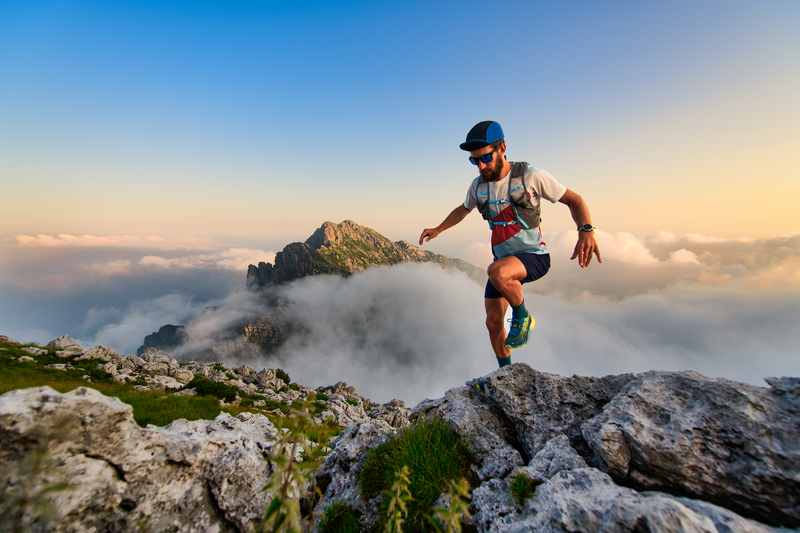
95% of researchers rate our articles as excellent or good
Learn more about the work of our research integrity team to safeguard the quality of each article we publish.
Find out more
MINI REVIEW article
Front. Environ. Sci. , 16 March 2021
Sec. Water and Wastewater Management
Volume 9 - 2021 | https://doi.org/10.3389/fenvs.2021.629767
This article is part of the Research Topic Frontiers in Environmental Science – Editor’s Picks 2021 View all 25 articles
Produced water (PW) generation has been increasing recently due to the expansion of fossil fuel extraction and the aging of oil wells worldwide, especially in the United States. The adverse health risks, seismicity, and environmental impacts associated with PW have become a challenging concern. Therefore, there is increased demand for improved PW treatment and reuse management options. There are multiple methods for treating PW; this article focuses on treatment through membrane filtration. Moreover, this mini review aims to summarize statistics on PW abundance and trends in PW generation over time, to briefly call attention to health-related issues, highlight some treatment challenges, and mention the potential purposes for reuse with an emphasis on the United States, the largest generator of PW worldwide.
Global energy demand has led to robust and widespread oil and gas industry with exploration and production activities around the world (Halvorsen, 1977; Cleveland, 2005; Caulk and Tomac, 2017). The environmental concerns associated with the diverse oil and gas extraction processes include threats to human and ecological health and contamination of water, air, and soil (Mondal and Wickramasinghe, 2008; Fakhru’l-Razi et al., 2009; Cordes et al., 2016; Mcintosh et al., 2018). Wastewater generated during the extraction of oil and gas is the largest waste stream in the oil and gas industry and is typically referred to as produced water (PW) (Veil, 2011; Dickhout et al., 2017; Al-Ghouti et al., 2019). PW generally contains substantial concentrations of petroleum components (such as phenols, polycyclic aromatic hydrocarbons, and volatile hydrocarbons), production chemicals (such as biocides and corrosion inhibitors), dissolved gases (such as CO2 and H2S), salts (such as sodium bicarbonate and sodium chloride), dissolved minerals including heavy metals (such as barium, zinc, lead, iron, etc.), and solids such as sand, silt, carbonates, and clays (Hansen and Davies, 1994; Duraisamy et al., 2013; Farag and Harper, 2014; Igunnu and Chen, 2014; Al-Haddabi et al., 2015).
Produced water can be categorized according to the extraction activity, for example, oilfield PW, natural gas PW, and coal bed methane PW (Veil et al., 2004; Igunnu and Chen, 2014; Al-Ghouti et al., 2019); of these three, oilfield PW contributes 60% of the total volume (Fakhru’l-Razi et al., 2009; Igunnu and Chen, 2014). Oilfield PW generally results from two different processes (Al-Ghouti et al., 2019): (I) as a result of oil diffusion into the seawater during the oil extraction (Bader, 2007; Al-Ghouti et al., 2019); and (II) due to the water injection to the oil field in order to push the oil rise to the surface (Puntervold and Austad, 2008; Al-Ghouti et al., 2019). Similarly, PW from a natural gas processing field is mainly made up of the fracturing fluid. This fluid is a water solution containing multiple chemicals used to assist the hydraulic fracturing operation (Chapman et al., 2012). Also, this PW usually contains large volume of brines (Chapman et al., 2012; Orem et al., 2014). In coalbed methane wells, the hydrostatic pressure caused by water does not allow the gas to be released from the coal; therefore, the water should be removed in order to be able to collect gas, resulting in the coal bed methane PW (Veil et al., 2004; Orem et al., 2014).
Improper management and control of PW can lead to the contamination of natural water resources as a result of oil spills or leaks (EPA, 2012; Esfahani et al., 2019). PW production is estimated to be more than 70 billion barrels annually (Al-Ghouti et al., 2019), and many of the associated contaminants are threats to marine life and human health (Neff, 2002; Chowdhury et al., 2004; Shakhawat et al., 2006; Mathieu et al., 2011; Beyer et al., 2012; Hosseini et al., 2012; Wilson and VanBriesen, 2012; Torres et al., 2016). High levels of organic and inorganic compounds have been reported in PW; these compounds are typically associated with shale gas wells (Torres et al., 2016). The level of these compounds varies based on the age of the reservoir, the type of produced hydrocarbon, and the local geology (Fakhru’l-Razi et al., 2009; Al-Ghouti et al., 2019). Dissolved organic carbon levels greater than 5.5 g/L have been reported for PW and include alkanes, carboxylic acids, aliphatic, and aromatic compounds with potential carcinogenic and other severe health effects (Torres et al., 2016). Animal studies indicate that PW-associated organic contaminants can cause respiratory complications in rats (Freeman et al., 2004; Torres et al., 2016), uterus complication in mice, and hemolytic anemia and adverse renal effects in animals (Vale and Meredith, 1981; ATSDR, 1999; Ismail et al., 2016; Alegbeleye et al., 2017), and respiratory complications and dysfunction of reproductive system in mice (Maguire-Boyle and Barron, 2014). Furthermore, volatile fatty acids (<53.7 mg/L) produced by bacteria in PW, cause unpleasant odors and have corrosive effects on skin with possible damage to eyes and nasal cavities (Maguire-Boyle and Barron, 2014; Orem et al., 2014). Furthermore, the inorganic contents of PW (Supplementary Table 1; Wilson and VanBriesen, 2012; Torres et al., 2016; Zhang et al., 2018) and their potential combination can cause health concerns (Warner et al., 2013). For example, if the bromide in PW is combined with disinfectant chlorine, it can yield brominated disinfection by-products that can cause brain, kidney, and liver damage (Brown, 2014; Vengosh et al., 2014). As another example, the interaction of the salt in the shale water with the surrounding rocks can mobilize radionuclides (Rich and Crosby, 2013). This results in a high concentration of radium-226 in some PW (Fisher, 1998; Osborn et al., 2011; Fan et al., 2016). Although water treatment approaches could reduce radioactive contamination by more than 90% (Rich and Crosby, 2013; Brown, 2014), chronic exposure to the remaining radium-226 contamination in drinking water can pose serious health risks such as cancer, anemia, and dental fractures (Rich and Crosby, 2013; Warner et al., 2013; Brown, 2014). Therefore, more investigation is required to identify more effective treatment methods for radioactive contaminants in PW.
Moreover, underground disposal of PW, the most widespread PW management strategy in the United States, has resulted in additional concerns such as induced seismicity (Scanlon et al., 2019). The state of Oklahoma is a particular example of observed seismic activity associated with subsurface injection sites (Rajesh and Gupta, 2021). Seismicity in Oklahoma has been linked statistically to PW volumes and PW injection rates (Van der Baan and Calixto, 2017; Roach, 2018; Scanlon et al., 2019). PW injection into disposal wells contributes to seismicity mostly by reducing normal stress which causes movement along a pre-existing fault inducing earthquakes (Murray and Holland, 2014; Rubinstein and Mahani, 2015). However, to induce felt earthquakes by injection, a combination of several factors is necessary such as being near an active fault, fault size, stress size, and fluid pressure (Capper and Lee, 2017). The injection risk factors in addition to health hazards and water demand further motivate the treatment and reuse of PW.
The ratio of water to oil in the process of oil extraction is reported to be around three to one (Veil, 2011; Dickhout et al., 2017; Al-Ghouti et al., 2019). However, the amount of PW generation increases as the oil well ages (Figure 1A; Igunnu and Chen, 2014; Kusworo et al., 2018). Some studies (Global Water Intelligence, 2011; McCabe, 2020) predict that this ratio will be 12 to one on average by 2025 considering crude oil reservoirs. Even in 2004, an average of 9.5:1 ratio (water:oil volume) in the United States has been reported (Veil et al., 2004; Veil and Clark, 2011). The PW volume could even reach 98% of extracted product in fields that are close to being depleted (McCabe, 2020).
