- 1Department of Materials Science and Engineering, University of North Texas, Denton, TX, United States
- 2Department of Mechanical Engineering, University of North Texas, Denton, TX, United States
Li-CO2 batteries with a theoretical energy density of 1,876 Wh kg−1 are attractive as a promising energy storage strategy and as an effective way to reduce greenhouse gas emissions by CO2 reduction and the formation of discharge product Li2CO3 and carbon. This article provides critical perspectives on the development of Li-CO2 batteries as well as a description of current issues and challenges associated with cathode catalysts, electrolyte, and anode for Li-CO2 batteries. Furthermore, the development and deployment of materials to overcome these challenges of Li-CO2 batteries are discussed briefly. Finally, a systematic analysis of beyond Li-CO2 batteries (other Metal-CO2 batteries) as a potential research direction in the development of energy storage and CO2 fixation and utilization in practical applications is provided.
1 Introduction
Climate change is a global phenomenon that must be addressed to avoid major environmental consequences due to the non-renewable property of fossil fuels and excessive CO2 emissions in the near future (Krane, 2017; Abbass et al., 2022). Even though significant progress has been made toward clean energy initiatives to mitigate the major cause of climate change, excessive greenhouse gas emission and usage of non-renewable fossil fuel still remain the dominant sources of climate crisis (Lelieveld et al., 2019). Among others, carbon dioxide (CO2), one of the leading greenhouse gases that arises from the use of fossil fuel, is accelerating climate change and likely will lead to global warming and endanger the sustainability of the planet Earth and its habitants. One way of preventing the emissions of CO2 into the atmosphere is its capture, fix, storage and transportation in its liquid form, which requires further compression or liquefaction procedures. Such an approach could be highly costly, and the processes add higher energy consumption and hence unwanted additional greenhouse gas emission. To slow down the emission, to some extent, CO2 could be used effectively in other energy forms by using metal-CO2 batteries such as Li-CO2 (Sun X. et al., 2021) and Zn-CO2 (Xie and Wang, 2019; Wang et al., 2020; Hao et al., 2021; Yang et al., 2022a; Liu S. et al., 2022; Liu W. et al., 2022). In parallel, ultra-high energy density (11,680 Wh kg−1) metal-air batteries are also considered promising battery technology which is environmentally friendly (Cao et al., 2021) and utilized unlimited source of oxygen present in atmosphere. Metal-air batteries include primarily oxygen as air, and have received tremendous research interest in recent years (Li and Lu, 2017; Yang et al., 2022b; Zhang et al., 2014). A typical metal-air battery consists of an external cathode of oxygen (O2) present in the ambient air, aqueous or aprotic electrolyte, and metal anode.
Several Li-air batteries have been evolved over the years, employing lithium as an anode and O2 or other gases as cathode including CO2 (Li and Lu, 2017; Tang et al., 2022; Zhao et al., 2021; Zhang et al., 2021a) Among these batteries, Li-CO2 batteries are of particular interest (Figure 1A) due to their high discharging voltage (∼2.8 V) and very high theoretical specific energy density (1,876 Wh kg−1). Also, Li-CO2 electrochemistry can be a good strategy for CO2 fixation by (Qiao et al., 2017) reduction of CO2 and formation of Li2CO3 and carbon via single step reaction. Thus, the Li-CO2 batteries not only contribute to next-generation initiatives toward clean and sustainable energy but also relieve the detrimental impact of CO2 on the environment. This mini review provides the state-of-the-art Li-CO2 battery technology in comparing Li-O2 batteries with their challenges and potential strategies to overcome the challenges. Furthermore, particular attention is devoted to the perspective and feasibility of the Li-CO2 battery system in space applications such as Mars mission.
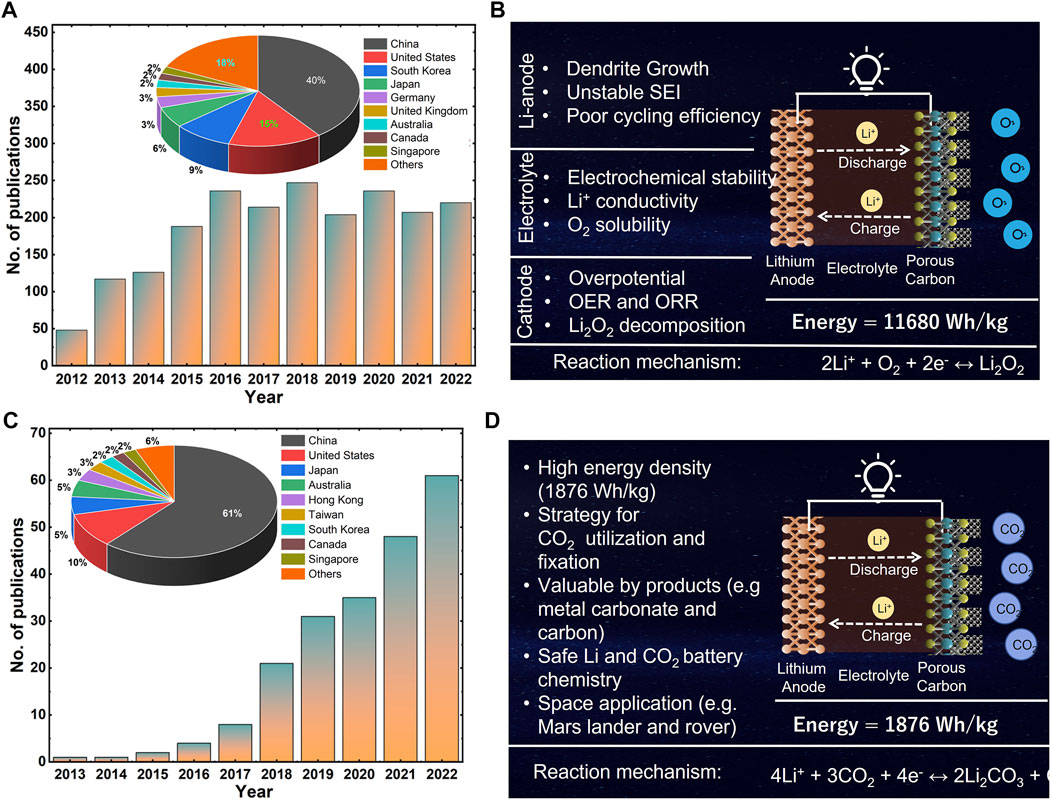
FIGURE 1. (A). Year-wise publication of Li-O2 battery research (data were collected from web Scopus search), (B). Challenges and schematic of Li-O2 battery, (C). Year-wise publication of Li-CO2 battery research (data were collected from web Scopus search), (D). Advantage and schematic of Li-CO2 battery, reported in the literature.
