- 1Department of Mechanical Engineering, University of Louisville, Louisville, KY, United States
- 2Department of Physics and Astronomy, University of Louisville, Louisville, KY, United States
Solid-state refrigeration represents a promising alternative to vapor compression refrigeration systems which are inefficient, unreliable, and have a high global warming potential. However, several solid-state cooling technologies—including those relying on a temperature change induced by an applied electric field (electrocaloric effect), magnetic field (magnetocaloric effect), and uniaxial stress (elastocaloric effect)—have been investigated, but their efficiency and scalability remain a concern. Materials with a large barocaloric response—temperature/entropy change induced by hydrostatic pressure—hold a significant promise for solid-state cooling but remain comparatively less explored. These materials need to be inexpensive, compressible, and show a large barocaloric response around the temperature of interest. Soft materials have the potential to meet these requirements and enable the development of low-cost high-efficiency solid-state heat pumps. Here, we investigate the barocaloric performance of commercially available block copolymer thermoplastic elastomers. We characterized the mechanical, thermal, and barocaloric properties of these materials and evaluated their potential for solid-state refrigeration. We utilized rheometric measurements to evaluate the isothermal compressibility and normalized refrigerant capacity of the thermoplastic elastomers. In addition, we directly measured the pressure-induced temperature change of the test materials and compared them with their normalized refrigeration capacity. The measured isothermal compressibility was in the 0.1–0.4 GPa−1 range, while the normalized refrigeration capacity varied between 13.2 and 41.9 kJ K−1 GPa−1 for a 100 MPa applied pressure and 65°C temperature span. The corresponding pressure-induced temperature change for an applied pressure of 434.1 MPa varied between 2.2 and 28°C. These results demonstrated the superior barocaloric properties of thermoplastic elastomers and their promise for next generation barocaloric solid-state refrigeration devices.
Introduction
Air conditioning and refrigeration account for about a quarter of global total energy demand (US Energy Information Administration, 2021). These cooling needs are primarily met by vapor compression systems which are inefficient, unreliable, and have a high global warming potential (de Paula et al., 2020; Pol Lloveras and Josep-Lluís Tamarit, 2021). Solid-state refrigeration (SSR)—utilizing the caloric response of materials subjected to external stimuli—provides a promising alternative to the vapor compression technology and has consequently received significant attention over the last three decades (Greco et al., 2019). Recent studies have investigated a wide variety of materials for SSR when subjected to an external electric field (based on the electrocaloric effect), magnetic field (based on the magnetocaloric effect), uniaxial stress (based on the elastocaloric effect), or hydrostatic pressure (based on the barocaloric effect) (Kitanovski et al., 2015). However, solid-state cooling technologies are not yet competitive with vapor compression systems and require investigation of different material systems and operational modalities (Kitanovski et al., 2015; Qian et al., 2016).
