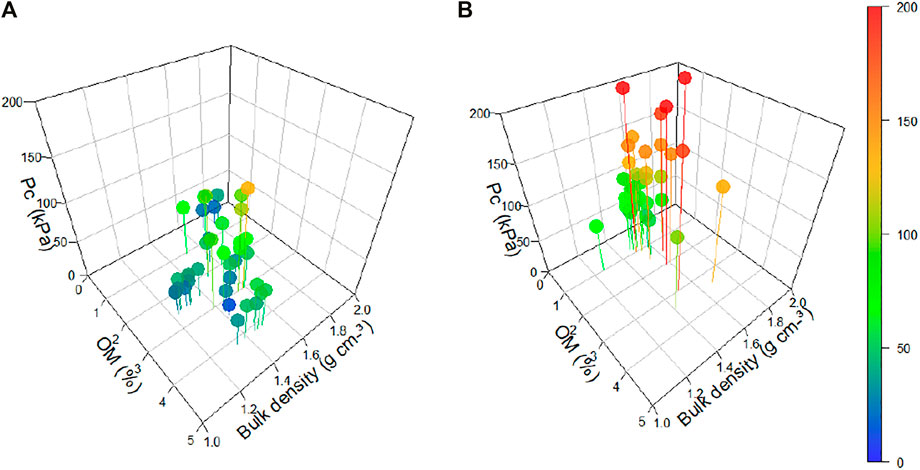
FIGURE 5. (A) Precompression stress (Pc) as a function of organic matter (OM) and bulk density (BD) of silty topsoils of conventionally managed arable soils at a pre-drainage of pF 1.8, n = 52. Figure 5 (B) Pc as a function of organic matter (OM) and bulk density (BD) of silty subsoils (n = 84) of conventionally managed arable soils at a pre-drainage of pF 1.8, n = 50. The colour scale shows the value of Pc.
Sand
Sandy soils samples were taken between 1991 and 2013. The minimum BD value range from 1.27 g cm−3 to 1.84 g cm−3 54% of the horizons had a mean BD between 1.4 and 1.6 g cm−3. The OM range from 0.2 to 3.9%, while the average Pc was 76 kPa, reaching a maximum value of 170 kPa at a BD of 1.62 g cm−3 and OM of 0.21%. The lowest Pc values, between 30 and 39 kPa, were observed at BD of 1.52–1.58 g cm−3 and an OM between 1 and 2% (Figure 3).
A large amount of reclaimed and colluviated horizons were noted between 2002 and 2013 and are characterized by very low Pc values in both TS and SS. This circumstance leads to a significant decrease in Pc as a function of time and must be considered (Eq. (3) and (4).
In sandy horizons, neither the carbonate nor the clay content (<12%) in the available data reached values that could lead to separated aggregates like (sub)angular blocky structure. When considering the TS (Figure 3A), an average Pc of 51 kPa was measured and the OM was on average 2.0%. There was only one value with <1% (n = 1).
The regression analysis for the TS showed a positive correlation between OM (p < 0.05) and Pc (Eq. (3)).
In the SS (Figure 3B), compared to the TS, the average Pc increased by 74%–94 kPa. The OM showed a 12% decrease to 1.4%. In comparison, the average BD of 1.58 g cm− 3 was increased by 3%. (Eq. (4)).
Neither in TS nor in SS BD have a significant effect on Pc. However, the year of sampling had a significant influence on Pc in SS. Pc decreased as a function of time, for the sandy SS (p < 0.01) which consists mainly of Stagnosols (28%) and soils derived from colluvic material (18.5%) in the later decades with low Pc values.
Loam
Loamy soils samples were taken between 1979 and 2019. Most of the conventionally farmed profiles in the database are loamy soils. The main soil types are classified as Cambisol (48%), followed by Stagnosol (30%). The ranges of values for OM (0.3–4.8%) and for BD (1.2–1.86 g cm−³) are wider in loamy soils (Figure 4) compared to the sandy ones (Figure 3). The average Pc of the soils with loamy texture is 67 kPa and is 12 kPa smaller than the Pc of sandy soils. However, their scattering is larger, ranging from 21 to 160 kPa.
There is a high amount of OM in loamy SS. Due to their pedogenesis and management methods over the last decades, a large number of the soil types (Cambisol, Luvisol, Stagnosol) show increasing OM in SS. On average, the OM of the loamy TS (Figure 4A) is 2.8%, with 50.9% of those classified as medium humous (h3) and 23.6% as weak humous (h2). The BD ranges from ρ 2 (1.1 g cm−3) to ρ 5 (1.81 g cm−3) with the maximum Pc of 113 kPa. Significances in the loamy TS is observed for BD (p < 0.01) and year (p < 0.001) with an R2 = 0.45. However, OM (p > 0.05) has no statistical effect on Pc at all.
In the loamy SS (Figure 4B), a larger scatter is observed for OM and BD (R2 = 0.17). The average Pc is 84 kPa, which is 66% more compared to that of TS. For OM < 1%, as well as for BD > 1.7 g cm−3, Pc values exceed 120 kPa mostly for Cambisol and Stagnosol. BD (p < 0.05) have a significant influence of Pc in both TS and SS. Normally, the SS, in contrast to the TS, has strongly reduced OM. For loamy SS 30% M-Horizons with high OM content and a slightly decrease of Pc over years was observed (p < 0.01).
Silt
Silty soils samples were taken between 1979 and 2019 and have the highest Pc (200 kPa in SS). They represent very low to high OM content (h0 - h4) and low to very high BD values (ρ 2—ρ 5). In silty TS a direct effect of OM nor BD on Pc is not detectable (R2 = 0.26). Due to mechanical tillage under conventional management, the mechanical strength (p < 0.05) decline significantly over years (Eq. (7)). The average Pc and OM are 49 kPa and 2.6%, respectively. The BD varies from low (ρ 2) to very high (ρ 4) due to different mechanical tillage methods and sampling times. Sampling with the highest BD took place direct after wheeling experiments.
In the silty SS (Figure 5A), a significantly lower humus content and a 220% increase in average Pc (up to 114 kPa) compared to TS can be observed. In the SS with Pc > 100 kPa, platy structures are found almost exclusively, accounting for 28.1% of the silty SS. Further, no colluvic material was detected in silty SS. Only OM have a significant effect on Pc (p < 0.001) but BD are not related to Pc at all (R2 = 0.25). Between 1981 and 1997, a steady increase in the Pc in the SS from 40 to over 200 kPa was also observed for identical silty plots. In addition, increased platy structures occurred, which were first used agriculturally since 1995.