Figure 1. (A) PW and oil production as a typical well ages [modified from Igunnu and Chen (2014)]. (B) Distribution of active shale plays across the United States with the ranges of PW total dissolved solids (TDS) concentrations [modified from Shaffer et al. (2013)]. (C) PW volumes and hydraulic fracturing water demand in 2017 and irrigation water demand in 2015 across the United States [modified from Scanlon et al. (2020)].
The United States is the largest generator of PW worldwide, reportedly producing about reported to be 24.4 billion barrels, ∼30% of total PW in the world (Veil, 2015; Dickhout et al., 2017; Al-Ghouti et al., 2019; Veil, 2020). There are around one million oil/gas wells in the United States which are producing different amounts of PW (Veil and Clark, 2011; Veil, 2015). Figure 1B indicates how oil and gas wells are distributed across the United States with the ranges of PW total dissolved solids (TDS) concentrations (Breit and Otton, 2002; Shaffer et al., 2013). Moreover, wells in the United States remain in production longer than the global average well (Veil and Clark, 2011). A comprehensive 10-year study by Veil et al. in three different articles has shown that PW generation in the United States has been increased by 16.2% between 2007 and 2017, with a particularly sharp increase in recent years (15.2% between 2012 and 2017) (Clark and Veil, 2009; Veil, 2015, 2020). Generation of PW in the United States in 2017 was dominated by three states, Texas, California, and Oklahoma, which generated 41, 13, and 12%, respectively, of the total (Veil, 2020). Conclusively, as the leading PW generator in the world, the United States is urged to put more effort into PW treatment and reuse.However, to reduce the potential health risks of PW spills and/or discharge, and to consider further reuse for different purposes, the aforementioned contaminations need to be investigated and lowered to acceptable levels (EPA, 2004). Here in this article, the aim is to discuss some reuse possibilities and examples, and review the application of membrane-based technologies for treating PW.
Reuse of treated PW has the potential to provide a useful water resource, particularly in water-scarce regions with increasing of oil and gas production (Echchelh et al., 2020; Gray, 2020; Nadella et al., 2020). The proposed uses of treated PW are diverse and include irrigation, habitat watering, livestock consumption, dust control, power generation, oil and gas field operations, and as an extreme example, drinking water (Echchelh et al., 2018; Al-Ghouti et al., 2019; Ginsberg et al., 2019; Scanlon et al., 2020). For instance, Figure 1C presents PW volumes and hydraulic fracturing water demand across the United States in 2017, in addition to water demand for irrigation in 2015 (Scanlon et al., 2020). Units are in billion gallons. This map includes Black Warrior, Powder River, San Juan, and Uinta basins as coal bed methane reservoirs, and Bakken, Barnett, Eagle Ford, Fayetteville, Haynesville, Marcellus, Niobrara, Oklahoma Area of Interest (AOI), and Permian (Delaware and Midland basins) as oil and gas reservoirs (Scanlon et al., 2020).
Depending on the intended application of treated water, different standards have been issued (Murray-Gulde et al., 2003; Uyttendaele et al., 2015). The United States Department of Agriculture (USDA) has provided such standards for irrigation and livestock consumption (Sirivedhin et al., 2004; Al-Ghouti et al., 2019). Additionally, regulations for treated water reuse as drinking water are generally provided by the United States Environmental Protection Agency (EPA) (Sirivedhin et al., 2004; Al-Ghouti et al., 2019). Supplementary Table 2 demonstrates the standards recommended for reuse in irrigation, livestock consumption, and drinking (Hildenbrand et al., 2018; Al-Ghouti et al., 2019). Recommendations for drinking water are necessarily stricter and therefore it requires more substantial treatment of PW. In contrast, little treatment is needed for PW reuse in oil and gas industry and dust controlling operations (Al-Ghouti et al., 2019). Pondering different applications, a factor that determines obtainable options and plays an important role in considering reuse is expense efficiency (Bagheri et al., 2018), which is very site-specific (Echchelh et al., 2018).
Reaching specified standards after the treatment, PW reuse for crop irrigation in arid and semi-arid areas has been recommended (Sirivedhin et al., 2004; Echchelh et al., 2018), especially for crops that are salt-tolerant (Gray, 2020). It also helps to preserve freshwater resources in the region (Echchelh et al., 2018, 2020). However, it is pivotal what crop type is determined to be irrigated by treated PW due to several challenges, largely due to the high content of TDS, including various salts and sodium (Drewes et al., 2009; Echchelh et al., 2018). For instance, the impact of PW on biofuel crops has been investigated in a case study of treated PW reuse for irrigation in the United States (Pica et al., 2017). The water used in this study contained ∼2 g/L total organic carbon (TOC) and ∼20 g/L TDS and was taken from a central processing facility which supplies ∼500 wells in the Denver–Julesburg Basin (Pica et al., 2017). The outcome of this study indicates that achieving 3.5 g/L TDS is necessary for the treated water to be considered for irrigation (Pica et al., 2017). Moreover, it is concluded that TOC concentration of <5 mg/l is needed to keep a viable biofuel crops production rate (Pica et al., 2017).
The water consumed by animals also requires some standards to avoid adverse impacts on their health (Embry et al., 1959; Faries and Loneragan, 2007). However, these standards are usually less strict compared to human ingestion standards, and many livestock are more tolerant (Al-Ghouti et al., 2019). Salinity and TDS tolerance vary among different species (Alam et al., 2017; Arora and Dagar, 2019), but generally livestock can be watered with a source containing 1 g/L of TDS (Veil et al., 2004; Al-Ghouti et al., 2019). Moreover, moderately treated PW with confirmed harmlessness based on water quality regulations can be used as a source of drinking water for wildlife and also a habitat for water birds and fish species (Sirivedhin et al., 2004). In the United States state of Wyoming, PW with TDS concentration of <5 g/L can be discharged to surface waters in order to be used by livestock and wildlife (Ramirez, 2002; Gray, 2020). This PW with low salinity is primarily from coal bed methane operations, and the aforementioned discharge is subject to permit requirements (Ramirez, 2002). However, due to uncertainties in the water quality, some studies do not support PW reuse other than in the energy sector (Scanlon et al., 2020). The two most common applications for reusing PW in the energy sector are enhanced oil recovery (EOR) and makeup water used for hydraulic fracturing fluid (Khatib, 2007; Graham et al., 2015; Arora and Dagar, 2019); for these applications, high salt concentrations do not preclude use, and thus, only minimal PW treatment may be required for reusage (Webb and Zodrow, 2020). PW reuse for hydraulic fracturing requires efficient removal of solids and iron (≤10 mg/L) (Munirasu et al., 2016; Nadella et al., 2020); in addition, it is necessary to inactivate microorganisms to reduce the chance of well clogging and damage (Barnes et al., 2015). For EOR use, filtration may be implemented to remove further targeted particles (Shams Ashaghi et al., 2007; Buono et al., 2019).
As with other liquid waste streams, the options for PW include proper treatment and discharge, beneficial reuse, or some combination of these (Shaffer et al., 2013; McLaughlin et al., 2020). Because contaminants and concentrations in PW vary, physical, chemical, and biological treatment processes may be required (Fakhru’l-Razi et al., 2009). There are generally three core steps in PW treatment: pre-treatment, main treatment, and post-treatment (Al-Ghouti et al., 2019). It is essential to select a treatment approach based on efficiency, selectivity, and cost. Additionally, many PW treatment operations must take place in settings (e.g., offshore) with severe restrictions on space, weight of the equipment, durability, and ability to operate in harsh environments (Arthur et al., 2011; Dolan et al., 2018). Widely studied techniques for PW treatment include adsorption (Al-Ghouti et al., 2019), chemical precipitation (Blumenschein and Banerjee, 2005; Fakhru’l-Razi et al., 2009), and membrane filtration (Dickhout et al., 2017). Briefly, chemical precipitation is a method to separate a substance from a solution either by converting the substance into an insoluble form or by altering the solvent composition to decrease the solubility of the substance (Firouzjaei et al., 2020a; Peng and Guo, 2020). Adsorption is the adhesion of dissolved constituents to the surface of an insoluble interface, which leads to formation of a thin film creation on the adsorbent surface (Fathy et al., 2018; Firouzjaei et al., 2020b). This article focuses on membrane treatment of PW.
Membrane filtration is a physical separation technique which selectively fractionates components from a flowing substance via pores in a continuous structure (Zirehpour et al., 2016; Firouzjaei et al., 2018a; Rahimpour et al., 2018; Mozafari et al., 2019). Membrane separations are categorized by the driving force and the pore size of applied materials (Xu et al., 2008; Pejman et al., 2020a). In addition to drinking water and wastewater treatment, there are many other applications associated with this technology in diverse industries including the petrochemical (Ravanchi et al., 2009), pharmaceutical (Firouzjaei et al., 2018b; Seyedpour et al., 2020a), and food industries (Pabby et al., 2015). There are different types of membrane materials and membrane processes applied to many applications, but the main goal is always the same: separation. The most common membrane materials are ceramic (Abadi et al., 2011), metal (Li et al., 2020), and polymer (Seyedpour et al., 2020b). Membranes can be classified based on the separation processes.