Solid-state cooling based on the barocaloric (BC) effect is among the least studied caloric technologies but has received a significant interest in the last few years due to the large barocaloric responses reported in several material classes (Aprea C. et al., 2019; C.; Aprea et al., 2018a; Aprea G. et al., 2019; Aznar et al., 2020; Garcia-Ben et al., 2021; Greco et al., 2019; Miliante et al., 2020; Min et al., 2020; Moya and Mathur, 2020; Lloveras and Tamarit, 2021). The barocaloric response of a material subjected to hydrostatic pressure is characterized by the resulting isothermal entropy change (
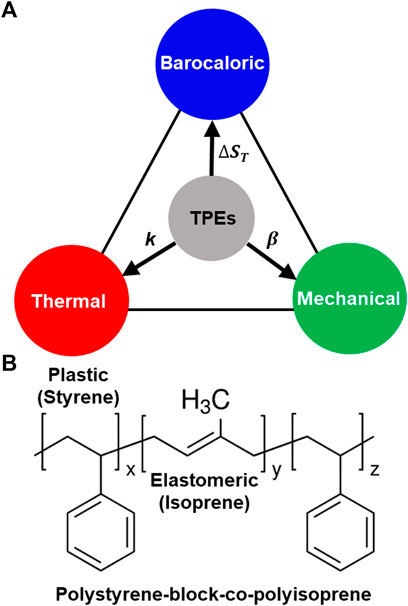
FIGURE 1. Thermoplastic elastomers (TPEs) as promising barocaloric materials. (A) Different material properties relevant for solid-state refrigeration based on the barocaloric effect. These include compressibility (
The BC effect has been demonstrated and investigated in a wide variety of materials (Boldrin, 2021) including soft materials, organic–inorganic salts, perovskites, and magnetic shape memory alloys (Mañosa et al., 2010; Patel et al., 2016; Li et al., 2019; Miliante et al., 2020). Despite their typically low thermal conductivity, soft materials are particularly attractive due to their large BC response, high compressibility, and low cost. One class of soft materials that has received significant attention is plastic crystals whose first-order phase transitions near room temperature lead to colossal
Although several polymers have demonstrated encouraging barocaloric properties, a class of soft materials that has not yet been investigated is thermoplastic elastomers (TPEs). TPEs are polymers with two distinct building blocks—a thermoplastic (hard) part comprising a glassy/semicrystalline material and an elastomeric (soft) part comprising a pure amorphous material (Figure 1B) (Roth et al., 2020). The soft elastomeric component confers high compressibility and a high BC response due to the large pressure-induced conformational changes in the polymer, while the semicrystalline component increases the phonon density of states, enhancing the thermal transport (dos Santos et al., 2008; Xu et al., 2020). Thus, TPEs represent a promising class of materials that can simultaneously achieve high isothermal volumetric compressibility (
In this work, we characterized the barocaloric and related properties of commercially available TPEs. The pressure–volume–temperature (
Materials
We investigated barocaloric and related properties of block copolymer TPEs near room temperature (20–100°C). Block copolymers are widely accessible at a low cost from numerous manufacturers. Table 1 lists the TPEs studied and their key components. For this study, we identified commercially important TPEs based on styrenic block copolymers, thermoplastic copolyesters, thermoplastic polyurethanes, ethylene-based copolymers, and thermoplastic polyamides (Holden, 1987). In this work, we tested nine TPEs and one pure thermoplastic material (ZYTEL) chosen based on their commercial availability. The material selection also ensured the TPEs were usable at room temperature, that is, the glass transition temperature of the elastomeric part was lower than room temperature, and the glass transition temperature of the thermoplastic part was sufficiently higher than room temperature. All materials obtained were in the form of millimeter-scale pellets (additional details regarding the material suppliers are provided in Supplementary Section S1).
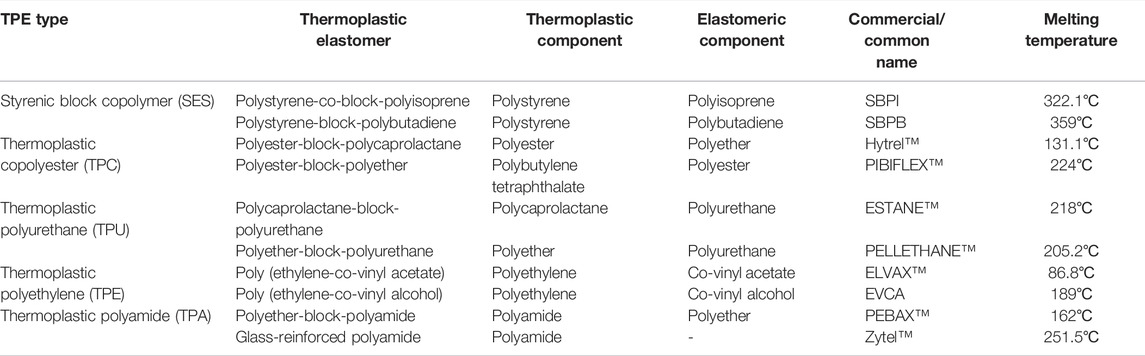
TABLE 1. List of materials studied. Thermoplastic elastomers (TPEs) tested, their classification, composition and common name, and melting temperature at ambient pressure and temperature obtained from DSC measurements.