Saturated Hydraulic Conductivity
In Figure 6 (top) ks in cm d−1 is shown as a function of Pc divide into classification groups (p1 - p6) for all soil profiles. Figure 6 (bottom) show the profile horizons which are subdivided into the MTG. For better comparability with the results of Horn and Fleige (2009) in Table 2, to evaluate the impact of Pc on ks.
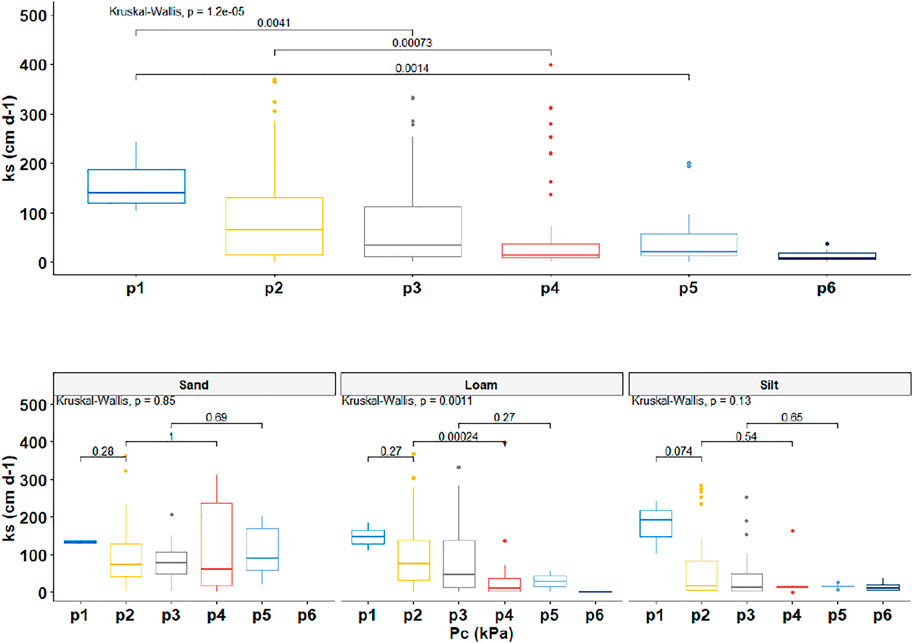
FIGURE 6. Saturated hydraulic conductivity (ks) to precompression stress (Pc) divided into groups p1 (0–30 kPa), n = 10, p2 (30–60 kPa), n = 96, p3 (60–90 kPa), n = 94, p4 (90–120 kPa), n = 55, p5 (120–150 kPa), n = 20, and p6 (>150 kPa), n = 14, terrestrial soils in total (top) and divided into the main soil texture classes (BHG) (bottom), n = 289.
With increasing Pc ks decreases significantly over all three MTG (p < 0.01) (Figure 6 top). From Pc group p2 (30–60 kPa) to group p4 (90–120 kPa), ks decreases by 72%, falling from 50 cm d−1–13.8 cm d−1 (p < 0.05). In p3 (60—90 kPa), 75% of the values are still above the critical value of 10 cm d−1, with a median of 26.6 cm d−1. Even at a Pc of 90–120 kPa, 50% of the soil horizons have a value of <14 cm d−1. In group p6 (>150 kPa), >50% of the measurements are below 10 cm d−1, with the median dropping further to 8.3 cm d−1.
Sandy soils from p3—p5 have the highest ks values with no significant decrease of ks (p = 0.7) between the Pc groups (Figure 6 bottom). The median for the groups p2—p5 is between 40 and 100 cm d−1 and shows no critical ks value up to 150 kPa. In loamy soils a pronounced decline of the ks occurs with increasing Pc. Up to 90 kPa, 75% of the conductivities are above 10 cm d−1, but experience a significant reduction from p2 to p4 to 11.2 cm d−1 (p < 0.01). Nearly 50% of the ks of loamy soils with Pc between 90 and 120 kPa are below 10 cm d−1 and are reduced by a factor of 10 as the Pc increases to 120—150 kPa. The ks of silty soils is more sensitive to Pc compared to sandy and loamy soils. Between group p1 and p2 the ks is reduced by 75% and drops from 69 cm d−1–17 cm d−1 (p = 0.07). As Pc continues to increase beyond 60 kPa, conductivity remains consistently low and varying between 11 and 16 cm d−1. If the Pc of 150 kPa is exceeded, all ks are smaller than the critical value of 10 cm d−1.
Discussion
Bulk Density, Organic Matter, and Soil Structure
The evaluation of physical and mechanical parameters of undisturbed soil samples is a time-consuming, but necessary, approach to document the natural behaviour of soils. This approach is even the more urgent today, because only by compilation of the data already collected can undisturbed soil samples be evaluated statistically and analysed to develop advanced pedotransfer functions. Also, changes over years can be documented. A section of the database presented in this paper allows differentiation of soil horizons using chemical and physical parameters. In addition, the changes of the parameters over time can help to elucidate alterations due to soil management - e.g., the long-term consequences of high axial loads on agricultural soils.
The very low Pc values in the TS of the conventionally farmed areas (78%) is due to continuous tillage, which repeatedly homogenizes the existing aggregates. Hartge and Horn (2016), Zheng et al. (2018) and Chellappa et al. (2021), showed that, compared to conservation soil management, conventional soil management homogenizes and destroys soil structure by reducing the number of macroaggregates (>1 mm), and it makes soils more susceptible to soil deformation. Furthermore, the database contains data for samples that were collected during spring to autumn, which furthermore will affect the soil strength. These interactions will be subject to further analyses. Kay et al. (1987), Ehlers et al. (2000) and Horn and Smucker (2005) described seasonal changes from the homogenization due to tillage and a small increase in soil strength throughout the year. They observed that, over time, conservation or no tillage resulted in a higher soil strength which comes along with a quasi-dynamic equilibrium at smallest free entropy and optimized accessibility of particle surfaces (for more details see also Hartge and Horn 2016). However, reduced stability in the TS of all MTG results in enhanced water erosion, loss of OM and following retarded structure formation and strengthening due to glueing etc. as well as an enhanced methane or N2O emission to the atmosphere (Puget and Lal, 2005; Mishra et al., 2010; Du et al., 2013; Haas et al., 2016; Zheng et al., 2018). Finally, it also causes an increase in dispersed clay in the soil solution (Watts and Dexter, 1997).