2 Li-CO2 battery as a potential energy storage system
Li-ion batteries have dominated the portable electronics and electric vehicle market ever since their commercialization in 1991 (Ji and Nazar, 2010; Li et al., 2009; El Kharbachi et al., 2020; Mahmud et al., 2022). With the realization that Li-ion batteries have reached their practical limits (energy densities of 240–250 Wh kg−1 and 550–600 Wh l-1 (Cao et al., 2020), alternative battery systems with much higher energy storage capabilities need to be sought. Li-air batteries, the promising candidates for beyond Li-ion chemistry with high energy density have attracted much attention in recent years (Wang L. et al., 2018; Xiao et al., 2010; Huang et al., 2022; Li W. et al., 2021). Li-air batteries can deliver an ultra-high energy density of 11,680 Wh kg−1, which puts the Li-air battery on higher ground as compared to other battery systems (Imanishi and Yamamoto, 2014). The electrochemical reaction involved in Li-O2 battery is 2Li + O2 ↔ Li2O2, with the forward direction as discharge (formation of Li2O2), and reverse direction as a charge with decomposition of Li2O2 (Chang et al., 2017). Mostly, Li-air batteries that have been tested are operated under pure oxygen, which can be costly considering the O2 purification process and the associated costs. In the ambient air, other gases such as nitrogen (N2), argon (Ar), carbon dioxide (CO2) and water moisture are present. The influence of these gases or air on the reaction mechanism and electrochemical performance of Li-air batteries remains questionable (Cai et al., 2018). The inert nature of Ar and N2 gases and the relatively high cathode voltage of 3.0 V are not able to activate the electrochemical reactions of lithium with Ar and N2 (Nam et al., 2001). Water moisture present in the air (H2O) and CO2 are active gases, and are theoretically expected to be involved in electrochemical reactions in Li-air cells. Water moisture in the ambient air potentially deteriorates cell performance due to the corrosion of the Li metal anode (Shui et al., 2013). Therefore, because of such issues with various Li-air batteries (also described in (Figure 1B)), overall, the development of Li-air batteries is hindered, mainly by selective filtration of O2 from the air and unwanted side reactions with other elements in the air, such as water vapor, and carbon dioxide, which leads to the large cell overpotential, limited reversibility, and poor cyclability (Liu T. et al., 2020).
On the other hand, Li-CO2 batteries, a type of metal-CO2 batteries, are attractive (Figure 1C) due to their dual functionality of utilizing CO2 gas and electrical energy storage, while demonstrating a high theoretical energy density (1,876 Wh kg−1; Cai et al., 2018). However, the Li-CO2 battery is an open cell configuration (Figure 1D), with Li metal anode, organic electrolyte and CO2 porous-carbon cathode. In Li-CO2 battery chemistry, during discharge, Li undergoes a redox reaction and react with CO2 with the formation of Li2CO3 and solid carbon at the cathode structure (4Li+3CO2↔2Li2CO3+C); whereas during charge, reverse reaction proceeded with decomposition of Li2CO3. Here, Li-CO2 could be an alternative for using the CO2 present in the air (or use of industrial CO2 emission) while simultaneously contributing to reduced greenhouse emissions (Figure 1D). The following sections in this work summarize the critical understanding of issues involving the cathode-catalyst, electrolyte, and anode and their strategies, all of which can help to accelerate the practical development of Li-CO2 batteries.
3 Challenges and development of cathode for Li-CO2 batteries
In general, Li-CO2 battery cathodes should be porous, catalytically active and conducting in nature with wide electrochemical stability across the operating potential during charge and discharge. In most studies, porous carbon generally acts as the cathode material supporting the catalyst layer (e.g., Ru (Zhang P.-F. et al., 2020), Pt (Zhang et al., 2015), Au (Qiao et al., 2017), metal oxide (Liu L. et al., 2020), MoS2 (Pipes et al., 2019), Mo2C (Yang et al., 2020)). However, the following critical challenges associated with the cathode-catalyst in the Li-CO2 battery must be addressed; namely, i) sluggish CO2 reduction reaction (CRR) and the CO2 evolution reaction (CER), ii) complex three-phase (CO2 gas-liquid electrolyte-solid cathode catalyst) interaction at electrode/electrolyte interface, iii) poor catalytic activity at electrode/electrolyte interface, iv) Li2CO3 discharge product being insoluble in the organic electrolyte, electronically insulating in nature and thermodynamically more stable, v) Large overpotential during discharge and charge, vi) in sufficient ionic and electronic conductivity of cathode/catalyst structure, and vii) poor reversibility and low energy efficiency of catalytic-cathode structure.
Various electrocatalysts that have been explored to enhance the reaction kinetics and reversibility of the Li-CO2 battery include carbon materials, transition metal, noble/precious metals, and organic frameworks and their compounds. The initial development of a Li-CO2 battery used mainly commercial carbon-based material [Ketjen Black (KB), Super P] as cathode as well as catalyst (without adding additional catalyst elements). For example, Liu et al. (Liu et al., 2014) developed a KB cathode for rechargeable Li/CO2-O2 and Li/CO2 batteries having discharge specific capacities of 1,808 mA h g−1 and 1,032 mA h g−1, respectively. However, the use of carbon nanomaterials [CNTs, Graphene, graphene oxide (r-GO)] is the good choice as a cathode structure due to its tunable morphology/porosity, electrical conductivity, high surface area and enhanced catalytic activity compared with conventional carbon materials. For instance, Zhang et al. (Zhang et al., 2015) first reported carbon nanotubes (CNTs) air cathodes with high electrical conductivity and porous three-dimensional networks for rechargeable Li-CO2 batteries with an initial discharge capacity of 8,379 mAh g−1. Further, catalytic activity and electrochemical stability of carbon material can be improved by adding defects in carbon structure such as voids, edges and doping heteroatoms. It has been observed that regulating the electronic structure and catalytic activity of pristine carbon material by heteroatoms doping is an effective strategy to improve the performance of Li-CO2 batteries. Single-atom doping involves B, N, O, S, and F, (Zhou et al., 2022); however, N-doped carbon material has been used as an effective strategy in Li-CO2 batteries (Jiao et al., 2021). In N-doping, electronegative N atoms placed near to a C atom alter the spin/charge density across the sp2 carbon structure and thus improved adsorption energy (Zhang Z. et al., 2020) and enhanced electronic conductivity of the overall carbon structure (Yang et al., 2018). For example, N-doped carbon nanotubes as binder-free cathodes were utilized for high performance flexible quasi-solid-state Li-CO2 batteries (Li X. et al., 2021). Here, N doping includes pyridinic, pyrrolic, quaternary nitrogen and pyridine-N-oxide structure, which provides low overpotential, good rate capability and high specific full discharge capacity, and long cycle life (1203 cycles). Furthermore, multiple atoms doping also enhances the overall catalytic, electronic conductivity and adsorption energy of cathode structure. N, S-doped CNTs cathode catalyst can deliver a high discharge capacity of 23,560 mAh g-1 for a quasi-solidus flexible Li-CO2 battery (Song et al., 2020). Here, multi atom (e.g., N, S, Co, Ir) doping increases the defects to enhance the kinetics of the CO2 fixing reaction with high adsorption of Li-ions and CO2 gas molecules (Song et al., 2019; Rho et al., 2022).
