- 1Environmental Biotechnology and Renewable Energies Group, Centro de Investigación y de Estudios Avanzados del IPN, Transdisciplinary Program of Science and Technology for Society, CONACYT’s Chair Young Researcher, Ciudad de México, Mexico
- 2Environmental Biotechnology and Renewable Energies Group, Centro de Investigación y de Estudios Avanzados del IPN, Ciudad de México, Mexico
- 3Department of Energy, Universidad Autónoma Metropolitana-Azcapotzalco, Ciudad de México, Mexico
Waste diapers (WD) handling and disposal in Mexico are typically based on their burial in dumping sites and landfills. Practically reclaiming and recycling of WD are non-existent. The clean diapers are composed of cellulose fibres (37–43% db), hemicellulose (5–9%), lignin (4–7%), protein (<1), plastics (polypropylene and polyethylene) (12–16%), absorbent sodium polyacrylate (14–18%), and elastic and adhesives tapes (9–12%). The latter can be valuable resources. WD composition is similar to clean diaper, although humidity is very high, and the ranges of faeces and urine are 1.5–2.5 and 6–9% dry weight, respectively. International literature searches indicate that there is some research on composting, fungal biodegradation, and methanogenic co-digestion of waste activated sludge with the organic fraction of waste diapers (OFWD.) However, research on dark fermentation of OFWD is limited. In this work, the generation of biohydrogen from dark fermentation of OFWD was optimised. We used the response surface methodology (RSM). Independent variables were the temperature of operation (37–55°C), ratio C/N of the feed (30, 40 gC/gN), and initial total solids of the feed (TSi) (15, 25%). The dependent (response) variables examined were Y’H2 (H2 produced per initial g of dry matter), contents of low molecular weight organic solvents and acids, lactic acid, the ratio A/B (acetic-to-butyric acid), and the quotient organic acids C2 to C4-to-solvents. The predicted maximum Y’H2 occurred at the combination of factors of 43 gC/gN, 12% and 31°C; its value was 2.79 mmolH2/gTS; its experimental validation gave 2.48 mmolH2/gTS, which shows a good agreement between values (11% lower than the predicted value). The maximum of Y’H2 with OFWD compared very favourably with bioH2 values obtained from a wide variety of wastes (organic municipal residues, agricultural wastes, etc.) using the same batch type fermentation with intermittent venting. Interestingly, the predicted temperature optimum fell in the lower side of the mesophilic range. Process heating savings would be in the order of 60.0 and 27.2% for thermophilic and mesophilic operation, respectively. In this way, it would be a contribution to the sustainability of the dark fermentation of OFWD. This result was somewhat counterintuitive and strongly indicates the usefulness of the response surface methodolog for analyzing the experimental results and uncovering favourable, although unexpected conditions.
Introduction
Hydrogen obtained from biomass or lignocellulosic waste is one of the promising biofuels used for energy generation and produces very few polluting emissions to the environment. Hydrogen is used in different annealing industries as a clean and environmentally friendly fuel because its main combustion product is water (Kotay and Das, 2008). It is also known that hydrogen production is preferred over methane because of its wide variety of applications (Kothari et al., 2012).
One of the characteristics that make biohydrogen production less favorable is the low yield obtained and process’s costs (Kumar et al., 2019). The drawbacks can be counteracted; some of the alternatives to achieve it is to improve yields by controlling critical process parameters (pH, temperature, nutrients, substrate type) as well as the valorization of by-products [volatile organic acids (VOA, i.e., members of the short-chain fatty acids family such as acetic acid, HAc, propionic acid, HPr, butyric acid, HBu), alcohols, lactic acid, non-hydrolyzed residues, among others] by some other biological processes, as well as the application of those by-products as precursors in chemical production processes (Ghimire et al., 2015).
Some authors have tried to take advantage of effluents from dark fermentation because they are rich in volatile organic acids converted into new valuable compounds. Kumar et al. (2019) addressed the issue of biopolymer production through the use of volatile organic acids (VOA) effluents from dark fermentation, finding that the whole process is economically viable because a residual effluent from the DF process can be exploited, together with the fact that these biopolymers can be an alternative to the use of polymers obtained by petrochemicals, becoming polymers easy to degrade employing microorganisms.
Among other alternatives that have been proposed for further processing and reclaiming of fermented solids from DF, is the subsequent anaerobic digestion with CH4 generation (Escamilla- Alvarado et al., 2012; Lee et al., 2010; Ueno et al., 2007). This approach, also known as H-M, allows for a higher gross generation of bioenergy due to the contribution of the methanogenesis. In some cases, the CH4 production from coupled H-M processes can be higher than that of single-stage anaerobic digestion, since the solids entering the methanogenic stage are already partially hydrolase or digested.
In Mexico, 37.5 million tons of waste are generated per year. Used disposable diapers represent nearly 7% (SEMARNAT, 2012) of this stream. Recent works indicate that most parents in Mexico use disposable diapers because they are perceived as hygienic and economic (PROFECO, 2003; PROFECO, 2011; Kim and Cho, 2017). It has been determined that up to six diapers/(child*day) are used in Mexico. Afterwards, waste diapers are disposed of in dumping sites and landfills (Espinosa-Valdemar et al., 2015).
The clean diapers are basically composed (on dry basis) of cellulose fibres (37–43% db), hemicellulose (5–9%), lignin (4–7%), protein (<1), plastics (polypropylene and polyethylene) (12–16%), an absorbent material made of sodium polyacrylate (SPA) (14–18), and elastic and adhesives tapes (9–12%) that can be valuable resources (Villagran, 2014). Typical weights of clean disposable diaper and a waste diaper are 41 and 212 g, respectively (EDANA, 2008). Component ranges of WD were similar to those of the clean diaper, with the difference that the humidity is very high, and the ranges of faeces and urine were 1.5–2.5 and 6–9%, respectively.
Applied research has made wide use of the response surface methodology or RSM. The RSM is a statistical optimization technique that is very useful in predictive analysis and modeling when a dependent (or several dependent variables) are influenced by independent factors of interest (Myers et al., 2009; Ntaikou et al., 2010).
According to literature, essential factors (independent variables) in DF of wastes can include total solids, pH, temperature, alkalinity, microorganisms, types of feedstocks, types of reactors, nutrient, among others (Valdez-Vázquez et al., 2006; Valdez-Vázquez and Poggi-Varaldo, 2009; Ntaikou et al., 2010; Amorim et al., 2012; Cavinato et al., 2013; Robledo-Narvaez et al., 2013; Sotelo-Navarro et al., 2017; García-Depraect et al., 2019). Several of those variables could be of interest in the dark fermentation of WD.
It is worth highlighting why waste diapers (WD) are attractive for biohydrogen production. Among the merits and benefits of this use of WD we can cite the following:
(i) the WD have a high content of cellulose (40% db); earlier research has demonstrated that substrates and wastes rich in cellulose and hemicellulose or carbohydrates can produce an attractive amount of biological hydrogen (Rodríguez-Valderrama et al., 2020; Alvarez et al., 2020; Sarangi and Nanda, 2020; Solowski et al., 2020; Weide et al., 2019; Catalán and Sánchez, 2019; Yeshanew et al., 2018; Roy 2017; Robledo-Narváez et al., 2013). Thus, in principle, the potential of biohydrogen production of WD, could be very high.
(ii) since WD are wastes, their cost as raw material is essentially free or low; their cost would be mainly associated to transport, conditioning, and preparation (Torrijos et al., 2014; Escamilla-Alvarado et al., 2017).
(iii) the fermented OFWD, and solids and liquids from conditioning could be reclaimed by further processing to obtain more energy and bioproducts. (Poggi-Varaldo et al., 2014).
(iv) also, the well-known advantages of hydrogen as a clean biofuel or commodity are merits that justify the DF of OFWD (Kotay and Das, 2008; Kothari et al., 2012).
Former research has evaluated the feasibility of treating disposable diapers by biological methods. Degradation with fungi, composting, and methanogenic digestion were the tested methods (Colón et al., 2011; Colón et al., 2013; Torrijos et al., 2013; Espinosa-Valdemar et al., 2014; Espinosa-Valdemar et al., 2015).
More recently, the feasibility of producing biohydrogen from disposable diapers by dark fermentation was evaluated by our Group (Sotelo-Navarro et al., 2017). Main results of this investigation allowed to establish that it was feasible to use disposable waste diapers as a substrate. As a first approach, different ways of conditioning the substrate and operating temperature in mesophilic and thermophilic were tested; however, the initial total solids and the carbon-nitrogen ratio were set at fixed values (20 and 30%, respectively). The yields obtained were low compared with those obtained in the DF of the OFMSW (organic fraction of municipal solid waste).
If successful, DF of the OFWD would hold promise for integration to biorefineries from wastes that could co-ferment this waste with a variety of agricultural and agroindustrial wastes of similar characteristics (cellulosic and lignocellulosic wastes, i.e., food and textile wastes) (Rodríguez-Valderrama et al., 2020; Sarangi and Nanda, 2020; Solowski et al., 2020; Weide et al., 2019; Catalán and Sánchez, 2019; Yeshanew et al., 2018; Roy 2017; Robledo-Narváez et al., 2013). As it was hinted above, biological hydrogen production can be easily incorporated to several biorefinery platforms as a first stage of biofuel generation, followed by a network of processes devoted to obtaining value-added products and possibly more energy (Poggi-Varaldo et al., 2014). The biorefinery approach for treating and reclaiming WD and other residues could be more attractive if an inverse cascading approach that maximizes energy generation from wastes were adopted. In this scheme, first energy (biofuels such as biological hydrogen and methane are produced from waste. Afterwards, the fermented waste is subject to value-added product generation, and finally, more bioenergy is produced from the remainder of residues of previous processes (Poggi-Varaldo et al., 2014; Escamilla-Alvarado et al., 2017). This approach could be an attractive model for waste biorefineries in developing countries that are net importers of fossil fuels.
Therefore, the objective of this work was to optimise the production of biohydrogen from disposable waste diapers using the statistical technique known as the response surface analysis. The effects of factors C/N ratio (30, 40 gC/gN), initial total solids (15, 25%), and temperature (35, 55°C) on biohydrogen generation were evaluated. To the best of our knowledge, there is no previous report of this type of study with WD. The design was established using Statgraphics v.19, based on a factorial experiment 23 with central and axial points. At this time, only the process evaluation was carried out; the economic feasibility analysis was out of the scope of this research.
Materials and Methods
Experimental Design
The optimization was performed using surface response analysis based on a factorial 23 with axial and central (Supplementary Table S1) (Myers et al., 2009; Montgomery, 2012). The factors were the ratio C/N in the feed (30, 40 g/g), initial total solids (TSi) (15, 25%) in the feedstock as well as process temperature (37, 55°C). The response variables were the biological production of H2 (Y’H2, in mmol H2/g TS substrate), organic acids concentrations, lactic acid, contents of low molecular weight solvents, the ratio of acetic-to-butyric acid (defined as A/B on COD basis; details on significance and calculations of the ratio A/B in Supplementary Material), and the ratio organic acids-to-solvents. The carbon-nitrogen ratio for the treatments and experimental units was adjusted in the range 27–43% (Supplementary Table S7). Given the Nitrogen and organic matter contents of the OFWD and the inoculum, the C/N targets of the RSM experimental design, mass balances were performed to estimate the additional nitrogen amount to each experimental unit. NH4Cl was selected as the N source. The required amount of N was supplemented as ammonium chloride to each experimental unit. Supplementary Table S6 shows that the OFWD exhibited a high contents of holocellulose (cellulose plus hemicellulose) and low concentration of total Kjeldahl nitrogen (TKN). The content of nitrogen was lower than that in the WD, presumably due to conditioning operations to remove plastics and moisture absorbent from the WD (Supplementary Figure S1) that likely washed-out the organic and ammonia nitrogen contained in the faeces and urine. As a result, the C/N ratio of the OFWD was very high (approximately 104.67 ± 1.05 gC/gN), well beyond the range recommended for anaerobic biological waste treatment (25–40 gC/gN, Wang et al., 2014; Poggi-Varaldo et al., 1997).
Therefore, the OFWD was supplemented with NH4Cl as explained below to set the ratio of C/N in a more adequte range 27–43%.
The increase of the content of holocellulose in the OFWD in % dry weight compared to that in both clean diapers and WD is due to the elimination of the weights of plastics and the sodium polyacrylate polymer SPA as well as faeces and urine from the latter, that account for nearly half of the dry weight of the WD. On absolute basis, the content of holocellulose of the OFWD is practically conserved after the conditioning operations of the WD (except for some marginal losses of small size fibres that cannot be recovered).
Reactors were loaded in such a way to comply with the target values of the factors indicated by the response surface design (Supplementary Table S2). The carbon-to-nitrogen ratio was adjusted using ammonium chloride. The experiment was run in a single block and with a completely randomized order to prevent bias and meet the randomness/independence requirements of the analysis of variance (Montgomery, 2012). Two replicates of each treatment were run. Background controls with the only inoculum were also tested.
Experiments were performed using the procedure known as solid substrate anaerobic H2 fermentation, with intermittent venting and headspace flushing (SSAHF-IV) reported by Sotelo-Navarro et al. (2017) and Robledo-Narvaez et al. (2013) (Supplementary Material). The reactors were serum bottles 120 ml capacity.