Microfiltration (MF) (Yang et al., 1998), ultrafiltration (UF) (Ma et al., 2017), nanofiltration (NF) (Anand et al., 2018), and reverse osmosis (RO) (Yang et al., 2019) are four main pressure-driven membrane processes (Van der Bruggen et al., 2003). They can also be categorized as porous (mainly MF and UF) and non-porous (mainly RO and NF) membranes (El-Samak et al., 2020; Zhao et al., 2020). In addition, forward osmosis (FO) is an osmotic pressure-driven membrane process (Pejman et al., 2020b). An ideal membrane for a specific separation purpose should have sufficient mechanical resistance, high flux, and high selectivity (Judd and Jefferson, 2003), with reasonable capital and operating costs. The mechanical resistance is dependent on material and the thickness (Elshorbagy and Chowdhury, 2013; Vajner et al., 2016). High flux can be achieved largely through high porosity and/or large pore sizes. In contrast, high selectivity can be achieved by having small pores with a narrow range of sizes. Therefore, there is usually a trade-off between flux and selectivity (Judd and Jefferson, 2003; Park et al., 2017; Moon et al., 2020). In addition, there is generally an inverse relationship between membrane thickness (number of layers) and flux (Shirazi et al., 2014) which suggests a general trade-off between mechanical resistance and flux. PW treatment using membranes is generally intended to remove contaminants with the size range of 1 nm–1 μm from the feed (Ebrahimi et al., 2010; Alkhudhiri et al., 2013; Dickhout et al., 2017). Ceramic membranes have attracted major interest due to their extreme thermal stability, mechanical stability, and their compatibility with harsh cleaning methods (i.e., intensifying the cleaning regimes) (Fakhru’l-Razi et al., 2009; Aziz et al., 2019; Ebrahimi et al., 2020). Periodic cleanings are cycles of chemical soaks and backwashes to remove foulants from the membrane surface and are required in any membrane system. Ceramic membranes endurance to higher pressures results in more efficient backwashing (Freeman and Shorney-Darby, 2011). Furthermore, the combination of a wider tolerance of pressure and cleaning chemicals lead to yield more stable permeability in ceramic membranes. For instance, a sustainable flux of 700 with a permeability of 1,400 has been reported by using silicon carbide MF ceramic membranes, fed by PW from an oilfield in the Persian Gulf (Zsirai et al., 2018). Moreover, more than 99% oil removal has been accomplished by using commercial α-alumina MF ceramic membranes (0.8 μm pore size), fed by synthetic PW containing 250–1,000 ppm concentrations of heavy crude oil droplets of 1–10 micron diameter (Mueller et al., 1997; Al-Ghouti et al., 2019); in all these experiments, the permeate contained <2 ppm total hydrocarbons (Mueller et al., 1997). Polymeric and composite membranes are generally less expensive than ceramic membranes, and their performance has been studied in several research studies (Alzahrani and Mohammad, 2014; Lee et al., 2015; Dickhout et al., 2017). Table 1 compares the membrane-based technologies utilized for the treatment of PW, highlighting their advantages and downsides (Igunnu and Chen, 2014; Al-Ghouti et al., 2019). Most PW contaminants can be removed efficiently with membrane treatment; for example, total suspended solids (TSS), oil and grease (O&G), and sulfate have been shown to be highly removed in PW treatment (Mondal and Wickramasinghe, 2008; Alzahrani and Mohammad, 2014; Al-Ghouti et al., 2019). For example, >99.9% removal of these three contaminants has been reported for NF followed by RO using commercially available polyamide thin film composite (PA-TFC) NF and low-pressure RO membranes, including NF270 (an NF membrane for salt rejection application) or BW30 (an RO membrane for brackish water treatment application) (Mondal and Wickramasinghe, 2008; Ozgun et al., 2013; Alzahrani and Mohammad, 2014; Al-Ghouti et al., 2019).
Table 1. The membrane technologies used for PW treatment and comparison of their properties [modified from Igunnu and Chen (2014)].
Applying non-pressure driven membrane processes such as membrane distillation (MD) is another separation approach (Wang and Chung, 2015). MD is a thermally driven membrane process and is based on the vapor pressure gradient and vapor transport across the membrane (Macedonio et al., 2014; Wang and Chung, 2015). There are many different MD techniques such as air gap MD (Meindersma et al., 2006), sweeping gas MD (Shirazi et al., 2014), and permeate gap MD (Shirazi et al., 2014). A process which has lately been considered for PW treatment is the direct contact membrane distillation (DCMD) (Ali et al., 2018; Anari et al., 2019; Zou et al., 2020). DCMD is a membrane process in which the aqueous feed and permeate are kept in different temperatures while in contact with a hydrophobic membrane which hinders the liquid permeation but allows the vapor to pass (Lawson and Lloyd, 1996; Ashoor et al., 2016); the temperature difference leads to a vapor pressure difference that drives the flux through the membrane from hot feed to the cold permeate (Lawson and Lloyd, 1996; Ashoor et al., 2016). Through DCMD, TDS can be removed by fabricated hollow fiber membranes with the ratio of >99% which sustains even for 100 h of operation (Ali et al., 2018; Zou et al., 2020).
It is also shown that pre-treatment techniques can improve membrane performance and reduce costs (Hilal et al., 2003; Padaki et al., 2015; Kusworo et al., 2018). For instance, the lifetime, flux, and anti-fouling properties of membranes can be improved by using adsorbents at the pre-treatment phase (Kusworo et al., 2018). Another example of pre-treatment is using bioreactors (Huang et al., 2020). For some classes of oil and gas industry wastewater including oil-gas field PW and petrochemical wastewater, bioreactors can be a very efficient approach to reducing chemical oxygen demand (COD), with reported reductions of 80% for a PW with a COD range of 1,710–3,030 mg/L (Ozgun et al., 2013; Huang et al., 2020). Regarding COD reduction, bioreactors may provide superior performance as pretreatment for RO; pretreatment with MF and UF membranes has been reported to yield <25% reduction of COD (Ozgun et al., 2013; Al-Ghouti et al., 2019).
Membrane filtration is an efficient technique to remove TDS from PW (Çakmakce et al., 2008; Strong et al., 2017; Venkatesan and Wankat, 2017; Atoufi and Lampert, 2020) due to the following reasons: (1) the energy consumption and cost are relatively low [82–84], (2) rejection rates are relatively high (Webb and Zodrow, 2020), and (3) adding less chemicals is required through the process (Webb and Zodrow, 2020). Generally, MF and UF membranes remove a considerable fraction of solids and can serve as pretreatment for NF, RO, or FO techniques in order to reach desired TDS rejection (Atoufi and Lampert, 2020). For example, 95% removal of TDS from PW has been reported by implementing RO (Funston et al., 2002; Atoufi and Lampert, 2020). Specifically, membrane distillation and FO are reported to be very promising for treating PW with high TDS concentration due to the fact that these two techniques use temperature and concentration gradients, respectively, instead of pressure gradient (Coday et al., 2014; Duong et al., 2015; Webb and Zodrow, 2020). However, pressure-driven membranes processes like RO are impractical for treatment of very high salinity (e.g., >35 g/L) PW (Shaffer et al., 2013); because the hydraulic pressure needed to overcome the osmotic pressure of high-salinity waters can be over the allowable pressure of the RO membrane modules, desalination of high-salinity PW with TDS concentrations higher than ∼35 g/L requires more energy intensive technologies (Greenlee et al., 2009; Shaffer et al., 2013). Unlike RO, FO is driven by osmotic pressure rather than hydraulic pressure, and is not restricted by the high-pressure operating limitation associated with TDS concentration as high as 70 g/L (Martinetti et al., 2009). Also, low-pressure NF has been practical for removing ions like sulfate and calcium (Fakhru’l-Razi et al., 2009; Webb and Zodrow, 2020). High heavy metal concentrations are an important concern for many PWs, as many heavy metals have major adverse health effects (Supplementary Table 3; Fakhru’l-Razi et al., 2009; Tchounwou et al., 2012; Atoufi and Lampert, 2020); NF and RO have been reported to be successful in treating feed water containing multiple heavy metals; reported results indicate an average removal efficiency of 97 and 99% for NF and RO, respectively (Qdais and Moussa, 2004).
In summary, membrane-based treatment of PW has shown success across many contaminants and applications. Although membrane filtration technologies are very effective, desalination of PW with high salinity (>70 g/L) still has room for improvement (Ahmad et al., 2020). Hybrid treating systems, i.e., combination of two or more membrane processes, show more efficacy for treating PW (Murray-Gulde et al., 2003; Ahmad et al., 2020). For example, a four-step method has recently been proposed by Atoufi and Lampert (2020) that is focused on removing salts from PW. The first step is called feed softening and is purposed to lower the fouling chances during the membrane processes by adding calcium hydroxide to the sample; large suspended solids can be removed by sand filtration which counts as the second step; the third step targets O&G to be removed and is based on ceramic membranes; using RO technique with PA-TFC membrane is the last step (Atoufi and Lampert, 2020). To boost the capabilities of hybrid systems and reduce the cost and energy, it is crucial to consider a right combination of pre-, main stage, and post-treatments based on the treatment purpose (Ahmad et al., 2020). Hence, thorough understanding is required to achieve the optimized hybrid system for any case.
