- 1Department of Endocrinology, The Third Hospital of Hebei Medical University, Shijiazhuang, Hebei, China
- 2Department of Pathophysiology, Hebei Medical University, Shijiazhuang, Hebei, China
- 3Department of Physiology, Hebei Medical University, Shijiazhuang, Hebei, China
- 4Hebei Collaborative Innovation Center for Cardio-Cerebrovascular Disease, Shijiazhuang, China
- 5Department of Ophthalmology, The Third Hospital of Hebei Medical University, Shijiazhuang, Hebei, China
Diabetic cardiomyopathy (DCM) is defined as structural and functional cardiac abnormalities in diabetes, and cardiomyocyte death is the terminal event of DCM. Ferroptosis is iron-dependent oxidative cell death. Evidence has indicated that iron overload and ferroptosis play important roles in the pathogenesis of DCM. Mitochondria, an important organelle in iron homeostasis and ROS production, play a crucial role in cardiomyocyte ferroptosis in diabetes. Studies have shown some anti-diabetic medicines, plant extracts, and ferroptosis inhibitors might improve DCM by alleviating ferroptosis. In this review, we systematically reviewed the evidence of ferroptosis in DCM. Anti-ferroptosis might be a promising therapeutic strategy for the treatment of DCM.
1 Introduction
The global prevalence of diabetes mellitus (DM) is increasing. Data from the International Diabetes Federation (IDF) has indicated that the global prevalence of DM was estimated to be 10.5% (536.6 million) of adults in 2021 (1). Cardiovascular disease is the leading cause of death in diabetic patients (2). Early in 1972, Rubler et al. described a pathological cardiac alteration in DM patients, which was characterized by ventricular hypertrophy and fibrosis, and termed diabetic cardiomyopathy (DCM) (3). Currently, DCM is defined as structural and functional cardiac abnormalities in diabetes, which cannot be explained by hypertension, coronary artery heart disease, valvular heart disease, or other heart diseases. DCM is the leading cause of heart failure and death in DM patients (4).
The death of cardiomyocytes is the terminal event of DCM (4–6). Ferroptosis, which was first described in 2012 by Dixon et al., is iron- and lipotoxicity-dependent cell death, and controlled by multiple pathways involved in iron accumulation, lipid peroxidation, or a disturbed antioxidant system (7). Evidence from recent years has indicated that ferroptosis participates in many heart diseases, including myocardial infarction (8), cardiac ischemia/reperfusion (I/R) injury (9), heart failure (10), myocardial hypertrophy (11), sepsis (12), and doxorubicin-induced heart injury (13). Therefore, therapies targeting ferroptosis or iron overload might be promising for cardiac diseases (14–17).
Iron overload and ferroptosis have been found to be closely correlated with diabetes and its complications (18, 19). Tissue iron overload causes increased ROS through the Fenton response, exacerbating diabetic cardiovascular complications (20). Therefore, in the present review, we summarized the related data about iron metabolism and ferroptosis and discussed their role in the pathogenesis of DCM, which may provide new evidence for the pathogenesis of DCM and its targeted therapy.
2 Iron and DCM
Iron is essential for many physiological processes including oxygen transport and mitochondrial energy metabolism. The iron enters the cardiomyocytes by chelating to transferrin, subsequently binding to the transferrin 1 receptor (TfR1) (21), but also through other routes including the T-type calcium channel (TTCC), divalent metal transporter 1(DMT1) (22), the L-type calcium channel (LTCC) (23), and Zrt-, Irt-like proteins (ZIP) 8 and 14 (24). Intracellular iron is utilized, stored bound to cytoplasmic ferritin, or imported by mitochondria. Excess iron can be extruded from cardiomyocytes by ferroportin (FPN) (Figure 1).
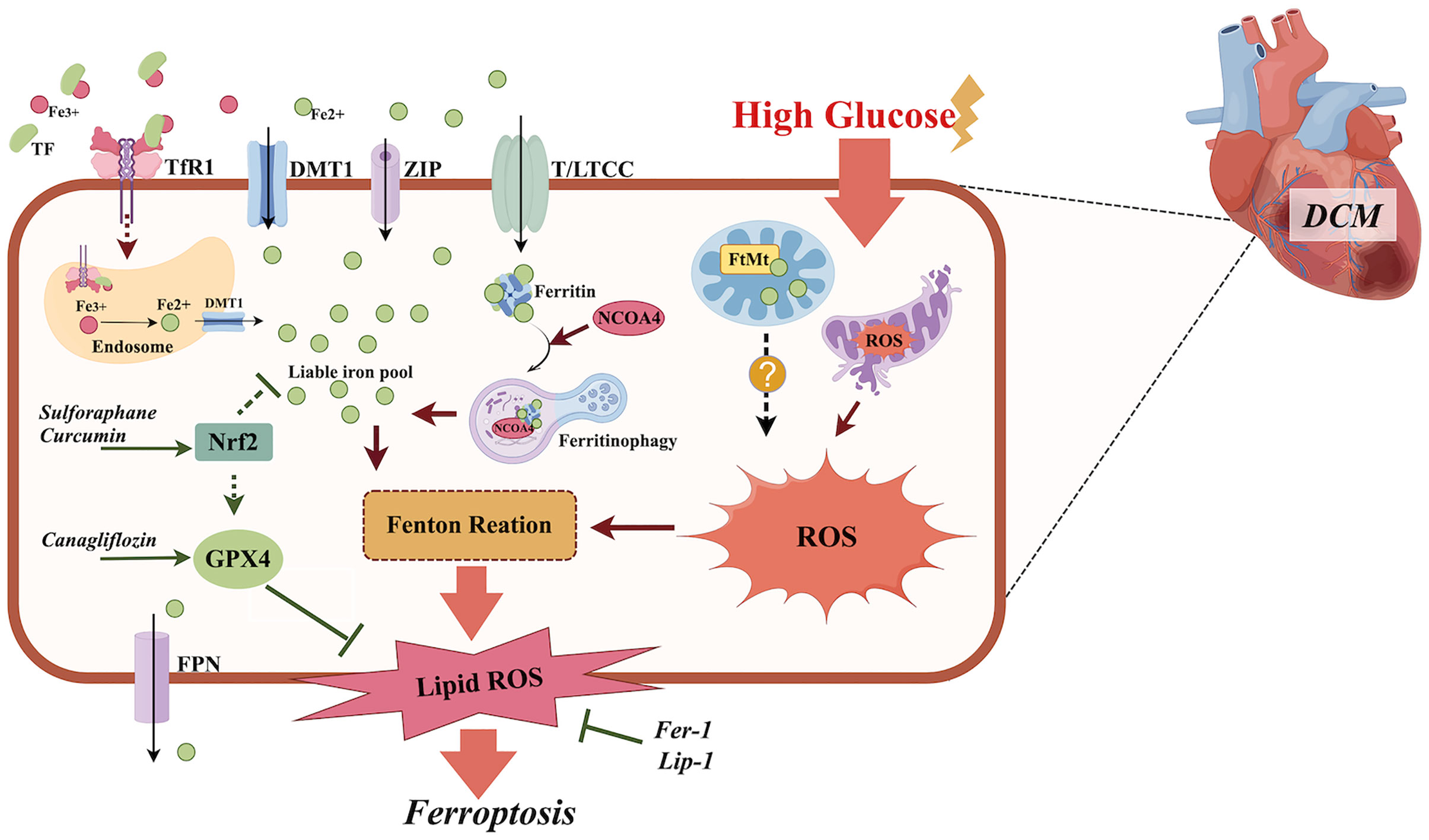
Figure 1. The role of ferroptosis in the pathogenesis of DCM. Diabetes or high glucose may cause iron overload, mitochondria disorder, increased NCOA4-mediated ferritinophagy, and an impaired anti-oxidative Nrf2/GPX4 pathway in cardiomyocytes, which all contribute to increased lipid peroxidation and ferroptosis. Ferroptosis inhibitors Fer-1 and Lip-1, anti-diabetic medicine canagliflozin, and the plant extract sulforaphane all exhibit anti-ferroptotic effects in DCM by suppressing lipid peroxidation, activating Nrf2 and promoting Xc-/GSH/GPX4 axis separately. TF, transferrin; TfR1, transferrin 1 receptor; DMT1, divalent metal transporter 1; ZIP, Zrt-, Irt-like Proteins; Nrf2, nuclear factor erythroid 2-related factor 2; NCOA4, nuclear receptor coactivator 4; GSH, glutathione; GPX4, glutathione peroxidase 4; L/TTCC, L/T-type calcium channel; FtMt, mitochondrial ferritin; ROS, reactive oxygen species; Fer-1, ferrostatin–1; Lip-1, liproxstatin–1. The figure was created by Figdraw.