Feedstock and Inoculum
In this research, the organic fraction of waste diaper (OFWD, mainly cellulose) was used, following the protocol described by Girotto et al. (2015). The OFWD was prepared as follows (Supplementary Figure S1): first, plastics and elastic materials were separated manually, leaving the core, i.e., SPA, holocellulose, faeces, and urine. Afterward, water was added. CaCl2 was incorporated to reach a target concentration of 3,000 mg L-1 calcium ion in the liquor to agglomerate and separate the SPA. The mixed liquor was sedimented for 24 h at room temperature. Two phases were formed, the liquid supernatant and the wet solids. The latter, in turn, consisted of 2 cm diameter balls of SPA-Ca and wet OFWD. The supernatant was withdrawn by decanting. The SAP-Ca balls were picked-up out from the wet solids phase. The remainder of the wet solids, the OFWD, was filtered and further dried at 70°C for 24 h (Supplementary Figure S1) (More details on the separation of the OFD can be consulted in Supplementary Material). The full characterization of the used waste diapers is presented in Supplementary Table S5 (Supplementary Material).
The ratio of substrate-to-inoculum (OFWD-to-inoculum) was 10% w/w. The inoculum was subjected to thermal shock at 92°C for 30 min to eliminate methanogenic archaea and other H2-consuming microbes from the consortium, as reported elsewhere (Muñoz-Paéz et al., 2013; Sotelo-Navarro et al., 2017) (more details in Supplementary Material).
Analyses
The hydrogen concentration in biogas was monitored as the main parameter, although CH4 and CO2 contents were also analysed. Biogas analysis was carried out 3 times/week for 30 days using a Gow-Mac Model 350 gas chromatograph, as reported by Muñoz-Páez et al. (2013) and Robledo-Narvaez et al. (2013). H2, CH4, and CO2 contents in the headspace of the bioreactor were routinely determined. The mass of each gas (in mmol) in the calibration was calculated by using the ideal (perfect) gas law (Castellan, 1983), departing from gas volumes at room pressure and temperature.
The contents of volatile organic acids (VOA, also known as volatile fatty acids or short-chain fatty acids C2 to C4, acetic, HAc, propionic HPr, and butyric acid, HBu), lactic acid, and low molecular weight solvents (acetone, methanol, ethanol, and butanol) were analysed at the beginning and at the end of the process (Valdez-Vazquez and Poggi-Varaldo, 2009; Muñoz-Páez et al., 2014) (Supplementary Material).
The pH was analysed in a suspension that consisted of one portion of the fermented sample and five portions of cooled deionized water, at a temperature of 5°C (Poggi-Varaldo and Oleszkiewicz, 1992). Alkalinities, both intermediate and total, as well as the coefficient α’ (a surrogate indicator of possible VOA accumulation in digesters, based on alkalinity values), was determined according to the procedures described by Poggi-Varaldo and Oleszkiewicz (1992) and Ripley et al. (1981).
The physicochemical characteristics of the feedstock, inoculum, and fermented solids analysed were organic matter as COD, VS, TS, ash (Clesceri et al., 1998), nitrogen (Andrew et al., 2005), cellulose (Van Soest and Wine, 1967), hemicellulose, and lignin (Technical Association of Pulp and Paper Industry, 2001). The values were reported in Supplementary Tables S6, S7 , ‘Composition of organic fraction of waste diapers (OFWD)’ and ‘Adjustment of Carbon-Nitrogen ratio’, respectively, and ‘Supplementary Material Inoculum and seed reactors’ in the SM.
Calculations
The variable ρ (Eq. 1) was the quotient of the summation of the concentrations of organic acids (COD basis) in the numerator and the summation of the solvents contents (COD basis) in the denominator. Thus, ρ can indicate whether metabolism is predominantly acidogenic or solventogenic.
where: ∑VOA = summation of the contents of VOA (C2 to C4, COD basis). ∑SOLV = summation of solvent contents (methanol, ethanol, butanol, acetone; COD basis).
The concentration of lactic acid was informed alone; it was not considered for estimating of the numerator of ρ. The reason for this was related to the fact that lactic acid acts as a hydrogen sink. For instance, the homolactic fermentation of hexoses leads to the generation of 2 moles of lactic acid. No biohydrogen is generated (Escamilla-Alvarado et al., 2012).
Statistical Processing
Results were processed with the statistical routines of Stathgraphics V.19 (Statgraphics Technologies, Inc.; The Plains, VA, United States). In calibration work, regression lines were fitted to calibration data using Excel®/Data/Data analysis/Regression.
Results and Discussion
Biohydrogen Production
Cumulative biohydrogen production (Y’H2), sum of VOA, and the sum of solvents for all the treatments in our experiment are presented in Supplementary Table S2 of the SM. Up to four cycles of fermentation with intermittent venting with N2 were achieved (Figure 1). Typically, the main contribution to total biohydrogen production was associated with the first two cycles of incubation.
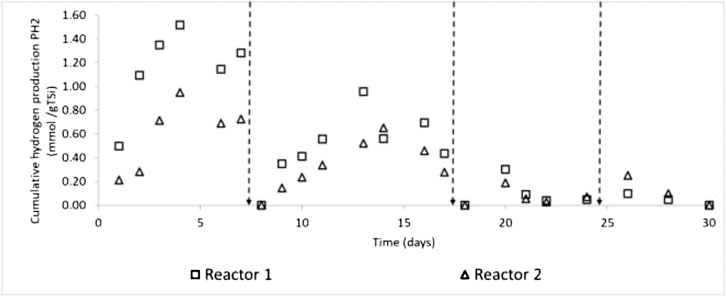
FIGURE 1. Typical time courses of biohydrogen production of diapers of two treatments in the experiment, treatments. Keys: Vertical arrows indicate flushing of bioreactor headspace with N2
Equation. 2 represents a surface represented by a second-order model that relates the response variable Y’H2 to the independent variables (factors) C/N, T, and TSi (actual values).
Figure 2 shows the response surface contour plots of Y’H2 for each pair of factors for the cumulative production of hydrogen (frames a to c). An inspection of the frame (a) in Figure 2 suggests that maximum Y’H2 occurred at low TSi and high C/N. Frame (b) indicates that maximum Y’H2 was in the region of low mesophilic temperatures and high values of C/N. Finally, the inspection of frame (c) displays the maximum value in the region of low mesophilic temperatures and low TSi of the feedstock. Overall, this preliminary analysis suggests that the max Y’H2 would fall in a region of low TSi, low temperature, and high C/N. This qualitative prediction was consistent with the mathematical value for max Y’H2 reported below.
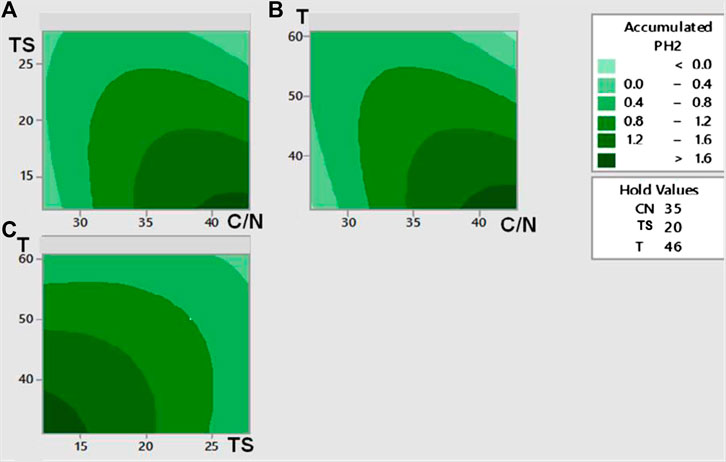
FIGURE 2. Contour plots of the response surface for the influence of different pairs of variables on the H2 production (mmolH2/gTSi) from the dark fermentation of the organic fraction of waste diapers. Cumulative H2 production (A) as a function of initial Total Solids and C/N, Temperature set a 46°C; (B) as a function of Temperature and C/N, Initial Total solids set at 20%; (C) as a function of Temperature and initial Total Solids, C/N ratio set at 35.
The maximum optimum value predicted by the response surface for Y’H2 was 2.79 mmol/gTSi; it occurred at the combination of factors of 43 gC/gN, 12% TSi, and 31°C (Table 1). This result was validated by running the fermentation in triplicate at that point, which yielded 2.48 mmol/gTSi, lower than the predicted value by only 11.32%.
Table 1 also shows a summary of the predicted value features for the maximum Y’H2, as well as other related statistical information (confidence interval of the prediction, etc.). The confidence interval of 95% of the predicted value contained the experimental Y’H2.
The analysis of variance for Y'H2 is displayed in Supplementary Table S3. The quadratic model adequately fitted the experimental data, as evidenced by a p-value >0.05 for the Lack-of-fit. The R2 statistic indicated that the adjusted model explained up to 66.3% of the cumulative variability of Y’H2. Regarding the source of variation, the main effects of the three factors were significant (p < 0.05), as well as the pure quadratic term in C/N and the two interactions where C/N participated. These results were consistent with the significance of the coefficients of the quadratic function (Supplementary Table S3). Therefore, the modified or simplified quadratic equation for the Surface of Y’H2 would retain only six terms (Eq. 3), instead of 10 terms in the complete Eq. 2 (Supplementary Table S3).
In general, the standardized residuals (the standardized difference between predicted and experimental values of Y’H2) fell in the interval (˗2, 2). According to statistical procedures, only the outliers associated with standardized residuals larger than two or lower than ˗2 are reported. So, Supplementary Table S3 should be interpreted in the sense that no outliers occurred (except for the result of the experimental unit 28, Supplementary Tables S1; Montgomery, 2012).
Prediction of Concentrations of Selected Organic Acids
Maximum of the Variable Sum of Volatile Organic Acids
The surface of the response variable ∑VOA (excluding HLac) was given by Eq. 4 in terms of actual values of factors C/N, T, and TSi.
Figure 3 exhibits the contour plots of ∑VOA for each pair of factors. An intuitive examination of Figure 3A indicates that the highest ∑VOA occurred on a diagonal ridge, and several pairs of coordinates of (C/N, TSi) gave high values of the response variable. According to Figure 3B, the highest ∑VOA was associated with a low range of temperature and high values of C/N. Last, Figure 3C suggests the highest ∑VOA fell at low temperature and moderate-to-high TSi.
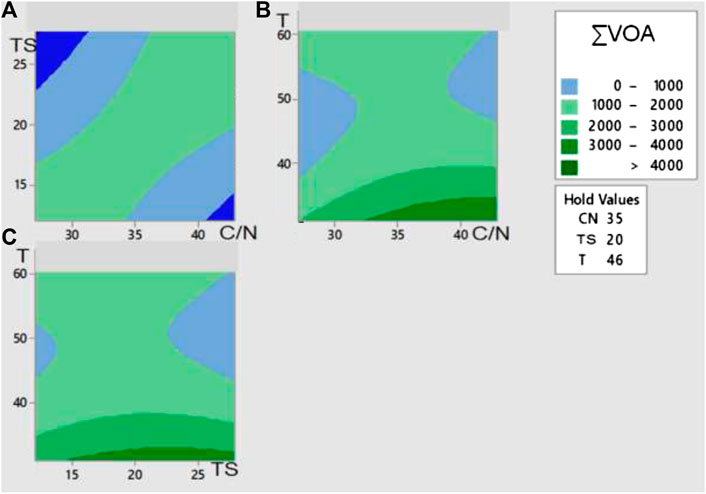
FIGURE 3. Contour plots of the response surface for the influence of different pairs of variables on the sum of volatile acids (mg COD/kgdb) from the dark fermentation of the organic fraction of waste diapers. Total volatile organic acids concentration (A) as a function of initial Total Solids and C/N, Temperature set a 46°C; (B) as a function of Temperature and C/N, Initial Total solids set at 20%; (C) as a function of Temperature and initial Total Solids, C/N ratio set at 35.
Our initial analysis suggests that the highest ∑VOA would be observed at high C/N, moderate-to-high TSi, and low temperature. As shown below, this preliminary prediction was congruent with the maximum value of ∑VOA extracted by mathematical methods of the software.
The maximum (optimum) value of ∑VOA predicted by the response surface was 5,213 mg COD/gwb, whereas the coordinates of such a maximum were 43 gC/gN, 28% TSi, and 31°C (Table 2). The 95% CI of the predicted value contained the maximum experimental ∑VOA. However, the difference between predicted and experimental (actual) maxima ∑VOA seemed to be significant. Interestingly, the values of the coordinates of the first and third factors above were consistent with the corresponding coordinates found for the maximum Y’H2 (Table 1).
Minimum of the Variable Sum of Solvents
The response surface for the variable response sum of solvents (ethanol, acetone, butanol, and methanol) corresponds to Eq. 5 below.
The minimum sum of solvents was found at C/N ratio of 27, TSi of 15, and a temperature of 31°C (Supplementary Table S5). This result, along with the low range of ΣSOLV in the experiment, is significant because a high value of ΣSOLV could be associated with a solventogenic shift of fermentation. In turn, the solventogenic deviation would lead to low bioH2 production (Lee et al., 2010). Therefore, it is a set of results that were determined independently and are consistent among them.
Two of the coordinates for the minimum predicted sum of solvents (Supplementary Table S5) were very close to the corresponding coordinates for the maximum predicted Y’H2 (Table 1), namely initial total solids and temperature.
Figure 4 shows the surface response graphs for each pair of factors for the sum of solvents (acetone, butanol, methanol, and ethanol).