Due to the environment and health concerns caused by PW discharge and/or leakage, the oil and gas industry is attempting to find better approaches to manage PW. Considering required standards, recycled PW reuse can be partly a great solution to reduce demand for freshwater. PW is being treated and reused in oil and gas field operations such as drilling and EOR. As demand for water increases globally, more governments have started to show interest in PW reuse outside of the oil and gas industry applications (Zolghadr, 2016; McLaughlin et al., 2020). Examples are irrigation, livestock consumption, wildlife watering, and, as an extreme example, drinking water. Regarding health concerns, these ideas of PW reusage require very cautious and responsible consideration of the potential advantages and downsides in a case-by-case manner. What took place in Pennsylvania, where water supplies were polluted by PW which was not properly treated (Webb and Zodrow, 2020), emphasizes the need for careful consideration how to handle PW treatment and reuse. On other hand, this experience also suggests that increasing restrictions on disposal can encourage reuse (Behl et al., 2018; Webb and Zodrow, 2020). A successful example is reusing the treated PW from fields with low concentration of TDS (∼750 mg/L), located in Kern County, California (Gray, 2020). This treated PW is used for irrigating by local districts after it is blended with other fresh water supplies. Additionally, PW reuse in drilling operations in the Permian Basin by the Apache Corporation is another successful example of PW reusage in the United States (US Energy Information Administration, 2016). Notably, Apache Corporation has constructed six recycling systems in the west of Texas since 2016 in order to treat around 90% of PW for drilling operations (Behl et al., 2018; Gray, 2020).
It is predicted that the average ratio of water to oil in the process of oil extraction will be 12 to one by 2025 (Global Water Intelligence, 2011; McCabe, 2020). This ratio is approximately four times larger compared to the average ratios reported in the last decade (Veil, 2011; Dickhout et al., 2017; Al-Ghouti et al., 2019). Even in 2004, an average ratio of 9.5–1 has been reported in the United States (Veil et al., 2004; Veil and Clark, 2011). Moreover, the amount of generated PW increases as the oil well ages. The PW volume could even reach 98% of extracted product in fields that are about to being depleted (McCabe, 2020). As a result, PW characterization, treatment, and reuse are expected to attract more attention in the market, and more guidelines are needed for this significant potential. As the largest contributor in the PW generation worldwide, the United States has a great role to investigate different potential reuse purposes and accordingly different techniques for PW treatment.
EZ contributed to the formal analysis, investigation, visualization, and writing–original draft. MDF contributed to the conceptualization, validation, supervision, project administration, and writing–review and editing. GA contributed to the investigation and writing–original draft. PL contributed to the resources, validation, supervision, funding acquisition, and writing–review and editing. ME contributed to the resources, validation, supervision, funding acquisition, project administration, and writing–review and editing. All authors contributed to the article and approved the submitted version.
The authors declare that the research was conducted in the absence of any commercial or financial relationships that could be construed as a potential conflict of interest.
The Supplementary Material for this article can be found online at: https://www.frontiersin.org/articles/10.3389/fenvs.2021.629767/full#supplementary-material
Abadi, S. R. H., Sebzari, M. R., Hemati, M., Rekabdar, F., and Mohammadi, T. (2011). Ceramic membrane performance in microfiltration of oily wastewater. Desalination 265, 222–228. doi: 10.1016/j.desal.2010.07.055
Ahmad, N. A., Goh, P. S., Yogarathinam, L. T., Zulhairun, A. K., and Ismail, A. F. (2020). Current advances in membrane technologies for produced water desalination. Desalination 493:114643. doi: 10.1016/j.desal.2020.114643
Alam, M. Z., Carpenter-Boggs, L., Mitra, S., Haque, M., Halsey, J., Rokonuzzaman, M., et al. (2017). Effect of salinity intrusion on food crops, livestock, and fish species at Kalapara Coastal Belt in Bangladesh. J. Food Qual. 2017, 1–23. doi: 10.1155/2017/2045157
Alegbeleye, O. O., Opeolu, B. O., and Jackson, V. A. (2017). Polycyclic aroma- tic hydrocarbons: a critical review of environmental occurrence and bio- remediation. Environ. Manag. 60, 758–783. doi: 10.1007/s00267-017-0896-2
Al-Ghouti, M. A., Al-Kaabi, M. A., Ashfaq, M. Y., and Da’na, D. A. (2019). Produced water characteristics, treatment and reuse: a review. J. Water Process Eng. 28, 222–239. doi: 10.1016/j.jwpe.2019.02.001
Al-Haddabi, M., Vuthaluru, H., Ahmed, M., and Znad, H. (2015). “Use of ceramic membrane technology for sustainable management of oil production water: a review,” in Recent Progress in Desalination, Environmental and Marine Outfall Systems, eds M. Baawain, B. Choudri, M. Ahmed, and A. Purnama (Cham: Springer), 11–23. doi: 10.1007/978-3-319-19123-2_2
Ali, A., Quist-Jensen, C. A., Drioli, E., and Macedonio, F. (2018). Evaluation of integrated microfiltration and membrane distillation/crystallization processes for produced water treatment. Desalination 434, 161–168. doi: 10.1016/j.desal.2017.11.035
Alkhudhiri, A., Darwish, N., and Hilal, N. (2013). Produced water treatment: application of air gap membrane distillation. Desalination 309, 46–51. doi: 10.1016/j.desal.2012.09.017
Alzahrani, S., and Mohammad, A. W. (2014). Challenges and trends in membrane technology implementation for produced water treatment: a review. J. Water Process Eng. 4, 107–133. doi: 10.1016/j.jwpe.2014.09.007
Anand, A., Unnikrishnan, B., Mao, J.-Y., Lin, H.-J., and Huang, C.-C. (2018). Graphene-based nanofiltration membranes for improving salt rejection, water flux and antifouling–A review. Desalination 429, 119–133. doi: 10.1016/j.desal.2017.12.012
Anari, Z., Sengupta, A., Sardari, K., and Wickramasinghe, S. R. (2019). Surface modification of PVDF membranes for treating produced waters by direct contact membrane distillation. Sep. Purif. Technol. 224, 388–396. doi: 10.1016/j.seppur.2019.05.032
Arora, S., and Dagar, J. C. (2019). “Salinity tolerance indicators,” in Research Developments in Saline Agriculture, eds J. Dagar, R. Yadav, and P. Sharma (Singapore: Springer), 155–201. doi: 10.1007/978-981-13-5832-6_5
Arthur, J. D., Hochheiser, W. H., Bottrell, M. D., Brown, A., Candler, J., Cole, L., et al. (2011). “Management of produced water from oil and gas wells,” in Working Document of the NPC North American Resource Development Study paper 2–17 (Washington D.C, USA: NPC North American Resource Development Study).
Ashoor, B. B., Mansour, S., Giwa, A., Dufour, V., and Hasan, S. W. (2016). Principles and applications of direct contact membrane distillation (DCMD): a comprehensive review. Desalination 398, 222–246. doi: 10.1016/j.desal.2016.07.043
Atoufi, H. D., and Lampert, D. J. (2020). “Membrane desalination to prepare produced water for reuse,” in World Environmental and Water Resources Congress 2020: Water, Wastewater, and Stormwater and Water Desalination and Reuse, eds S. Ahmad and R. Murray (Reston, VA: American Society of Civil Engineers), 8–15. doi: 10.1061/9780784482988.002
Aziz, M. H. A., Othman, M. H. D., Hashim, N. A., Rahman, M. A., Jaafar, J., Hubadillah, S. K., et al. (2019). Pretreated aluminium dross waste as a source of inexpensive alumina-spinel composite ceramic hollow fibre membrane for pretreatment of oily saline produced water. Ceram. Int. 45, 2069–2078. doi: 10.1016/j.ceramint.2018.10.110
Bader, M. S. H. (2007). Seawater versus produced water in oil-fields water injection operations. Desalination 208, 159–168. doi: 10.1016/j.desal.2006.05.024
Bagheri, M., Roshandel, R., and Shayegan, J. (2018). Optimal selection of an integrated produced water treatment system in the upstream of oil industry. Process Saf. Environ. Prot. 117, 67–81. doi: 10.1016/j.psep.2018.04.010
Barnes, C. M., Marshall, R., Mason, J., Skodack, D., DeFosse, G., Smith, D. G., et al. (2015). “The new reality of hydraulic fracturing: treating produced water is cheaper than using fresh,” in SPE Annual Technical Conference and Exhibition, (Richardson, TX: Society of Petroleum Engineers). doi: 10.2118/174956-MS
Behl, N., Bohm, B., Bruton, M., Fleming, S., and Vance, S. (2018). “Case study: Apache’s approach to societal responsibility thru the environmental media monitoring program for the alpine high play,” in SPE Annual Technical Conference and Exhibition, (Richardson, TX: Society of Petroleum Engineers). doi: 10.2118/191589-MS
Beyer, J., Myhre, L. P., Sundt, R. C., Meier, S., Tollefsen, K.-E., Vabø, R., et al. (2012). Environmental risk assessment of alkylphenols from offshore produced water on fish reproduction. Mar. Environ. Res. 75, 2–9. doi: 10.1016/j.marenvres.2011.11.011
Blumenschein, C. D., and Banerjee, K. (2005). Method of Treating Water and Wastewater with A Ballasted Flocculation Process and A Chemical Precipitation Process. United States Patent, Patent No.: US 6,919,031 B2.