Iron overload is an excessive accumulation of iron, which has been found to be associated with insulin resistance, diabetes, and its complications (20, 25). In human studies, iron overload, which is demonstrated by serum iron levels, was associated with increased fasting plasma glucose and the occurrence of type 2 diabetes mellitus (T2DM) (26), and is positively associated with higher visceral fat mass in T2DM patients (27). Iron homeostasis is important to maintain cardiac function. Cardiac iron overload has been found to participate in the pathogenesis of 5-fluorouracil(5-FU) induced cardiotoxicity (28), doxorubicin-induced cardiomyopathy (29), and cardiac I/R injury (30). It has been suggested that iron overload may induce insulin resistance in cardiomyocytes (31), manipulate cardiac calcium regulation (32, 33), cause reactive oxygen species (ROS) accumulation and lipid peroxidation (34, 35), and therefore lead to cardiac dysfunction (36). Although insulin resistance plays a key role in the pathogenesis of DCM, whether an impaired insulin signaling pathway could cause iron overload in cardiomyocytes has almost never been reported in the literature.
In 2016, Li et al. examined the myocardial iron content using the aromatic absorption spectrophotometry method in diabetic rats induced by high fat diet and streptozotocin (STZ) injection, and no alteration was found between the diabetic rats and control rats (37). In another study performed in type 2 diabetic mice by Wang et al., labile iron content was significantly increased in myocardial tissue (38). Furthermore, in another study in STZ-induced diabetic rats, Fe2+ content was found to be increased in the heart tissue, indicating iron overload existed in DCM (39). An in vitro study conducted by Li et al. (40), found increased Fe2+ content in H9C2 cells treated with high glucose. Therefore, iron overload was found in DCM and might be correlated with the pathogenesis of DCM (Table 1).
3 Ferroptosis and DCM
Iron overload may trigger ferroptosis, a unique form of non-apoptotic cell death, which is characterized by iron-dependent lipid peroxidation (41). Cells that undergo ferroptosis exhibit malformed mitochondria, a decreased crest, membrane concentration, rupture of the outer membrane, and an absence of features of apoptosis. The main cause of ferroptosis is the depletion of glutathione and impaired function of phospholipid peroxidase glutathione peroxidase 4 (GPX4) which can protect cell membranes from peroxidative damage (7, 42). Ferroptosis participates in many cardiomyopathies including doxorubicin-induced cardiomyopathy (29), myocardial infarction (43), and heart failure (44). In diabetes, increased advanced glycation end-products (38), lipid peroxidation, and oxidative stress (45) all participate in the pathogenesis of DCM, which are also the triggers for cellular iron overload and ferroptosis. The expression of ferroptosis-promoting genes was increased in the heart tissue from STZ-induced diabetic mice (46) and ferroptosis was found in T2DM mice with DCM (38). A ferroptosis-promoting gene profile was also found by Gawargi et al. (47) in the heart tissue of diabetic patients with heart failure. Furthermore, ferroptosis inhibition led to improved cardiac contract function in T2DM mice (38). Therefore, ferroptosis participates in DCM and could be an intervention target in DCM therapy.
Ferroptosis is a complicated process in which many regulators and pathways are involved (48, 49). The detailed mechanisms underlying ferroptosis in DCM have been investigated but are quite limited. Nuclear factor erythroid 2-related factor 2 (Nrf2) is an important transcriptional factor and participates in multiple biological processes including anti-oxidation (50). It has been found that the activation of Nrf2 may improve DCM (51–53) and this effect might be partly via anti-ferroptotic effects. Wang et al. found that sulforaphane can increase ferritin expression in myocardial tissue by activating Nrf2, which may inhibit advanced glycation end-product-induced ferroptosis in DCM (38). Nrf2/GPX4, Nrf2/HO-1 pathway activation could inhibit ferroptosis and thus alleviate high glucose-induced cardiomyocyte injury (54–56) (Figure 1). Recently, novel evidence has indicated that alterations in the intestinal microbiota (57) reduced cardiac expression of retinol dehydrogenase 10 (58) and upregulated lysine acetyltransferase 2 A (Kat2a) (59), both of which participate in ferroptosis in DCM and provide more therapy targets for DCM.