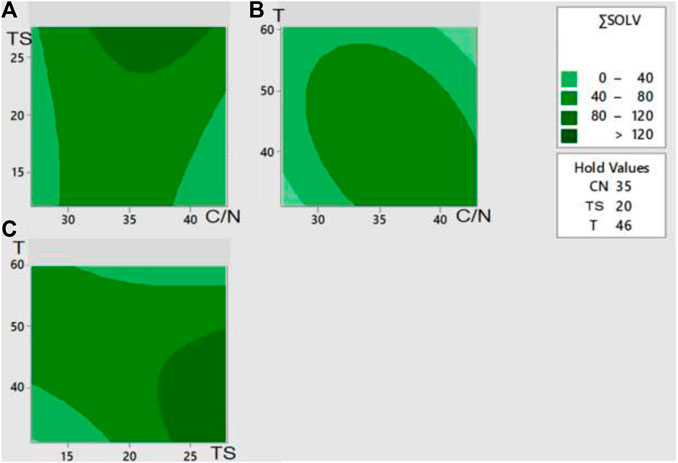
FIGURE 4. Contour plots of the response surface for the influence of different pairs of variables on the sum of solvents (mg COD/kgdb) from the dark fermentation of the organic fraction of waste diapers. Total concentration of low molecular weight solvents: (A) as a function of initial Total Solids and C/N, Temperature set a 46°C; (B) as a function of Temperature and C/N, Initial Total solids set at 20%; (C) as a function of Temperature and initial Total Solids, C/N ratio set at 35.
Combined Desirability (Combined Optimisation) of Both Biohydrogen and Volatile Organic Acids Productions
We explored the composite desirability of the variables Y’H2 and ∑VOA, looking for simultaneous maxima. The rationale behind this was to locate a combination of attractive biohydrogen production and high VOA contents in the fermented solids. The first feature is self-explanatory. The second was related to a favourable profile of organic acids in the fermented solids in case that either an anaerobic digestion post-treatment of the fermented solids or recovery/purification of VOA were chosen.
According to Sotelo-Navarro et al. (2020), organic acids (as well as low molecular weight solvents) produced by fermentation of organic residues have attractive applications and market: either they can be used in chemical synthesis as building blocks in such a way to replace those of fossil origin, or used bielectricity and biofuels production, or photoheterotrophic bioH2 (Silva et al., 2014; Ghimire et al., 2015; Venkata Mohan et al., 2016). Examples of organic acids of interest include both value-added and commodities compounds, i.e., glutamic acid, citric, 3-hydroxy propionic acid, levulinic, pyruvic, malic, succinic acid, fumaric, aspartic acid, 2,5-furan dicarboxylic acid, lactic acid, itaconic, glucaric acid, and C1 to C7 volatile fatty acids (Werpy and Petersen, 2004; Brown, 2007; Murali et al., 2017).
Previous studies (Werpy and Petersen, 2004; Brown, 2007; Silva et al., 2014; Ghimire et al., 2015; Murali et al., 2017; Venkata Mohan et al., 2019) showed that organic acids constitute a valuable platform for a great variety of lucrative applications, i.e., biorefineries based on organic acids platforms, “bio(petro)chemistry,” biofuels, pharmaceuticals, and more.
In this way, DF of OFWD could be envisioned as a two-product process: on the one hand, the H2 is recognized as a clean energy carrier with the highest enthalpy of combustion per unit of mass among the more conventional fuels (Mizuno et al., 2002; Kapdan and Kargi, 2006). On the other hand, organic acids, and selected solvents (Ebrahimian and Karimi, 2020) can be concomitantly produced in DF. As our discussion above attempted to show, these compounds are of great technical and economic interest. Therefore, it seems that a desirable performance of dark fermentation would be related to determining conditions where both response variables (bioH2 production and organic acids production) could be maximized.
The effect of temperature on DF bioH2 production in the open literature is still not conclusive. On the one hand, Escamilla-Alvarado et al. (2012), Puhakka et al. (2012), Lee et al. (2008); Valdez-Vázquez et al. (2006) have found that highest hydrogen yields, or concentrations corresponded to the mesophilic regime in previous studies. Yet, a few works have observed high H2 productions at temperatures around 20–28°C (Muñoz-Páez et al., 2013; Alvarez-Guzmán et al., 2020). In contrast, Sotelo et al. (2017), Escamilla-Alvarado et al. (2013), Kim and Kim (2012), Kargi et al. (2012), Cakir et al. (2010), and Akutsu et al. (2008) have reported the highest biohydrogen yields in the thermophilic regime. Interestingly, a list of papers has dealt with high biohydrogen yields at temperatures beyond the thermophilic range, which can be considered hyperthermophilic (Yokoyama et al., 2009).
However, it seems that the preferred outcome would be high biohydrogen productions at lower temperatures because significant savings on heating the feedstock as well as heating the bioreactors and ancillary equipment for compensating the heat losses to the surroundings could be achieved. Any improvement of H2 production at higher temperature should be analysed in terms of energy balances to check whether the energy increase associated with increased H2 is higher than the additional energy requirements for feedstock and equipment heating to reach a higher temperature of operation, or not.
Likely, the temperature per se is not the only significant factor in biohydrogen yield from DF. The nature of the substrate, osmotic pressure (the content of TS), the balance of nutrients, pH control or lack of control, could influence the role of temperature in what is known to be “interaction” (in the statistical sense) that is very common in multifactorial experiments with complex systems (Montgomery, 2012). For instance, previous work with a similar substrate (Sotelo et al., 2017) demonstrated that the highest yield of hydrogen occurred in the thermophilic regime; however, in that case, different and fixed values of C/N and TSi were considered, so it would be expected that the current work will provide new and insightful information on the effects of these factors on biohydrogen generation.
According to Table 3, the treatment that gave high organic acids and a still attractive Y’H2 occurred at 43 gC/gN, 19% TSi, and 31°C, with a predicted Y’H2 of 1.96 mmol/gTSi and 3,767 mg COD-VOA/kgwb. The factor ∑SOLV was not considered for the composite desirability because all treatments in this work exhibited relatively low values (9–239 mg COD-SOLV/kgwb) that in turn were associated with high values of ρ (mean value of 28), pointing out to the absence of solventogenic shift in our experimental units (Lee et al., 2010; Escamilla-Alvarado et al., 2013; Muñoz-Páez et al., 2014).
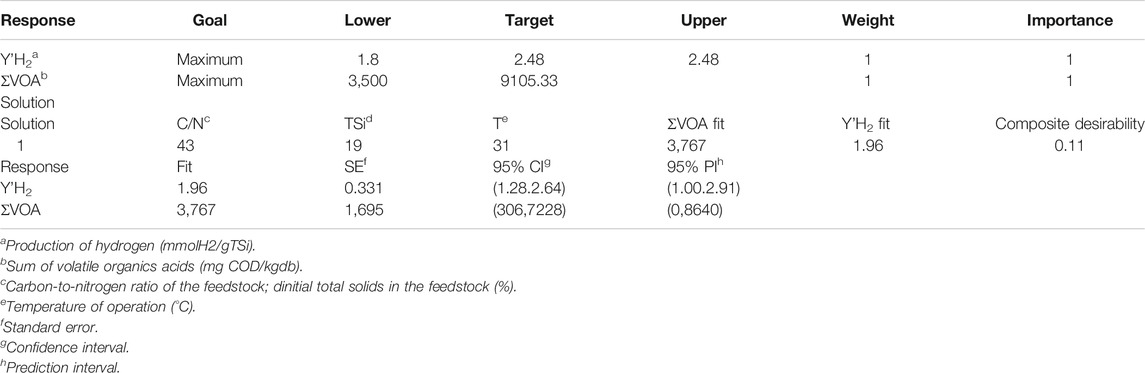
TABLE 3. Optimization by combined desirability studies on simultaneous cumulative biohydrogen production and the sum of volatile organic acids.
To the best of our knowledge, there are no works in the open literature dealing with the evaluation of the effects of the independent variables (factors, i.e., TSi of the feed, the temperature of operation, and ratio C/N of the feed) tested in our experiment on the biohydrogen production from OFWD. Moreover, we could not find results on the combined optimization (combined desirability) of both biohydrogen production and the VOA production represented by the response variable ΣVOA, in dark fermentation of OFWD. Recently, the interest in VOA production using DF has increased as we have pointed out above, as well as the debate between more H2 or more VOA from DF. More details on this discussion can be found in Supplementary Material.
Table 4 summarises the research results focused on the statistical optimisation of production and some other variables (i.e., HLac accumulation, ΣVOA). These works explored a great variety of substrates, from glucose and sucrose (Lin and Lay, 2004; Chen et al., 2011; Akhbaria et al., 2019; Yin and Wang, 2019; Alvarez-Guzmán et al., 2020) to solid or semisolid organic wastes (Robledo-Narváez et al., 2013; Muñoz-Páez et al., 2014; Astie et al., 2018; Ghimire et al., 2018; López-Hidalgo et al., 2018), passing by fermentation effluents (Astie et al., 2018). Factors typically tested were temperature (in 8 works), either initial total solids (in 3 works) or initial substrate concentration (in 5 works), pH (in 6 works), ratio C/N (in 2 works), and concentration of yeast extract (as a supplement, only in 1 work).
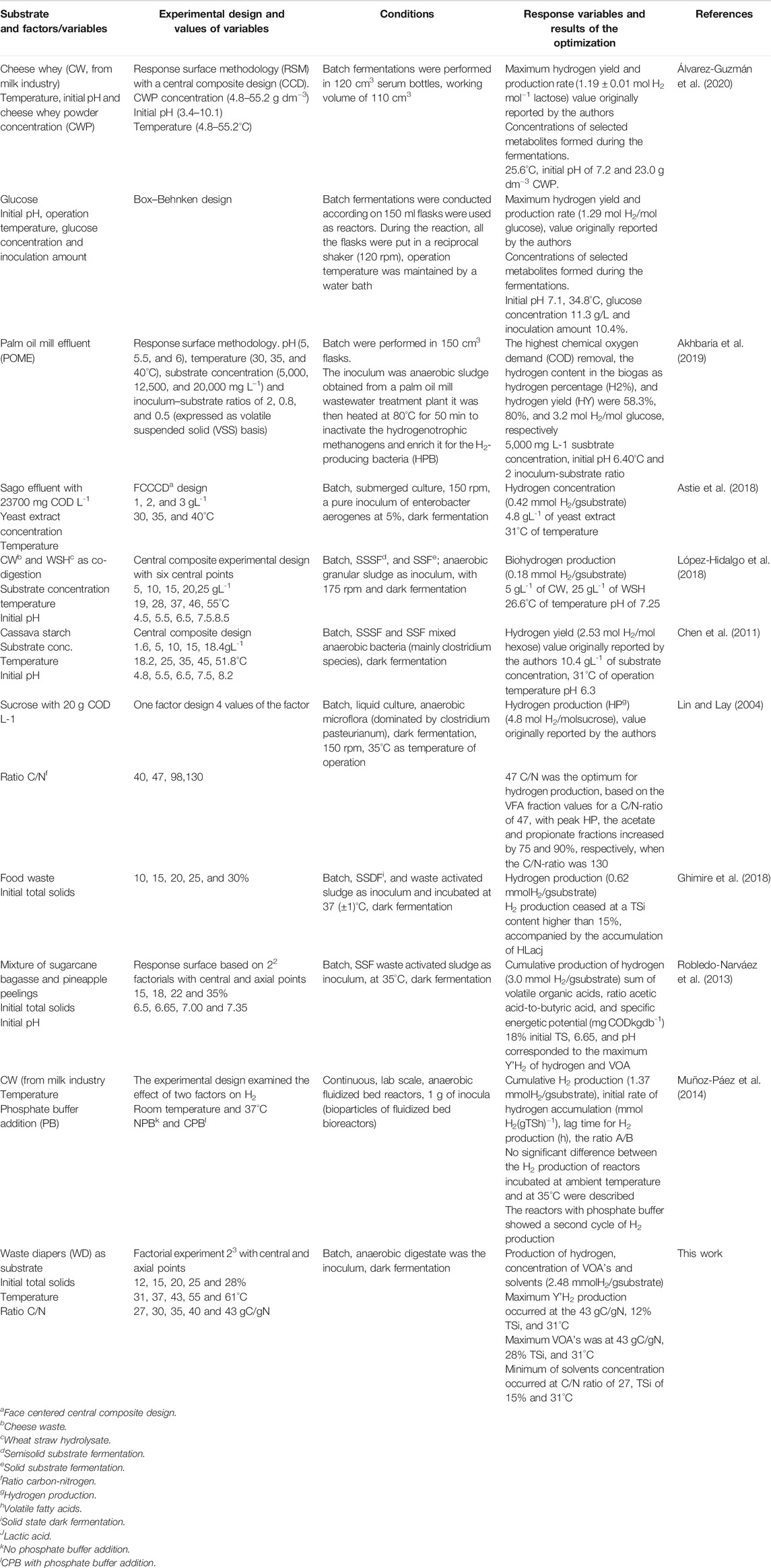
TABLE 4. Results of several studies on optimization of biohydrogen production from dark fermentation of organics substrates or wastes.
Generally, it seems that the maximum biohydrogen production, as well as ΣVOA, occurred in the range 25–31°C (6 works), like what was found in our work with OFWD. From the energy/economic point of view it is advantageous that maximum yields of the biofuel H2 could occur at relatively low temperatures, i.e., the low side of the mesophilic range in our case. Indeed, significant savings in heating substrates and media (sensible heat), as well as thermal energy (heating loads) savings in keeping the process temperature of the major equipment, pipes, appurtenances (heat loss to the surroundings), can be expected.
Few works have addressed the effect of C/N ratio on biological H2 generation. One work assayed the effect of the ratio C/N of the feed (in the range 40–139; Lin and Lay, 2004); it was found that the maximum value occurred at a C/N ratio of 47; glucose was the substrate. Abdullah et al. (2020) analysed a C/N range from 47 to 190; they found that the highest H2 production occurred at C/N = 140. Kalil et al. (2008) studied the range from 36 to 230 and reported an optimal value of 70. In our work, we found that maximum biohydrogen production occurred at C/N = 43, a comparatively lower value than those of Abdullah et al. (2020) and Kalil et al. (2008), but close to results reported by Lin and Lay (2004) (Table 4). The practical consequence of our optimum C/N ratio of 43 is that diapers and lignocellulosic substrates should be supplemented with nitrogen sources, preferably wastes rich in N, before fermentation.