There is nearly an inverse relationship in the SS below the plough depth (>25–30 cm) and the TS, when BD, OM, and soil strength are considered. The lack of soil loosening on arable land combined with the rapid increase in axle loads of agricultural machinery by about 65% between 1989 and 2009 (Schjønning et al., 2015; Keller et al., 2019) should led to a strong increase in Pc with a coinciding worsening of ks and air permeability. Keller et al. (2019) concluded that high axle loads and driving in the plough furrow directly provoked SS over-compaction. This result can partial be confirmed for SS from the database. 20% of loamy SS and 62% of silty SS show platy structures at Pc of >140 kPa and a significant relation to BD in silty and loamy SS. This development increases the penetration resistance for plant roots and reduces accessibility of particle surfaces and exchange places for nutrient storage and availability (Jansson and Johansson, 1998; Bygdén et al., 2004). At OM < 1%, Bt and Bw-horizons with polyhedral and platy structures dominate and indicate a strong increase in soil strength. The influence of BD also has an impact on the composition of the soil horizons, and results for BD are similar to the horizons with OM < 1%. Again, the Bt- and Bw-horizons have both the highest Pc (by low OM < 1%) and BD > 1.5 g cm−³. Our results confirm the model predictions of Hartmann et al. (2012) concerning reduced aeration and plant available water storage in combination with soil compaction effects.
On the other hand, proportion of stagnic M-horizons of colluvic material (17%) and buried A-horizons for loamy and silty soils are particularly high, which were recorded between the 80s and 00’s. Thus, we explain the strong presence of OM in the SS with colluvial materials, which also have the lowest Pc. This observation is consistent with the conclusions of Blanco-Canqui et al. (2009). This circumstance lead to the fact that in unstructured sandy and loamy SS with high OM values (>1%) decreasing values of Pc over years (p < 0.05) are observed. This unnatural increase of OM in SS thus causes a stronger slope of the regression line in the sandy and loamy soils and leads to implausible values outside the given time frame in years. For further considerations, especially OM contents have to be looked at more closely under the aspect of soil genesis on agricultural soils.
Further, the reduced number, deformation and destruction of soil aggregates by ploughing (Horn and Fleige, 2003; Six et al., 2004; Arvidsson and Keller, 2007; Page-Dumroese et al., 2006; Imhoff et al., 2015) and the increasing axle loads (Schjønning et al., 2015), result not only in higher stress induced strains and soil shearing in the TS but also in the SS with low OM content. This deformation due to compaction and shearing cannot be explained by the densification of the soil with increasing BD which confirm the findings of Huang et al. (2021a, b), who showed that different forms of stress (static or cyclic loading) application resulted in variable internal soil processes, including a loosening, irrespective of high stresses applied. These relations also confirmed that the primary classification based on soil texture is not sufficient to document general differences in soil strength, if soils as three-phase-systems are considered. OM and the matric potential in each case are the main factors influencing soil stability in highly disturbed agricultural TS and SS, since natural structuring by swelling and shrinkage and following strengthening by organic compounds like acids, exudates etc. can only occur in very short time frames before the next destruction due to repeated tillage, seedbed preparation followed by wheeling throughout the season due to spraying and fertiliser applications occurs. These impacts result in a continuous arrangement of particles, changes in pore continuity and coinciding altered up to short term positive pore water pressure. All this makes the predictions using texture dependencies less reliable and does not allow long-term predictions (Horn et al., 2019a).
Arable soils with reduced stability due tillage are much more susceptible to over-compaction (Arvidsson and Hakansson, 1996) and this is evident because excessive Pc (>150 kPa) especially of silty soils results in poor ecological properties like ks. This observation leads to the assumption that MTG, depending on the structuring and matric potential, have different Pc ranges in which sufficient soil strength with good ecological parameters are given.
Soil structure down to deeper depths needs to be linked with soil strength data and soil functions. At a given matric potential down to depth are structured soils the stronger the more developed are the aggregates (Horn, 1981) which results in minor or no changes in hydraulic conductivity or air permeability (Hartge and Horn, 1989; Wiermann, 1998; Zink et al., 2010; Horn et al., 2018). This observation can be one of the main reasons for the low R2 between Pc, OM and BD in the three MTG under consideration. Horn (1981) arranged various aggregate types according to increasing soil strength starting with coherent-prismatic-blocky, subangular blocky, and finally crumbly structure. However, due to the today’s stresses applied will the aggregate dependent Pc values of such structures be exceeded by anthropogenic (tillage, wheeling etc.) and geological processes (ice pressure), and form plats which prevent not only an easy deep rooting and high accessibility of particle surfaces for nutrient storage etc., but even more important is the newly formed horizontal anisotropy for fluxes (Horn et al., 2019a). The link between these aggregate types and soil mechanical properties is essential to know for a reliable prediction as it was already published by Fleige et al. (2002) and Horn and Fleige, (2009) for smaller datasets. Thus, the low R2 is due to the extreme highly variable OM contents (0–4%) in SS.
In further papers we will compare selected data (based on soil type and perennial sampling) over time, because it would prove and enlarge the findings of Ehlers et al. (1983) that soil strength is one of the most important factors for root growth and that over time the over-compaction status of soils reaches deeper soil layers. The consequences of the more compacted soil horizons can be also derived from the delayed root growth down to depth (Keller et al., 2019) which especially concerning the necessary nutrient and water uptake by plant roots will cause yield loss besides increasing soil loss due to water and wind erosion.
Saturated Hydraulic Conductivity
It is important to determine when detrimental degradation of the soil occurs. The concept of Pc, according to Horn et al. (1991b) and Lebert and Horn (1991), describes the mechanical strength of soil structure against vertical compressive stresses. If the mean normal stress (σn) is smaller than the Pc, the soil retains its physical integrity and no changes occur with respect to pore volume, pore connectivity, and continuity. If σn, however, exceeds the Pc, i.e (Pc/σn < 1), irreversible changes result, including reduced gas diffusion, hydraulic conductivity, and negatively altered pore size distribution. The DVWK (1997) documents the link between Pc and limiting soil properties like ks (Table 3), which is based on the new database.
In sandy soils, the single grain structure is almost exclusively present, according to the results the primary pore size distribution allows a high ks to be measured even beyond the critical stress value of >150 kPa.