Mitochondria are not only the organelle that produces ROS but are also pivotal for iron metabolism and ferroptosis (60). Cardiomyocytes need a continuous energy supply to maintain their beating. Mitochondria, as the energy factories, are crucial for maintaining heart function. It has been suggested that mitochondrial dysfunction participates in the occurrence and the devolvement of DCM (61, 62). The role of mitochondria in iron overload and ferroptosis has been studied but is still complicated. Iron overload caused cardiac and mitochondria dysfunction in rats (63) and led to mitochondrial iron accumulation, an increase in mitochondrial ROS, and ferroptosis in cardiomyocytes (64). Data from an in vitro study indicated that oxidative stress, which was induced by a tert-butyl hydroperoxide treatment, induced mitochondrial iron overload and cardiomyocyte ferroptosis by targeting the Bach1-HO-1 pathway (65). Furthermore, in doxorubicin-induced cardiomyopathy, doxorubicin triggered iron accumulation in mitochondria, which further caused cardiomyocyte ferroptosis (29, 66). Therefore, mitochondrial iron overload is crucial for cardiomyocyte ferroptosis; however, detailed evidence of its role in DCM has never been reported.
The iron-sulfur cluster (ISC) is an ancient and conserved cofactor that is mainly assembled in mitochondria, and the loss of its synthesis leads to iron overload and ferroptosis. Frataxin is a mitochondrial ISC-related protein and an important regulator for ferroptosis (67). Patients with reduced frataxin expression have an increased risk of diabetes mellitus (68) and cardiomyopathy (69). Furthermore, decreased frataxin expression has been found to cause cell ferroptosis in adipose tissue (70) and heart tissue (71).
Mitochondrial ferritin (FtMt) is structurally similar to ferritin-heavy chains but has lower ferroxidase activity. FtMt overexpression can lead to intracellular iron redistribution by transferring iron from the cytoplasm into mitochondria, consequently leading to reduced iron content in the cytoplasm (72, 73). It has been found that FtMt could protect cells from oxidative stress by regulating the mitochondrial labile iron pool and ROS production (73). Mice with a FtMt deficiency are more sensitive to cardiomyocyte damage caused by doxorubicin (74) and fatigue (75), indicating that cardiomyocytes with FtMt deficiency are more prone to injury. FtMt overexpression could inhibit oxidative stress-induced ferroptosis through the inhibition of mitochondrial iron overload and ROS in cardiomyocytes (65). Wang et al. found that the overexpression of FtMt could ameliorate oxidative stress and ferroptosis in osteoblasts caused by high glucose (76). Unfortunately, thus far, there is no data available on the role of FtMt in DCM.
Mitophagy refers to the targeted phagocytosis and destruction of mitochondria by the cellular autophagy apparatus and is considered to be the main mechanism of mitochondrial quality control. Studies on DCM have suggested that decreased mitophagy may lead to the accumulation of abnormal mitochondria, and result in increased intracellular oxidative stress, which triggers the occurrence and development of DCM (77). Improving mitophagy can improve the risk of developing DCM (78). Studies conducted in non-cardiomyocytes have found that activating mitophagy may inhibit ferroptosis. Li et al. found that activating PINK1-Parkin-dependent mitophagy could protect cells from CISD3-induced ferroptosis (79). Therefore, whether reduced mitophagy may trigger ferroptosis in DCM is a promising research topic that needs to be studied.
4 Ferritinophagy and DCM
Ferritin is a cytosolic storage protein complex consisting of ferritin heavy-chain (FTH1) and light-chain (FTL) subunits, responsible for intracellular iron storage (80), and exerts antioxidant effects by isolating redox-active iron. Ferritin can affect cell susceptibility to ferroptosis (81). Ferritinophagy is a selective form of ferritin autophagy degradation whose overactivation induces increased degradation of ferritin which binds to iron, and increased iron release leads to iron overload, leading to cellular ferroptosis. Thus, ferritinophagy plays an important role in the regulation of ferroptosis by regulating intracellular iron balance (82).
Nuclear receptor coactivator 4 (NCOA4) is a selective ferritinophagy cargo receptor that directly recognizes and binds to FTH1 and transports ferritin to autophagosomes for lysosomal degradation and iron release (83). In non-cardiomyocytes, NCOA4 knockdown (84) or inhibition of the NCOA4-FTH1 association (85) was found to inhibit ferroptosis. Cardiac NCOA4 expression was significantly increased while GPX4 expression was decreased in diabetic rats (39) and activated NCOA4-mediated ferroptinophagy and ferroptosis were found in the heart tissue of db/db mice (86). NCOA4 knockdown or inhibition alleviated ferroptosis in a DCM model in vitro (87) and in vivo (86), suggesting that increased ferritinophagy plays an important role in the occurrence of DCM (Figure 1).
5 Hypoglycemic drugs, ferroptosis, and DCM
5.1 Metformin
As a classic hypoglycemic drug, metformin has been previously found to be protective in DCM by alleviating apoptosis (88), improving autophagy, inhibiting pyroptosis (89), and alleviating fibrosis (90). In doxorubicin-induced cardiotoxicity mouse models, metformin treatment inhibited ferroptosis and improved cardiac function by activating AMP-activated protein kinase (AMPK)α2 phosphorylation (13). In the study by Wu et al., metformin alleviated cardiac I/R damage in vivo and in vitro by relieving non-heme iron content and ferroptosis by activating AMPKα and inhibiting nicotinamide adenine dinucleotide phosphate oxidase 4 expression (91). However, thus far, there is no data available on whether metformin may alleviate ferroptosis in DCM.