Noble/precious metals are also a good choice as an effective electro-catalyst for Li-CO2 battery applications due to their enhanced electrolytic properties, such as low over-potential, electrochemical stability against electrolyte, efficient CO2 capture, and long-term durability over the operation. To the best of the author’s knowledge, only three precious metals (Au, Ru, and Ir) have been explored as an electrocatalyst in the development of Li-CO2 batteries. Ru metal has been observed to be an efficient electro-catalyst to improve the capacity and cycling stability by providing lower charging potential (3.6 V) compared to Au-based (>4.0 V) and Ir based (4.14 V) cathode (Qiao et al., 2017; Wang C. et al., 2018). The development of noble metal oxide (e.g., RuO), phosphides (e.g., RuP2) and their composite (IrO2/MnO2) is also an effective strategy to overcome electrocatalytic potential and improve the CRR and CER of Li-CO2 batteries. However, availability of the precious metal’s precursors and their high processing cost can limit the usage of noble metals in large scale and commercial production of Li-CO2 batteries.
Transition metals and their compounds are effective and alternative electro-catalysts due to their multivalent nature of transition metal, tunable property (morphology, shape, pore size) and availability of precursor and cost effectiveness. Transitional metal-based materials such as oxide, carbides, nitrides and sulfides have also been explored in Li-CO2 battery systems, and have improved reversibility of CRR and CER.
For example, Ge et al. (Ge et al., 2019) developed a Co-doped MnO2 catalyst for high-performance Li-CO2 batteries with a high initial capacity of 8,160 mA h g−1 (at 100 mA g−1). The Co0.2Mn0.8O2/carbon cathode delivered an ultralong cycle life of 500 cycles with low overpotential (∼0.73 V). Yuyang Hou et al. (Hou et al., 2017) show the type of catalyst used for Li-CO2 batteries can alter their discharge product. For example, they have used a Mo2C/carbon nanotube composite cathode which can stabilize Li2C2O4 as a final discharge product over insulating Li2CO3. The Mo2C/carbon nanotube composite cathode catalyst can be effective at lowering the decomposition of the discharge product below 3.5 V on charge with high energy efficiency (77%). Transition Metal Dichalcogenides (TMDs) can also be crucial catalysts for Li-CO2 batteries due to their mixed 1T (metallic) + 2H (semiconducting) phase (Wonbong et al., 2017 ; Bhoyate et al., 2021), which can provide a good catalytic effect with electronic conductivity to the structure. Further, NiO, (Zhang et al., 2018), Mn2O3 (Ma et al., 2018b), TiO2 (Pipes et al., 2018), MoS2 (Ahmadiparidari et al., 2019), and ZnS (H. Wang et al., 2019) have been explored as cathode catalyst for Li-CO2 batteries. However, there are other materials that can be explored for efficient catalyst including single metal-based compounds (Fe, Co, Ni, Ti, Zn and Cu), and mixed metal compounds (FeCoW, NiCoOx, CoFeOx, LaMnO3; C. Liu et al., 2012; Sun Y. et al., 2021). Furthermore, potential transition metal based catalysts can be reviewed in literature (Franco et al., 2020; Azaiza-Dabbah et al., 2022; Lv et al., 2022).
Unique properties such as ultrahigh porosity, versatile functionalities, long-range order, tunable pore sizes, synthetic versatility, CO2 capture, and controllable structures (Zhao et al., 2018; Baumann et al., 2019; Zhu et al., 2019) of covalent organic frameworks (COFs) and metal-organic frameworks (MOFs) certify them as being potential catalyst for Li-CO2 batteries. Wang and co-authors (Li et al., 2018) have explored for the first time eight porous MOFs (Mn2 (dobdc), Co2(dobdc), Ni2(dobdc), Mn (bdc), Fe (bdc), Cu(bdc), Mn(C2H2N3)2, and Mn(HCOO)2) and two non-porous materials (MnCO3 and MnO) for Li-CO2 batteries. However, Mn2 (dobdc) porous MOF delivered excellent discharge capacity of 18,022 mA h g−1 at 50 mA g−1 with a low charge potential of 3.96 V; whereas Meng et al. (Huang et al., 2019) for the first time reported the case of COFs as a catalyst for Li-CO2 batteries. They developed Graphene@COF as a catalyst, which delivered a high discharge capacity of 27,833 mAh g−1 at 75 mA g−1, with a low charge potential of 3.5 V. Xing Li et al. (Li et al., 2019) introduced a hydrazone/hydrazide-containing COF-Ru@CNT as a cathode catalyst which shows a high capacity of 27,348 mAh g−1 at 200 mA g−1 with an accelerated decomposition of discharge product (low overpotential of 1.24 V).
4 Challenges and development of electrolyte system for Li-CO2 batteries
The efficient electrochemical performance of Li-CO2 batteries requires appropriate electrolyte chemistries that should be compatible with other integral parts of the battery, particularly with the cathode-catalyst. Some challenges associated with the electrolyte system of Li-CO2 batteries that need to be addressed include, i) interface stability with cathode catalyst during the cycle, ii) limited CO2 diffusion and solubility, iii) self-decomposition under high voltage during charging, flammability, leakage, and evaporation of liquid electrolyte, iv) Li-ion conductivity and diffusivity (particularly for quasi and all solid-state electrolyte), and v) electrochemical stability against intermediates (particularly C and O based radicals) that form during reaction.
The majority of reports on Li-CO2 batteries have relied on very limited electrolytes, either sulfones-based dimethyl sulfoxide (DMSO) or ethers based tetraethylene glycol dimethyl ether (TEGDME). DMSO solvent is promising because of its high CO2 solubility, dielectric-strength, high conductivity, and low viscosity (Zhang et al., 2022; Zhao et al., 2019). However, DMSO solvent caused failure of the Li-CO2 battery with lasting depletion via evaporation and side reactions (Lu et al., 2022); whereas TEGDME electrolyte was used in Li-CO2 batteries due to its low evaporation loss, high oxidation potentials (>4.5 V vs. Li/Li+), high boiling point and stability against intermediates (Xu, 2004; Zhang et al., 2022). However, lithium trifluoromethanesulfonate (1 M LiCF3SO3) and lithium bis(trifluoromethanesulfonyl)imide (1 M LiTFSI) have been explored as promising salts for Li-CO2 battery development. Quasi-solid electrolytes (gel polymer electrolyte (Li et al., 2017), composite polymer electrolyte (Hu et al., 2017a) and all solid state electrolytes (e.g., Li1.5Al0.5Ge1.5(PO4)3 (LAGP); Guan et al., 2022), lithium aluminum titanium phosphate (LATP; Na et al., 2022) have also been explored for the development of Li-CO2 batteries, and provide electrolyte stability, safety and a way forward to achieve high energy density.
5 Challenges and progress of lithium anode for Li-CO2 batteries
Lithium metal anode is an important component to improve the performance of Li-CO2 batteries.