Methods
We performed material characterization to obtain mechanical, thermal and BC properties by rheometry, differential scanning calorimetry (DSC), and quasi-adiabatic direct measurement techniques. Atmospheric pressure DSC measurements were initially used to characterize the melting temperatures of different materials and confirm their suitability for the study. We also used the DSC data to identify other relevant (solid–solid) phase transitions near room temperature and evaluate the specific heat capacity of the material (Supplementary Section S2). In addition, we performed direct barocaloric characterization by measuring the temperature change induced in each material when subjected to hydrostatic pressure. These quasi-adiabatic measurements were performed near ambient temperature (
Isothermal Compressibility
To determine the isothermal compressibility (
Figure 2A presents
where
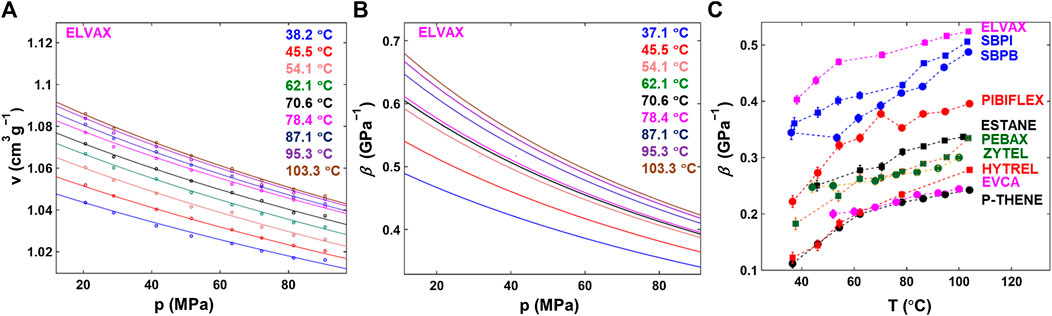
FIGURE 2. Mechanical property measurement. (A)
Thermal Conductivity
The thermal conductivity (
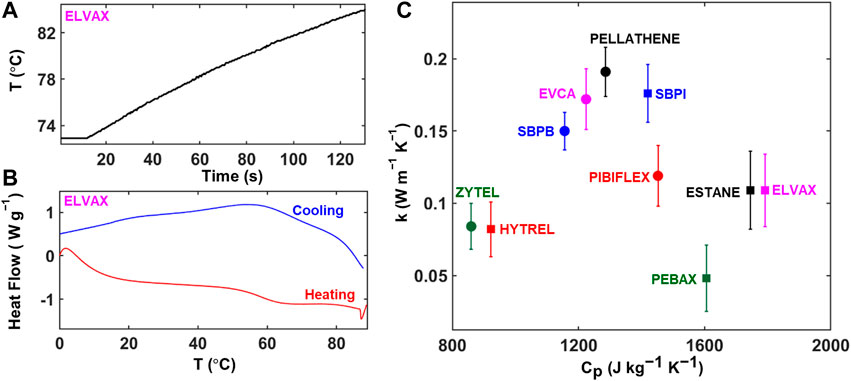
FIGURE 3. Thermal property measurement. (A) Transient temperature profile from the thermal conductivity measurement for a representative material (ELVAX). (B) DSC endo- and exotherms used for specific heat capacity calculation shown for ELVAX. (C) Plot showing the melt thermal conductivity and specific heat capacity at 35°C for the TPEs tested.
Figure 3C shows the measured thermal conductivity and specific heat capacity for all materials tested to compare their heat transfer properties. As would be expected from thermally insulating polymers, the thermal conductivities of the materials tested are relatively low (<0.2 W m−1 K−1; measurement uncertainty <0.05 W m−1 K−1). The thermal conductivities are, however, comparable to those of typical elastomers (e.g., silicone rubber
Barocaloric Properties
Maxwell’s relations are used to calculate the BC isothermal entropy change (
where
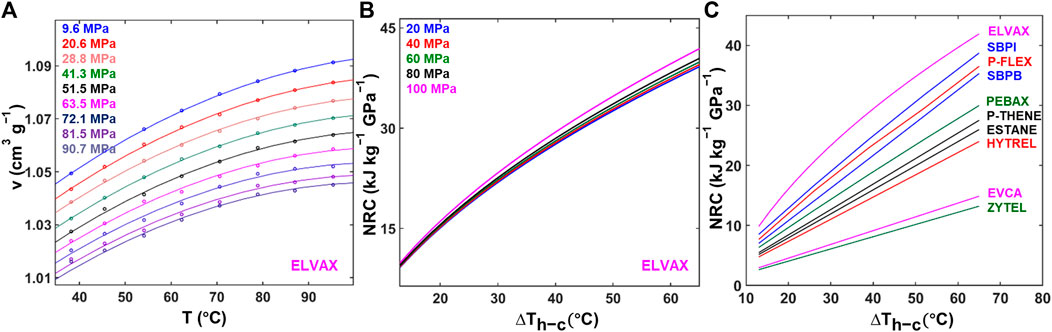
FIGURE 4. Barocaloric property measurement. (A)
This NRC value is dependent on the temperature range of operation
Figure 4B shows the NRC of ELVAX as a function of
Results and Discussion
The performance of solid-state materials as refrigerants for SSR is dependent on their barocaloric, mechanical, and thermal properties. Figure 5 summarizes the barocaloric property measurements—showing the normalized refrigerant capacity (at