However, loamy soils are much more sensitive to elevated Pc. Nearly 50% of the measured ks are below the critical value of 10 cm d−1 (UBA, 2004a) at Pc between 90 and 120 kPa. According to Horn and Fleige (2009) the limit of 90 kPa for over-compression threat at 1.8 pF can thus be confirmed for loamy soils. Given the average Pc values of loamy (84 kPa) and silty (114 kPa) SS, we can assume that the recorded soils under agricultural use of the last 40 years show critical ks values. Between 30 and 150 kPa, silty soils have lower ks than loamy and sandy soils. A total of 27% of silty SS fall below the critical value, which is an indicator of the sensitivity of these soils. Although silty soils are among the most productive soils also in Germany and have been intensively used for agriculture for decades or even centuries, no restriction values regarding maximum allowed stress application have been defined. According to the studies of Imhoff et al. (2015) and Díaz-Zorita and Grosso (1999), soil vulnerability increases with increasing silt content and humid conditions (precipitation > evaporation), which confirms the results in this study.
Final Remarks
The data presented in this first paper indicate that soil strength or remained constant but the coinciding ecological soil functions of arable soils decreased and can confirm the analyses of Keller et al. (2019). The dataset can be the basis for predictions how soil management can limit soil type and structure dependent sustainability if applied stresses exceed the internal soil strength and following changes in plant growth, fluxes including groundwater recharge, surface runoff and carbon sequestration. It is irrefutable, that soil deformation processes and intensities depend on texture in combination with soil aggregation which require corresponding and varying threshold values to avoid further soil degradation and preserve the actual soil properties for further generations.
Conclusion
Agricultural soils are anthropogenically influenced and differ from natural sites in terms of their mechanical properties. For the derivation of valid mechanical soil models, the development of a soil database, such as the one presented in this paper, is essential to estimate the consequences of mechanical cultivation. The data show that the three MTG (sand, loam, and silt) have different Pc values as a consequence of long-term agricultural soil management. The factors BD and OM of the TS and SS alone do not characterize sufficiently the Pc, because the latter depends on soil structure and pore water pressure, too. Within the scope of this project, these connections for the entire soil profile data are described in the following papers with detailed analyses.
The link and comparison between the Pc, and the limiting soil ecological functions can be the basis for soil type specific management recommendations as they are defined in the German soil Protection law (BBodSchG, 1998) by means of the actual, precaution and activity values to avoid further irreversible soil degradation.
Data Availability Statement
The original contributions presented in the study are included in the article/supplementary material, further inquiries can be directed to the corresponding author.
Author Contributions
HF supervises my dissertation work. CH collaborated on the structure of the database. RH, as a former prof, is reviewing the research.
Conflict of Interest
The authors declare that the research was conducted in the absence of any commercial or financial relationships that could be construed as a potential conflict of interest.
Publisher’s Note
All claims expressed in this article are solely those of the authors and do not necessarily represent those of their affiliated organizations, or those of the publisher, the editors and the reviewers. Any product that may be evaluated in this article, or claim that may be made by its manufacturer, is not guaranteed or endorsed by the publisher.
Acknowledgments
The authors are highly indebted to Prof. M.B. Kirkham United States (Kansas State University) for her very valuable English language corrections and intense discussion contributions.
References
Ad-hoc AG Boden (2005). Bodenkundliche Kartieranleitung, 5. Aufl. Stuttgart: Schweizerbart´sche Verlag, 438
AG Boden (1994). Bodenkundliche Kartieranleitung, 4. Aufl. Nachdr. Stuttgart: Schweizerbart´sche Verlag, 392pp.
AG Bodenkunde (1982). Bodenkundliche Kartieranleitung, 3. Aufl. Stuttgart: Schweizerbart´sche Verlag, 331.
Alakukku, L., Weisskopf, P., Chamen, W. C. T., Tijink, F. G. J., van der Linden, J. P., Pires, S., et al. (2003). Prevention Strategies for Field Traffic-Induced Subsoil Compaction: a Review. Soil Tillage Res. 73, 145–160. doi:10.1016/S0167-1987(03)00107-7
Alakukku, L. (1996). Persistence of Soil Compaction Due to High Axle Load Traffic. I. Short-Term Effects on the Properties of clay and Organic Soils. Soil Tillage Res. 37, 211–222. doi:10.1016/0167-1987(96)01016-1
Alekseeva, T., and Alekseev, A. (1999). Factors Affecting the Structural Stability of Three Contrasting Soils of China. Catena 38, 45–64. doi:10.1016/S0341-8162(99)00055-7
Arthur, E., Schjønning, P., Moldrup, P., and de Jonge, L. W. (2012). Soil Resistance and Resilience to Mechanical Stresses for Three Differently Managed sandy Loam Soils. Geoderma 173-174, 50–60. doi:10.1016/j.geoderma.2012.01.007
Arvidsson, J., and Hakansson, I. (1996). Do effects of Soil Compaction Persist after Ploughing? Results from 21 Long-Term Field Experiments in Sweden. Soil Tillage Res. 39, 175–197. doi:10.1016/S0167-1987(96)01060-4
Arvidsson, J., and Keller, T. (2007). Soil Stress as Affected by Wheel Load and Tyre Inflation Pressure. Soil Tillage Res. 96, 284–291. doi:10.1016/j.still.2007.06.012
Arvidsson, J., Trautner, A., Van Den Akker, J. J. H., and Schjønning, P. (2001). Subsoil Compaction Caused by Heavy Sugarbeet Harvesters in Southern Sweden II. Soil Tillage Res. 60, 79–89. doi:10.1016/s0167-1987(01)00168-4
Batey, T., and McKenzie, D. C. (2006). Soil Compaction: Identification Directly in the Field. Soil Use Manage. 22, 123–131. doi:10.1111/j.1475-2743.2006.00017.x
Baumgartl, W., and Horn, R. (2013). “Assessing Soil Degradation by Using a Scale-Spanning Soil Mechanical Approach: A Review. Soil Degradation 1-61,” in Advances in Geoecology (Catena Verlag), 42. 978-3-923381-59-3.
BBodSchG (1998). Gesetz zum Schutz des Bodens vom 17.03.1998. Germany: The Federal Ministry of Justice and Consumer Protection, 502. BGBl. I S.
Berisso, F. E., Schjønning, P., Keller, T., Lamandé, M., Etana, A., de Jonge, L. W., et al. (2012). Persistent Effects of Subsoil Compaction on Pore Size Distribution and Gas Transport in a Loamy Soil. Soil Tillage Res. 122, 42–51. doi:10.1016/j.still.2012.02.005
Blanco-Canqui, H., Stone, L. R., Schlegel, A. J., Lyon, D. J., Vigil, M. F., Mikha, M. M., et al. (2009). No-till Induced Increase in Organic Carbon Reduces Maximum Bulk Density of Soils. Soil Sci. Soc. Am. J. 73, 1871–1879. doi:10.2136/sssaj2008.0353
Blume, H. P., Stahr, K., and Leinweber, P. (2011). Bodenkundlichen Praktikum. Heidelberg: Spektrum Verlag, 255. 978-3-8274-1553-0.