Critical challenges that need to be considered for safe and high energy density Li-CO2 batteries include, particularly, i) corrosion of Li-anode with CO2 and formation insulating Li2CO3 on surface, ii) stability of Li anode with organic electrolyte, iv) unstable solid electrolyte interface (SEI) on Li-anode and, v) unwanted and unsafe growth of Li dendrites.
Very limited work has been explored for the development of Li anode in Li-CO2 battery application. However, Li corrosion due to reaction between diffused CO2 gas (passed through electrolyte) and Li surface which forms Li2CO3 as a corrosion product considered as critical issues of Li-CO2 battery. Zhang et al. (Zhang et al., 2021b) constructed Li-ethylenediamine (LE) layer on a Li anode to overcome Li anode issues in a Li-CO2 battery such as dendrite, side reaction, passivation/pulverization, and interfacial impedance. However, other approaches that can be adopted to overcome Li-anode issues such deploying artificial SEI (Yu et al., 2020), developing electrolyte additive (Li L. et al., 2021), and coating of protection layer (Cha et al., 2018).
6 Li-CO2 battery application on Earth and Mars atmosphere
To consider the practical operation of Li-CO2 batteries on Earth atmosphere, the source of pure CO2 needs to be onboard/supplemented, particularly for electric vehicles (EVs) and grid applications. However, it is all about the economics and technical feasibility of extracting CO2 from the Earth atmosphere to operate the Li-CO2 battery in “CO2 breathing mode”. D. W. Keith et al. (Keith et al., 2018) provided a systematic cost analysis for capturing CO2 (1 ton/year) from the atmosphere. Here, atmospheric air is directed through towers that contain potassium hydroxide (KOH) solution. As CO2 contains air in contact with KOH, it forms potassium carbonate (K2CO3). Further, K2CO3 is heated with calcium carbonate (CaCO3), which releases CO2 and is pressurized in a pipeline for underground storage. Capturing a ton of CO2 has been calculated and analyzed potentially to cost about $232, and can drop to below $100 per ton. Here, instead of feeding the CO2 collected gas and transporting it to ground, it is wise to use the strategy of metal-CO2 battery for better utilization and provide alternative energy resource.
Beyond planet Earth, recently, with the accelerated Mars exploration from different countries, the possibility of a Li-CO2 battery in the Mars mission has been discussed heavily. According to National Aeronautics and Space Administration (NASA), Mars Perseverance carried Li-ion batteries that weighed 26.5 kg to power herself, which is a significant weight coming only from the battery. The batteries in Mars landers are used during descent, landing operations, and support during the initial deployments until the solar panels are in full operation. To make these missions more efficient and economically feasible, the vehicles should have batteries with higher specific energy density and lightweight, so as to help reduce overall mission cost and significantly increase payload. Some feasibility studies have been conducted at the research level on whether the Li-CO2 battery system is suitable for Mars missions. Sharma et al. were the first to develop a working prototype of a Li-CO2 battery operated at simulated Martian gases atmosphere (Pathak and Sharma, 2021), and confirmed the feasibility of Li-CO2 battery operated in simulated Martian atmosphere consisting of 95% CO2 gas. Further, Jiaxin Li et al. (Li et al., 2020) developed 1,3-dioxolane-based electrolytes and iridium-based cathodes for ultra-low-temperature Li-CO2 batteries which were operated at realistic temperature fluctuations of Mars atmosphere. Knowing the feasibility of Li-CO2 battery in saturated air (100% humidity) and low-pressure (∼6 mbar) conditions of Mars has been interesting. However, current efforts to the development of Li-CO2 battery design for the Mars mission have the potential of reducing significant payload and cost of the overall mission spacecraft.
7 Beyond Li-CO2 batteries
The theoretical energy density of metal-CO2 batteries is calculated based on thermodynamic reaction as described in Figure 2A. Theoretical energy density and average discharge potential of metal-CO2 systems (Figures 2B,C) provide a comparison of these parameters. Li-CO2 batteries have great potential in terms of high specific energy density (1,876 Wh kg−1) with high discharge potential (−2.8 V) compared to other metal-CO2 batteries such as Na-CO2, K-CO2, Al-CO2, Mg-CO2, and Zn-CO2, batteries. Here, the energy density and potential of Mg-CO2, and Zn-CO2, batteries are limited due to the use of an aqueous electrolyte which limits cell voltage greatly. Therefore, developing a non-aqueous electrolyte system for Mg-CO2, and Zn-CO2 that can improve overall energy density and overcoming the issues of anode corrosion and hydrogen evolution is highly suggested.
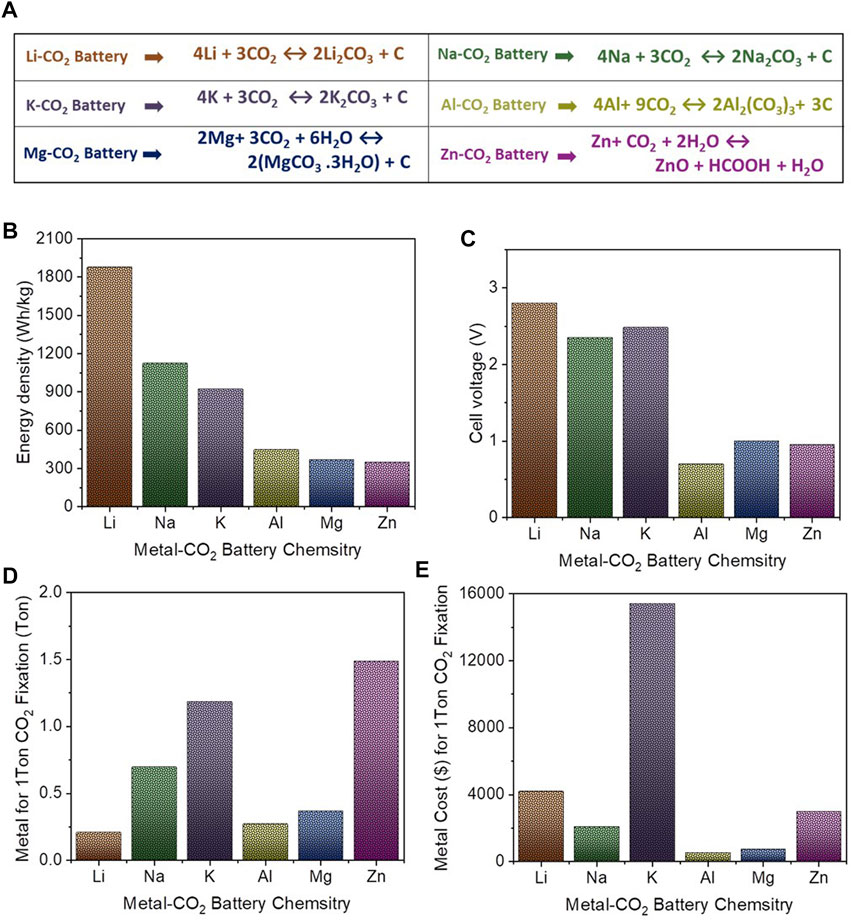
FIGURE 2. (A). Thermodynamic reaction of Li-CO2 (Sun X. et al., 2021), Na-CO2 (Hu et al., 2017b), K-CO2 (Zhang W. et al., 2020), Al-CO2 (Ma et al., 2018a), Mg-CO2 (Zhang W. et al., 2020), and Zn-CO2 (Xie et al., 2018), battery chemistries, (B). Comparative theoretical energy density of metal-CO2 batteries, (C). Comparative discharge potential of metal-CO2 batteries, (D). Comparative amount of metal anode required for 1 ton CO2 fixation in metal-CO2 batteries, (E). Comparative cost ($) of metal anode required for 1 ton CO2 fixation in metal-CO2 batteries [unit cost of metal is taken from Ref (Chao et al., 2020)].