5.2 Glucagon-like peptide-1 receptor agonists
Glucagon-like peptide-1 receptor agonists (GLP–1RAs) have attracted much attention in recent years for their cardiac protective effects. Studies have found that liraglutide can improve cardiac function in diabetic patients (92) and improve the endoplasmic reticulum stress of cardiomyocytes in diabetic animals (93). In studies of db/db diabetic mice, liraglutide has been found to reduce iron overload in the liver as well as the hippocampus, and reduce ferroptosis (94, 95). In a nationwide register-based study performed by Bain et al., GLP-1RA administration was found to be associated with lower circulating ferritin levels in patients with type 2 diabetes and hemochromatosis (96). Therefore, while alleviating ferroptosis might partly contribute to the cardio-protective effects of GLP-1RAs in DCM, more investigations are warranted.
5.3 Sodium-glucose co-transporter-2 inhibitors
The cardiovascular benefits of sodium-glucose co-transporter-2 (SGLT2) inhibitors have been increasingly documented in recent years. Evidence from in vivo and in vitro studies has indicated that SGLT2 inhibitors, such as empagliflozin and dapagliflozin, can improve DCM by attenuating oxidative stress (97, 98). Empagliflozin exhibited anti-ferroptotic effects in high glucose-treated muscle C2C12 cells by restoring the expression of GPX4 (99) and in diabetic kidney disease models by activating Nrf2 (100). Thus far, only canagliflozin has been found to inhibit ferroptosis in DCM by balancing cardiac iron homeostasis, promoting Xc-/glutathione(GSH)/GPX4 axis (101), and activating the AMPK pathway (102) (Figure 1).
5.4 Dipeptidyl peptidase 4 enzyme inhibitors
The protective effects of dipeptidyl peptidase 4 (DPP-4) inhibitors on the DCM have been emerging in research in recent years. For example, linagliptin improved cardiac function in diabetic mice by inhibiting the NF-κB signaling pathway and relieving the cardiac inflammatory response by targeting the NOD-, lrr-, and pyrin domain-containing protein 3/apoptosis-associated speck-like protein containing a caspase recruitment domain (Nlrp3/ASC) inflammasome (103, 104). Sitagliptin was found to attenuate DCM by attenuating myocardial apoptosis, inflammation, and nitroxidative stress by targeting the liver kinase B1/AMPK/Protein kinase B (LKB-1/AMPK/Akt) and Janus kinase/signal transducers and activators of transcription (JAK/STAT) pathways and promoting cardiomyocyte autophagy separately (105–107). Furthermore, alogliptin could improve mitochondrial function in DCM (108). However, the effect of DPP-4 inhibitors on iron metabolism or ferroptosis has been scarcely investigated. In brain tissue, vildagliptin has been found to reduce iron deposition and inhibit ferroptosis following intracerebral hemorrhage (109). The role of DPP-4 inhibitors on ferroptosis in DCM is an interesting subject that needs to be further explored.
5.5 Thiazolidinediones
Thiazolidinediones (TZDs), a class of peroxisome proliferator-activated receptor gamma (PPARγ) agonist, is the inhibitor of the ferroptosis marker ACSL4 (110), and has been found to prevent ferroptosis in many tissues and models including acute kidney injury (111), a ferroptosis mouse model (112), lung I/R injury (113), and renal fibrosis (114). However, in a diabetic rat model, TZD treatment was found to be detrimental as it caused cardiomyocyte ferroptosis and structural heart disorders (115).
Thus, in addition to their hypoglycemic effects, the effects of these drugs on ferroptosis in DCM need to be further explored (Table 2).
6 Plant extracts, DCM, and ferroptosis
6.1 Resveratrol
Resveratrol is a non-flavonoid polyphenol mainly found in a variety of fruits and vegetables, including peanuts, grapes, and berries. In recent years, much attention has been paid to the effects of resveratrol due to its antidiabetic and cardiovascular protective properties. Data have indicated that resveratrol, including its natural precursor polydatin, could alleviate DCM by improving mitochondrial function, alleviating oxidative stress, and inhibiting nuclear factor kappa B (NF-κB) activity (116, 117). Resveratrol has been found to inhibit cardiomyocyte ferroptosis in I/R models in vivo and in vitro by decreasing TfR1 while increasing GPX4 and FTH1 expressions, regulating of ubiquity specific peptidase 19 (USP19)-Beclin1 autophagy (118), and targeting the voltage-dependent anion channel 1/glutathione peroxidase 4 (VDAC1/GPX4) pathways (119). Whether its anti-ferroptotic effects exist in DCM needs to be further investigated.
6.2 Flavonoids
Flavonoids are natural plant polyphenolic phytochemicals and are widely found in fruits, nuts, vegetables, flowers, vegetables, and herbs. There is a large amount of evidence from in vitro and in vivo studies that indicates that flavonoids possess iron-chelating and antioxidant abilities (120). Flavonoids could improve DCM mainly through their anti-inflammatory and anti-oxidation effects (121). In recent years, much attention has been paid to the anti-ferroptotic effects of flavonoids (122–124) and studies have indicated that flavonoids could protect against ferroptosis-mediated tissue damage. Therefore, there are strong possibilities that flavonoids could achieve their DCM protective effects by alleviating ferroptosis. However, the existing research mainly focuses on liver and kidney injury, and the evidence for DCM is still lacking.
6.3 Sulforaphane
Sulforaphane is found in cruciferous vegetables and is a natural isothiocyanate compound. An activator of Nrf2, the literature has revealed the effects of sulforaphane on the amelioration of diabetic complications (125, 126) and cardiovascular disease (127). Studies performed in DCM models have shown that sulforaphane could improve cardiac function, cardiac hypertrophy, fibrosis, inflammation, and oxidative damage (38, 128–131). The anti-ferroptotic effects of sulforaphane have been found in diabetic livers (132), cardiac arrest and resuscitation (133), and myocardial I/R models (127). In DCM models, sulforaphane could inhibit cardiomyocyte ferroptosis by upregulating ferritin and SLC7A11 levels via AMPK-mediated Nrf2 activation (38), but more evidence on the effect of sulforaphane on DCM is still needed.
6.4 Curcumin
Curcumin is a polyphenolic compound extracted from the rhizomes of the turmeric plant and exhibits DCM protective effects through its antioxidant (134, 135) and anti-inflammatory (136) properties. Evidence has indicated its favorable effects on osteoarthritis (137), acute kidney injury (138), and cigarette smoke-caused lung epithelial injury (139) by alleviating ferroptosis. Zhang et al. (55) found that curcumin inhibited ferroptosis in cardiomyocytes by promoting the function of Nrf2 and increasing the expression of GPX4 and heme oxygenase-1 in DCM models.