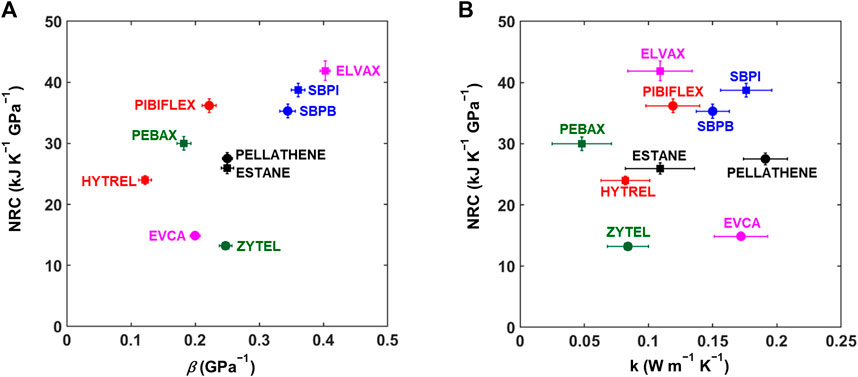
FIGURE 5. Mechanical, thermal, and barocaloric properties. (A) Normalized refrigerant capacity (NRC, shown for
In addition to rheometric measurements, we also used a custom-fabricated quasi-adiabatic test setup similar to that reported in the literature (Bom et al., 2018) to directly measure the pressure-induced temperature change of all materials. Figures 6A,B show the test rig used to measure the BC response of the polymers around ambient temperature (
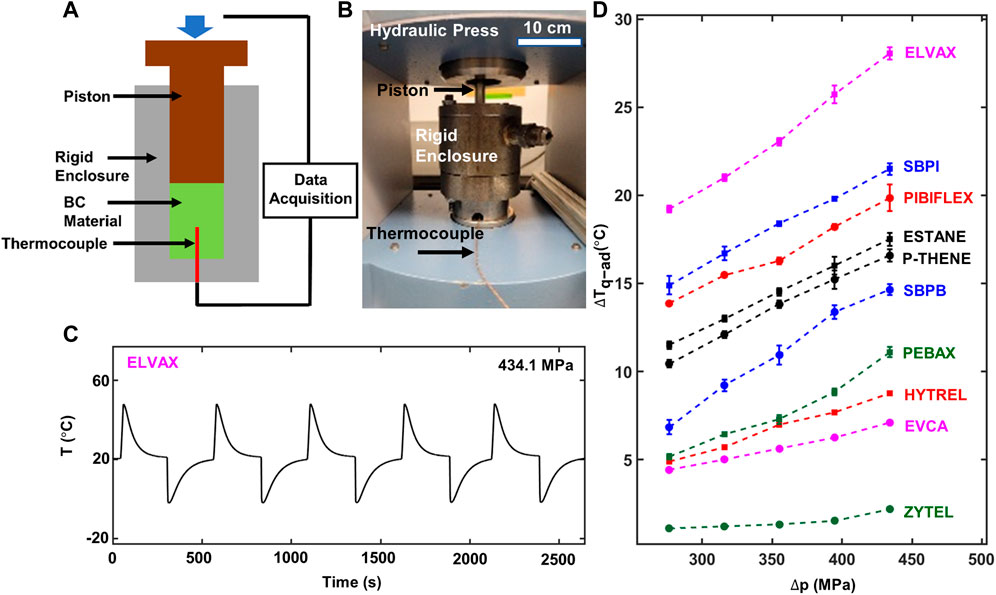
FIGURE 6. Direct barocaloric temperature change measurement. (A) Schematic representation and (B) photograph of the custom-fabricated setup for the direct measurement of the pressure-induced temperature change. (C) Measured temperature of a representative material (ELVAX) subjected to five pressure (
Figure 6D shows the measured quasi-adiabatic temperature change (
The data from the quasi-adiabatic approach can be compared with the results obtained using rheometric measurements. Figure 7 shows the barocaloric NRC (for
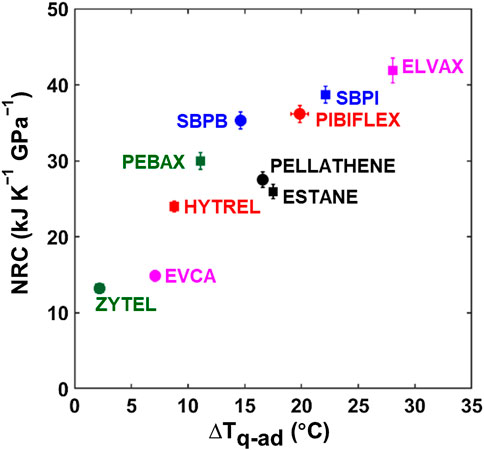
FIGURE 7. Direct versus indirect barocaloric measurement. Comparison of the normalized refrigeration capacity (NRC, for
The performance of barocaloric materials is dependent upon their compressibility, thermal conductivity and heat capacity, and BC entropy change. Among the materials studied in this study, the highest NRC and
Another key metric relevant for the performance of a refrigerator is the coefficient of performance (COP). To predict the performance of the tested barocaloric materials as solid-state refrigerants, we evaluated the material COP using the methods described in the literature (Carvalho et al., 2018; Schmidt et al., 2016; Usuda et al., 2019). Details are given in Supplementary Section S8. Table 2 lists the predicted COP values of the tested materials calculated for a working temperature of 35°C and compared with the barocaloric performance of other soft materials reported in the literature. The COP values of the TPEs studied in this work are comparable to those reported for pure elastomeric compounds (Carvalho et al., 2018; Usuda et al., 2019). The COP values are also consistent with the NRC values described earlier, demonstrating ELVAX, SBPI and SBPB as promising barocaloric materials for refrigeration.