In solid substrate fermentation (SSF) and semisolid substrate fermentation (SSSF) works, the trend from Table 4 indicates that the level of TSi in the feed (that can be interpreted as the surrogate of concentration of substrate in this particular type of fermentation) also had a significant influence on biohydrogen production. Typically, the highest values occurred when TSi was in the range of 15–18%. This was consistent with our findings. Robledo-Narváez et al. (2013) observed the maximum Y’H2 at low TSi of their agroindustrial organic waste. In their case, an increase of feedstock TSi (the feedstock consisted of agroindustrial organic wastes) was associated with solvents accumulation in the fermented solids and lower yields of H2. In our experiments, even if there was no evidence of solvent accumulation irrespective of the values of TSi (solvent concentrations were in the low range, 9–239 mg/kg), a decrease of bioH2 production with increasing TSi was observed.
Ghimire et al. (2018) found that an increase in TSi corresponded with an increase in ΣVOA. A similar trend was observed in our work, where maximum ΣVOA corresponded to 28% TSi instead of 18% that was the value for maximum bioH2 production.
Patterns of Volatile Organic Acid Concentrations
Trends of Main Effects of Factors on VOA and Lactic Acid Production
Table 5 contains the main effects of the factors on the response variables VOAs, HLac, and A/B. The information is useful, although it should be taken with caution because the main effects do not consider the interactions among the factors. In contrast, the response hyper-surface analysis does.
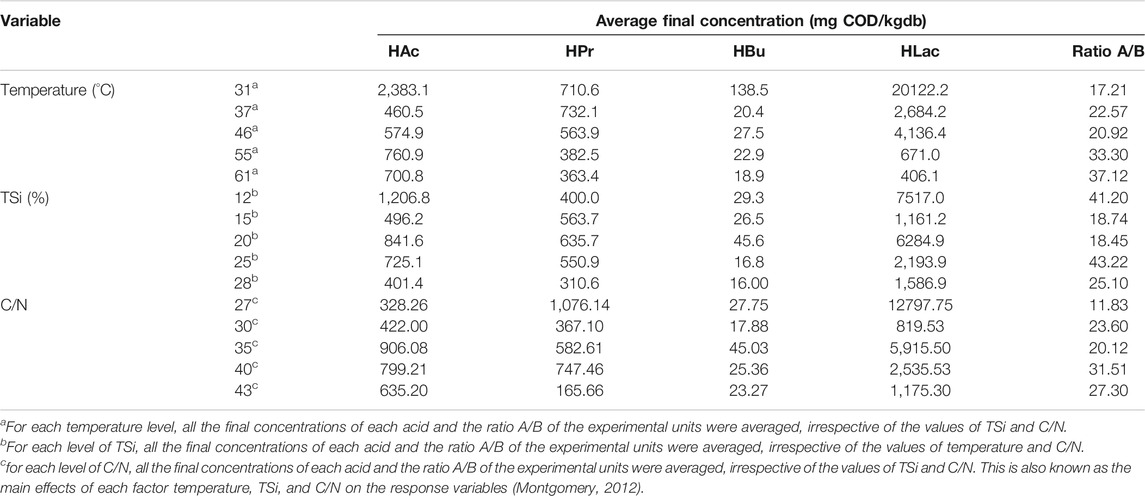
TABLE 5. Average final concentrations of volatile organic acids, lactic acid, and ratio acetic acid-to-butyric acid A/B for each level of the main factors temperature, initial total solids, and ratio C/N.
Regarding the overall effect of temperature, it seems that the low side of the mesophilic regime favoured the accumulation of VOAs and HLac. In general, the ratio A/B increased with temperature.
Concerning the effect of TSi, as a general trend, it seems that higher concentrations of HAc and HLac occurred at the low-to-central side of the TS range. Concentrations of HPr and HBu were substantially lower, and their patterns were different from those of HAc and HLac. The first couple seemed to reach a maximum in the central point of TSi i.e., 20%. The ratio A/B did not show a consistent pattern.
Finally, the effect of C/N depended on the response variable. For instance, HAc, HBu concentrations, and the ratio A/B exhibited maxima at medium-to-high C/N. On the other hand, HLac displayed a maximum at the lowest value of C/N, whereas the ratio A/B showed a significant minimum at the same point.
In particular, the combined trends of HAc concentration, with maxima in the low side of the mesophilic regime, the low side of the TSi range, and the medium-to-high values of C/N range, is consistent with the optimum predicted of Y’H2 that occurred at 31°C, 12% TSi, and 43 C/N.
Referring to Table 6 that gathered the main results for the 15 treatments, we defined ‘high concentration of a chemical species’ when its Conc >1.5 average Conc.
The following trends can be highlighted:
In all treatments, HAc > HBu, and in most cases, HAc >> HBu.
In all units, A/B > 0.79, which is the threshold associated with a predominance of the fermentation pathway hexose to 4H2 plus HAc over the route hexose to 2H2 plus HBu that are characteristic of strains of the genus Clostridia (Escamilla-Alvarado et al., 2012; Robledo-Narváez et al., 2013). To some extent, these results suggest that in most treatments, it was likely that the microbial consortia were enriched in bacteria that could ferment the hexose to 4H2 with the concomitant production of HAc, considering the DF of hexose as a model substrate for the glycolysis and further fermentations.
5 treatments exhibited high concentrations of HAc; three corresponded to the mesophilic range and central point temperature and medium contents of initial TS as well as moderate-to-high values of C/N;
3 treatments displayed relatively high concentrations of HPr that corresponded to the mesophilic range and central point temperature, medium contents of initial TS as well as moderate-to-high values of C/N;
2 treatments had relatively high concentrations of HBu in the low side of the mesophilic range and the central point of temperature, medium TSi, and medium-to-high C/N.
5 treatments showed high concentrations of HLac, distributed in the mesophilic range and central point of temperature, low-to-moderate TSi, and medium-to-high C/N.
According to Cabrol et al. (2017) and Ramírez-Morales et al. (2015), H2 generation from hexoses as a model substrate in DF typically follows two pathways catalyzed by particular sets of coenzymes. One route relies on the reoxidation of nicotinamide adenine dinucleotide (NADH). The second pathway presents the key enzyme pyruvate-formate-lyase (PYFL) that gives the name to the route. As a common denominator, the hexose (glucose) is first converted to pyruvate, which is converted to acetyl-CoA plus formate or reduced ferredoxin (redFdx).
In the PYFL pathway, the formate is split into CO2 and H2 catalyzed by formate-hydrogen lyase. This route is characteristic of some facultative anaerobes, typically members of the family Enterobacteria, and some members of Clostridia. The microorganisms that exhibit only the PYFL route in principle are limited to a generation of 2 mol H2/mol hexose, Eq. 6 below (Brock and Madigan, 1991).
ptIn the second route, the H2 generation is performed by oxidizing the redFdx in concert with a ferredoxin-dependent hydrogenase (Fdx-[FeFe]). Obligate anaerobic microbes exhibit this pathway. For example, selected members of Clostridia can oxidase the NADH generated during glycolysis; in this way, two more moles of H2 are generated, and a total 4 moles of H2 per hexose can be obtained, accompanied typically by 2 mol of HAc, Eq. 7 (Brock and Madigan, 1991).
Regarding the acetyl-CoA again, this compound is converted into either HAc, or HBu, or EtOH. Type and distribution of these metabolites depend upon the selective pressures (for example, environmental/process conditions) and the type of microbe.
Summarizing, the overall net H2 produced depends on the main metabolite pathway. On the one hand, if the microorganisms deal with the acetate route, a maximum yield of 4 molH2/mol glucose could be harvested. On the other hand, either the butyrate or EtOH pathway provide a maximum of 2 molH2/mol hexose glucose. The EtOH pathway in our work did not seem to be very active since the solvent concentrations were very low in all treatments. This, in turn, suggested that bacteria that convey this pathway, such as Enterobacteriaceae were not very active in our treatments.
According to Cabrol et al. (2017) and very important, maximum H2 yields from hexose fermentation are attained when only low molecular weight fatty acids are produced and ignoring the substrate used for microbial growth If the latter is considered, along with “losses” of substrate and H2, the above pathways' actual yields can be up to 50% lower than the theoretical yields of 4 and 2 mol H2/mol hexose. Therefore, articles in the literature that report four or higher yields deserve to be scrutinised with care (Lin and Lay, 2004) because the results are very debatable.
A significant example of H2 “loss” relevant to our experimental results is the accumulation of HLac in the analysis of selected treatments, as shown in Tables 5, 6. Pyruvate from the glycolysis can be converted to HLac (homolactic and heterolactic fermentations), and then less pyruvate is available for conversion to acetyl CoA. This, in turn, typically leads to decreased or collapsed H2 generation. This type of negative impact on H2 production is known as competition for the substrate. In our experiment, methanogenic and solvent deviations of the DF were negligible: CH4 was not found in the biogas-H2, solvents concentrations at the end of the experiments were relatively low in all the treatments. However, in our work, the lactic fermentation seemed to be important in selected treatments.
In addition to the competition for substrate, there are other issues of the HLac generation/accumulation, such as tne impact on pH and excretion of toxic substances to the hydrogen-producing bacteria (HPB). A discussion on how the lactic deviation of the DF affected our results, as well as the estimation of the “lost” H2 due to HLac, is presented with detail in the subsection “Lactic acid and “lost” hydrogen” below.
Response Surfaces for Acetic and Lactic Acids
The key chemical species were acetic and lactic acid because they reached high concentrations in selected “treatments” and showed interesting patterns. Therefore, each one was analysed as a response variable; we determined the equation of the corresponding response surface, the contour graphs, and the coordinates of factors where maximum or minimum values of HAc and HLac were predicted.
For the concentration of HAc, the quadratic equation of the response hypersuface was given by Eq. 8 below:
where the values of the factors should be in original units (not standardized).
The maximum predicted value was 2,872 mg COD-HAc/kgTS (Table 7). It occurred at Temperature = 31°C, C:N = 43 gC/gN; and TSi = 28%, that is, a region characterized by low side of the mesophilic range, high C/N ratio and high total solids.
Again, the maximum of (HAc) was found at the low side of the mesophilic regime, a trend that paralleled the temperature where the maximum Y’H2 was reached. The coordinates for optimum (HAc) in terms of C/N and TSi do not coincide with the trends examined in Table 5 on the main effects. This was somewhat expected because the RSM equation takes into account the interaction of factors whereas the analysis of main effects does not.
Supplementary Table S8 compiles the values of the coefficients of the quadratic hypersurface.
Typically, high TS contents can lead to accumulation of HLac and low H2 yields. However, in our case, the RSM predicted a positive influence of TSi on HAc concentration (increase-increase) but not on HLac. This suggested that there could be a predominance of the pathway.
Another issue that could be interesting to know more is the role of lower water activity on the different groups of fermentative microorganism at high TS, i.e., whether the low water activity would affect more the LAB than the Clostridia microbes and alike.
A contour plot of HAc is shown in Supplementary Figure S2. It can be seen that HAc concentration increases in a diagonal SW to NE, with a maximum at high TS and high C/N when the temperature is 31°C.
The quadratic equation of the response hypersurface for lactic acid was given by Eq. 9 below.
where the values of the factors should be in original units (not standardized).
The maximum predicted value was 18,709 mg COD-HLac/kgTS. It occurred at Temperature = 31.0°C, C/N = 33.9 gC/gN, and TSi = 19.6% (Table 7.) The coordinates of the region of the maximum predicted by Eq. 9 are generally consistent with the trends depicted in Table 5 of the main effects. Supplementary Table S9 compiles the values of the coefficients of the quadratic hypersuface.
In principle, the region of maximum HLac is a location to be avoided in our process, contingent with results of Y’H2 due to possible H2 “losses” associated with the competition for substrate and other effects of the lactic fermentation and LAB on the HPB and their metabolism.
A contour plot of HLac concentration (Supplementary Figure S2) confirmed a maximum value around central (moderate) TSi and C/N, for a set value of the temperature of 31°C.
It is worth highlighting the differential role of TSi on HAc and HLac production. As discussed above, HAc maximum concentration was predicted at high TSi. That was not the case for HLac, whose maximum was predicted at central values of TSi. One may speculate that lower water activity's influence would have more severe on LAB than on other groups of fermentative bacteria such as Clostridia. Regarding water activity (aw) and lactic fermentation, Larsen and Añón (1989) examined the influence of aw on HLac production from milk. They worked with Lactobacillus delbrueckii ssp. bulgaricus and Streptococcus thermophilus. It was observed that decreasing aw led to an inhibition of HLac generation. Also, the minimum aw in HLac production of the solids concentration in milk adjusted with sucrose or glucose was lower than that of milk solids adjusted with glycerol.
Shah and Ravula (2000) determined the effect of aw on yoghurt's fermentation utilised in the manufacture of frozen dairy desserts. The aw was adjusted by sugars addition. They found that sugar concentration higher than 12% resulted in low survival of LAB and longer fermentation times. The authors also observed that Lactobacillus acidophilus and bifidobacteria’s growths were negatively affected by 16% sugar concentration, the first more than the second.
Multivariate Statistical Analysis of Concentrations of VOA, Lactic, and Selected Variables
To further explore possible patterns of organic acids in our work, we performed a principal component analysis (PCA) to the set of variables organic acid concentrations, ratio A/B, end (final pH), and the factors ratio C/N, initial total solids TSi, and operation temperature to search for latent trends and relationships among fermentation metabolites. For an introduction on PCA’s benefits and advantages, see Moreno-Medina et al. (2017) and Alvarez-Guzmán et al. (2020).
In the following discussion, we will refer with the word “objects” to the treatments (15) in our experiment (Table 6). The expression “original variables” will mean the set of organic acid concentrations, A/B ratio, end pH, and the three factors.