6.5 Berberine
Berberine, an isoquinoline alkaloid isolated from the Chinese herb Coptis chinensis and other Berberis plants, has been found to alleviate DCM by preventing cardiac dysfunction and remodeling (140), being anti-fibrotic (141), interfering with lipidomic profiles (142), and inhibiting pyroptosis (143, 144). However, although a large amount of data has found that berberine could alleviate ferroptosis in many cells and models including islet beta cell loss in T1DM (145), a polycystic ovarian syndrome (PCOS) cell model (146), contrast-induced nephropathy (147), and bone loss induced by nonalcoholic fatty liver disease (148), no data are available on whether these anti-ferroptotic effects also participate in its DCM protective effects.
Therefore, the cardio-protective benefits in DCM of these plant extracts might be partly achieved through their anti-ferroptotic effects, but these still need further investigation (Table 3).
7 Ferroptosis inhibitors and DCM
Ferrostatin-1 (Fer-1) and liproxstatin-1 (Lip-1) are ferroptosis inhibitors and achieve their effects by suppressing lipid peroxidation (149). Fer-1 ameliorates cardiac injury caused by lipopolysaccharide (150), H2O2 (150, 151), isoproterenol (152), 5-fluorouracil (153), and doxorubicin (154). Lip-1 has been found to reduce cardiomyocyte ferroptosis induced by heat shock (155), 2,3,7,8-Tetrachlorodibenzo-p-dioxin (156), and I/R injury (138). Furthermore, Fer-1 was found to inhibit cardiomyocyte ferroptosis induced by palmitic acid (40, 102). Both Fer-1 (40) and Lip-1 (38) were found to be effective in inhibiting ferroptosis in DCM (Figure 1).
8 Conclusion and perspectives
As a severe complication of diabetes, the mechanisms underlying DCM’s pathogenesis and relative therapy strategies have drawn attention in recent years. Due to the unique high energy and high iron demand of heart tissue, both energy and iron dyshomeostasis have been found in DCM. Ferroptosis is novel cell death induced by iron overload and iron-dependent lipid peroxidation. In this review, we summarized the evidence on iron metabolism and ferroptosis in DCM, in particular the role of mitochondria in iron metabolism. However, detailed and in-depth evidence of the contribution of ferroptosis in DCM is still lacking. Insulin resistance plays key role in the pathogenesis of DCM; however, thus far, whether insulin resistance could trigger ferroptosis has never been explored in DCM. The causal relationship of mitochondria, the most important organelle for energy metabolism in cardiomyocytes, with iron metabolism and ferroptosis in DCM has been scarcely investigated. In vivo data on the effects of ferroptosis inhibitors on cardiac function is lacking, although they have been found to be protective in DCM models. In addition, some anti-diabetic drugs that are potentially cardio-protective in DCM might possess anti-ferroptotic effects, but this still needs to be confirmed by more direct research, both in vivo and in vitro. Clinical evidence for ferroptosis-related screening and therapy in DCM patients is also lacking. Therefore, the existing evidence on the role of ferroptosis in DCM is the tip of the iceberg, as more studies on the detailed mechanisms underlying the role of ferroptosis and regulation pathways in DCM are warranted. Targeting ferroptosis might provide more perspectives for DCM therapy but this still needs to be further explored.
Author contributions
MT: Writing – original draft, Writing – review & editing. XH: Writing – original draft, Data curation, Software. ML: Writing – original draft, Methodology. PL: Writing – original draft, Formal analysis. HM: Writing – review & editing, Formal Analysis, Project administration, Validation. XJ: Writing – review & editing, Formal analysis, Funding acquisition, Project administration, Validation. YL: Writing – review & editing, Writing – original draft, Funding acquisition, Project administration, Resources, Validation, Conceptualization, Data curation, Formal Analysis, Investigation, Methodology, Software, Supervision, Visualization. YZ: Writing – review & editing, Validation, Visualization.
Funding
The author(s) declare financial support was received for the research, authorship, and/or publication of this article. This work was supported by the Natural Science Foundation of Hebei Province (grant number H2020206478), the Government-funded Clinical Medicine Outstanding Talent Training Project (grant number ZF2025150), the Central Government Guides Local Science and Technology Development Project (grant number 246Z7711G), and Projects of Medical Science Research of Health Commission of Hebei Province, China (grant numbers 20210725, 20210513, 20210372 and 20170642).
Acknowledgments
The authors would like to thank Tianpeng Sun (Department of Physiology, Hebei Medical University, Shijiazhuang, China) for participating in the discussion on ferroptosis in cardiomyocytes.
Conflict of interest
The authors declare that the research was conducted in the absence of any commercial or financial relationships that could be construed as a potential conflict of interest.
Publisher’s note
All claims expressed in this article are solely those of the authors and do not necessarily represent those of their affiliated organizations, or those of the publisher, the editors and the reviewers. Any product that may be evaluated in this article, or claim that may be made by its manufacturer, is not guaranteed or endorsed by the publisher.