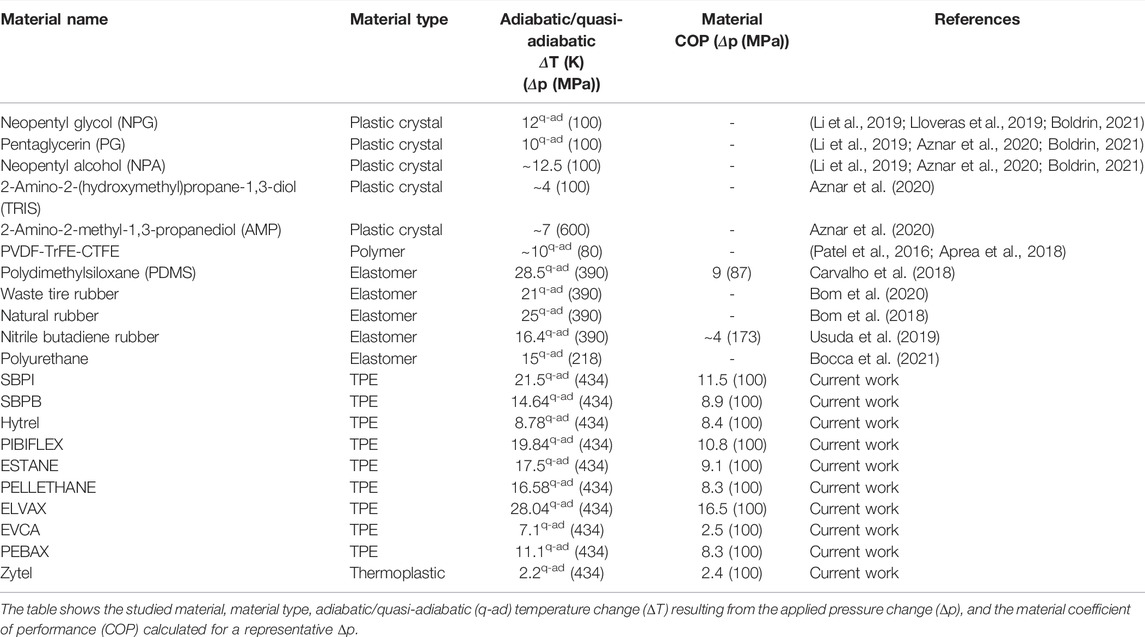
TABLE 2. Comparison of the current work with previous studies on barocaloric properties of soft materials.
Conclusion
This study reports a large barocaloric response in TPEs at relatively low applied pressures ≲100 MPa. We identified and experimentally measured mechanical, thermal and BC properties that are relevant for SSR. Mechanical characterization relied upon the volumetric compressibility, while thermal characterization focused on the thermal conductivity of the materials. The BC properties of the materials were quantified by their NRC evaluated using rheometric measurements and directly measured quasi-adiabatic temperature change. The rheometric
Data Availability Statement
The original contributions presented in the study are included in the article/Supplementary Material; further inquiries can be directed to the corresponding author.
Author Contributions
BB and NW conceptualized the project. NW performed the DSC measurements. NW, KPKA, and KS conducted the rheological material characterization. NW designed and fabricated the setup and performed the direct barocaloric temperature change measurements. NW collected and analyzed all the data with guidance from BB and GS. NW and BB wrote the manuscript with input from all authors. BB, KK, and GS supervised and guided the project.
Funding
The material is based upon work supported by the NASA Kentucky under the NASA award number 80NSSC20M0047.
Conflict of Interest
The authors declare that the research was conducted in the absence of any commercial or financial relationships that could be construed as a potential conflict of interest.
Publisher’s Note
All claims expressed in this article are solely those of the authors and do not necessarily represent those of their affiliated organizations, or those of the publisher, the editors, and the reviewers. Any product that may be evaluated in this article, or claim that may be made by its manufacturer, is not guaranteed or endorsed by the publisher.
Acknowledgments
We would like to thank Dr. Ian McKinley (NASA JPL, United States) for his guidance and support throughout this project. We would also like to thank Dr. Erik Usuda (CNPEM, Brazil) for advising us on the design and operation of the quasi-adiabatic temperature measurement setup.