Further, the amount of metal anode required for 1 ton CO2 fixation was also calculated (based on thermodynamic reaction) and compared with the cost of metal anode (Figures 2C,D). In metal anode chemistry, Al-CO2 batteries show comparatively good potential that requires 0.27 tons of Al for fixing 1 ton of CO2 at a cost of ∼$545 and that can produce energy density of 447 W h/kg. Also worth noting is that Al is a better choice when considering the availability and safety of alkali metals (e.g., Li, Na, K).
8 Summary
A Li-CO2 battery system based on a CO2 fixation and energy conversion mechanism can transform CO2 gases into electrical energy directly without using additional electricity/energy input. The system can be sustainable in terms of continuous utilization of CO2, production of electricity and other valuable products (e.g., methane in case of Zn-CO2 battery) in a CO2 environment. A metal-CO2 battery system can provide a competitive and cheaper technology for carbon capture and would open an industrial, scalable route for CO2 fixation and electrical energy generation. For example, the Al-CO2 system can capture 1 ton of CO2 with usage of approximately 0.27 tons of aluminum at the low cost of ∼$545. Also, a Li-CO2 battery system that can demonstrate high energy density seems to be a promising technology for energy storage for future Mars missions. Despite the advancements in development of Li-CO2 batteries, strategies to maximize their energy density and cycle life for practical application are still in great demand.
Author contributions
Writing, and original manuscript preparation: AP; writing, and review: PA: writing, editing and overall management: WC.
Conflict of interest
The authors declare that the research was conducted in the absence of any commercial or financial relationships that could be construed as a potential conflict of interest.
Publisher’s note
All claims expressed in this article are solely those of the authors and do not necessarily represent those of their affiliated organizations, or those of the publisher, the editors and the reviewers. Any product that may be evaluated in this article, or claim that may be made by its manufacturer, is not guaranteed or endorsed by the publisher.
References
Abbass, K., Qasim, M. Z., Song, H., Murshed, M., Mahmood, H., and Younis, I. (2022). A review of the global climate change impacts, adaptation, and sustainable mitigation measures. Environ. Sci. Pollut. Res. 29 (28), 42539–42559. doi:10.1007/s11356-022-19718-6
Ahmadiparidari, A., Warburton, R. E., Majidi, L., Asadi, M., Chamaani, A., Jokisaari, J. R., et al. (2019). A long-cycle-life lithium–CO2 battery with carbon neutrality. Adv. Mater. 31 (40), 1902518. doi:10.1002/adma.201902518
Azaiza-Dabbah, D., Vogt, C., Wang, F., Masip-Sánchez, A., de Graaf, C., Poblet, J. M., et al. (2022). Molecular transition metal oxide electrocatalysts for the reversible carbon dioxide–carbon monoxide transformation. Angew. Chem. Int. Ed. 61 (5), e202112915. doi:10.1002/anie.202112915
Baumann, A. E., Burns, D. A., Liu, B., and Thoi, V. S. (2019). Metal-organic framework functionalization and design strategies for advanced electrochemical energy storage devices. Commun. Chem. 2 (1), 86–14. doi:10.1038/s42004-019-0184-6
Bhoyate, S., Park, B., Oh, S. H., and Choi, W. (2021). Defect engineered MoWS alloy catalyst boost the polysulfide conversion in lithium–sulfur battery. J. Power Sources 511, 230426. doi:10.1016/j.jpowsour.2021.230426
Cai, F., Hu, Z., and Chou, S.-L. (2018). Progress and future perspectives on Li(Na)–CO2 batteries. Adv. Sustain. Syst. 2 (8–9), 1800060. doi:10.1002/adsu.201800060
Cao, D., Bai, Y., Zhang, J., Tan, G., and Wu, C. (2021). Irreplaceable carbon boosts Li-O2 batteries: From mechanism research to practical application. Nano Energy 89, 106464. doi:10.1016/j.nanoen.2021.106464
Cao, W., Zhang, J., and Li, H. (2020). Batteries with high theoretical energy densities. Energy Storage Mater. 26, 46–55. doi:10.1016/j.ensm.2019.12.024
Cha, E., Patel, M. D., Park, J., Hwang, J., Prasad, V., Cho, K., et al. (2018). Publisher correction: 2D MoS2 as an efficient protective layer for lithium metal anodes in high-performance Li–S batteries. Nat. Nanotechnol. 13 (6), 521. doi:10.1038/s41565-018-0095-1
Chang, Z., Xu, J., and Zhang, X. (2017). Recent progress in electrocatalyst for Li-O2 batteries. Adv. Energy Mater. 7 (23), 1700875. doi:10.1002/aenm.201700875
Chao, D., Zhou, W., Xie, F., Ye, C., Li, H., Jaroniec, M., et al. (2020). Roadmap for advanced aqueous batteries: From design of materials to applications. Sci. Adv. 6 (21), eaba4098. doi:10.1126/sciadv.aba4098
El Kharbachi, A., Zavorotynska, O., Latroche, M., Cuevas, F., Yartys, V., and Fichtner, M. (2020). Exploits, advances and challenges benefiting beyond Li-ion battery technologies. J. Alloys Compd. 817, 153261. doi:10.1016/j.jallcom.2019.153261
Franco, F., Rettenmaier, C., Jeon, H. S., and Cuenya, B. R. (2020). Transition metal-based catalysts for the electrochemical CO2 reduction: From atoms and molecules to nanostructured materials. Chem. Soc. Rev. 49 (19), 6884–6946. doi:10.1039/D0CS00835D
Ge, B., Sun, Y., Guo, J., Yan, X., Fernandez, C., and Peng, Q. (2019). A Co-doped MnO2 catalyst for Li-CO2 batteries with low overpotential and ultrahigh cyclability. Small 15 (34), 1902220. doi:10.1002/smll.201902220