Supplementary Table S10 shows the results of the Pearson correlation between the variables. There were seven significant positive and three significant negative correlations. Among the first group, we found positive correlations between HAc concentration with all the organic acids. Also, HBu and HLac concentrations were correlated each with all the organic acids as well. Therefore, when performing the PCA, one might expect that one principal component would represent something like a “metabolite component” and express the relationships between organic acids determined in this work.
From the biological point of view, the occurrence of these positive correlations is consistent with the mechanisms of fermentation that are typically active in DF, as discussed in the sub-sections ‘Trends of main effects of factors on VOA and lactic acid production’ above and ‘Lactic acid and “lost” hydrogen’ below. Succinctly, among these mechanisms, we highlight the production of HAc and HBu by members of the Firmicutes from acetyl Co-A, and the production of HLac from pyruvate by both homolactic and heterolactic pathways (Brock and Madigan, 1991; Escamilla-Alvarado et al., 2012; Saady, 2013; Zumar and Mohee, 2016). The contribution of HLac to HAc and HBu pools by the production of H2, from HLac, as well as the role of syntrophic bacteria that anaerobically transform HBu and HPr to HAc plus H2 cannot be ruled out, despite their possible unfavourable thermodynamic conditions (barely endergonic or plainly exergonic at biological standard conditions.)
Two out the three factors of the experiment participated in the negative correlations. The latter were T-HBu, T-HLac, and TSi-pH. On the one hand, they expressed that HLac and HBu concentrations increased at lower operation temperature values. As expected, this was consistent with the experimental results shown in Table 6.
The third negative correlation represented the increase of substrate concentration (organic matter content of the feed is proportional to TSi in the bioreactors) associated with decreases final pH. This makes sense since, at high TSi, there is more organic matter available for fermentation and in principle, high absolute amounts of one or more organic acids can be expected. For instance, this was consistent with the predicted maximum of [HAc] for high TSi in the RSM analysis above. The software extracted three principal components with eigenvalues λ > 1 (Table 8 and Supplementary Figure S3). They explained up to 77% of the variance of the experiment.
Table 9 depicts the coordinates of the original variables in terms of the main three principal components (important values of coordinates are highlighted in italics). In this regard, the PC1 held 38% of the experimental variance. It can be interpreted as a new variable that expresses the concentrations of the treatments' organic acids and its anticorrelation with temperature. The PC1 summarises the occurrence of several treatments that exhibited high concentrations of organic acids in the experiment's low range of operation temperatures (Tables 5).
On the other hand, PC2 represented approximately 22% of the variance and mainly denoted the anticorrelation between TSi and pH. We also found this anticorrelation at the time of presenting the Pearson matrix results (Supplementary Table S10); it was already discussed above.
Finally, PC3 held approximately 17% of the variance and conveyed the anticorrelation effect between ratio A/B and ratio C/N. Likely, it indicated that treatments with low C/N in the feedstock were associated with A/B, i.e., with a possible predominance of the pathway that generates H2 and acetic acid from hexoses over the route that produces H2 and HBu (Robledo-Narváez et al., 2013; Cabrol et al., 2017).
Figure 5 and Supplementary Table S11 show the coordinates of the objects in the planes PC1-PC2 and PC1-PC3. The numbers of the objects correspond to the numbers of each treatment in Table 6.
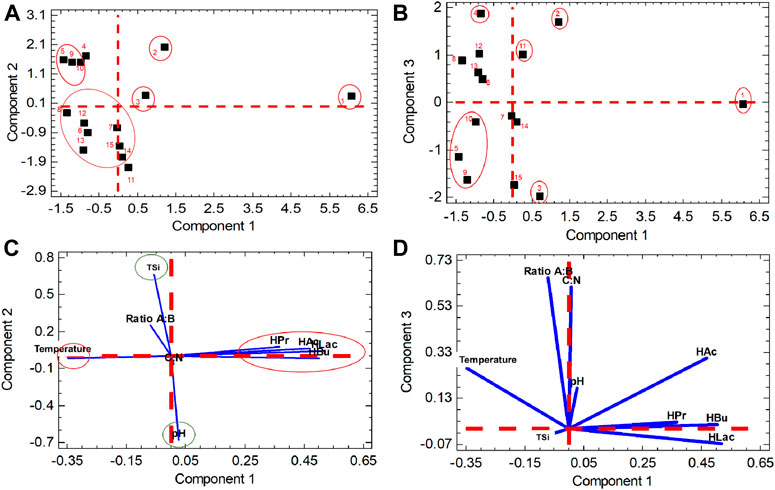
FIGURE 5. Objects vs. Components; (A) in the PC1-PC2 plane; (B) in the PC1-PC3 plane; and Original variables vs. Principal Components: (C) in the PC1-PC2 plane; (D) in the PC2-PC3 plane.
Particularly in Figure 5 can be seen the tentative grouping of the treatments. Please note that the reader must examine and compare the frames (a) and (b) of Figure 5 simultaneously to check the best empirical grouping. For instance, in the frame (b) it is evident that the treatments 4 and 11 are separated from others, which is not so clear in the frame (a). [Therefore, the treatment 11 was not grouped with treatments 6-7-8-12-13-1415 in the frame (a).]
First, there is a set of five separated treatments, characterized by either simultaneous high HAc and HLac, or one high and the other moderate, etc., accompanied by different values of the levels of the factors, that was constituted by treatments 1, 2, 3, 4, and 11. They have little in common. Each one was surrounded by its own red circle.
Second, there is a group that included treatments 5, 9, and 10. These treatments were characterized by high TSi, moderate organic acids concentrations, and low final pH.
Third, there is a group that consisted of seven treatments. They shared some common features such as low to moderate concentrations of HAc and HLac, and relatively high final pH.
It is worth highlighting that grouping the objects by PCA was very approximate, because only 77% of the information is used (Miller and Miller, 2018). Please recall that only the first three principal components are used. Therefore, it is needed to carry out a cluster analysis that uses all the experiment information for grouping the treatments. This analysis will be shown at the end of this subsection.
Figure 5 (c) and (d) depicts the positions of the original variables in the PC1-PC2 and PC1-PC3 planes. The two groups in red, together, express the vector or battery of correlated concentrations of organic acids, anticorrelated to temperature, that reflects the composition of the PC1 (Table 8).
On the other hand, the two variables circled in green revealed the anticorrelation between the end pH and TSi, that is relevant to the composition of PC2 (Table 8). Finally, with no circling, it can be appreciated some opposition between ratio C/N and ratio A/B, that reflects the anticorrelation between these two variables conveyed in the composition of PC3 (Table 9).
The implications of these results in terms of biochemical pathways have been discussed in the presentation of PC compositions above as well as in other subsections (‘Trends of main effects of factors on VOA and lactic acid production,’ ‘Lactic acid and “lost” hydrogen’.)
A cluster analysis was performed for the nine variables, based on the Euclidean distance and the closest neighbour algorithm (Moreno-Medina et al., 2017; Miller and Miller. 2018). Results are shown in Figure 6 and Supplementary Table S12.
Considering an Euclidean distance of ca. 4.15 for cut-off, the dendrogram showed seven groups (Figure 6). To a great extent, the grouping was similar to that obtained with Figure 5 of the PCA. However, the cluster analysis works as a more secure confirmation because it uses all the experimental information.
Five out the seven groups were separate treatments, i.e., 1, 2, 3, 4, and 11. They did not have very much in common. They were characterized by either simultaneous high HAc and HLac, or one high and the other moderate, etc., accompanied by different values of the levels of the factors C/N and TSi. In general, the operation temperature was from low to central values, except for treatment four in the thermophilic range.
A second group included treatments 5, 9, and 10. These treatments were characterized by high TSi, low-to-moderate organic acids, and low final pH. Likely, high TSi meant higher organic carbon source (proportional to TSi) and this, in turn, after fermentation would have resulted in a higher mass of organic acids, lowering the pH.
Third, there was a group that consisted of seven treatments. They shared some common features such as high TSi, and relatively high final pH. This somewhat inverse feature was consistent with results in the Pearson correlation matrix (Table SM10) and the composition of PC2 (Table 9). The treatments in this group exhibited low to moderate concentrations of HAc and HLac.
Lactic Acid and “Lost” Hydrogen
In our experiment, it was very likely that some LAB could survive the inocula's heat-shock and produce significant concentrations of HLac in selected treatments (treatments 1, 2, 3, 11 in our RSM experiment, Table 6) whereas simultaneously the average H2 production was still reasonable.
This was somewhat unexpected because it is generally perceived that lactic fermentation is deleterious to HPB and their H2 production.
Some of the beneficial effects of LAB on H2 production discussed below could explain the paradox that occurred in several treatments of our work because, despite the high concentrations of HLac, the H2 production did not collapse.
A representative reaction involving lactate in dark fermentation is the homolactic fermentation of hexoses:
This biochemical reaction does not produce any H2. The microbes use this reaction to perform substrate-level phosphorylation that leads to the production of two ATP per reaction (Brock and Madigan, 1991). The enzyme lactate-dehydrogenase catalyses the interconversion between pyruvate and lactate that produces two molecules of HLac. This, in turn, allows the interconversion of NADH and NAD+. Examples of microorganisms that carry out this pathway are Lactobacillus delbrueckii and Streptococcus thermophiles.
On the other hand, other bacteria can ferment hexose to HLac via heterolactic pathways. Up to three fermentation patterns have been described when glucose is the substrate (Nelson and Werkman, 1935; BioCyc, 2020):
(i) the production of HLac, EtOH, and CO2 by the phosphoketolase route,
(ii) the formation of glycerol along with HLac, HAc, and CO2, and
(iii) the pathway of Bifidobacterium bifidum that generates five ATPs, HLac. and HAc from 2 moles of glucose
Equation 11 below represents an example of the first pattern where one ATP is produced:
with a ΔGo’ nearly half of that of Eq. 10.
Some taxa are known to perform the heterolactic fermentation pattern (i) include strains from Lactobacillus genus (casei, brevis, fermentum, sanfranciscensis, buchneri) and Leuconostoc genus (i.e., mesenteroides cremoris, lactis, mesenteroides dextranicum), and genus Weissella.
The biochemical reaction characteristic of Bifidobacterium bifidus follows:
Lactate, in turn, could generate H2 as follows (Saady, 2013).
Thus, it has been recognized that HLac can be involved in both “H2-consuming” (more specifically, “H2-non producing”, Eqs. 10–12) and “H2-producing” processes (Eq. 13) (Saady, 2013; Zumar and Mohee, 2016). The latter is not thermodynamically very favourable since the free energy of Gibbs change is marginally negative. Thus, even if this pathway is present in fermentations driven by consortia, its contribution to HLac removal would be likely low. HLac can be a significant metabolite in DF, typically associated with a zero-H2 balance pathway.
According to Cabrol et al. (2017), the bacteria of the order Lactobacillales of the LAB are typically mesophilic, aerotolerant anaerobes. They cannot sporulate. However, other eubacteria can produce HLac either as primary or secondary end fermentation products that are thermotolerant and/or endospore-forming microbes.
In principle and as mentioned above, accumulation of HLac has been considered deleterious to H2 generation in DF; it implies a deviation of the acetyl-CoA pathway since pyruvate is converted to HLac in the glycolysis route (Ramírez et al., 2015).
Yet, there is growing experimental evidence that indicates that there can be a positive role played by LAB on biological H2 generation, although generalizations on the latter are still in debate (Sikora et al., 2013; Cabrol et al., 2017).
LAB's negative effect on biological H2 generation, which most often is considered to be related to process failure, could be due to the inhibition of H2-producing bacteria (HPB) and competition for substrate (pyruvate produced in the glycolysis pathway from sugars). The inhibitory action of LAB on HPB, in turn, can be effected by the excretion of toxicants by LAB (bacteriocins, H2O2) and pH decreases due to the production of HAc, HPr, HBu, and mainly HLac (Cabrol et al., 2017). Competition for substrate would imply that HLac accumulation is proportional to “lost” production of biological H2. Therefore, in general, LAB's growth and eventual dominance and alike in DF cultures are often thought to be related to process malfunction or collapse.
As a part of the debate, it seems that HLac can have beneficial effects on biological H2 generation. Cabrol et al. (2017) and Sikora et al. (2013) have stated that these phenomena could be due to a beneficial symbiosis between HPB and LAB. For example, early research by Fang et al. (2002) and Sikora et al. (2013) has shown that a significant H2 production could be sustained in DF driven by predominant Clostridia and several HLac-producing microbes (as a minority of the consortia). Interestingly, other works reported the predominance of LAB, or at least, the important presence of LAB, that coexisted with Clostridia in processes with high H2 production rates (Wongtanet et al., 2007; Laothanachareon et al., 2014).
Some conditions and characteristics associated with the beneficial effects of HLac and LAB on bioH2 production follow (Yang et al., 2007; Baghchehsaraee et al., 2009; Ohnishi et al., 2010; Marone et al., 2012; Sady, 2013; Marone et al., 2014; Zumar and Mohee, 2016; Cabrol et al., 2017):
-the presence of some other carbon source, i.e., HAc, is needed for the degradation of HLac to H2;
-the favourable effect of HLac on H2 generation in microbial consortia is generally ascribed to its capability as an electron donor;
-related to the previous feature, HLac conversion to HAc or HBu with simultaneous H2generation has also been observed in fermentative microbial consortia (for example, Eq. 13);
the latter seemed to be stimulated by the presence of acetate in fermentations driven by Clostridium diolis and other Clostridiastrains,
-there is some evidence, that selected LAB strains (a Lactobacillus strain and Lactococcus lactis) could be involved in HLac degradation with the production of H2.