Supplementary Material
The Supplementary Material for this article can be found online at: https://www.frontiersin.org/articles/10.3389/fenrg.2022.887006/full#supplementary-material
References
Aprea, C., Greco, A., Maiorino, A., and Masselli, C. (2019a). Enhancing the Heat Transfer in an Active Barocaloric Cooling System Using Ethylene-Glycol Based Nanofluids as Secondary Medium. Energies 12, 2902. doi:10.3390/en12152902
Aprea, C., Greco, A., Maiorino, A., and Masselli, C. (2018a). Solid-State Refrigeration: A Comparison of the Energy Performances of Caloric Materials Operating in an Active Caloric Regenerator. Energy 165, 439–455. doi:10.1016/j.energy.2018.09.114
Aprea, C., Greco, A., Maiorino, A., and Masselli, C. (2018b). The Environmental Impact of Solid-State Materials Working in an Active Caloric Refrigerator Compared to a Vapor Compression Cooler. IJHT 36, 1155–1162. doi:10.18280/ijht.360401
Aprea, C., Greco, A., Maiorino, A., and Masselli, C. (2020). The Use of Barocaloric Effect for Energy Saving in a Domestic Refrigerator with Ethylene-Glycol Based Nanofluids: A Numerical Analysis and a Comparison with a Vapor Compression Cooler. Energy 190, 116404. doi:10.1016/j.energy.2019.116404
Aprea, G., Greco, M., Maiorino, A., and Masselli, C. (2019b). Is Barocaloric an Eco-Friendly Technology? A TEWI Comparison with Vapor Compression Under Different Operation Modes. Climate 7, 115. doi:10.3390/cli7090115
Aznar, A., Lloveras, P., Barrio, M., Negrier, P., Planes, A., Mañosa, L., et al. (2020). Reversible and Irreversible Colossal Barocaloric Effects in Plastic Crystals. J. Mat. Chem. A 8, 639–647. doi:10.1039/C9TA10947A
Bocca, J. R., Favaro, S. L., Alves, C. S., Carvalho, A. M. G., Barbosa, J. R., Santos, A. D., et al. (2021). Giant Barocaloric Effect in Commercial Polyurethane. Polym. Test. 100, 107251. doi:10.1016/j.polymertesting.2021.107251
Boldrin, D. (2021). Fantastic Barocalorics and Where to Find Them. Appl. Phys. Lett. 118, 170502. doi:10.1063/5.0046416
Bom, N. M., Imamura, W., Usuda, E. O., Paixão, L. S., and Carvalho, A. M. G. (2018). Giant Barocaloric Effects in Natural Rubber: A Relevant Step Toward Solid-State Cooling. ACS Macro Lett. 7, 31–36. doi:10.1021/acsmacrolett.7b00744
Bom, N. M., Usuda, É. O., da Silva Gigliotti, M., de Aguiar, D. J. M., Imamura, W., Paixão, L. S., et al. (2020). Waste Tire Rubber-Based Refrigerants for Solid-State Cooling Devices. Chin. J. Polym. Sci. 38, 769–775. doi:10.1007/s10118-020-2385-y
Brogly, M., Nardin, M., and Schultz, J. (1998). Effect of Vinylacetate Content on Crystallinity and Second- Order Transitions in Ethylene–Vinylacetate Copolymers. Appl. Polym. Sci. 64, 1903–1912. doi:10.1002/(SICI)1097-4628(19970606)64:10<1903::AID-APP4>3.0.CO;2-M
Carvalho, A. M. G., Imamura, W., Usuda, E. O., and Bom, N. M. (2018). Giant Room-Temperature Barocaloric Effects in PDMS Rubber at Low Pressures. Eur. Polym. J. 99, 212–221. doi:10.1016/j.eurpolymj.2017.12.007
de Paula, C. H., Duarte, W. M., Rocha, T. T. M., de Oliveira, R. N., Mendes, R. d. P., and Maia, A. A. T. (2020). Thermo-Economic and Environmental Analysis of a Small Capacity Vapor Compression Refrigeration System Using R290, R1234yf, and R600a. Int. J. Refrig. 118, 250–260. doi:10.1016/j.ijrefrig.2020.07.003
dos Santos, W. N., Iguchi, C. Y., and Gregorio, R. (2008). Thermal Properties of Poly(Vinilidene Fluoride) in the Temperature Range from 25 to 210 °C. Polym. Test. 27, 204–208. doi:10.1016/j.polymertesting.2007.10.005