Guan, D.-H., Wang, X.-X., Li, F., Zheng, L.-J., Li, M.-L., Wang, H.-F., et al. (2022). All-solid-state photo-assisted Li-CO2 battery working at an ultra-wide operation temperature. ACS Nano 16 (8), 12364–12376. doi:10.1021/acsnano.2c03534
Hao, X., An, X., Patil, A. M., Wang, P., Ma, X., Du, X., et al. (2021). Biomass-derived N-doped carbon for efficient electrocatalytic CO2 reduction to CO and Zn–CO2 batteries. ACS Appl. Mater. Interfaces 13 (3), 3738–3747. doi:10.1021/acsami.0c13440
Hou, Y., Wang, J., Liu, L., Liu, Y., Chou, S., Shi, D., et al. (2017). Mo2C/CNT: An efficient catalyst for rechargeable Li–CO2 batteries. Adv. Funct. Mater. 27 (27), 1700564. doi:10.1002/adfm.201700564
Hu, X., Li, Z., and Chen, J. (2017a). Flexible Li-CO2 batteries with liquid-free electrolyte. Angew. Chem. Int. Ed. 56 (21), 5785–5789. doi:10.1002/anie.201701928
Hu, X., Li, Z., Zhao, Y., Sun, J., Zhao, Q., Wang, J., et al. (2017b). Quasi–solid state rechargeable Na-CO2 batteries with reduced graphene oxide Na anodes. Sci. Adv. 3 (2), e1602396. doi:10.1126/sciadv.1602396
Huang, S., Chen, D., Meng, C., Wang, S., Ren, S., Han, D., et al. (2019). CO2 nanoenrichment and nanoconfinement in cage of imine covalent organic frameworks for high-performance CO2 cathodes in Li-CO2 batteries. Small 15 (49), 1904830. doi:10.1002/smll.201904830
Huang, Y., Lin, L., Zhang, C., Liu, L., Li, Y., Qiao, Z., et al. (2022). Recent advances and strategies toward polysulfides shuttle inhibition for high-performance Li–S batteries. Adv. Sci. 9 (12), 2106004. doi:10.1002/advs.202106004
Imanishi, N., and Yamamoto, O. (2014). Rechargeable lithium–air batteries: Characteristics and prospects. Mater. Today 17 (1), 24–30. doi:10.1016/j.mattod.2013.12.004
Ji, X., and Nazar, L. F. (2010). Advances in Li–S batteries. J. Mater. Chem. 20 (44), 9821–9826. doi:10.1039/B925751A
Jiao, Y., Qin, J., Sari, H. M. K., Li, D., Li, X., and Sun, X. (2021). Recent progress and prospects of Li-CO2 batteries: Mechanisms, catalysts and electrolytes. Energy Storage Mater. 34, 148–170. doi:10.1016/j.ensm.2020.09.014
Keith, D. W., Holmes, G., Angelo, D. S., and Heidel, K. (2018). A process for capturing CO2 from the atmosphere. Joule 2 (8), 1573–1594. doi:10.1016/j.joule.2018.05.006
Krane, J. (2017). Climate change and fossil fuel: An examination of risks for the energy industry and producer states. MRS Energy and Sustain. 4, E2. doi:10.1557/mre.2017.3
Lelieveld, J., Klingmüller, K., Pozzer, A., Burnett, R. T., Haines, A., and Ramanathan, V. (2019). Effects of fossil fuel and total anthropogenic emission removal on public health and climate. Proceed. Nation. Acad. Sci. U. S. A. 116 (15), 7192–7197. doi:10.1073/pnas.1819989116
Li, C., Guo, Z., Yang, B., Liu, Y., Wang, Y., and Xia, Y. (2017). A rechargeable Li-CO2 battery with a gel polymer electrolyte. Angew. Chem. Int. Ed. 56 (31), 9126–9130. doi:10.1002/anie.201705017
Li, H., Wang, Z., Chen, L., and Huang, X. (2009). Research on advanced materials for Li-ion batteries. Adv. Mater. 21 (45), 4593–4607. doi:10.1002/adma.200901710
Li, J., Wang, L., Zhao, Y., Li, S., Fu, X., Wang, B., et al. (2020). Li-CO2 batteries efficiently working at ultra-low temperatures. Adv. Funct. Mater. 30 (27), 2001619. doi:10.1002/adfm.202001619
Li, L., Dai, H., and Wang, C. (2021). Electrolyte additives: Adding the stability of lithium metal anodes. Nano Sel. 2 (1), 16–36. doi:10.1002/nano.202000164
Li, S., Dong, Y., Zhou, J., Liu, Y., Wang, J., Gao, X., et al. (2018). Carbon dioxide in the cage: Manganese metal–organic frameworks for high performance CO2 electrodes in Li–CO2 batteries. Energy and Environ. Sci. 11 (5), 1318–1325. doi:10.1039/C8EE00415C
Li, W., Guo, X., Geng, P., Du, M., Jing, Q., Chen, X., et al. (2021). Rational design and general synthesis of multimetallic metal–organic framework nano-octahedra for enhanced Li–S battery. Adv. Mater. 33 (45), 2105163. doi:10.1002/adma.202105163
Li, X., Wang, H., Chen, Z., Xu, H.-S., Yu, W., Liu, C., et al. (2019). Covalent-organic-framework-based Li–CO2 batteries. Adv. Mater. 31 (48), 1905879. doi:10.1002/adma.201905879
Li, X., Zhang, J., Qi, G., Cheng, J., and Wang, B. (2021). Vertically aligned N-doped carbon nanotubes arrays as efficient binder-free catalysts for flexible Li-CO2 batteries. Energy Storage Mater. 35, 148–156. doi:10.1016/j.ensm.2020.11.020
Li, Y., and Lu, J. (2017). Metal–air batteries: Will they Be the future electrochemical energy storage device of choice? ACS Energy Lett. 2 (6), 1370–1377. doi:10.1021/acsenergylett.7b00119
Liu, C., Cundari, T. R., and Wilson, A. K. (2012). CO2 reduction on transition metal (Fe, Co, Ni, and Cu) surfaces: In comparison with homogeneous catalysis. J. Phys. Chem. C 116 (9), 5681–5688. doi:10.1021/jp210480c
Liu, L., Zhang, L., Wang, K., Wu, H., Mao, H., Li, L., et al. (2020). Understanding the dual-phase synergy mechanism in Mn2O3–Mn3O4 catalyst for efficient Li–CO2 batteries. ACS Appl. Mater. Interfaces 12 (30), 33846–33854. doi:10.1021/acsami.0c09644
Liu, S., Wang, L., Yang, H., Gao, S., Liu, Y., Zhang, S., et al. (2022). Nitrogen-doped carbon polyhedrons confined Fe–P nanocrystals as high-efficiency bifunctional catalysts for aqueous Zn−CO2 batteries. Small 18 (10), 2104965. doi:10.1002/smll.202104965
Liu, T., Vivek, J. P., Zhao, E. W., Lei, J., Garcia-Araez, N., and Grey, C. P. (2020). Current challenges and routes forward for nonaqueous lithium–air batteries. Chem. Rev. 120 (14), 6558–6625. doi:10.1021/acs.chemrev.9b00545