Bridges, E. M., and Oldeman, L. R. (1999). Global Assessment of Human-Induced Soil Degradation. Arid Soil Res. Rehabil. 13 (4), 319–325. doi:10.1080/089030699263212
Bygdén, G., Eliasson, L., and Wästerlund, I., (2003). Rut Depth, Soil Compaction and Rolling Resistance when Using Bogie Tracks, J. Terramechanics 40, 179–190. doi:10.1016/j.jterra.2003.12.001
Casagrande, A. (1936). “The Determination of Pre-consolidation Load and its Practical Significance,” in Proc. Int. Conf. Soil Mech. Found. Eng. Harvard University Cambridge, 60–64.
Chambers, J. M. (1992). “Linear Models,” in Chapter 4 of Statistical Models in S. Editors J. M. Chambers, and T. J. Hastie (Wadsworth & Brooks/Cole).
Chellappa, J., Sagar, K. L., Sekaran, U., Kumar, S., and Sharma, P. (2021). Soil Organic Carbon, Aggregate Stability and Biochemical Activity under Tilled and No-Tilled Agroecosystems. J. Agric. Food Res. 4, 100139. doi:10.1016/j.jafr.2021.100139
Dexter, A. R., Richard, G., Arrouays, D., Czyż, E. A., Jolivet, C., and Duval, O. (2008). Complexed Organic Matter Controls Soil Physical Properties. Geoderma 144 (Issues 3–4), 620–627. doi:10.1016/j.geoderma.2008.01.022
Díaz-Zorita, M., and Grosso, G. A. (1999). Effect of Soil Texture, Organic Carbon and Water Retention on the Compactability of Soils from the Argentinean Pampas. Soil Tillage Res. 54, 121–126.
Dörner, J., and Horn, R. (2006). Anisotropy of Pore Functions in Structured Stagnic Luvisols in the Weichselian Moraine Region in N Germany. Z. Pflanzenernähr. Bodenk. 169, 213–220. doi:10.1002/JPLN.200521844
Du, Z.-l., Ren, T.-s., Hu, C.-s., Zhang, Q.-z., and Blanco-Canqui, H. (2013). Soil Aggregate Stability and Aggregate-Associated Carbon under Different Tillage Systems in the North China Plain. J. Integr. Agric. 12, 2114–2123. doi:10.1016/S2095-3119(13)60428-1
Duttmann, R., Schwanebeck, M., Nolde, M., and Horn, R. (2014). Predicting Soil Compaction Risks Related to Field Traffic during Silage Maize Harvest. Soil Sci. Soc. America J. 78 (2), 408–421. doi:10.2136/sssaj2013.05.0198
DVWK (1997). Soil Strength in Structured Unsaturated Soils. Part II Physical Soil Properties (In German, with English Summary and Captures) Gefügestabilität Ackerbaulich Genutzter Mineralböden. Teil II: Ableitung Physikalischer Bodenkenngrößen. Merkblätter 235, Wirtschafts- and Verlagsges. Bonn: Gas and Wasser.
Ehlers, W., Kopke, U., Hesse, F., and Bohm, W. (1983). Penetration Resistance and Root Growth of Oats in Tilled and Untilled Loess Soil. Soil Tillage Res. 3, 261–275. doi:10.1016/0167-1987(83)90027-2
Ehlers, W., Werner, D., and Mähner, T. (2000). Wirkung mechanischer Belastung auf Gefüge und Ertragsleistung einer Löss-Parabraunerde mit zwei Bearbeitungssystemen. J. Plant Nutr. Soil Sci. 163, 321–333. doi:10.1002/1522-2624(200006)163:3<321::aid-jpln321>3.0.co;2-y
Fazekas, O., and Horn, R. (2005). Zusammenhang zwischen hydraulischer und mechanischer Bodenstabilität in Abhängigkeit von der Belastungsdauer. Z. Pflanzenernähr. Bodenk. 168, 60–67. doi:10.1016/j.geoderma.2011.02.006
Feller, C., and Beare, M. H. (1997). Physical Control of Soil Organic Matter Dynamics in the Tropics. Geoderma Vol. 79 (Issues 1–4), 69–116. doi:10.1016/S0016-7061(97)00039-6
Fleige, H., Horn, R., and Stange, F. (2002). Soil Mechanical Parameters Derived from the CA-database on Subsoil Compaction. Adv. GeoEcology 35, 359–366. doi:10.1016/j.still.2011.04.004
Fraters, B. (1996). Generalized Soil Map of Europe. Aggregation of the FAO-UNESCO Soil Units Based on the Characteristics Determining the Vulnerability to Degradation Processes. Bilthoven, Netherlands: National Institute of Public Health and the Environment, 67.
Haas, C., Holthusen, D., Mordhorst, A., Lipiec, J., and Horn, R. (2016). Elastic and Plastic Soil Deformation and its Influence on Emission of Greenhouse Gases. Int. Agrophys. 30, 173–184. doi:10.1515/intag-2015-0088
Håkansson, I., Voorhees, W. B., Elonen, P., Raghavan, G. S. V., Lowery, B., Van Wijk, A. L. M., et al. (1987). Effect of High Axle-Load Traffic on Subsoil Compaction and Crop Yield in Humid Regions with Annual Freezing. Soil Tillage Res. 10 (3), 259–268. doi:10.1016/0167-1987(87)90032-8
Hamamoto, S., Moldrup, P., Kawamoto, K., Wollesen de Jonge, L., Schjønning, P., and Komatsu, T. (2011). Two-Region Extended Archie's Law Model for Soil Air Permeability and Gas Diffusivity. Soil Sci. Soc. America J. 75, 795–806. doi:10.2136/sssaj2010.0207
Hartge, K. H., and Horn, R. (1989). Die physikalische Untersuchung von Boden. 2.ed. Stuttgart: Enke Verlag.
Hartge, K. H., and Horn, R. (1992). Die physikalische Untersuchung von Boden. 3.ed. Stuttgart: Enke Verlag.
Hartge, K. H., and Horn, R. (2009). Die physikalische Untersuchung von Böden. Stuttgart 4. Schweitzerbart´sche Vertragsbuchhandlung. 978-3-510-65246-4.