References
1. Sun H, Saeedi P, Karuranga S, Pinkepank M, Ogurtsova K, Duncan BB, et al. IDF Diabetes Atlas: Global, regional and country-level diabetes prevalence estimates for 2021 and projections for 2045. Diabetes Res Clin Pract. (2022) 183:109119. doi: 10.1016/j.diabres.2021.109119
2. Saeedi P, Karuranga S, Hammond L, Kaundal A, Malanda B, Prystupiuk M, et al. Cardiovascular diseases and risk factors knowledge and awareness in people with type 2 diabetes mellitus: a global evaluation. Diabetes Res Clin Pract. (2020) 165:108194. doi: 10.1016/j.diabres.2020.108194
3. Rubler S, Dlugash J, Yuceoglu YZ, Kumral T, Branwood AW, Grishman A. New type of cardiomyopathy associated with diabetic glomerulosclerosis. Am J Cardiol. (1972) 30:595–602. doi: 10.1016/0002-9149(72)90595-4
4. Jaquenod De Giusti C, Palomeque J, Mattiazzi A. Ca(2+) mishandling and mitochondrial dysfunction: a converging road to prediabetic and diabetic cardiomyopathy. Pflugers Arch. (2022) 474:33–61. doi: 10.1007/s00424-021-02650-y
5. Chen Y, Hua Y, Li X, Arslan IM, Zhang W, Meng G. Distinct types of cell death and the implication in diabetic cardiomyopathy. Front Pharmacol. (2020) 11:42. doi: 10.3389/fphar.2020.00042
6. Baba Y, Higa JK, Shimada BK, Horiuchi KM, Suhara T, Kobayashi M, et al. Protective effects of the mechanistic target of rapamycin against excess iron and ferroptosis in cardiomyocytes. Am J Physiol Heart Circ Physiol. (2018) 314:H659–h668. doi: 10.1152/ajpheart.00452.2017
7. Dixon SJ, Lemberg KM, Lamprecht MR, Skouta R, Zaitsev EM, Gleason CE, et al. Ferroptosis: an iron-dependent form of nonapoptotic cell death. Cell. (2012) 149:1060–72. doi: 10.1016/j.cell.2012.03.042
8. Liu F, Jiang LJ, Zhang YX, Xu ST, Liu SL, Ye JT, et al. Inhibition of miR-214-3p attenuates ferroptosis in myocardial infarction via regulating ME2. Biochem Biophys Res Commun. (2023) 661:64–74. doi: 10.1016/j.bbrc.2023.04.031
9. Jiang YQ, Yang XY, Duan DQ, Zhang YY, Li NS, Tang LJ, et al. Inhibition of MALT1 reduces ferroptosis in rat hearts following ischemia/reperfusion via enhancing the Nrf2/SLC7A11 pathway. Eur J Pharmacol. (2023) 950:175774. doi: 10.1016/j.ejphar.2023.175774
10. Zhang LL, Chen GH, Tang RJ, Xiong YY, Pan Q, Jiang WY, et al. Levosimendan reverses cardiac malfunction and cardiomyocyte ferroptosis during heart failure with preserved ejection fraction via connexin 43 signaling activation. Cardiovasc Drugs Ther. (2024) 38:705–18. doi: 10.1007/s10557-023-07441-4
11. Zhang X, Zheng C, Gao Z, Chen H, Li K, Wang L, et al. SLC7A11/xCT prevents cardiac hypertrophy by inhibiting ferroptosis. Cardiovasc Drugs Ther. (2022) 36:437–47. doi: 10.1007/s10557-021-07220-z
12. Zeng Y, Cao G, Lin L, Zhang Y, Luo X, Ma X, et al. Resveratrol Attenuates Sepsis-Induced Cardiomyopathy in Rats through Anti-Ferroptosis via the Sirt1/Nrf2 Pathway. J Invest Surg. (2023) 36:2157521. doi: 10.1080/08941939.2022.2157521
13. Liao HH, Ding W, Zhang N, Zhou ZY, Ling Z, Li WJ, et al. Activation of AMPKα2 attenuated doxorubicin-induced cardiotoxicity via inhibiting lipid peroxidation associated ferroptosis. Free Radic Biol Med. (2023) 205:275–90. doi: 10.1016/j.freeradbiomed.2023.06.004
14. Kumfu S, Chattipakorn SC, Chattipakorn N. Iron overload cardiomyopathy: Using the latest evidence to inform future applications. Exp Biol Med (Maywood). (2022) 247:574–83. doi: 10.1177/15353702221076397
15. Hu H, Chen Y, Jing L, Zhai C, Shen L. The link between ferroptosis and cardiovascular diseases: A novel target for treatment. Front Cardiovasc Med. (2021) 8:710963. doi: 10.3389/fcvm.2021.710963
16. Chen Y, Li X, Wang S, Miao R, Zhong J. Targeting iron metabolism and ferroptosis as novel therapeutic approaches in cardiovascular diseases. Nutrients. (2023) 15:591. doi: 10.3390/nu15030591
17. Sun H, Chen D, Xin W, Ren L, Li Q, Han X. Targeting ferroptosis as a promising therapeutic strategy to treat cardiomyopathy. Front Pharmacol. (2023) 14:1146651. doi: 10.3389/fphar.2023.1146651
18. Liu J, Li Q, Yang Y, Ma L. Iron metabolism and type 2 diabetes mellitus: A meta-analysis and systematic review. J Diabetes Investig. (2020) 11:946–55. doi: 10.1111/jdi.13216
19. He J, Li Z, Xia P, Shi A, FuChen X, Zhang J, et al. Ferroptosis and ferritinophagy in diabetes complications. Mol Metab. (2022) 60:101470. doi: 10.1016/j.molmet.2022.101470
20. Liu Q, Sun L, Tan Y, Wang G, Lin X, Cai L. Role of iron deficiency and overload in the pathogenesis of diabetes and diabetic complications. Curr Med Chem. (2009) 16:113–29. doi: 10.2174/092986709787002862
21. Hentze MW, Muckenthaler MU, Galy B, Camaschella C. Two to tango: regulation of Mammalian iron metabolism. Cell. (2010) 142:24–38. doi: 10.1016/j.cell.2010.06.028
22. Yanatori I, Kishi F. DMT1 and iron transport. Free Radic Biol Med. (2019) 133:55–63. doi: 10.1016/j.freeradbiomed.2018.07.020
23. Oudit GY, Sun H, Trivieri MG, Koch SE, Dawood F, Ackerley C, et al. L-type Ca2+ channels provide a major pathway for iron entry into cardiomyocytes in iron-overload cardiomyopathy. Nat Med. (2003) 9:1187–94. doi: 10.1038/nm920