Breit, G. N., and Otton, J. K. (2002). Produced Waters Database. Reston, VA: United States Geological Survey.
Brown, V. J. (2014). Radionuclides in fracking wastewater: managing a ToxicBlend. Environ. Health Perspect. 122, A50–A55. doi: 10.1289/ehp.122-A50
Buono, R. M., Gunn, E. L., McKay, J., and Staddon, C. (2019). Regulating Water Security in Unconventional Oil and Gas. Berlin: Springer. doi: 10.1007/978-3-030-18342-4
Çakmakce, M., Kayaalp, N., and Koyuncu, I. (2008). Desalination of produced water from oil production fields by membrane processes. Desalination 222, 176–186. doi: 10.1016/j.desal.2007.01.147
Capper, L. H., and Lee, M. C. (2017). “US Class II underground injection wells: injection and seismicity operational risk factors,” in SPE Eastern Regional Meeting, (Richardson, TX: Society of Petroleum Engineers). doi: 10.2118/187522-MS
Caulk, R. A., and Tomac, I. (2017). Reuse of abandoned oil and gas wells for geothermal energy production. Renew. Energy 112, 388–397. doi: 10.1016/j.renene.2017.05.042
Chapman, E. C., Capo, R. C., Stewart, B. W., Kirby, C. S., Hammack, R. W., Schroeder, K. T., et al. (2012). Geochemical and strontium isotope characterization of produced waters from Marcellus Shale natural gas extraction. Environ. Sci. Technol. 46, 3545–3553. doi: 10.1021/es204005g
Chowdhury, S., Husain, T., Veitch, B., Bose, N., and Sadiq, R. (2004). Human health risk assessment of naturally occurring radioactive materials in produced water—A case study. Hum. Ecol. Risk Assess. 10, 1155–1171. doi: 10.1080/10807030490887203
Clark, C. E., and Veil, J. A. (2009). Produced Water Volumes and Management Practices in the United States. Argonne, IL: Argonne National Lab. doi: 10.2172/1007397
Cleveland, C. J. (2005). Net energy from the extraction of oil and gas in the United States. Energy 30, 769–782. doi: 10.1016/j.energy.2004.05.023
Coday, B. D., Xu, P., Beaudry, E. G., Herron, J., Lampi, K., Hancock, N. T., et al. (2014). The sweet spot of forward osmosis: treatment of produced water, drilling wastewater, and other complex and difficult liquid streams. Desalination 333, 23–35. doi: 10.1016/j.desal.2013.11.014
Cordes, E. E., Jones, D. O. B., Schlacher, T. A., Amon, D. J., Bernardino, A. F., Brooke, S., et al. (2016). Environmental impacts of the deep-water oil and gas industry: a review to guide management strategies. Front. Environ. Sci. 4:58. doi: 10.3389/fenvs.2016.00058
Dickhout, J. M., Moreno, J., Biesheuvel, P. M., Boels, L., Lammertink, R. G. H., and de Vos, W. M. (2017). Produced water treatment by membranes: a review from a colloidal perspective. J. Colloid Interface Sci. 487, 523–534. doi: 10.1016/j.jcis.2016.10.013
Dolan, F. C., Cath, T. Y., and Hogue, T. S. (2018). Assessing the feasibility of using produced water for irrigation in Colorado. Sci. Total Environ. 640, 619–628. doi: 10.1016/j.scitotenv.2018.05.200
Drewes, J. E., Cath, T. Y., Xu, P., Graydon, J., Veil, J., and Snyder, S. (2009). An Integrated Framework for Treatment and Management of Produced Water. RPSEA Project 07112-22. Houston, TX: RPSEA.
Duong, H. C., Chivas, A. R., Nelemans, B., Duke, M., Gray, S., Cath, T. Y., et al. (2015). Treatment of RO brine from CSG produced water by spiral-wound air gap membrane distillation—A pilot study. Desalination 366, 121–129. doi: 10.1016/j.desal.2014.10.026
Duraisamy, R. T., Beni, A. H., and Henni, A. (2013). “State of the art treatment of produced water,” in Water Treatment, (Rijeka: InTech), 199–222.
Ebrahimi, M., Schmidt, A. A., Kaplan, C., Schmitz, O., and Czermak, P. (2020). Innovative optical-sensing technology for the online fouling characterization of silicon carbide membranes during the treatment of oily water. Sensors 20:1161. doi: 10.3390/s20041161
Ebrahimi, M., Willershausen, D., Ashaghi, K. S., Engel, L., Placido, L., Mund, P., et al. (2010). Investigations on the use of different ceramic membranes for efficient oil-field produced water treatment. Desalination 250, 991–996. doi: 10.1016/j.desal.2009.09.088
Echchelh, A., Hess, T., and Sakrabani, R. (2018). Reusing oil and gas produced water for irrigation of food crops in drylands. Agric. Water Manag. 206, 124–134. doi: 10.1016/j.agwat.2018.05.006
Echchelh, A., Hess, T., and Sakrabani, R. (2020). Agro-environmental sustainability and financial cost of reusing gasfield-produced water for agricultural irrigation. Agric. Water Manag. 227, 105860. doi: 10.1016/j.agwat.2019.105860
El-Samak, A. A., Ponnamma, D., Hassan, M. K., Ammar, A., Adham, S., Al-Maadeed, M. A. A., et al. (2020). Designing flexible and porous fibrous membranes for oil water separation—A review of recent developments. Polym. Rev. 60, 671–716. doi: 10.1080/15583724.2020.1714651
Elshorbagy, W., and Chowdhury, R. (2013). Water treatment. BoD–Books on Demand (Rijeka, Croatia: InTech), doi: 10.5772/2883
Embry, L. B., Hoelscher, M. A., Wahlstrom, R. C., and Carlson, C. W. (1959). Salinity and livestock water quality (Brookings, South Dakota: South Dakota Agricultural Experiment Station Bulletin).
EPA (2012). Study of the Potential Impacts of Hydraulic Fracturing on Drinking Water Re- sources Progress Report. Washington, DC: EPA.
Esfahani, M. R., Aktij, S. A., Dabaghian, Z., Firouzjaei, M. D., Rahimpour, A., Eke, J., et al. (2019). Nanocomposite membranes for water separation and purification: fabrication, modification, and applications. Sep. Purif. Technol. 213, 465–499. doi: 10.1016/j.seppur.2018.12.050
Fakhru’l-Razi, A., Pendashteh, A., Abdullah, L. C., Biak, D. R. A., Madaeni, S. S., and Abidin, Z. Z. (2009). Review of technologies for oil and gas produced water treatment. J. Hazard. Mater. 170, 530–551. doi: 10.1016/j.jhazmat.2009.05.044
Fan, W., Liberati, B., Novak, M., Cooper, M., Kruse, N., Young, D., et al. (2016). Radium-226 removal from simulated produced water using natural zeolite and ion-exchange resin. Ind. Eng. Chem. Res. 55, 12502–12505. doi: 10.1021/acs.iecr.6b03230
Farag, A. M., and Harper, D. D. (2014). A review of environmental impacts of salts from produced waters on aquatic resources. Int. J. Coal Geol. 126, 157–161. doi: 10.1016/j.coal.2013.12.006
Faries, F. C., and Loneragan, F. C. G. H. (2007). “Livestock water quality standards,” in Encyclopedia of Water Science, eds B. A. Stewart and T. Howell (Boca Raton, FL: CRC Press), 727–730. doi: 10.1201/NOE0849396274.ch172
Fathy, M., El-Sayed, M., Ramzi, M., and Abdelraheem, O. H. (2018). Adsorption separation of condensate oil from produced water using ACTF prepared of oil palm leaves by batch and fixed bed techniques. Egypt. J. Pet. 27, 319–326. doi: 10.1016/j.ejpe.2017.05.005
Firouzjaei, M. D., Afkhami, F. A., Esfahani, M. R., Turner, C. H., and Nejati, S. (2020a). Experimental and molecular dynamics study on dye removal from water by a graphene oxide-copper-metal organic framework nanocomposite. J. Water Process Eng. 34, 101180. doi: 10.1016/j.jwpe.2020.101180
Firouzjaei, M. D., Seyedpour, S. F., Aktij, S. A., Giagnorio, M., Bazrafshan, N., Mollahosseini, A., et al. (2020b). Recent advances in functionalized polymer membranes for biofouling control and mitigation in forward osmosis. J. Memb. Sci. 596:117604. doi: 10.1016/j.memsci.2019.117604
Firouzjaei, M. D., Shamsabadi, A. A., Aktij, S. A., Seyedpour, S. F., Sharifian Gh, M., Rahimpour, A., et al. (2018a). Exploiting synergetic effects of graphene oxide and a silver-based metal–organic framework to enhance antifouling and anti-biofouling properties of thin-film nanocomposite membranes. ACS Appl. Mater. Interfaces 10, 42967–42978. doi: 10.1021/acsami.8b12714
Firouzjaei, M. D., Shamsabadi, A. A., Gh, M. S., Rahimpour, A., and Soroush, M. (2018b). A novel nanocomposite with superior antibacterial activity: a silver−based metal organic framework embellished with graphene oxide. Adv. Mater. Interfaces 5:1701365. doi: 10.1002/admi.201701365
Fisher, R. S. (1998). Geologic and geochemical controls on naturally occurring radioactive materials (NORM) in produced water from oil, gas, and geothermal operations. Environ. Geosci. 5, 139–150. doi: 10.1046/j.1526-0984.1998.08018.x
Freeman, C., Fenner, N., Ostle, N. J., Kang, H., Dowrick, D. J., Reynolds, B., et al. (2004). Export of dissolved organic carbon from peatlands under elevated carbon dioxide levels. Nature 430, 195–198. doi: 10.1038/nature02707
Freeman, S., and Shorney-Darby, H. (2011). What’s the buzz about ceramic membranes? J. Am. Water Work. Assoc. 103, 12–13. doi: 10.1002/j.1551-8833.2011.tb11572.x
Funston, R., Ganesh, R., and Leong, L. Y. C. (2002). “Evaluation of technical and economic feasibility of treating oilfield produced water to create a “new” water resource,” in Proceedings of the Ground Water Protection Council (GWPC) Meeting, (Colorado Springs, CO).