Hartge, K. H., and Horn, R. (2016). Essential Soil Physics. Stuttgart: Schweizerbart Science Publisher. 978-3-510-65288-4.
Hartge, K. H. (1978). Einführung in die Bodenphysik, 364 Seiten, Ferd. Stuttgart: Enke Verlag. 3‐432‐89682‐4. doi:10.1002/jpln.19811440222
Hartmann, P., Zink, A., Fleige, H., and Horn, R. (2012). Effect of Compaction, Tillage and Climate Change on Soil Water Balance of Arable Luvisols in Northwest Germany. Soil Tillage Res. 124, 211–218. doi:10.1016/j.still.2012.06.004
Hettiaratchi, D. R. P. (1987). A Critical State Soil Mechanics Model for Agricultural Soils. Soil Use Manage. 3, 94–105. doi:10.1111/j.1475-2743.1987.tb00718.x
Horn, R., and Blum, W. E. H. (2020). Effect of Land-Use Management Systems on Coupled Physical and Mechanical, Chemical and Biological Soil Processes: How Can We Maintain and Predict Soil Properties and Functions? Front. Agr. Sci. Eng. 7 (3), 243. Article Number 104709. doi:10.15302/J-FASE-2020334
Horn, R., and Dexter, A. R. (1989). Dynamics of Soil Aggregation in an Irrigated Desert Loess. Soil Tillage Res. 13 (3), 253–266. doi:10.1016/0167-1987(89)90002-0
Horn, R., and Fleige, H. (2009). Risk Assessment of Subsoil Compaction for Arable Soils in Northwest Germany at Farm Scale. Soil Tillage Res. 102, 201–208. doi:10.1016/j.still.2008.07.015
Horn, R., and Smucker, A. (2005). Structure Formation and its Consequences for Gas and Water Transport in Unsaturated Arable and forest Soils. Soil Tillage Res. 82 (1), 5–14. doi:10.1016/j.still.2005.01.002
Horn, R., Baumgartl, T., Kühner, S., Lebert, M., and Kayser, R. (1991a). Zur Bedeutung des Aggregierungsgrades für die Spannungsverteilung in strukturierten Böden. Z. Pflanzenernaehr. Bodenk. 154, 21–26. doi:10.1002/jpln.19911540106
Horn, R., Lebert, M., and Burger, N. (1991b). “Vorhersage der mechanischen Belastbarkeit von Böden als Pflanzenstandort auf der Grundlage von Labor- und In Situ-Messungen,” in Materialien 73: Mechanische Belastbarkeit von Böden Bayerns. Bayerisches Staatsministerium für Landesentwicklung und Umweltfragen München. doi:10.1016/j.jenvman.2005.11.022
Horn, R., Fleige, H., and Zimmermann, I. (2017). “Soil Texture and Structure: Role in Soil Health,” in Managing Soil Health for Sustainable Agriculture, Volume 1: Fundamentals. Editor D. Reicosky (Cambridge, UK: Burleigh Dodds Science Publishing). 978 1 78676 188 0. doi:10.1016/j.geodrs.2021.e00398
Horn, R., Fleige, H., Lal, R., and Zimmermann, I. (2018). “Soil Health and Functions as a Basic Requirement for Advancing the SDG´s, 52-60,” in Soils and Sustainable Development Goals. GeoEcology Essay. Catena Soil Sciences. Editors R. Lal, R. Horn, and T. Kosaki (Stuttgart. 978-3-510-65425-3.
Horn, R., Mordhorst, A., Fleige, H., Zimmermann, I., Burbaum, B., Filipinski, M., et al. (2019a). Soil Type and Land Use Effects on Tensorial Properties of Saturated Hydraulic Conductivity in Northern Germany. Eur. J. Soil Sci. 71, 179–189. doi:10.1111/ejss.12864
Horn, R. (1980). Die Ermittlung der vertikalen Druckfortpflanzung im Boden mit Hilfe von Dehnungsmeßstreifen. Z. Kulturtechnik Flugbereinigung 21, 343–349.
Horn, R. (1981). “Die Bedeutung der Aggregierung von Böden für die mechanische Belastbarkeit.” in Schriftenreihe TU Berlin. 200. 3-7983-0792-X
Horn, R. (1983). Die Bedeutung der Aggregierung für die Druckfortpflanzung im Boden. Z. Kulturtechnik Flugbereinigung 24, 238–249.
Horn, R., and Fleige, H. (2003). A Method for Assessing the Impact of Load on Mechanical Stability and on Physical Properties of Soils. Soil and Tillage Research 73, 89–99. doi:10.1016/S0167-1987(03)00102-8
Horn, R., Mordhorst, A., Fleige, H., Zimmermann, I., Burbaum, B., and Filipinski, M. (2019). Soil Type and Management Effects on Organic Carbon Stocks and Soil Structure Quality in North Germany. Bulgarian Journal of Soil Science 4.
Horn, R. (2021). “Soils in Agricultural Engineering: Effect of Land-Use Management Systems on Mechanical Soil Processes,” in Hydrogeology, Chemical Weathering, and Soil Formation. Editors A. Hunt, M. Egli, and B. Faybishenko (Hoboken, NJ, USA: Wiley & Sons), 187–199. 978-1-119-56396-9. Chapter 10A.
Huang, X., Horn, R., and Ren, T. (2021a). Deformation and Pore Water Pressure Change during Static and Cyclic Loading with Subsequent Shearing on Soils with Different Textures and Matric Potentials. Soil Tillage Res. 209, 104909. doi:10.1016/j.still.2020.104909
Huang, X., Horn, R., and Ren, T. (2021b). Soil Structure Effects on Deformation, Pore Water Pressure, and Consequences for Air Permeability during Compaction and Subsequent Shearing. Geoderma in press.
Imhoff, S., Pires da Silva, A., Ghiberto, P. J., Tormena, C. A., Pilatti, M. A., and Libardi, P. L. (2015). Physical Quality Indicators and Mechanical Behavior of Agricultural Soils of Argentina. PLoS ONE 11 (4), e0153827. doi:10.1371/journal.pone.0153827
IPCC (2003). Good Practice Guidance for Land Use, Land-Use Change and Forestry. Geneva: Intergovernmental Panel on Climate Change IPCC. 4-88788-003-0.
Janssens, I. A., Freibauer, A., Schlamadinger, B., Ceulemans, R., Ciais, P., Dolman, A. J., et al. (2005). The Carbon Budget of Terrestrial Ecosystems at Country-Scale - a European Case Study. Biogeosciences 2, 15–26. doi:10.5194/bg-2-15-2005
Jansson, K-J., and Johansson, J. (1998). Soil Changes after Traffic with a Tracked and a Wheeled forest Machine: A Case Study on a silt Loam in Sweden. S-750 07 Uppsala, Sweden: Swedish University of Agricultural Sciences, Department of Operational Efficiency.