Liu, W., Feng, J., Wei, T., Liu, Q., Zhang, S., Luo, Y., et al. (2022). Active-site and interface engineering of cathode materials for aqueous Zn—gas batteries. Nano Res. 16, 2325–2346. doi:10.1007/s12274-022-4929-7
Liu, Y., Wang, R., Lyu, Y., Li, H., and Chen, L. (2014). Rechargeable Li/CO2–O2 (2 : 1) battery and Li/CO2 battery. Energy and Environ. Sci. 7 (2), 677–681. doi:10.1039/C3EE43318H
Lu, Z., Xiao, M., Wang, S., Han, D., Huang, Z., Huang, S., et al. (2022). A rechargeable Li–CO2 battery based on the preservation of dimethyl sulfoxide. J. Mater. Chem. A 10 (26), 13821–13828. doi:10.1039/D2TA02586H
Lv, H., Huang, X. L., Zhu, X., and Wang, B. (2022). Metal-related electrocatalysts for Li–CO2 batteries: An overview of the fundamentals to explore future-oriented strategies. J. Mater. Chem. A 10 (48), 25406–25430. doi:10.1039/D2TA05756E
Ma, W., Liu, X., Li, C., Yin, H., Xi, W., Liu, R., et al. (2018a). Rechargeable Al–CO2 batteries for reversible utilization of CO2. Adv. Mater. 30 (28), 1801152. doi:10.1002/adma.201801152
Ma, W., Lu, S., Lei, X., Liu, X., and Ding, Y. (2018b). Porous Mn2O3 cathode for highly durable Li–CO2 batteries. J. Mater. Chem. A 6 (42), 20829–20835. doi:10.1039/C8TA06143B
Mahmud, S., Rahman, M., Kamruzzaman, M., Ali, M. O., Emon, M. S. A., Khatun, H., et al. (2022). Recent advances in lithium-ion battery materials for improved electrochemical performance: A review. Results Eng. 15, 100472. doi:10.1016/j.rineng.2022.100472
Na, D., Jeong, H., Baek, J., Yu, H., Lee, S.-M., Lee, C.-R., et al. (2022). Highly safe and stable Li–CO2 batteries using conducting ceramic solid electrolyte and MWCNT composite cathode. Electrochimica Acta 419, 140408. doi:10.1016/j.electacta.2022.140408
Nam, S. C., Yoon, Y. S., Cho, W. I., Cho, B. W., Chun, H. S., and Yun, K. S. (2001). Reduction of irreversibility in the first charge of tin oxide thin film negative electrodes. J. Electrochem. Soc. 148 (3), A220. doi:10.1149/1.1346603
Pathak, A. D., and Sharma, C. S. (2021). Candle soot carbon cathode for rechargeable Li-CO2-Mars battery chemistry for Mars exploration: A feasibility study. Mater. Lett. 283, 128868. doi:10.1016/j.matlet.2020.128868
Pipes, R., Bhargav, A., and Manthiram, A. (2018). Nanostructured anatase titania as a cathode catalyst for Li–CO2 batteries. ACS Appl. Mater. Interfaces 10 (43), 37119–37124. doi:10.1021/acsami.8b13910
Pipes, R., He, J., Bhargav, A., and Manthiram, A. (2019). Efficient Li–CO2 batteries with molybdenum disulfide nanosheets on carbon nanotubes as a catalyst. ACS Appl. Energy Mater. 2 (12), 8685–8694. doi:10.1021/acsaem.9b01653
Qiao, Y., Yi, J., Wu, S., Liu, Y., Yang, S., He, P., et al. (2017). Li-CO2 electrochemistry: A new strategy for CO2 fixation and energy storage. Joule 1 (2), 359–370. doi:10.1016/j.joule.2017.07.001
Rho, Y.-J., Kim, B., Shin, K., Henkelman, G., and Ryu, W.-H. (2022). Atomically miniaturized bi-phase IrOx/Ir catalysts loaded on N-doped carbon nanotubes for high-performance Li–CO2 batteries. J. Mater. Chem. A 10 (37), 19710–19721. doi:10.1039/D2TA02234F
Shui, J.-L., Okasinski, J. S., Kenesei, P., Dobbs, H. A., Zhao, D., Almer, J. D., et al. (2013). Reversibility of anodic lithium in rechargeable lithium–oxygen batteries. Nat. Commun. 4 (1), 2255. doi:10.1038/ncomms3255
Song, L., Hu, C., Xiao, Y., He, J., Lin, Y., Connell, J. W., et al. (2020). An ultra-long life, high-performance, flexible Li–CO2 battery based on multifunctional carbon electrocatalysts. Nano Energy 71, 104595. doi:10.1016/j.nanoen.2020.104595
Song, L., Wang, T., Wu, C., Fan, X., and He, J. (2019). A long-life Li–CO2 battery employing a cathode catalyst of cobalt-embedded nitrogen-doped carbon nanotubes derived from a prussian blue analogue. Chem. Commun. 55 (85), 12781–12784. doi:10.1039/C9CC05392A
Sun, X., Hou, Z., He, P., and Zhou, H. (2021). Recent advances in rechargeable Li–CO2 batteries. Energy and Fuels 35 (11), 9165–9186. doi:10.1021/acs.energyfuels.1c00635
Sun, Y., Chen, G., Xi, S., and Xu, Z. J. (2021). Catalytically influential features in transition metal oxides. ACS Catal. 11 (22), 13947–13954. doi:10.1021/acscatal.1c04393
Tang, W., Li, B., Teng, K., Wang, X., Liu, R., Wu, M., et al. (2022). Advanced noble-metal-free bifunctional electrocatalysts for metal-air batteries. J. Materiomics 8 (2), 454–474. doi:10.1016/j.jmat.2021.07.001
Wang, C., Zhang, Q., Zhang, X., Wang, X.-G., Xie, Z., and Zhou, Z. (2018). Fabricating Ir/C nanofiber networks as free-standing air cathodes for rechargeable Li-CO2 batteries. Small 14 (28), 1800641. doi:10.1002/smll.201800641
Wang, H., Xie, K., You, Y., Hou, Q., Zhang, K., Li, N., et al. (2019). Realizing interfacial electronic interaction within ZnS quantum dots/N-rgo heterostructures for efficient Li–CO2 batteries. Adv. Energy Mater. 9 (34), 1901806. doi:10.1002/aenm.201901806
Wang, K., Wu, Y., Cao, X., Gu, L., and Hu, J. (2020). A Zn–CO2 flow battery generating electricity and methane. Adv. Funct. Mater. 30 (9), 1908965. doi:10.1002/adfm.201908965
Wang, L., Pan, J., Zhang, Y., Cheng, X., Liu, L., and Peng, H. (2018). A Li–air battery with ultralong cycle life in ambient air. Adv. Mater. 30 (3), 1704378. doi:10.1002/adma.201704378
Wonbong, C., Nitin, C., Juhong, P., Deji, A., and Younghee, L. (2017). Recent development of two-dimensional transition metal dichalcogenides and their applications. Materials Today 20, 116–130.