As a summary, this suggests that some lactic acid bacteria can be positively involved in biological H2 generation in three ways: i) by converting HLac to H2 (Sikora et al., 2013; Saady et al.,2013); ii) by supplying additional substrate (HLac) to HPB; and iii) by an indirect contribution associated with a variety of auxiliary mechanisms discussed by Cabrol et al. (2017).
Another point is how LAB have apparently prospered and possibly dominated some cultures after the inoculum pretreatment with heat-shock in our work. This could be partially explained by the possible existence of either thermotolerant or spore-forming LAB bacteria that could have survived the heat-shock pretreatment of the inoculum.
For instance, Ramírez-Chavarín et al. (2010) reported that LAB strains (some of them employed in meat products) could survive thermal treatment. From nearly 70 presumptive LAB isolated strains, nearly ten were thermotolerant because they survived the heating test at 70°C/30 min. They belonged to genera Lactobacillus, Pediococcus, Enterococcus, and Aerococcus.
On the other hand, it has been demonstrated that members of the genus Sporolactobacillus (Firmicutes), known as HLac producers by homolactic fermentation are endospore-forming strains (Kitahara and Suzuki, 1963). They are gram-positive, anaerobic, with rod morphology. They thrive on various substrates, mainly sugars (sucrose, fructose, glucose, maltose, mannose, among others). The optimum growth temperature is 35°C, with an interval starting at the high side of the psychrophilic range (25°C) up to 40°C. Thus, on the one hand, these strains could survive the heat-shock of the inoculum. On the other hand, they could produce HLac from hexoses and sugars derived from the holocellulose in the mesophilic regime. This is consistent with results on our treatments 1, 2, 3, 11, and Table 6, where high concentrations of HLac were observed in the mesophilic regime and central (46°C) temperature.
As mentioned above the presence of HLac could imply a loss of biohydrogen (see calculations below) likely due to competition between LAB and HPB for the substrate. Consequently, efforts should be made to eliminate LAB, particularly the thermotolerant and endospore-forming strains. Thus, it seems that there is a need to implement more effective pretreatment inoculum strategies beyond the mere one-time heat-shock. It may be speculated whether more than one heat shock could be performed during the batch process when frequent monitoring reveals increasing concentrations of HLac, or to implement a combined strategy, i.e., heat-shock and acid or alkali pretreatments (Kim et al., 2014; Dessi et al., 2018) or other combination that could effectively remove “fastidious” LAB.
Now we will focus on the estimation of the H2 production “lost” due to the production of HLac in DF. According to Escamilla-Alvarado et al. (2012), we can consider a typical scenario where 1.5 mol of HLac is produced per mole of hexose (average from homolactic and heterolactic fermentation). We can also assume an average of 3 moles of H2 per mole of hexose in the biohydrogen pathways related to Clostridia. In turn, this assumption is related to the effect of heat-shock of the inoculum, which is generally reported to inhibit/kill non-sporulating microbes and select for an inoculum rich in spore-forming bacteria such as those of key genera of Firmicutes.
Indices and technical information
For instance, for Treatment 1 (Table 6) with 30,183.3 mg HLac-COD/kgTS and 1.05 mmol H2/g TS hydrogen production, the loss of biohydrogen production is ca.
The total H2 production in Treatment 1, with no lactic deviation of the DF, would have been
The estimated amounts of losses of H2 due to lactic acid fermentation for the treatments in our experiment are shown in Table 6. There was a “peak” loss in treatments 1 and 3, approximately 60%. Five treatments exhibited H2 “losses” in the neighbourhood of 10%. The remainder displayed minor losses.
Conclusion
The predicted maximum Y’H2 occurred at the combination of factors of 43 gC/gN, 12%, and 31°C for C/N, TSi, and temperature, respectively; its value was 2.79 mmolH2/gTS. The experimental validation gave a value of 2.48 mmolH2/gTS, which shows a good agreement (11% difference). The three main factors had significant effects on Y’H2, as well as some interactions of the 2nd. order, including C/N*TSi and C/N*T.
The experimental maximum of Y’H2 with OFWD compared very favourably with biohydrogen values obtained from a wide variety of wastes (OFMSW, agricultural wastes, etc.) using the same type of batch fermentation with intermittent venting.
The ∑VOA optimisation suggested a predicted maximum at coordinates 43 gC/gN, 28%, and 31°C. That value was experimentally validated.
The treatment that simultaneously exhibited a high organic acid concentration and a still attractive Y’H2 occurred at 43 gC/gN, 19% TSi, and 31°C, with a predicted Y’H2 of 1.96 mmol/gTS and 3,767 mg VOA-COD/kgwb.
Interestingly, the predicted optima fell in the lower side of the mesophilic interval of temperatures for the three response variables explored in this work. From the energy/economic viewpoint, this represents significant savings in heating substrates and media (sensible heat) and savings in keeping the process temperature of the major equipment, pipes, and appurtenances (heat loss to the surroundings. This would be a contribution to the sustainability of the dark fermentation of OFWD.
Finally, DF of OFWD holds promise for integration to biorefineries from wastes that could co-ferment this type of residues with a variety of agricultural and agroindustrial wastes of similar characteristics (cellulosic and lignocellulosic wastes, i.e., food and textile wastes) as well as wastes rich in N for amending (lowering) the C/N ratio around 40–50 gC/gN. Also, the biorefinery approach for treating and reclaiming OFWD and other residues could be more attractive for developing countries (that are net importers of fossil fuels) if an inverse cascading approach were adopted. In this framework, first energy is produced from waste; Afterward, the fermented waste is used for value-added products generation. Finally, more bioenergy is produced from the remainder of residues that exit the upstream processes.
Data Availability Statement
The original contributions presented in the study are included in the article/Supplementary Material, further inquiries can be directed to the corresponding authors.
Author Contributions
HMP-V: Research strategy, project management, experimental design, data processing and discussion and interpretation, writing the article and other documents. PXS-N: carried out the experiments, data processing and interpretation, writing the article.
Funding
This research was funded by personal grants of HMP-V to GBAER, CINVESTAV, and partial funding from CINVESTAV.
Conflict of Interest
The authors declare that the research was conducted in the absence of any commercial or financial relationships that could be construed as a potential conflict of interest
Acknowledgments
The authors wish to thank the Editors, the Handling Editor, and the Reviewers, for their insightful comments, advice, and guidance with the manuscript. CONACYT support to PXS-N and HMP-V (Infrastructure Project). The excellent technical help of Rafael Hernández-Vera from the GBAER-EBRE Group and Gustavo Medina from Central Analítica is gratefully acknowledged. The experiments were partially funded by personal grants of HMP-V to the Environmental Biotechnology and Renewable Energies Group (GBAER-EBRE) through CINVESTAV.
Supplementary Material
The Supplementary Material for this article can be found online at: https://www.frontiersin.org/articles/10.3389/fenrg.2021.630212/full#supplementary-material
Notation
A/B ratio of the acetic acid concentration to butyric acid concentration, both on a COD basis
Aj external area of the bioreactor or tank, j = 1, 2, or opt
aw water activity
BR bioreactor
C/N carbon-nitrogen ratio
CI confidence interval
COD chemical oxygen demand
cp specific heat of the OFWD
CPB with phosphate buffer addition
CPI chemical and process industry
CW cheese whey
db dry basis
DF dark fermentation
Dj internal diameter of the bioreactor or tank, j = 1, 2, or opt
EtOH ethanol
FCCCD face centered central composite design
Fexp value of the Fisher statistic based on experimental results
HAc acetic acid
HBu butyric acid
HE heat exchanger
HLac lactic acid
H-M coupled process that consists of series hydrogen DF followed by anaerobic
digestion
HPB hydrogen-producing bacteria
HPr propionic acid
KE kinetic energy
LAB lactic acid bacteria
LP low pressure
m′ mass flowrate of OFWD
NA not applicable
NADH nicotine adenine dinucleotide (reduced)
NPB no phosphate buffer addition
OFMSW organic fraction of municipal solid waste
OFWD organic fraction of the waste diaper
opt optimal or optimum
PC principal component
PCA principal component analysis
PE potential energy
PI prediction interval
POME palm oil mill effluent
p see p-value below
p-value probability of the Fisher statistic based on experimental results
PYFL pyruvate formate liase
Q′ loss rate of heat loss to ambient temperature of bioreactor and ancillary equipment sensible,HE rate of heat consumed to increase the temperature of the OFWD
Q′ loss rate of heat loss to ambient temperature of bioreactor and ancillary equipment sensible,HE rate of heat consumed to increase the temperature of the OFWD
ȓ average rate of H2 production in the net period of incubation
R2 correlation coefficient of the model
redFdx reduced ferredoxin
RSM response surface methodology
S.E standard error
SPA sodium polyacrylate
SPA-Ca sodium polyacrylate plus calcium
SM upplementary Material
Solv solvents
SSAHF-IV solid substrate anaerobic hydrogen fermentation with intermittent venting and headspace flushing
SSDF solid-state dark fermentation
SSF solid substrate fermentation
SSSF semisolid substrate fermentation
T temperature of operation
T1 temperature of the mesophilic process
T2 temperature of the thermophilic process
Topt optimal temperature of the process for biohydrogen production
TS total solids or dry matter
TSi initial total solids content
U overall heat transfer coefficient of the heat losses to the atmosphere
U1 ibidem of the mesophilic process
U2 ibidem of the thermophilic process
Uopt ibidem of the optimal process
VIF Inflation factor of the variance
VOA volatile organic acids, i.e., low molecular weight fatty acids
VS volatile solids volatile solids
VS volatile solids volatile solids
VSS volatile suspended solids
W′ shaft mechanical work
wb wet basis
WD waste diapers
WSH wheat straw hydrolysate
Y’H2 production of H2 lost production of Hydrogen due to HLac generation
Y’H2 production of H2 lost production of Hydrogen due to HLac generation
zj wet height of the bioreactor or tank, j = 1, 2, or opt
Greek characters
β correction factor for the heat losses from the top and bottom areas of bioreactor or tank. It accounts for the fact that the U for these surfaces is lower than the U for the side area of the bioreactor/tank. Typically, β = 1
δ ratio z/D of the bioreactor or tank
Δ change or generic increase of a variable placed at the right of the symbol
γ coefficient of thermal variation of U and thermal conductivities (1/K)
ΔG°′ change of standard free energy of Gibbs at neutral pH for the associated biochemical reaction
ΔHr enthalpy of reaction for the DF
λ eigenvalue in principal component analysis
ϕ additional heat losses to the atmosphere of ancillary equipment, as a decimal fraction of the heat loss of the bioreactor
ρ density of the OFWD ratio of the sum of volatile organic acids to the sum of solvents, both numerator and denominator on a COD basis
ρ density of the OFWD ratio of the sum of volatile organic acids to the sum of solvents, both numerator and denominator on a COD basis
ΣVOA sum of volatile organic acids, i.e., low molecular weight organic acids, on COD basis
ΣSOLV sum of solvents (acetone, butanol, ethanol, methanol) on COD basis
θ (mass or hydraulic) retention time in DF continuous process, or incubation time in batch process
ω1 heat process savings, in percentage, of the optimal temperature process with; respect to mesophilic one
ω2 heat process savings, in percentage, of the optimal temperature process with; respect to thermophilic one
References
Abdullah, M. F., Jahim, J. M., Abdul, P. M., and Mahmod, S. S. (2020). Effect of carbon/nitrogen ratio and ferric ion on the production of biohydrogen from palm oil mill effluent (POME). Biocatal. Agric. Biotechnol. 23, 101445. doi:10.1016/j.bcab.2019.10144510
Akhbaria, A., Zinatizadeh, A. A., Vafaeifarda, M., Mohammadi, P., Zainala, B. S., and Ibrahim, S. (2019). Effect of operational variables on biological hydrogen production from palm oil mill effluent by dark fermentation using response surface methodology. Desalin. Water Treat. 137, 101–113. doi:10.5004/dwt.2019.23169
Akutsu, Y., Li, Y. Y., Tandukar, M., Kengo, K., and Hideki, H. (2008). Effects of seed sludge on fermentative characteristics and microbial community structures in thermophilic hydrogen fermentations of starch. Int. J. Hydrogen Energy 33, 6541–6548. doi:10.1016/j.ijhydene.2008.08.038
Alvarez-Guzmán, C. L., Cisneros-de la Cueva, S., Balderas-Hernández, V. E., Smoliński, A., and De León-Rodríguez, A. (2020). Biohydrogen production from cheese whey powder by Enterobacter asburiae: effect of operating conditions on hydrogen yield and chemometric study of the fermentative metabolites. Energy Rep. 6, 1170–1180. doi:10.1016/j.egyr.2020.04.038
Amorim, E. L. C., Takano, S. L., and Silva, E. L. (2012). Effect of substrate concentration on dark fermentation hydrogen production using an anaerobic fluidized bed reactor. Appl. Biochem. Biotechnol. 166 (5), 1248–1263. doi:10.1007/s12010-011-9511-9
Andrew, D. E., Arnold, E. G., and Lenore, S. C. (2005). Standard methods for the examination of water and wastewater. 21st Edn. Washington, DC: American Public Health Association.
Astie, T. U., Mohamad, N. I. P., and Suhaida, A. A. (2018). Optimization of culture conditions for biohydrogen production from sago wastewater by Enterobacter aerogenes using response surface methodology. Int. J. Hydrogen Energy 43, 22148–22158. doi:10.1016/j.ijhydene.2018.10.057
Baghchehsaraee, B., Nakhla, G., Karamanev, D., and Margaritis, A. (2009). Effect of extrinsic lactic acid on fermentative hydrogen production. Int. J. Hydrogen Energy 34, 2573–2579. doi:10.1016/j.ijhydene.2009.01.010
BioCyc (2020). A genomic and metabolic web portal with multiple omics analytical tools. Available at: https://biocyc.org/META/NEW-IMAGE?type=PATHWAY&object=P122-PWY (Accessed December 29, 2020).