Ginsberg, G., Liew, Z., Pollitt, K., Deziel, N., Whirledge, S., Anastas, P., et al. (2019). Yale Symposium on Per−and Polyfluoroalkyl Substances (PFAS): Challenges and Opportunities December 13, 2019. New Haven, CT: Yale School of Public Health.
Global Water Intelligence (2011). Produced Water Market: Opportunities in the Oil, Shale and Gas Sectors in North America. Oxford: Global Water Intelligence.
Graham, E. J. S., Chu, S., and Pawar, R. J. (2015). Probabilistic cost estimation methods for treatment of water extracted during CO2 storage and EOR. Int. J. Greenh. Gas Control 41, 316–327. doi: 10.1016/j.ijggc.2015.07.026
Gray, M. (2020). “Reuse of produced water in the oil and gas industry,” in Proceedings of the SPE International Conference and Exhibition on Health, Safety, Environment, and Sustainability, (Richardson, TX: Society of Petroleum Engineers).
Greenlee, L. F., Lawler, D. F., Freeman, B. D., Marrot, B., and Moulin, P. (2009). Reverse osmosis desalination: water sources, technology, and today’s challenges. Water Res. 43, 2317–2348. doi: 10.1016/j.watres.2009.03.010
Halvorsen, R. (1977). Energy substitution in US manufacturing. Rev. Econ. Stat. 59, 381–388. doi: 10.2307/1928702
Hansen, B. R., and Davies, S. R. (1994). Review of potential technologies for the removal of dissolved components from produced water. Chem. Eng. Res. Des. 72, 176–188.
Hilal, N., Mohammad, A. W., Atkin, B., and Darwish, N. A. (2003). Using atomic force microscopy towards improvement in nanofiltration membranes properties for desalination pre-treatment: a review. Desalination 157, 137–144. doi: 10.1016/S0011-9164(03)00393-X
Hildenbrand, Z. L., Santos, I. C., Liden, T., Carlton, D. D.Jr., Varona-Torres, E., Martin, M. S., et al. (2018). Characterizing variable biogeochemical changes during the treatment of produced oilfield waste. Sci. Total Environ. 634, 1519–1529. doi: 10.1016/j.scitotenv.2018.03.388
Hosseini, A., Brown, J. E., Gwynn, J. P., and Dowdall, M. (2012). Review of research on impacts to biota of discharges of naturally occurring radionuclides in produced water to the marine environment. Sci. Total Environ. 438, 325–333. doi: 10.1016/j.scitotenv.2012.08.047
Huang, S., Pooi, C. K., Shi, X., Varjani, S., and Ng, H. Y. (2020). Performance and process simulation of membrane bioreactor (MBR) treating petrochemical wastewater. Sci. Total Environ. 747, 141311. doi: 10.1016/j.scitotenv.2020.141311
Igunnu, E. T., and Chen, G. Z. (2014). Produced water treatment technologies. Int. J. Low Carbon Technol. 9, 157–177. doi: 10.1093/ijlct/cts049
Ismail, A. A. O. O., Amouzandeh, G., and Grant, S. C. (2016). Structural connectivity within neural ganglia: a default small-world network. Neuroscience 337, 276–284. doi: 10.1016/j.neuroscience.2016.09.024
Judd, S., and Jefferson, B. (2003). Membranes for Industrial Wastewater Recovery and Re-use. Amsterdam: Elsevier.
Khatib, Z. (2007). “Produced water management in MENA region: is it a legacy or an opportunity for IOR/EOR field development,” in Proceedings of the International Petroleum Technology Conference, (Kuala Lumpur: International Petroleum Technology). doi: 10.2523/11624-MS
Kusworo, T. D., Aryanti, N., and Utomo, D. P. (2018). Oilfield produced water treatment to clean water using integrated activated carbon-bentonite adsorbent and double stages membrane process. Chem. Eng. J. 347, 462–471. doi: 10.1016/j.cej.2018.04.136
Lawson, K. W., and Lloyd, D. R. (1996). Membrane distillation. II. Direct contact MD. J. Memb. Sci. 120, 123–133. doi: 10.1016/0376-7388(96)00141-X
Lee, M., Wu, Z., and Li, K. (2015). “Advances in ceramic membranes for water treatment,” in Advances in Membrane Technologies for Water Treatment, eds A. Basile, A. Cassano, and N. Rastogi (Amsterdam: Elsevier), 43–82. doi: 10.1016/B978-1-78242-121-4.00002-2
Li, W., Qi, W., Chen, J., Zhou, W., Li, Y., Sun, Y., et al. (2020). Effective removal of fluorescent microparticles as Cryptosporidium parvum surrogates in drinking water treatment by metallic membrane. J. Memb. Sci. 594:117434. doi: 10.1016/j.memsci.2019.117434
Ma, J., Guo, X., Ying, Y., Liu, D., and Zhong, C. (2017). Composite ultrafiltration membrane tailored by MOF@ GO with highly improved water purification performance. Chem. Eng. J. 313, 890–898. doi: 10.1016/j.cej.2016.10.127
Macedonio, F., Ali, A., Poerio, T., El-Sayed, E., Drioli, E., and Abdel-Jawad, M. (2014). Direct contact membrane distillation for treatment of oilfield produced water. Sep. Purif. Technol. 126, 69–81. doi: 10.1016/j.seppur.2014.02.004
Maguire-Boyle, S. J., and Barron, A. R. (2014). Organic compounds in produced waters from shale gas wells. Environ. Sci. Process. Impacts 16, 2237–2248. doi: 10.1039/C4EM00376D
Martinetti, C. R., Childress, A. E., and Cath, T. Y. (2009). High recovery of concentrated RO brines using forward osmosis and membrane distillation. J. Memb. Sci. 331, 31–39. doi: 10.1016/j.memsci.2009.01.003
Mathieu, A., Hanlon, J., Myers, M., Melvin, W., French, B., DeBlois, E. M., et al. (2011). “Studies on fish health around the Terra Nova oil development site on the Grand Banks before and after discharge of produced water,” in Produced Water, eds K. Lee and J. Neff (New York, NY: Springer), 375–399. doi: 10.1007/978-1-4614-0046-2_20
McCabe, P. J. (2020). Oil and natural gas: global resources. Foss. Energy 1, 5–16. doi: 10.1007/978-1-4939-9763-3_71
Mcintosh, J. C., Hendry, M. J., Ballentine, C., Haszeldine, R. S., Mayer, B., Etiope, G., et al. (2018). A critical review of state-of-the-art and emerging approaches to identify fracking-derived gases and associated contaminants in aquifers. Environ. Sci. Technol. 53, 1063–1077. doi: 10.1021/acs.est.8b05807
McLaughlin, M. C., Blotevogel, J., Watson, R. A., Schell, B., Blewett, T. A., Folkerts, E. J., et al. (2020). Mutagenicity assessment downstream of oil and gas produced water discharges intended for agricultural beneficial reuse. Sci. Total Environ. 715:136944. doi: 10.1016/j.scitotenv.2020.136944
Meindersma, G. W., Guijt, C. M., and De Haan, A. B. (2006). Desalination and water recycling by air gap membrane distillation. Desalination 187, 291–301. doi: 10.1016/j.desal.2005.04.088
Mondal, S., and Wickramasinghe, S. R. (2008). Produced water treatment by nanofiltration and reverse osmosis membranes. J. Memb. Sci. 322, 162–170. doi: 10.1016/j.memsci.2008.05.039
Moon, J. D., Freeman, B. D., Hawker, C. J., and Segalman, R. A. (2020). Can self-assembly address the permeability/selectivity trade-offs in polymer membranes? Macromolecules 53, 5649–5654. doi: 10.1021/acs.macromol.0c01111
Mozafari, M., Seyedpour, S. F., Salestan, S. K., Rahimpour, A., Shamsabadi, A. A., Firouzjaei, M. D., et al. (2019). Facile Cu-BTC surface modification of thin chitosan film coated polyethersulfone membranes with improved antifouling properties for sustainable removal of manganese. J. Memb. Sci. 588:117200. doi: 10.1016/j.memsci.2019.117200
Mueller, J., Cen, Y., and Davis, R. H. (1997). Crossflow microfiltration of oily water. J. Memb. Sci. 129, 221–235. doi: 10.1016/S0376-7388(96)00344-4
Munirasu, S., Haija, M. A., and Banat, F. (2016). Use of membrane technology for oil field and refinery produced water treatment–A review. Process Saf. Environ. Prot. 100, 183–202. doi: 10.1016/j.psep.2016.01.010
Murray, K. E., and Holland, A. A. (2014). Subsurface fluid injection in oil and gas reservoirs and wastewater disposal zones of the midcontinent. AAPG Datapages 80377, 6–9.