Fazli, A., and Rodrigue, D. (2020). Waste Rubber Recycling: A Review on the Evolution and Properties of Thermoplastic Elastomers. Materials 13, 782. doi:10.3390/ma13030782
Garcia-Ben, J., Delgado-Ferreiro, I., Salgado-Beceiro, J., and Bermudez-Garcia, J. M. (2021). Simple and Low-Cost Footstep Energy-Recover Barocaloric Heating and Cooling Device. Materials 14, 5947. doi:10.3390/ma14205947
Greco, A., Aprea, C., Maiorino, A., and Masselli, C. (2019). A Review of the State of the Art of Solid-State Caloric Cooling Processes at Room-Temperature Before 2019. Int. J. Refrig. 106, 66–88. doi:10.1016/j.ijrefrig.2019.06.034
Gschwandl, M., Kerschbaumer, R. C., Schrittesser, B., Fuchs, P. F., Stieger, S., and Meinhart, L. (2019). Thermal Conductivity Measurement of Industrial Rubber Compounds Using Laser Flash Analysis: Applicability, Comparison and Evaluation Presented at the Materials Characterization Using X-Rays and Related Techniques. Malaysia: Kelantan, 030041. doi:10.1063/1.5088299
Heckele, M., and Schomburg, W. K. (2004). Review on Micro Molding of Thermoplastic Polymers. J. Micromech. Microeng. 14, R1–R14. doi:10.1088/0960-1317/14/3/R01
Holden, G. (1987). “Thermoplastic Elastomers,” in Rubber Technology (Boston, MA: Springer), 465–481. doi:10.1007/978-1-4615-7823-9_16
Janssen, S., Schwahn, D., Springer, T., and Mortensen, K. (1995). Coil and Melt Compressibility of Polymer Blends Studied by SANS and pVT Experiments. Macromolecules 28, 2555–2560. doi:10.1021/ma00111a059
Kashi, S., Varley, R., De Souza, M., Al-Assafi, S., Di Pietro, A., de Lavigne, C., et al. (2018). Mechanical, Thermal, and Morphological Behavior of Silicone Rubber During Accelerated Aging. Polymer-Plastics Technol. Eng. 57, 1687–1696. doi:10.1080/03602559.2017.1419487
Kıroğlu, C., and Kızılcan, N. (2021). Production and Characterization of Thermoplastic Elastomer Foams Based on the Styrene-Ethylene-Butylene-Styrene (SEBS) Rubber and Thermoplastic Material. Open Chem. 19, 929–937. doi:10.1515/chem-2021-0084
Kitanovski, A., Plaznik, U., Tomc, U., and Poredoš, A. (2015). Present and Future Caloric Refrigeration and Heat-Pump Technologies. Int. J. Refrig. 57, 288–298. doi:10.1016/j.ijrefrig.2015.06.008
Li, B., Kawakita, Y., Ohira-Kawamura, S., Sugahara, T., Wang, H., Wang, J., et al. (2019). Colossal Barocaloric Effects in Plastic Crystals. Nature 567, 506–510. doi:10.1038/s41586-019-1042-5
Li, F. B., Li, M., Xu, X., Yang, Z. C., Xu, H., Jia, C. K., et al. (2020). Understanding Colossal Barocaloric Effects in Plastic Crystals. Nat. Commun. 11, 4190. doi:10.1038/s41467-020-18043-1
Lloveras, P., Aznar, A., Barrio, M., Negrier, P., Popescu, C., Planes, A., et al. (2019). Colossal Barocaloric Effects Near Room Temperature in Plastic Crystals of Neopentylglycol. Nat. Commun. 10, 1803. doi:10.1038/s41467-019-09730-9
Lloveras, P., and Tamarit, J. L. (2021). Advances and Obstacles in Pressure-Driven Solid-State Cooling: A Review of Barocaloric Materials. MRS Energy & Sustain. 236, 2. doi:10.1557/s43581-020-00002-4
Maiorino, A., Del Duca, M. G., Tušek, J., Tomc, U., Kitanovski, A., and Aprea, C. (2019). Evaluating Magnetocaloric Effect in Magnetocaloric Materials: A Novel Approach Based on Indirect Measurements Using Artificial Neural Networks. Energies 12, 1871. doi:10.3390/en12101871
Mañosa, L., González-Alonso, D., Planes, A., Bonnot, E., Barrio, M., Tamarit, J.-L., et al. (2010). Giant Solid-State Barocaloric Effect in the Ni--Mn--In magnetic shape-memory alloy. Nat. Mater. 9, 4.