Brock, T. D., and Madigan, M. T. (1991). Biology of microorganisms. 6th Edn. Vol. 17. Englewood Cliffs, NJ: Prentice-Hall Inc. Sections 2. and 19.9.
Brown, R. (2007). “The future of biorefining for fuels and chemicals production,” in Issues in new crops and new uses. Editors J. Janick, and A. Whipkey (Alexandria, VA:ASHS Press). 30–38.
Cabrol, L., Marone, A., Tapia Venegas, E., Steyer, J. P., Ruiz-Filippi, G., and Trably, E. (2017). Microbial ecology of fermentative hydrogen producing bioprocesses: useful insights for driving the ecosystem function. FEMS Microbiol. Rev. 41 (2), 158–181. doi:10.1093/femsre/fuw043
Cakir, A., Ozmihci, S., and Kargi, F. (2010). Comparison of bio-hydrogen production from hydrolyzed wheat starch by mesophilic and thermophilic dark fermentation. Int. J. Hydrogen Energy 35, 13214–13218. doi:10.1016/j.ijhydene.2010.09.029
Catalán, E., and Sánchez, A. (2019). Environmental impact of cellulase production from coffee husks by solid-state fermentation: a life-cycle assessment. J. Clean. Prod. 233, 954–962. doi:10.1016/j.jclepro.2019.06.100
Cavinato, C., Bolzonella, D., Pavan, P., Fatone, F., and Cecchi, F. (2013). Mesophilic and thermophilic anaerobic co-digestion of waste activated sludge and source sorted biowaste in pilot-and full-scale reactors. Renew. Energy 55, 260–265. doi:10.1016/j.renene.2012.12.044
Chen, J., Su, H., Zhou, J., Song, W., and Cen, K. (2011). Hydrogen production by mixed bacteria through dark and photo fermentation. Int. J. Hydrogen Energy 36, 450–457. doi:10.1016/j.ijhydene.2010.10.007
Clesceri, L. S., Greenberg, A. E., and Eaton, A. D. (1998). Standard methods for the examination of water and wastewater. 20th Edn. Vol. 20. Washington DC: APHA/AWWA/WPCF. 1134.
Colón, J., Mestre-Montserrat, M., Puig-Ventosa, I., and Sánchez, A. (2013). Performance of compostable baby used diapers in the composting process with the organic fraction of municipal solid waste. Waste Manage. 33 (5), 1097–1103. doi:10.1016/j.wasman.2013.01.018
Colón, J., Ruggieri, L., Sánchez, A., González, A., and Puig, I. (2011). Possibilities of composting disposable diapers with municipal solid wastes. Waste Manage. Res. 29 (3), 249–259. doi:10.1177/0734242x10364684
Dessì, P., Porca, E., Frunzo, L., Lakaniemi, A. M., Collins, G., Esposito, G., et al. (2018). Inoculum pretreatment differentially affects the active microbial community performing mesophilic and thermophilic dark fermentation of xylose. Int. J. Hydrogen Energy 43 (19), 9233–9245. doi:10.1016/j.ijhydene.2018.03.117
Ebrahimian, F., and Karimi, K. (2020). Efficient biohydrogen and advanced biofuel coproduction from municipal solid waste through a clean process. Bioresour. Tech. 300, 122656. doi:10.1016/j.biortech.2019.122656
EDANA (2008). Sustainability report 2007–2008. Available at: https://www.edana.org/docs/default-source/sustainability/edana-sustainabilityreport---2007.pdf?sfvrsn=33a7d9b3_2 (Accessed December 16, 2020).
Escamilla-Alvarado, C., Poggi-Varaldo, H. M., and Ponce-Noyola, M. T. (2017). Bioenergy and bioproducts from municipal organic waste as alternative to landfilling: a comparative life cycle assessment with prospective application to Mexico. Environ. Sci. Pollut. Res. 24 (33), 25602–25617. doi:10.1007/s11356-016-6939-z
Escamilla-Alvarado, C., Ponce-Noyola, M. T., Ríos-Leal, E., and Poggi-Varaldo, H. M. (2013). A multivariable evaluation of biohydrogen production by solid substrate fermentation of organic municipal wastes in the semi-continuous and batch operation. Int. J. Hydrogen Energy 38, 12527–12538. doi:10.1016/j.ijhydene.2013.02.124
Escamilla-Alvarado, C., Ríos-Leal, E., Ponce-Noyola, M. T., and Poggi-Varaldo, H. M. (2013). Gas biofuels from solid substrate hydrogenogenic–methanogenic fermentation of the organic fraction of municipal solid waste. Process. Biochem. 47 (11), 1572–1587. doi:10.1016/j.procbio.2011.12.006
Espinosa-Valdemar, R. M., Sotelo-Navarro, P. X., Quecholac-Piña, X., Beltrán-Villavicencio, M., Ojeda-Benítez, S., and Vázquez-Morillas, A. (2014). Biological recycling of used baby diapers in a small-scale composting system. Resour. Conserv. Recycl. 87, 153–157. doi:10.1016/j.resconrec.2014.03.015
Espinosa-Valdemar, R., Vázquez-Morillas, A., Ojeda-Benítez, S., Arango-Escorcia, G., Cabrera-Elizalde, S., Quecholac-Piña, X., et al. (2015). Assessment of gardening wastes as a co-substrate for diapers degradation by the fungus Pleurotus ostreatus. Sustainability 7, 6033–6045. doi:10.3390/su7056033
Fang, H. H. P., Liu, H., and Zhang, T. (2002). Characterization of a hydrogen producing granular sludge. Biotechnol. Bioeng. 78, 44–52. doi:10.1002/bit.10174
García-Depraect, O., Rene, E. R., Gómez-Romero, J., López-López, A., and León-Becerril, E. (2019). Enhanced biohydrogen production from the dark co-fermentation of tequila vinasse and nixtamalization wastewater: novel insights into ecological regulation by pH. Fuel 253 (1), 159–166. doi:10.1016/j.fuel.2019.04.147
Ghimire, A., Frunzo, L., Pirozzi, F., Trably, E., Escudie, R., Lens, P. N. L., et al. (2015). A review on dark fermentative biohydrogen production from organic biomass: process parameters and use of by-products. Appl. Energy 144, 73–95. doi:10.1016/j.apenergy.2015.01.045
Ghimire, A., Trably, E., Frunzo, L., Pirozzi, F., Lens, P. N. L., Esposito, G., et al. (2018). Effect of total solids content on biohydrogen production and lactic acid accumulation during dark fermentation of organic waste biomass. Bioresour. Tech. 248, 180–186. doi:10.1016/j.biortech.2017.07.062
Girotto, F., Matsufuji, Y., and Tanaka, A. (2015). Removal of ammonia using Ca-P (calcium polymer) from wastewaters produced in the recycling of disposable diapers. J. Mater. Cycles Waste Manage. 19 (1), 570–576. doi:10.1007/s10163-015-0420-9
Kalil, M. S., Alshiyab, H. S., Mohtar, W., Yusoff, W., and Selangor, B. (2008). Effect of nitrogen source and carbon to nitrogen ratio on hydrogen production using C. acetobutylicum. Am. J. Biochem. Biotechnol. 4 (4), 393–401. doi:10.3844/ajbbsp.2008.393.401
Kapdan, I. K., and Kargi, F. (2006). Bio-hydrogen production from waste materials. Enzyme Microb. Tech. 38, 569–582. doi:10.1016/j.enzmictec.2005.09.01510.1016/j.enzmictec.2005.09.015
Kargi, F., Eren, N. S., and Ozmihci, S. (2012). Bio-hydrogen production from cheese whey powder (CWP) solution: comparison of thermophilic and mesophilic dark fermentations. Int. J. Hydrogen Energy 37 (10), 8338–8342. doi:10.1016/j.ijhydene.2012.02.162
Kim, D. H., and Kim, M. S. (2012). Thermophilic fermentative hydrogen production from various carbon sources by anaerobic mixed cultures. Int. J. Hydrogen Energy 37, 2021–2027. doi:10.1016/j.ijhydene.2011.07.043
Kim, D. H., Jang, S., Yun, Y. M., Lee, M. K., Moon, C. M., Kang, W. S., et al. (2014). Effect of acid-pretreatment on hydrogen fermentation of food waste: microbial community analysis by next generation sequencing. Int. J. Hydrogen Energy 39, 16302–16309. doi:10.1016/j.ijhydene.2014.08.004
Kim, K. S., and Cho, H. S. (2017). Pilot trial on separation conditions for diaper recycling. Waste Manage. 67, 11–19. doi:10.1016/j.wasman.2017.04.027
Kitahara, K., and Suzuki, J. (1963). Sporolactobacillus nov. subgen. J. Gen. Appl. Microbiol. 9, 59–71. doi:10.2323/jgam.9.59
Kotay, S. M., and Das, D. (2008). Biohydrogen as a renewable energy resource. Prospects and potentials. Int. J. Hydrogen Energy 33, 258–263. doi:10.1016/j.ijhydene.2007.07.031
Kothari, R., Singha, D. P., Tyagi, V. V., and Tyagi, S. K. (2012). Fermentative hydrogen production – an alternative clean energy source. Renew. Sust. Energ. Rev. 16 (4), 2337–2346. doi:10.1016/j.rser.2012.01.002
Kumar, A. N., Chatterjee, S., Hemalatha, M., Althuri, A., Min, B., Kim, S.-H., et al. (2019). Deoiled algal biomass derived renewable sugars for bioethanol andbiopolymer production in biorefinery framework. Biores. Technol. 296 (4), 122315. doi:10.1016/j.biortech.2019.122315
Laothanachareon, T., Kanchanasuta, S., Mhuanthong, W., Phalakornkule, C., Pisutpaisal, N., and Champreda, V. (2014). Analysis of microbial community adaptation in mesophilic hydrogen fermentation from food waste by tagged 16S rRNA gene pyrosequencing. J. Environ. Manage. 144, 143–151. doi:10.1016/j.jenvman.2014.05.019
Larsen, R. F., and Añón, A. (1989). Effect of water activity aw of milk on acid production by Streptococcus thermophilus and Lactobacillus bulgaricus. J. Food Sci. 54, 917–921. doi:10.1111/j.1365-2621.1989.tb07912.x
Lee, D. Y., Ebie, Y., Xu, K. Q., Li, Y. Y., and Inamori, Y. (2010). Continuous H2 and CH4 production from high-solid food waste in the two-stage thermophilic fermentation process with the recirculation of digester sludge. Bioresour. Tech. 101, S42–S47. doi:10.1016/j.biortech.2009.03.037
Lee, K. S., Hsu, Y. F., Lo, Y. C., Lin, P. J., Lin, C. Y., and Chang, J. S. (2008). Exploring optimal environmental factors for fermentative hydrogen production from starch using mixed anaerobic microflora. Int. J. Hydrogen Energy 33, 1565–1572. doi:10.1016/j.ijhydene.2007.10.019
Lin, C. Y., and Lay, C. H. (2004). Carbon/nitrogen-ratio effect on fermentative hydrogen production by mixed microflora. Int. J. Hydrogen Energy 29, 41–45. doi:10.1016/S0360-3199(03)00083-1
López-Hidalgo, A. M., Alvarado-Cuevas, Z. D., and De Leon-Rodríguez, A. (2018). Biohydrogen production from mixtures of agro-industrial wastes: chemometric analysis, optimization and scaling up. Energy 159, 32–41. doi:10.1016/j.energy.2018.06.124
Marone, A., Izzo, G., Mentuccia, L., Massini, G., Paganin, P., Rosa, S., et al. (2014). Vegetable waste as substrate and source of suitable microflora for bio-hydrogen production. Renew. Energy 68, 6–13. doi:10.1016/j.renene.2014.01.013
Marone, A., Massini, G., Patriarca, C., SignoriniVarrone, A. C., and Izzo, G. (2012). Hydrogen production from vegetable waste by bioaugmentation of indigenous fermentative communities. Int. J. Hydrogen Energy 37, 5612–5622. doi:10.1016/j.ijhydene.2011.12.159
Miller, J., and Miller, J. C. (2018). Statistics and chemometrics for analytical chemistry. 7th Edn. London: Pearson Higher Education.
Mizuno, O., Dinsdale, R., Hawkes, F. R., Hawkes, D. L., and Noike, T. (2002). Enhancement of hydrogen production from glucose by nitrogen gas spacing. Bioresour. Tech. 73, 59–65. doi:10.1016/S0960-8524(99)00130-3
Montgomery, D. C. (2012). Design and analysis of experiments. 8th Edn. Arizona, United States.:Wiley.
Moreno-Medina, C. U., Poggi-Varaldo, H. M., Breton-Deval, L., and Rinderknecht-Seijas, N. (2017). Effect of sudden addition of PCE and bioreactor coupling to ZVI filters on performance of fluidized bed bioreactors operated in simultaneous electron acceptor modes. Environ. Sci. Pollut. Res. 24 (33), 25534–25549. doi:10.1007/s11356-016-7275-z
Muñoz-Páez, K. M., Poggi-Varaldo, H. M., García-Mena, J., et al. (2014). Cheese whey as substrate of batch hydrogen production: effect of temperature and addition of buffer. Waste Manag. Res. 32, 434–440. doi:10.1177/0734242x14527333
Muñoz-Paez, K. M., Ruiz-Ordáz, N., Garcia-Mena, J., Ponce-Noyola, M. T., Ramos-Valdivia, A. C., Robles-Gonzalez, I. V., et al. (2013). Comparison of biohydrogen production in fluidized bed bioreactors at room temperature and 35 °C. Int. J. Hydrogen Energy 38 (28), 12570–12579. doi:10.1016/j.ijhydene.2012.09.172
Murali, N., Srinivas, K., and Ahring, B. K. (2017). Biochemical production and separation of carboxylic acids for biorefinery applications. Fermentation 3, 22. doi:10.3390/fermentation3020022
Myers, R. H., Montgomery, D. C., and Anderson-Cook, C. M. (2009). Response surface methodology: process and product optimization using designed experiments. 3rd Edn. Hoboken, NJ: John Wiley & Sons, Inc.