Murray-Gulde, C., Heatley, J. E., Karanfil, T., Rodgers, J. H.Jr., and Myers, J. E. (2003). Performance of a hybrid reverse osmosis-constructed wetland treatment system for brackish oil field produced water. Water Res. 37, 705–713. doi: 10.1016/S0043-1354(02)00353-6
Nadella, M., Sharma, R., and Chellam, S. (2020). Fit-for-purpose treatment of produced water with iron and polymeric coagulant for reuse in hydraulic fracturing: temperature effects on aggregation and high-rate sedimentation. Water Res. 170:115330. doi: 10.1016/j.watres.2019.115330
Neff, J. M. (2002). Bioaccumulation in Marine Organisms: Effect of Contaminants from Oil Well Produced Water. Amsterdam: Elsevier. doi: 10.1016/B978-008043716-3/50002-6
Orem, W., Tatu, C., Varonka, M., Lerch, H., Bates, A., Engle, M., et al. (2014). Organic substances in produced and formation water from unconventional natural gas extraction in coal and shale. Int. J. Coal Geol. 126, 20–31. doi: 10.1016/j.coal.2014.01.003
Osborn, S. G., Vengosh, A., Warner, N. R., and Jackson, R. B. (2011). Methane contamination of drinking water accompanying gas-well drilling and hydraulic fracturing. Proc. Natl. Acad. Sci. U.S.A. 108, 8172–8176. doi: 10.1073/pnas.1100682108
Ozgun, H., Ersahin, M. E., Erdem, S., Atay, B., Kose, B., Kaya, R., et al. (2013). Effects of the pre−treatment alternatives on the treatment of oil−gas field produced water by nanofiltration and reverse osmosis membranes. J. Chem. Technol. Biotechnol. 88, 1576–1583. doi: 10.1002/jctb.4007
Pabby, A. K., Rizvi, S. S. H., and Requena, A. M. S. (2015). Handbook of Membrane Separations: Chemical, Pharmaceutical, Food, and Biotechnological Applications. Boca Raton, FL: CRC press. doi: 10.1201/b18319
Padaki, M., Murali, R. S., Abdullah, M. S., Misdan, N., Moslehyani, A., Kassim, M. A., et al. (2015). Membrane technology enhancement in oil–water separation. A review. Desalination 357, 197–207. doi: 10.1016/j.desal.2014.11.023
Park, H. B., Kamcev, J., Robeson, L. M., Elimelech, M., and Freeman, B. D. (2017). Maximizing the right stuff: The trade-off between membrane permeability and selectivity. Science 356:eaab0530. doi: 10.1126/science.aab0530
Pejman, M., Dadashi Firouzjaei, M., Aghapour Aktij, S., Das, P., Zolghadr, E., Jafarian, H., et al. (2020a). In situ Ag-MOF growth on pre-grafted zwitterions imparts outstanding antifouling properties to forward osmosis membranes. ACS Appl. Mater. Interfaces 12, 36287–36300. doi: 10.1021/acsami.0c12141
Pejman, M., Firouzjaei, M. D., Aktij, S. A., Das, P., Zolghadr, E., Jafarian, H., et al. (2020b). Improved antifouling and antibacterial properties of forward osmosis membranes through surface modification with zwitterions and silver-based metal organic frameworks. J. Memb. Sci. 611:118352. doi: 10.1016/j.memsci.2020.118352
Peng, H., and Guo, J. (2020). Removal of chromium from wastewater by membrane filtration, chemical precipitation, ion exchange, adsorption electrocoagulation, electrochemical reduction, electrodialysis, electrodeionization, photocatalysis and nanotechnology: a review. Environ. Chem. Lett. 18, 2055–2068. doi: 10.1007/s10311-020-01058-x
Pica, N. E., Carlson, K., Steiner, J. J., and Waskom, R. (2017). Produced water reuse for irrigation of non-food biofuel crops: Effects on switchgrass and rapeseed germination, physiology and biomass yield. Ind. Crops Prod. 100, 65–76. doi: 10.1016/j.indcrop.2017.02.011
Puntervold, T., and Austad, T. (2008). Injection of seawater and mixtures with produced water into North Sea chalk formation: Impact of fluid–rock interactions on wettability and scale formation. J. Pet. Sci. Eng. 63, 23–33. doi: 10.1016/j.petrol.2008.07.010
Qdais, H. A., and Moussa, H. (2004). Removal of heavy metals from wastewater by membrane processes: a comparative study. Desalination 164, 105–110. doi: 10.1016/S0011-9164(04)00169-9
Rahimpour, A., Seyedpour, S. F., Aghapour Aktij, S., Dadashi Firouzjaei, M., Zirehpour, A., Arabi Shamsabadi, A., et al. (2018). Simultaneous improvement of antimicrobial, antifouling, and transport properties of forward osmosis membranes with immobilized highly-compatible polyrhodanine nanoparticles. Environ. Sci. Technol. 52, 5246–5258. doi: 10.1021/acs.est.8b00804
Rajesh, R., and Gupta, H. K. (2021). Characterization of injection-induced seismicity at north central Oklahoma, USA. J. Seismol. doi: 10.1007/s10950-020-09978-5
Ramirez, P.Jr. (2002). Oil Field Produced Water Discharges Into Wetlands in Wyoming. Washington, DC: U.S. Fish and Wildlife Service.
Ravanchi, M. T., Kaghazchi, T., and Kargari, A. (2009). Application of membrane separation processes in petrochemical industry: a review. Desalination 235, 199–244. doi: 10.1016/j.desal.2007.10.042
Rich, A. L., and Crosby, E. C. (2013). Analysis of reserve pit sludge from unconventional natural gas hydraulic fracturing and drilling operations for the presence of technologically enhanced naturally occurring radioactive material (Tenorm). New Solut. 23, 117–135. doi: 10.2190/NS.23.1.h
Roach, T. (2018). Oklahoma earthquakes and the price of oil. Energy Policy 121, 365–373. doi: 10.1016/j.enpol.2018.05.040
Rubinstein, J. L., and Mahani, A. B. (2015). Myths and facts on wastewater injection, hydraulic fracturing, enhanced oil recovery, and induced seismicity. Seismol. Res. Lett. 86, 1060–1067. doi: 10.1785/0220150067
Scanlon, B. R., Reedy, R. C., Xu, P., Engle, M., Nicot, J. P., Yoxtheimer, D., et al. (2020). Can we beneficially reuse produced water from oil and gas extraction in the US? Sci. Total Environ. 717:137085. doi: 10.1016/j.scitotenv.2020.137085
Scanlon, B. R., Weingarten, M. B., Murray, K. E., and Reedy, R. C. (2019). Managing basin−scale fluid budgets to reduce injection−induced seismicity from the recent US shale oil revolution. Seismol. Res. Lett. 90, 171–182. doi: 10.1785/0220180223
Seyedpour, S. F., Arabi Shamsabadi, A., Khoshhal Salestan, S., Dadashi Firouzjaei, M., Sharifian Gh, M., Rahimpour, A., et al. (2020a). Tailoring the biocidal activity of novel silver-based metal azolate frameworks. ACS Sustain. Chem. Eng. 8, 7588–7599. doi: 10.1021/acssuschemeng.0c00201
Seyedpour, S. F., Dadashi Firouzjaei, M., Rahimpour, A., Zolghadr, E., Arabi Shamsabadi, A., Das, P., et al. (2020b). Toward sustainable tackling of biofouling implications and improved performance of TFC FO membranes modified by Ag-MOFs nanorods. ACS Appl. Mater. Interfaces 12, 38285–38298. doi: 10.1021/acsami.0c13029
Shaffer, D. L., Arias Chavez, L. H., Ben-Sasson, M., Romero-Vargas Castrilloìn, S., Yip, N. Y., and Elimelech, M. (2013). Desalination and reuse of high-salinity shale gas produced water: drivers, technologies, and future directions. Environ. Sci. Technol. 47, 9569–9583. doi: 10.1021/es401966e
Shakhawat, C., Tahir, H., and Neil, B. (2006). Fuzzy rule-based modelling for human health risk from naturally occurring radioactive materials in produced water. J. Environ. Radioact. 89, 1–17. doi: 10.1016/j.jenvrad.2006.03.002
Shams Ashaghi, K., Ebrahimi, M., and Czermak, P. (2007). Ceramic ultra-and nanofiltration membranes for oilfield produced water treatment: a mini review. Open Environ. Sci. 1, 1–8. doi: 10.2174/1874233500701010001
Shirazi, M. M. A., Kargari, A., Bastani, D., and Fatehi, L. (2014). Production of drinking water from seawater using membrane distillation (MD) alternative: direct contact MD and sweeping gas MD approaches. Desalin. Water Treat. 52, 2372–2381. doi: 10.1080/19443994.2013.797367
Sirivedhin, T., McCue, J., and Dallbauman, L. (2004). Reclaiming produced water for beneficial use: salt removal by electrodialysis. J. Memb. Sci. 243, 335–343. doi: 10.1016/j.memsci.2004.06.038
Strong, J. D., Cunningham, J., and Dunkel, M. (2017). Oklahoma Water for 2060: Produced Water Reuse and Recycling. Oklahoma City: Oklahoma Water Resources Board.