Miliante, C. M., Christmann, A. M., Usuda, E. O., Imamura, W., Paixão, L. S., Carvalho, A. M. G., et al. (2020). Unveiling the Origin of the Giant Barocaloric Effect in Natural Rubber. Macromolecules 53, 2606–2615. doi:10.1021/acs.macromol.0c00051
Min, J., Sagotra, A. K., and Cazorla, C. (2020). Large Barocaloric Effects in Thermoelectric Superionic Materials. Phys. Rev. Mater. 4, 015403. doi:10.1103/PhysRevMaterials.4.015403
Mirizzi, L., Carnevale, M., D’Arienzo, M., Milanese, C., Di Credico, B., Mostoni, S., et al. (2021). Tailoring the Thermal Conductivity of Rubber Nanocomposites by Inorganic Systems: Opportunities and Challenges for Their Application in Tires Formulation. Molecules 26, 3555. doi:10.3390/molecules26123555
Moya, X., and Mathur, N. D. (2020). Caloric Materials for Cooling and Heating. Science 370, 797–803. doi:10.1126/science.abb0973
Patel, S., Chauhan, A., Vaish, R., and Thomas, P. (2016). Elastocaloric and Barocaloric Effects in Polyvinylidene Di-Fluoride-Based Polymers. Appl. Phys. Lett. 108, 072903. doi:10.1063/1.4942000
Pottiger, M. T., Coburn, J. C., and Edman, J. R. (1994). The Effect of Orientation on Thermal Expansion Behavior in Polyimide Films. J. Polym. Sci. B Polym. Phys. 32, 825–837. doi:10.1002/polb.1994.090320506
Qian, S., Geng, Y., Wang, Y., Ling, J., Hwang, Y., Radermacher, R., et al. (2016). A Review of Elastocaloric Cooling: Materials, Cycles and System Integrations. Int. J. Refrig. 64, 1–19. doi:10.1016/j.ijrefrig.2015.12.001
Raasch, J., Ivey, M., Aldrich, D., Nobes, D. S., and Ayranci, C. (2015). Characterization of Polyurethane Shape Memory Polymer Processed by Material Extrusion Additive Manufacturing. Addit. Manuf. 8, 132–141. doi:10.1016/j.addma.2015.09.004
Roth, B., Wildner, W., and Drummer, D. (2020). Dynamic Compression Induced Solidification. Polymers 12, 488. doi:10.3390/polym12020488
Schmidt, M., Schütze, A., and Seelecke, S. (2016). Elastocaloric Cooling Processes: The Influence of Material Strain and Strain Rate on Efficiency and Temperature Span. Apl. Mater. 4, 064107. doi:10.1063/1.4953433
US Energy Information Administration (2021). EIA Independent Statistics and Analysis. Washington, DC: Total Energy.
Usuda, E. O., Imamura, W., Bom, N. M., Paixão, L. S., and Carvalho, A. M. G. (2019). Giant Reversible Barocaloric Effects in Nitrile Butadiene Rubber Around Room Temperature. ACS Appl. Polym. Mat. 1, 1991–1997. doi:10.1021/acsapm.9b00235
Wang, J., Hopmann, C., Schmitz, M., Hohlweck, T., and Wipperfürth, J. (2019). Modeling of pvT Behavior of Semi-Crystalline Polymer Based on the Two-Domain Tait Equation of State for Injection Molding. Mater. Des. 183, 108149. doi:10.1016/j.matdes.2019.108149
Wang, K., and Deng, Q. (2019). The Thermal and Mechanical Properties of Poly(Ethylene-co-Vinyl Acetate) Random Copolymers (PEVA) and Its Covalently Crosslinked Analogues (cPEVA). Polymers 11, 1055. doi:10.3390/polym11061055
Keywords: thermoplastic elastomers, solid-state refrigeration, barocaloric effect, material characterization, rheology
Citation: Weerasekera N, Ajjarapu KPK, Sudan K, Sumanasekera G, Kate K and Bhatia B (2022) Barocaloric Properties of Thermoplastic Elastomers. Front. Energy Res. 10:887006. doi: 10.3389/fenrg.2022.887006
Received: 01 March 2022; Accepted: 13 April 2022;
Published: 30 May 2022.
Edited by:
Lin Zhao, 3M, United StatesReviewed by:
Angelo Maiorino, University of Salerno, ItalyJean Rodrigo Bocca, State University of Maringá, Brazil
Copyright © 2022 Weerasekera, Ajjarapu, Sudan, Sumanasekera, Kate and Bhatia. This is an open-access article distributed under the terms of the Creative Commons Attribution License (CC BY). The use, distribution or reproduction in other forums is permitted, provided the original author(s) and the copyright owner(s) are credited and that the original publication in this journal is cited, in accordance with accepted academic practice. No use, distribution or reproduction is permitted which does not comply with these terms.
*Correspondence: Bikram Bhatia, YmlrcmFtLmJoYXRpYUBsb3Vpc3ZpbGxlLmVkdQ==