24. Liuzzi JP, Aydemir F, Nam H, Knutson MD, Cousins RJ. Zip14 (Slc39a14) mediates non-transferrin-bound iron uptake into cells. Proc Natl Acad Sci U.S.A. (2006) 103:13612–7. doi: 10.1073/pnas.0606424103
25. Harrison AV, Lorenzo FR, McClain DA. Iron and the pathophysiology of diabetes. Annu Rev Physiol. (2023) 85:339–62. doi: 10.1146/annurev-physiol-022522-102832
26. Liu X, Hong X, Jiang S, Li R, Lv Q, Wang J, et al. Epidemiological and transcriptome data identify potential key genes involved in iron overload for type 2 diabetes. Diabetol Metab Syndr. (2023) 15:134. doi: 10.1186/s13098-023-01110-0
27. Zimiao C, Dongdong L, Shuoping C, Peng Z, Fan Z, Rujun C, et al. Correlations between iron status and body composition in patients with type 2 diabetes mellitus. Front Nutr. (2022) 9:911860. doi: 10.3389/fnut.2022.911860
28. Li D, Song C, Zhang J, Zhao X. ROS and iron homeostasis dependent ferroptosis play a vital role in 5-Fluorouracil induced cardiotoxicity in vitro and in vivo. Toxicology. (2022) 468:153113. doi: 10.1016/j.tox.2022.153113
29. Abe K, Ikeda M, Ide T, Tadokoro T, Miyamoto HD, Furusawa S, et al. Doxorubicin causes ferroptosis and cardiotoxicity by intercalating into mitochondrial DNA and disrupting Alas1-dependent heme synthesis. Sci Signal. (2022) 15:eabn8017. doi: 10.1126/scisignal.abn8017
30. Li JY, Liu SQ, Yao RQ, Tian YP, Yao YM. A novel insight into the fate of cardiomyocytes in ischemia-reperfusion injury: from iron metabolism to ferroptosis. Front Cell Dev Biol. (2021) 9:799499. doi: 10.3389/fcell.2021.799499
31. Sung HK, Song E, Jahng JWS, Pantopoulos K, Sweeney G. Iron induces insulin resistance in cardiomyocytes via regulation of oxidative stress. Sci Rep. (2019) 9:4668. doi: 10.1038/s41598-019-41111-6
32. Khamseekaew J, Kumfu S, Chattipakorn SC, Chattipakorn N. Effects of iron overload on cardiac calcium regulation: translational insights into mechanisms and management of a global epidemic. Can J Cardiol. (2016) 32:1009–16. doi: 10.1016/j.cjca.2015.10.012
33. Siri-Angkul N, Song Z, Fefelova N, Gwathmey JK, Chattipakorn SC, Qu Z, et al. Activation of TRPC (Transient receptor potential canonical) channel currents in iron overloaded cardiac myocytes. Circ Arrhythm Electrophysiol. (2021) 14:e009291. doi: 10.1161/CIRCEP.120.009291
34. Gammella E, Recalcati S, Cairo G. Dual role of ROS as signal and stress agents: iron tips the balance in favor of toxic effects. Oxid Med Cell Longev. (2016) 2016:8629024. doi: 10.1155/2016/8629024
35. He H, Qiao Y, Zhou Q, Wang Z, Chen X, Liu D, et al. Iron overload damages the endothelial mitochondria via the ROS/ADMA/DDAHII/eNOS/NO pathway. Oxid Med Cell Longev. (2019) 2019:2340392. doi: 10.1155/2019/2340392
36. Jayakumar D, S. Narasimhan KK, Periandavan K. Triad role of hepcidin, ferroportin, and Nrf2 in cardiac iron metabolism: From health to disease. J Trace Elem Med Biol. (2022) 69:126882. doi: 10.1016/j.jtemb.2021.126882
37. Li X, Li W, Gao Z, Li H. Association of cardiac injury with iron-increased oxidative and nitrative modifications of the SERCA2a isoform of sarcoplasmic reticulum Ca(2+)-ATPase in diabetic rats. Biochimie. (2016) 127:144–52. doi: 10.1016/j.biochi.2016.05.011
38. Wang X, Chen X, Zhou W, Men H, Bao T, Sun Y, et al. Ferroptosis is essential for diabetic cardiomyopathy and is prevented by sulforaphane via AMPK/NRF2 pathways. Acta Pharm Sin B. (2022) 12:708–22. doi: 10.1016/j.apsb.2021.10.005
39. Li W, Li W, Wang Y, Leng Y, Xia Z. Inhibition of DNMT-1 alleviates ferroptosis through NCOA4 mediated ferritinophagy during diabetes myocardial ischemia/reperfusion injury. Cell Death Discovery. (2021) 7:267. doi: 10.1038/s41420-021-00656-0
40. Li W, Li W, Leng Y, Xiong Y, Xia Z. Ferroptosis is involved in diabetes myocardial ischemia/reperfusion injury through endoplasmic reticulum stress. DNA Cell Biol. (2020) 39:210–25. doi: 10.1089/dna.2019.5097
41. Chen X, Yu C, Kang R, Tang D. Iron metabolism in ferroptosis. Front Cell Dev Biol. (2020) 8:590226. doi: 10.3389/fcell.2020.590226
42. Tang D, Kang R, Berghe TV, Vandenabeele P, Kroemer G. The molecular machinery of regulated cell death. Cell Res. (2019) 29:347–64. doi: 10.1038/s41422-019-0164-5
43. Liu K, Chen S, Lu R. Identification of important genes related to ferroptosis and hypoxia in acute myocardial infarction based on WGCNA. Bioengineered. (2021) 12:7950–63. doi: 10.1080/21655979.2021.1984004
44. Xiong Y, Liu X, Jiang L, Hao T, Wang Y, Li T. Inhibition of ferroptosis reverses heart failure with preserved ejection fraction in mice. J Transl Med. (2024) 22:199. doi: 10.1186/s12967-023-04734-y
45. Kaludercic N, Di Lisa F. Mitochondrial ROS formation in the pathogenesis of diabetic cardiomyopathy. Front Cardiovasc Med. (2020) 7:12. doi: 10.3389/fcvm.2020.00012
46. Chen L, Yin Z, Qin X, Zhu X, Chen X, Ding G, et al. CD74 ablation rescues type 2 diabetes mellitus-induced cardiac remodeling and contractile dysfunction through pyroptosis-evoked regulation of ferroptosis. Pharmacol Res. (2022) 176:106086. doi: 10.1016/j.phrs.2022.106086