Jones, R. J. A., Hiederer, R., Rusco, E., and Montanarella, L. (2005). Estimating Organic Carbon in the Soils of Europe for Policy Support. Eur. J. Soil Sci. 56, 655–671. doi:10.1111/j.1365-2389.2005.00728.x
Kay, B. D., Groenevelt, P. H., Angers, D. A., and Baldock, J. A. (1988). Quantifying the Influence of Cropping History on Soil Structure. Can. J. Soil Sci. 68, 359–368. doi:10.4141/cjss88-033
Keller, T., Arvidsson, J., Dawidowski, J. B., and Koolen, A. J. (2004). Soil Precompression Stress. Soil Tillage Res. 77, 97–108. doi:10.1016/j.still.2003.11.003
Keller, T., Lamandé, M., Schjønning, P., and Dexter, A. R. (2011). Analysis of Soil Compression Curves from Uniaxial Confined Compression Tests. Geoderma 163, 13–23. doi:10.1016/j.geoderma.2011.02.006
Keller, T., Colombi, T., Ruiz, S., Manalili, M. P., Rek, J., Stadelmann, V., et al. (2017). Long-term Soil Structure Observatory for Monitoring post-compaction Evolution of Soil Structure. Vadose Zone J. 16, vzj2016.11.0118. doi:10.2136/vzj2016.11.0118
Keller, T., Sandin, M., Colombi, T., Horn, R., and Or, D. (2019). Historical Increase in Agricultural Machinery Weights Enhanced Soil Stress Levels and Adversely Affected Soil Functioning. Soil Tillage Res. 194, 104293. doi:10.1016/j.still.2019.104293
Kirby, J. M. (1991b). Strength and Deformation of Agricultural Soil: Measurement and Practical Significance. Soil Use Manage. 7 (4), 223–229. doi:10.1111/j.1475-2743.1991.tb00878.x
Kühner, S. (1997). Simultane Messung Von Spannungen Und Bodenbewegungen Bei Statischen Und Dynamischen Belastungen Zur Abschätzung Der Dadurch Induzierten Bodenbeanspruchung. PhD Thesis. Germany: Christian-Albrechts-Universität zu Kiel, Schriftenreihe Institut für Pflanzenernährung und Bodenkunde. Band 39.
Larson, W. E., Gupta, S. C., and Useche, R. A. (1980). Compression of Agricultural Soils from Eight Soil Orders. Soil Sci. Soc. Am. J. 44, 450–457. doi:10.2136/sssaj1980.03615995004400030002x
Lebert, M., and Horn, R. (1991). A Method to Predict the Mechanical Strength of Agricultural Soils. Soil Tillage Res. 19, 275–286. doi:10.1016/0167-1987(91)90095-F
Lebert, M. (1989). Beurteilung und Vorhersage der mechanischen Belastbarkeit von Ackerboden. Bayreuther bodenkundliche Berichte 12, 131.
McBride, R. A. (1989). Estimation of Density-Moisture-Stress Functions from Uniaxial Compression of Unsaturated, Structured Soils. Soil Tillage Res. 13, 383–397. doi:10.1016/0167-1987(89)90045-7
Mishra, U., Lal, R., Liu, D., and Van Meirvenne, M. (2010). Predicting the Spatial Variation of the Soil Organic Carbon Pool at a Regional Scale. Soil Sci. Soc. Am. J. 74, 906–914. doi:10.2136/sssaj2009.0158
Mordhorst, A., Fleige, H., Burbaum, B., Filipinski, M., and Horn, R. (2020). Natural and Anthropogenic Compaction in North Germany (Schleswig‐Holstein): Verification of Harmful Subsoil Compactions. Soil Use Manage 37, 556–569. doi:10.1111/sum.12631
O'Sullivan, M. F. (1992). Uniaxial Compaction Effects on Soil Physical Properties in Relation to Soil Type and Cultivation. Soil Tillage Res. 24, 257–269. doi:10.1016/0167-1987(92)90091-O
Page-Dumroese, D. S., Jurgensen, M. F., Tiarks, A. E., Ponder, Jr., F., Sanchez, F. G., Fleming, R. L., et al. (2006). Soil Physical Property Changes at the North American Long-Term Soil Productivity Study Sites: 1 and 5 Years after Compaction. Can. J. For. Res. 36 (3), 551–564. doi:10.1139/x05-273
Paz, A., and Guérif, J. (2000). Influence of Initial Packing Density, Water Content and Load Applied during Compaction on Tensile Strength of Dry Soil Structural Units. Adv. Geoecol. 32, 22–31.
Piccolo, A., Pietramellara, G., and Mbagwu, J. S. C. (1997). Use of Humic Substances as Soil Conditioners to Increase Aggregate Stability. Geoderma 75, 267–277. doi:10.1016/S0016-7061(96)00092-4
Puget, P., and Lal, R. (2005). Soil Organic Carbon and Nitrogen in a Mollisol in central Ohio as Affected by Tillage and Land Use. Soil Tillage Res. 80, 201–213. doi:10.1016/j.still.2004.03.018
R Core Team (2020). R: A Language and Environment for Statistical Computing. Vienna, Austria: R Foundation for Statistical Computing. URL: https://www.R-project.org/.
Riggert, R. (2015). Spannungseinträge unter Holzerntemaschinen und Auswirkungen auf bodenphysikalische Parameter. Dissertation. Germany: Institut für Pflanzenernährung und Bodenkunde der CAU Kiel. Band 107.
Saha, D., Kukal, S. S., and Sharma, S. (2011). Landuse Impacts on SOC Fractions and Aggregate Stability in Typic Ustochrepts of Northwest India. Plant Soil 339, 457–470. doi:10.1007/s11104-010-0602-0
Scheffer, F., and Schachtschabel, P. (2018). Lehrbuch der Bodenkunde. Auflage 17. Heidelberg: Akademischer Verlag.
Schjønning, P., Stettler, M., Keller, T., Lassen, P., and Lamandé, M. (2015). Predicted Tyre-Soil Interface Area and Vertical Stress Distribution Based on Loading Characteristics. Soil Tillage Res. 152, 52–66. doi:10.1016/j.still.2015.03.002
Schlichting, E., Blume, H-P., and Stahr, K. (1966). Bodenkundliches Praktikum. Hamburg:Parey Verlag. doi:10.1002/jpln.19671160209
Schlichting, E., Blume, H-P., and Stahr, K. (1995). Bodenkundliches Praktikum. Berlin, Wien: Blackwell Wissenschafts-Verlag. 978-3-8274-2733-5.