Nelson, M. E., and Werkman, C. H. (1935). Dissimilation of glucose by heterofermentative lactic acid bacteria. J. Bacteriol. 30 (6), 547–557. doi:10.1128/jb.30.6.547-557.1935
Ntaikou, I., Antonopoulou, G., and Lyberatos, G. (2010). Biohydrogen production from biomass and wastes via dark fermentation: a review. Waste Biomass Valor. 1 (1), 21–39. doi:10.1007/s12649-009-9001-2
Ohnishi, A., Bando, Y., Fujimoto, N., and Suzuki, M. (2010). Development of a simple bio-hydrogen production system through dark fermentation by using unique microflora. Int. J. Hydrogen Energy 35, 8544–8553. doi:10.1016/j.ijhydene.2010.05.113
Poggi-Varaldo, H. M., Munoz-Paez, K. M., Escamilla-Alvarado, C., Robledo-Narváez, P. N., Ponce-Noyola, M. T., Calva-Calva, G., et al. (2014). Biohydrogen, biomethane and bioelectricity as crucial components of biorefinery of organic wastes: a review. Waste Manag. Res. 32 (5), 353–365. doi:10.1177/0734242x14529178
Poggi-Varaldo, H. M., and Oleszkiewicz, J. A. (1992). Anaerobic co-composting of municipal solid waste and waste sludge at high total solids levels. Environ. Tech. 13 (5), 409–421. doi:10.1080/09593339209385169
Poggi-Varaldo, H. M., Rodríguez-Vazquez, R., Fernandez-Villagomez, G., and Esparza-García, F. (1997). Inhibition of mesophilic solid-substrate anaerobic digestion by ammonia nitrogen. Appl. Microbiol. Biotechnol. 47, 284–291. doi:10.1007/s002530050928
PROFECO (2003). Estudio de calidad de los pañales desechables. A survey on quality of disposable diapers. Revista del consumidor Septiembre 2013, 32–44. México. (In Spanish). Available at: https://www.gob.mx/profeco/articulos/revista-del-consumidor?idiom=es (Accessed 15, 2010), 32–44.
PROFECO (2011). Estudio de calidad: pañales desechables. Quality studies: disposable napkins. México: procuraduría Federal del Consumidor (In Spanish). Available at: http://www.profeco.gob.mx/revista/pdf/est03/pdesech.pdf (Accessed 20, 1906).
Puhakka, J. A., Karadag, D., and Nissilä, M. E. (2012). Comparison of mesophilic and thermophilic anaerobic hydrogen production by hot spring enrichment culture. Int. J. Hydrogen Energy 37(21),16453–(16459.) doi:10.1016/j.ijhydene.2012.02.121
Ramírez Chavarín, N. L., Wacher Rodarte, C., and Pérez Chabela, M. L. (2010). Characterization and identification of thermotolerant lactic acid bacteria isolated from cooked sausages as bioprotective cultures. J. Muscle Foods 21 (3), 585–596. doi:10.1111/j.1745-4573.2009.00206
Ramírez-Morales, J. E., Tapia-Venegas, E., Toledo-Alarcón, J., and Ruiz-Filippi, G. (2015). Simultaneous production and separation of biohydrogen in mixed culture systems by continuous dark fermentation. Water Sci. Tech. 71, 1271–1285. doi:10.2166/wst.2015.104
Ripley, L. E., Boyle, W. C., and Converse, J. C. (1986). Improved alkalimetric monitoring for anaerobic digestion of high-strength wastes. J. Water Pollut. Control. Fed. 58, 406–411.
Robledo-Narvaez, P. N., Muñoz-Páez, K. M., Poggi-Varaldo, H. M., Ríos-Leal, E., Calva-Calva, G., Ortega-Clemente, L. A., et al. (2013). The influence of total solids content and initial pH on batch biohydrogen production by solid substrate fermentation of agroindustrial wastes. J. Environ. Manage., 128, 126–137. doi:10.1016/j.jenvman.2013.04.042
Roy, S. (2017). “Biological gaseous energy recovery from lignocellulosic biomass,” in Lignocellulosic production and industrial applications (book chapter). Editor V. Sharma, 27–45. doi:10.1002/9781119323686
Rodríguez-Valderrama, S., Escamilla-Alvarado, C., Magnin, J.-P., Rivas-García, P., Valdez-Vazquez, I., and Ríos-Leal, E. (2020). Batch biohydrogen production from dilute acid hydrolyzates of fruits-and-vegetables wastes and corn stover as co-substrates. Biom. Bioenergy. 105666. doi:10.1016/j.biombioe.2020.105666
Saady, N. M. C. (2013). Homoacetogenesis during hydrogen production by mixed cultures dark fermentation: unresolved challenge, Int. J. Hydrogen Energy, 38 (30), 13172–13191. doi:10.1016/j.ijhydene.2013.07.122
Sarangi, P. K., and Nanda, S. (2020). Biohydrogen production through dark fermentation. Chem. Eng. Technol. 43 (4), 601–612. doi:10.1016/j.biombioe.2020.10566610.1002/ceat.201900452
SEMARNAT (Secretaria de Medio Ambiente y Recursos Naturales) (2014). Diagnóstico básico para la gestión integral de los residuos. Basic diagnosis on integrated waste mangement in Mexico. In Spanish. Available at: https://biblioteca.semarnat.gob.mx/Documentos/Ciga/libros2009/CD001408.pdf.
Shah, N. P., and Ravula, R. R. (2000). Influence of water activity on fermentation, organic acids production and viability of yogurt and probiotic bacteria. Aust. J. Dairy Tech. 55 (3), 127–131.
Sikora, A., Blaszczyk, M., Jurkowski, M., and Zielenkiewicz, U. (2013). “Lactic acid bacteria in hydrogen-producing consortia: on purpose or by coincidence?” in Lactic acid bacteria—R & D for food, health and livestock purposes. Editor M. Kongo, Rijeka: InTech Open, 487–514.
Silva, A. J., Pozzi, E., Foresti, E., and Zaiat, M. (2014). The influence of the buffering capacity on the production of organic acids and alcohols from wastewater in the anaerobic reactor. Appl. Biochem. Biotechnol. 175, 2258–2265. doi:10.1007/s12010-014-1424-y
Solowski, G., Konkol, I., and Cenian, A. (2020). Methane and hydrogen production from cotton waste by dark fermentation under anaerobic and micro-aerobic conditions. Biomass Bioenergy 138, 105576. doi:10.1016/j.biombioe.2020.105576
Sotelo-Navarro, P. X., Poggi-Varaldo, H. M., Turpin-Marion, S. J., and Rinderknecht Seijas, N. F. (2020). Sodium polyacrylate inhibits fermentative hydrogen production from waste diaper-like material. J. Chem. Technol. Biotechnol. 95, 78–85. doi:10.1002/jctb.6208
Sotelo-Navarro, P. X., Poggi-Varaldo, H. M., Turpin-Marion, S. J., Vázquez-Morillas, A., Beltrán-Villavicencio, M., et al. (2017). Biohydrogen production from used diapers: evaluation of effect of temperature and substrate conditioning. Waste Manage. Res. 35 (3), 267–275. doi:10.1177/0734242x16677334
Technical Association of Pulp and Paper Industry (2001). Standard test method for acid-insoluble lignin in wood. Standard Method. D1106.
Torrijos, M., Sousbie, P., Rouez, M., Lemunier, M., Lessard, Y., Galtier, L., et al. (2013). Treatment of the biodegradable fraction of used disposable diapers by co-digestion with waste activated sludge. Waste Manage. 34 (3), 669–675. doi:10.1016/j.wasman.2013.11.009
Ueno, Y., Fukui, H., and Goto, M. (2007). Operation of a two-stage fermentation process producing hydrogen and methane from organic waste. Environ. Sci. Technol. 41, 1413–1419. doi:10.1021/es062127f
Valdez-Váquez, I., Ríos-Leal, E., Carmona-Martínez, A., Muñoz-Páez, K. M., and Poggi-Varaldo, H. M. (2006). Improvement of biohydrogen production from solid wastes by intermittent venting and gas flushing of batch reactors headspace. Environ. Sci. Technol. 40, 3409–3415. doi:10.1021/es062127f
Valdez-Vazquez, I., and Poggi-Varaldo, H. M. (2009). Hydrogen production by fermentative consortia. Renew. Sust. Energy Rev. 13 (5), 1000–1013. doi:10.1016/j.rser.2008.03.003
Van Soest, P. J., and Wine, R. H. (1967). Use of detergents in the analysis of fibrous feed. IV. Determination on plant cell-wall constituents. J. Assoc. Off. Anal. Chem. 50, 50–55. doi:10.1093/jaoac/50.1.50
Venkata Mohan, S., Rohit, M. V., Amulya, K., Naresh Kumar, A., Annie Modestra, J., et al. (2019). “Acidogenic biohydrogen production integrated with biorefinery approach,” in Biohydrogen. 2nd Edn, Editors A. Pandey, S. Venkata Mohan, J. S. Chang, P. C. Hallenbeck, and C. Larroche (Amsterdam, Netherlands: Elsevier B.V). Chap 14. 369–380.
Venkata Mohan, S., Sai Kishore Butti, K. A., Dahiya, S., and Annie Modestra, J. (2016). Waste biorefinery: a new paradigm for a sustainable bioelectro economy. Trends Biotechnol. 34 (11), 852–855. doi:10.1016/j.tibtech.2016.06.006
Villagran, E. (2014). Internal report in research assistant log, Solid waste project, sustainable technologies laboratory. Universidad Autónoma Metropolitan. CDMX, México: Unidad Azcapotzalco.
Wang, X. J., Lu, X. G., Li, F., and Yang, G. H. (2014). Effects of temperature and carbon-nitrogen (C/N) ratio on the performance of anaerobic co-digestion of dairy manure, chicken manure and rice straw: focusing on ammonia inhibition. PLoS One 9 (5), e97265. doi:10.1371/journal.pone.0097265
Weide, T., Brügging, E., Wetter, C., Ierardi, L., and Wichern, M. (2019). Use of organic waste for biohydrogen production and volatile fatty acids via dark fermentation and further processing to methane. Int. J. Hydrogen Energy 44 (44), 24110–(24125.)
Werpy, T., and Petersen, G. (2004). Top value-added chemicals from biomass. Vol. I: results of screening for potential candidates from sugars and synthesis gas. National Renewable Energy Laboratory Technical Report NREL/TP-510-35523; Golden, CO: doi 1500885/15008859 Available at: https://www.nrel.gov/docs/fy04osti/35523.pdf (Accessed August 01. 2004)
Wongtanet, J., Sang, B. I., Lee, S. M., and Pak, D. (2007). Biohydrogen production by fermentative process in continuous stirred-tank reactor. Int. J. Green. Energy 4, 385–395. doi:10.1080/15435070701465581
Yang, P., Zhang, R., McGarvey, J. A., and Benemann, J. R. (2007). Biohydrogen production from cheese processing wastewater by anaerobic fermentation usingmixed microbial communities. Int. J. Hydrogen Energy 32, 4761–4771. doi:10.1016/j.ijhydene.2007.07.038
Yeshanew, M. M., Paillet, F., and Barrau, C., Frunzo, L., Lens, P. N., Esposito, G., et al. (2018). Co-production of hydrogen and methane from the organic fraction of municipal solid waste in a pilot scale dark fermenter and methanogenic biofilm reactor. Front. Environ. Sci. 6. doi:10.3389/fenvs.2018.00041
Yin, Y., and Wang, J. (2019). Optimization of fermentative hydrogen production by Enterococcus faecium INET2 using response surface methodology. Int. J. Hydrogen Energy 44, 1483–1491. doi:10.1016/j.ijhydene.2018.11.154
Yokoyama, H., Ohmori, H., Waki, M., Ogino, A., and Tanaka, Y. (2009). Continuous hydrogen production from glucose by using extreme thermophilic anaerobic microflora. J. Biosci. Bioeng., 107 (1), 64–66. doi:10.1016/j.jbiosc.2008.09.010
Keywords: biohydrogen generation, total solids, ptimization, ratio C/N, temperature, response surface analysis, waste diapers
Citation: Sotelo-Navarro PX and Poggi-Varaldo HM (2021) Hydrogen from Dark Fermentation of the Organic Fraction of Waste Diapers: Optimization Based on Response Surface Experiments. Front. Energy Res. 9:630212. doi: 10.3389/fenrg.2021.630212
Received: 17 November 2020; Accepted: 14 January 2021;
Published: 05 May 2021.
Edited by:
Keikhosro Karimi, Isfahan University of Technology, IranReviewed by:
Farinaz Ebrahimian, Isfahan University of Technology, IranAnish Ghimire, Kathmandu University, Nepal
Copyright © 2021 Sotelo-Navarro and Poggi-Varaldo. This is an open-access article distributed under the terms of the Creative Commons Attribution License (CC BY). The use, distribution or reproduction in other forums is permitted, provided the original author(s) and the copyright owner(s) are credited and that the original publication in this journal is cited, in accordance with accepted academic practice. No use, distribution or reproduction is permitted which does not comply with these terms.
*Correspondence: Héctor M. Poggi-Varaldo, cjRjZXBlQHlhaG9vLmNvbQ==; Perla X. Sotelo‐Navarro, cGVybGEuc290ZWxvQGNpbnZlc3Rhdi5teA==