Tchounwou, P. B., Yedjou, C. G., Patlolla, A. K., and Sutton, D. J. (2012). “Heavy metal toxicity and the environment,” in Molecular, Clinical and Environmental Toxicology, ed. A. Luch (Basel: Springer), 133–164. doi: 10.1007/978-3-7643-8340-4_6
Torres, L., Yadav, O. P., and Khan, E. (2016). A review on risk assessment techniques for hydraulic fracturing water and produced water management implemented in onshore unconventional oil and gas production. Sci. Total Environ. 539, 478–493. doi: 10.1016/j.scitotenv.2015.09.030
US Energy Information Administration (2016). Trends in US Oil and Natural Gas Upstream Costs. Washington, DC: US Energy Information Administration.
Uyttendaele, M., Jaykus, L., Amoah, P., Chiodini, A., Cunliffe, D., Jacxsens, L., et al. (2015). Microbial hazards in irrigation water: standards, norms, and testing to manage use of water in fresh produce primary production. Compr. Rev. Food Sci. Food Saf. 14, 336–356. doi: 10.1111/1541-4337.12133
Vajner, C., Yan, H., Guo, L., Mathews, M., Kuhlman, M., Benefield, S., et al. (2016). Thickness identification of epitaxial Bi2Te3 via optical contrast. 2D Mater. 3:021010. doi: 10.1088/2053-1583/3/2/021010
Vale, J. A., and Meredith, T. J. (1981). “Poisoning due to aliphatic, aromatic and chlorinated hydrocarbons,” in Poisoning Diagnosis and Treatment, eds J. A. Vale and T. J. Meredith (Dordrecht: Springer), 153–159. doi: 10.1007/978-94-011-6763-5_23
Van der Baan, M., and Calixto, F. J. (2017). Human−induced seismicity and large−scale hydrocarbon production in the USA and C anada. Geochem. Geophys. Geosystems 18, 2467–2485. doi: 10.1002/2017GC006915
Van der Bruggen, B., Vandecasteele, C., Van Gestel, T., Doyen, W., and Leysen, R. (2003). A review of pressure−driven membrane processes in wastewater treatment and drinking water production. Environ. Prog. 22, 46–56. doi: 10.1002/ep.670220116
Veil, J. (2015). US Produced Water Volumes and Management Practices in 2012. Oklahoma City: Ground Water Protection Council, Inc.
Veil, J., and Clark, C. (2011). Produced water volume estimates and management practices. SPE Prod. Oper. 26, 234–239. doi: 10.2118/125999-PA
Veil, J. A. (2011). “Produced water management options and technologies,” in Produced Water, eds K. Lee and J. Neff (New York, NY: Springer), 537–571. doi: 10.1007/978-1-4614-0046-2_29
Veil, J. A. (2020). Produced Water Volumes and Management Practices For 2017. Annapolis, MD: Veil Environmental, LLC.
Veil, J. A., Puder, M. G., Elcock, D., and Redweik, R. J.Jr. (2004). A White Paper Describing Produced Water from Production of Crude Oil, Natural Gas, and Coal Bed Methane. Lemont, IL: Argonne National Laboratory. doi: 10.2172/821666
Vengosh, A., Jackson, R. B., Warner, N., Darrah, T. H., and Kondash, A. (2014). A critical review of the risks to water resources from unconventional shale gas development and hydraulic fracturing in the United States. Environ. Sci. Technol. 48, 8334–8348. doi: 10.1021/es405118y
Venkatesan, A., and Wankat, P. C. (2017). Produced water desalination: an exploratory study. Desalination 404, 328–340. doi: 10.1016/j.desal.2016.11.013
Wang, P., and Chung, T.-S. (2015). Recent advances in membrane distillation processes: membrane development, configuration design and application exploring. J. Memb. Sci. 474, 39–56. doi: 10.1016/j.memsci.2014.09.016
Warner, N. R., Christie, C. A., Jackson, R. B., and Vengosh, A. (2013). Impacts of shale gas wastewater disposal on water quality in Western Pennsylvania. Environ. Sci. Technol. 47, 11849–11857. doi: 10.1021/es402165b
Webb, R., and Zodrow, K. R. (2020). “Disposal of water for hydraulic fracturing: case study on the US,” in Regulating Water Security in Unconventional Oil and Gas, eds R. M. Buono, E. López-Gunn, J. McKay, and C. Staddon (Berlin: Springer), 221–241. doi: 10.1007/978-3-030-18342-4_11
Wilson, J. M., and VanBriesen, J. M. (2012). Research article: oil and gas produced water management and surface drinking water sources in Pennsylvania. Environ. Pract. 14, 288–300. doi: 10.1017/S1466046612000427
Xu, P., Drewes, J. E., and Heil, D. (2008). Beneficial use of co-produced water through membrane treatment: technical-economic assessment. Desalination 225, 139–155. doi: 10.1016/j.desal.2007.04.093
Yang, C., Zhang, G., Xu, N., and Shi, J. (1998). Preparation and application in oil–water separation of ZrO2/α-Al2O3 MF membrane. J. Memb. Sci. 142, 235–243. doi: 10.1016/S0376-7388(97)00336-0
Yang, Z., Zhou, Y., Feng, Z., Rui, X., Zhang, T., and Zhang, Z. (2019). A review on reverse osmosis and nanofiltration membranes for water purification. Polymers (Basel). 11:1252. doi: 10.3390/polym11081252
Zhang, H., Zhou, X., Wang, L., Wang, W., and Xu, J. (2018). Concentrations and potential health risks of strontium in drinking water from Xi’an, Northwest China. Ecotoxicol. Environ. Saf. 164, 181–188. doi: 10.1016/j.ecoenv.2018.08.017
Zhao, D. L., Japip, S., Zhang, Y., Weber, M., Maletzko, C., and Chung, T.-S. (2020). Emerging thin-film nanocomposite (TFN) membranes for reverse osmosis: a review. Water Res. 173, 115557. doi: 10.1016/j.watres.2020.115557
Zirehpour, A., Rahimpour, A., Khoshhal, S., Firouzjaei, M. D., and Ghoreyshi, A. A. (2016). The impact of MOF feasibility to improve the desalination performance and antifouling properties of FO membranes. RSC Adv. 6, 70174–70185. doi: 10.1039/C6RA14591D
Zolghadr, E. (2016). Krapivin VF, Varotsos CA, Soldatov VY| New ecoinformatics tools in environmental science. Environ. Earth Sci. 75, 450. doi: 10.1007/s12665-016-5411-1
Zou, L., Gusnawan, P., Zhang, G., and Yu, J. (2020). Novel Janus composite hollow fiber membrane-based direct contact membrane distillation (DCMD) process for produced water desalination. J. Memb. Sci. 597:117756. doi: 10.1016/j.memsci.2019.117756
Keywords: produced water, water management, wastewater reuse, membrane, review article, water pollution
Citation: Zolghadr E, Firouzjaei MD, Amouzandeh G, LeClair P and Elliott M (2021) The Role of Membrane-Based Technologies in Environmental Treatment and Reuse of Produced Water. Front. Environ. Sci. 9:629767. doi: 10.3389/fenvs.2021.629767
Received: 15 November 2020; Accepted: 24 February 2021;
Published: 16 March 2021.
Edited by:
Alberto Tiraferri, Politecnico di Torino, ItalyReviewed by:
Devin L. Shaffer, University of Houston, United StatesCopyright © 2021 Zolghadr, Firouzjaei, Amouzandeh, LeClair and Elliott. This is an open-access article distributed under the terms of the Creative Commons Attribution License (CC BY). The use, distribution or reproduction in other forums is permitted, provided the original author(s) and the copyright owner(s) are credited and that the original publication in this journal is cited, in accordance with accepted academic practice. No use, distribution or reproduction is permitted which does not comply with these terms.
*Correspondence: Mostafa Dadashi Firouzjaei, bWRmaXJvdXpqYWVpQGNyaW1zb24udWEuZWR1; Mark Elliott, bWVsbGlvdHRAZW5nLnVhLmVkdQ==
Disclaimer: All claims expressed in this article are solely those of the authors and do not necessarily represent those of their affiliated organizations, or those of the publisher, the editors and the reviewers. Any product that may be evaluated in this article or claim that may be made by its manufacturer is not guaranteed or endorsed by the publisher.
Research integrity at Frontiers
Learn more about the work of our research integrity team to safeguard the quality of each article we publish.