Xiao, J., Wang, D., Xu, W., Wang, D., Williford, R. E., Liu, J., et al. (2010). Optimization of air electrode for Li/air batteries. J. Electrochem. Soc. 157 (4), A487. doi:10.1149/1.3314375
Xie, J., Wang, X., Lv, J., Huang, Y., Wu, M., Wang, Y., et al. (2018). Reversible aqueous zinc–CO2 batteries based on CO2–HCOOH interconversion. Angew. Chem. Int. Ed. 57 (52), 16996–17001. doi:10.1002/anie.201811853
Xie, J., and Wang, Y. (2019). Recent development of CO2 electrochemistry from Li–CO2 batteries to Zn–CO2 batteries. Accounts Chem. Res. 52 (6), 1721–1729. doi:10.1021/acs.accounts.9b00179
Xu, K. (2004). Nonaqueous liquid electrolytes for lithium-based rechargeable batteries. Chem. Rev. 104 (10), 4303–4418. doi:10.1021/cr030203g
Yang, C., Guo, K., Yuan, D., Cheng, J., and Wang, B. (2020). Unraveling reaction mechanisms of Mo2C as cathode catalyst in a Li-CO2 battery. J. Am. Chem. Soc. 142 (15), 6983–6990. doi:10.1021/jacs.9b12868
Yang, M., Liu, S., Sun, J., Jin, M., Fu, R., Zhang, S., et al. (2022a). Highly dispersed Bi clusters for efficient rechargeable Zn−CO2 batteries. Appl. Catal. B Environ. 307, 121145. doi:10.1016/j.apcatb.2022.121145
Yang, M., Liu, Y., Sun, J., Zhang, S., Liu, X., and Luo, J. (2022b). Integration of partially phosphatized bimetal centers into trifunctional catalyst for high-performance hydrogen production and flexible Zn-air battery. Sci. China Mater. 65 (5), 1176–1186. doi:10.1007/s40843-021-1902-2
Yang, Z., Guo, H., Li, F., Li, X., Wang, Z., Cui, L., et al. (2018). Cooperation of nitrogen-doping and catalysis to improve the Li-ion storage performance of lignin-based hard carbon. J. Energy Chem. 27 (5), 1390–1396. doi:10.1016/j.jechem.2018.01.013
Yu, Z., Cui, Y., and Bao, Z. (2020). Design principles of artificial solid electrolyte interphases for lithium-metal anodes. Cell Rep. Phys. Sci. 1 (7), 100119. doi:10.1016/j.xcrp.2020.100119
Zhang, P.-F., Zhang, J.-Y., Sheng, T., Lu, Y.-Q., Yin, Z.-W., Li, Y.-Y., et al. (2020). Synergetic effect of Ru and NiO in the electrocatalytic decomposition of Li2CO3 to enhance the performance of a Li-CO2/O2 battery. ACS Catal. 10 (2), 1640–1651. doi:10.1021/acscatal.9b04138
Zhang, S., Liu, X., Feng, Y., Wang, D., Yin, H., Chi, Z., et al. (2021b). Protecting Li-metal anode with ethylenediamine-based layer and in-situ formed gel polymer electrolyte to construct the high-performance Li–CO2 battery. J. Power Sources 506, 230226. doi:10.1016/j.jpowsour.2021.230226
Zhang, S., Chen, M., Zhao, X., Cai, J., Yan, W., Yen, J. C., et al. (2021a). Advanced noncarbon materials as catalyst supports and non-noble electrocatalysts for fuel cells and metal–air batteries. Electrochem. Energy Rev. 4 (2), 336–381. doi:10.1007/s41918-020-00085-0
Zhang, S., Sun, L., Fan, Q., Zhang, F., Wang, Z., Zou, J., et al. (2022). Challenges and prospects of lithium–CO2 batteries. Nano Res. Energy 1 (1), e9120001. doi:10.26599/NRE.2022.9120001
Zhang, T., Tao, Z., and Chen, J. (2014). Magnesium–air batteries: From principle to application. Mater. Horizons 1 (2), 196–206. doi:10.1039/C3MH00059A
Zhang, W., Hu, C., Guo, Z., and Dai, L. (2020). High-performance K–CO2 batteries based on metal-free carbon electrocatalysts. Angew. Chem. Int. Ed. 59 (9), 3470–3474. doi:10.1002/anie.201913687
Zhang, X., Wang, C., Li, H., Wang, X-G., Chen, Y-N., Xie, Z., et al. (2018). High performance Li–CO2 batteries with NiO–CNT cathodes. J. Mater. Chem. A 6 (6), 2792–2796. doi:10.1039/C7TA11015D
Zhang, X., Zhang, Q., Zhang, Z., Chen, Y., Xie, Z., Wei, J., et al. (2015). Rechargeable Li–CO2 batteries with carbon nanotubes as air cathodes. Chem. Commun. 51 (78), 14636–14639. doi:10.1039/C5CC05767A
Zhang, Z., Bai, W.-L., Wang, K.-X., and Chen, J.-S. (2020). Electrocatalyst design for aprotic Li–CO2 batteries. Energy and Environ. Sci. 13 (12), 4717–4737. doi:10.1039/D0EE03058A
Zhao, J., Wei, D., Zhang, C., Shao, Q., Murugadoss, V., Guo, Z., et al. (2021). An overview of oxygen reduction electrocatalysts for rechargeable zinc-air batteries enabled by carbon and carbon composites. Eng. Sci. 15, 1–19. doi:10.30919/es8d420
Zhao, R., Liang, Z., Zou, R., and Xu, Q. (2018). Metal-organic frameworks for batteries. Joule 2 (11), 2235–2259. doi:10.1016/j.joule.2018.09.019
Zhao, Z., Su, Y., and Peng, Z. (2019). Probing lithium carbonate formation in trace-O2-assisted aprotic Li-CO2 batteries using in situ surface-enhanced Raman spectroscopy. J. Phys. Chem. Lett. 10 (3), 322–328. doi:10.1021/acs.jpclett.8b03272
Zhou, J., Wang, T., Chen, L., Liao, L., Wang, Y., Xi, S., et al. (2022). Boosting the reaction kinetics in aprotic lithium-carbon dioxide batteries with unconventional phase metal nanomaterials. Proc. Natl. Acad. Sci. 119 (40), e2204666119. doi:10.1073/pnas.2204666119
Keywords: Li-CO2 batteries, Li-O2 batteries, metal-CO2 batteries, CO2 fixation, greenhouse emission
Citation: Pathak AD, Adhikari PR and Choi W (2023) Lithium-CO2 batteries and beyond. Front. Energy Res. 11:1150737. doi: 10.3389/fenrg.2023.1150737
Received: 24 January 2023; Accepted: 22 February 2023;
Published: 03 March 2023.
Edited by:
Sheng S. Zhang, United States Army Research Laboratory, United StatesReviewed by:
Xijun Liu, Guangxi University, ChinaCopyright © 2023 Pathak, Adhikari and Choi. This is an open-access article distributed under the terms of the Creative Commons Attribution License (CC BY). The use, distribution or reproduction in other forums is permitted, provided the original author(s) and the copyright owner(s) are credited and that the original publication in this journal is cited, in accordance with accepted academic practice. No use, distribution or reproduction is permitted which does not comply with these terms.
*Correspondence: Wonbong Choi, V29uYm9uZy5jaG9pQHVudC5lZHU=