Six, J., BossuytDegryze, H. S., Degryze, S., and Denef, K. (2004). A History of Research on the Link between (Micro)aggregates, Soil Biota, and Soil Organic Matter Dynamics. Soil Tillage Res. 79 (1), 7–31. doi:10.1016/j.still.2004.03.008
Smith, J., Smith, P., Wattenbach, M., Zaehle, S., Hiederer, R., Jones, R. J. A., et al. (2005). Projected Changes in mineral Soil Carbon of European Croplands and Grasslands, 1990-2080. Glob. Change Biol. 11, 2141–2152. doi:10.1111/j.1365-2486.2005.001075.x
Soane, B. D., and van Ouwerkerk, C. (1995). Implications of Soil Compaction in Crop Production for the Quality of the Environment. Soil Tillage Res. 35, 5–22. doi:10.1016/0167-1987(95)00475-8
Soane, B. D. (1990). The Role of Organic Matter in Soil Compactibility: A Review of Some Practical Aspects. Soil Tillage Res. 16, 179–201. doi:10.1016/0167-1987(90)90029-D
Stolbovoy, V., Montanarella, L., Filippi, N., Jones, A., Gallego, J., and Grassi, G. (2007). Soil Sampling Protocol to Certify the Changes of Organic Carbon Stock in Mineral Soil of the European Union. Luxembourg: Office for Official Publications of the European Communities.
The European Soil Framework Directive (2006). The European Soil Framework Directive. (Brussels: Commission of the European Communities). Available at: https://www.euronatur.org/fileadmin/migration/uploads/media/Info43_EU-Bodenschutzrichtlinie.pdf (Accessed September 22, 2006).
Trautner, A., van den Akker, J. J. H., van den Akker, J. J. H., Fleige, H., Arvidsson, J., and Horn, R. (2003). A Subsoil Compaction Database: its Development, Structure and Content. Soil Tillage Res. 73, 9–13. doi:10.1016/S0167-1987(03)00095-3
UBA (2004a). Ableitung von Kriterien zur Charakterisierung einer schädlichen Bodenveränderung, entstanden durch nutzungsbedingte Verdichtung von Böden/Regelungen zur Gefahrenabwehr. Berlin:Umweltbundesamt Hrsg., Texte 46/04, 122.0722-186X
Van den Akker, J. J. H., Arvidsson, J., and Horn, R. (1999). “Experiences with the impact and prevention of subsoil compaction in the European Community,” in Proceedings of the first workshop of the Concerted Action 'Experiences with the impact of subsoil compaction on soil, crop growth and environment and ways to prevent subsoil compaction (Wageningen, The Netherlands), 28–30. May 1998, 0927-4499
Watts, C. W., and Dexter, A. R. (1997). The Influence of Organic Matter in Reducing the Destabilization of Soil by Simulated Tillage. Soil Tillage Res. 42, 253–275. doi:10.1016/S0167-1987(97)00009-3
Wickham, H., François, R., Henry, L., and Müller, K. (2021). Dplyr: A Grammar of Data Manipulation. R package version 1.0.3. Available at: https://CRAN.R-project.org/package=dplyr.
Wiermann, C., Werner, D., Horn, R., Rostek, J., and Werner, B. (2000). Stress/strain Processes in a Structured Unsaturated Silty Loam Luvisol under Different Tillage Treatments in Germany. Soil Tillage Res. 53, 117–128. doi:10.1016/S0167-1987(99)00090-2
Wiermann, C. (1998). Auswirkungen Differenzierter Bodenbearbeitungen Auf Die Bodenstabilitat Und Das Regenerationsvermogen Losburtiger Ackerstandorte. PhD Thesis. Kiel: Christian-Albrechts-Universitat zu Kiel, Schriftenreihe Institut für Pflanzenernahrung und Bodenkunde. Band 45.
WRB, (2014). IUSS Working Group World Reference Base for Soil Resources, Update 2015: International Soil Classification System for Naming Soils and Creating Legends for Soil Maps. FAO, Rome: World Soil Resources Reports.
Zhang, H., Hartge, K. H., and Ringe, H. (1997). Effectiveness of Organic Matter Incorporation in Reducing Soil Compactibility. Soil Sci. Soc. Am. J. 61, 239–245. doi:10.2136/sssaj1997.03615995006100010033x
Zheng, H., Liu, W., Zheng, J., Luo, Y., Li, R., Wang, H., et al. (2018). Effect of Long-Term Tillage on Soil Aggregates and Aggregate-Associated Carbon in Black Soil of Northeast China. PLoS One 13, e0199523. doi:10.1371/journal.pone.0199523
Zink, A., Fleige, H., and Horn, R. (2010). Load Risks of Subsoil Compaction and Depths of Stress Propagation in Arable Luvisols. Soil Sci. Soc. Am. J. 74 (5), 1733–1742. doi:10.2136/sssaj2009.0336
Keywords: database, stress strain, precompression stress, saturated hydraulic conductivity, bulk density, organic matter, ecological properties, undisturbed soil profiles
Citation: Schroeder R, Fleige H, Hoffmann C, Vogel HJ and Horn R (2022) Mechanical Soil Database—Part I: Impact of Bulk Density and Organic Matter on Precompression Stress and Consequences for Saturated Hydraulic Conductivity. Front. Environ. Sci. 10:793625. doi: 10.3389/fenvs.2022.793625
Received: 12 October 2021; Accepted: 14 January 2022;
Published: 14 February 2022.
Edited by:
Thomas Keller, Swedish University of Agricultural Sciences, SwedenReviewed by:
Mario Rolim, Federal Rural University of Pernambuco, BrazilMiriam Fernanda Rodrigues, Federal Technological University of Paraná Dois Vizinhos, Brazil
Copyright © 2022 Schroeder, Fleige, Hoffmann, Vogel and Horn. This is an open-access article distributed under the terms of the Creative Commons Attribution License (CC BY). The use, distribution or reproduction in other forums is permitted, provided the original author(s) and the copyright owner(s) are credited and that the original publication in this journal is cited, in accordance with accepted academic practice. No use, distribution or reproduction is permitted which does not comply with these terms.
*Correspondence: Richard Schroeder, r.schroeder@soils.uni-kiel.de