47. Gawargi FI, Mishra PK. Regulation of cardiac ferroptosis in diabetic human heart failure: uncovering molecular pathways and key targets. Cell Death Discovery. (2024) 10:268. doi: 10.1038/s41420-024-02044-w
48. Jiang X, Stockwell BR, Conrad M. Ferroptosis: mechanisms, biology and role in disease. Nat Rev Mol Cell Biol. (2021) 22:266–82. doi: 10.1038/s41580-020-00324-8
49. Liu J, Kang R, Tang D. Signaling pathways and defense mechanisms of ferroptosis. FEBS J. (2022) 289:7038–50. doi: 10.1111/febs.v289.22
50. Chen QM, Maltagliati AJ. Nrf2 at the heart of oxidative stress and cardiac protection. Physiol Genomics. (2018) 50:77–97. doi: 10.1152/physiolgenomics.00041.2017
51. Ge ZD, Lian Q, Mao X, Xia Z. Current status and challenges of NRF2 as a potential therapeutic target for diabetic cardiomyopathy. Int Heart J. (2019) 60:512–20. doi: 10.1536/ihj.18-476
52. He X, Kan H, Cai L, Ma Q. Nrf2 is critical in defense against high glucose-induced oxidative damage in cardiomyocytes. J Mol Cell Cardiol. (2009) 46:47–58. doi: 10.1016/j.yjmcc.2008.10.007
53. Li B, Liu S, Miao L, Cai L. Prevention of diabetic complications by activation of Nrf2: diabetic cardiomyopathy and nephropathy. Exp Diabetes Res. (2012) 2012:216512. doi: 10.1155/2012/216512
54. Li F, Hu Z, Huang Y, Zhan H. Dexmedetomidine ameliorates diabetic cardiomyopathy by inhibiting ferroptosis through the Nrf2/GPX4 pathway. J Cardiothorac Surg. (2023) 18:223. doi: 10.1186/s13019-023-02300-7
55. Wei Z, Shaohuan Q, Pinfang K, Chao S. Curcumin attenuates ferroptosis-induced myocardial injury in diabetic cardiomyopathy through the nrf2 pathway. Cardiovasc Ther. (2022) 2022:3159717. doi: 10.1155/2022/3159717
56. Wu S, Zhu J, Wu G, Hu Z, Ying P, Bao Z, et al. 6-gingerol alleviates ferroptosis and inflammation of diabetic cardiomyopathy via the nrf2/HO-1 pathway. Oxid Med Cell Longev. (2022) 2022:3027514. doi: 10.1155/2022/3027514
57. Wu H, Zhang P, Zhou J, Hu S, Hao J, Zhong Z, et al. Paeoniflorin confers ferroptosis resistance by regulating the gut microbiota and its metabolites in diabetic cardiomyopathy. Am J Physiol Cell Physiol. (2024) 326:C724–41. doi: 10.1152/ajpcell.00565.2023
58. Wu Y, Huang T, Li X, Shen C, Ren H, Wang H, et al. Retinol dehydrogenase 10 reduction mediated retinol metabolism disorder promotes diabetic cardiomyopathy in male mice. Nat Commun. (2023) 14:1181. doi: 10.1038/s41467-023-36837-x
59. Zhen J, Sheng X, Chen T, Yu H. Histone acetyltransferase Kat2a regulates ferroptosis via enhancing Tfrc and Hmox1 expression in diabetic cardiomyopathy. Cell Death Dis. (2024) 15:406. doi: 10.1038/s41419-024-06771-x
60. Fu C, Cao N, Zeng S, Zhu W, Fu X, Liu W, et al. Role of mitochondria in the regulation of ferroptosis and disease. Front Med (Lausanne). (2023) 10:1301822. doi: 10.3389/fmed.2023.1301822
61. Aon MA, Foster DB. Diabetic cardiomyopathy and the role of mitochondrial dysfunction: novel insights, mechanisms, and therapeutic strategies. Antioxid Redox Signal. (2015) 22:1499–501. doi: 10.1089/ars.2015.6349
62. Wang S, Chen Y, Li X, Zhang W, Liu Z, Wu M, et al. Emerging role of transcription factor EB in mitochondrial quality control. BioMed Pharmacother. (2020) 128:110272. doi: 10.1016/j.biopha.2020.110272
63. Kumfu S, Sripetchwandee J, Thonusin C, Sumneang N, Maneechote C, Arunsak B, et al. Ferroptosis inhibitor improves cardiac function more effectively than inhibitors of apoptosis and necroptosis through cardiac mitochondrial protection in rats with iron-overloaded cardiomyopathy. Toxicol Appl Pharmacol. (2023) 479:116727. doi: 10.1016/j.taap.2023.116727
64. Tam E, Sung HK, Lam NH, You S, Cho S, Ahmed SM, et al. Role of mitochondrial iron overload in mediating cell death in H9c2 cells. Cells. (2022) 12:118. doi: 10.3390/cells12010118
65. Chen Y, Guo X, Zeng Y, Mo X, Hong S, He H, et al. Oxidative stress induces mitochondrial iron overload and ferroptotic cell death. Sci Rep. (2023) 13:15515. doi: 10.1038/s41598-023-42760-4
66. Sumneang N, Siri-Angkul N, Kumfu S, Chattipakorn SC, Chattipakorn N. The effects of iron overload on mitochondrial function, mitochondrial dynamics, and ferroptosis in cardiomyocytes. Arch Biochem Biophys. (2020) 680:108241. doi: 10.1016/j.abb.2019.108241
67. Du J, Zhou Y, Li Y, Xia J, Chen Y, Chen S, et al. Identification of Frataxin as a regulator of ferroptosis. Redox Biol. (2020) 32:101483. doi: 10.1016/j.redox.2020.101483
68. Tamaroff J, DeDio A, Wade K, Wells M, Park C, Leavens K, et al. Friedreich’s Ataxia related Diabetes: Epidemiology and management practices. Diabetes Res Clin Pract. (2022) 186:109828. doi: 10.1016/j.diabres.2022.109828
69. Legrand L, Weinsaft JW, Pousset F, Ewenczyk C, Charles P, Hatem S, et al. Characterizing cardiac phenotype in Friedreich’s ataxia: The CARFA study. Arch Cardiovasc Dis. (2022) 115:17–28. doi: 10.1016/j.acvd.2021.10.010
70. Turchi R, Tortolici F, Guidobaldi G, Iacovelli F, Falconi M, Rufini S, et al. Frataxin deficiency induces lipid accumulation and affects thermogenesis in brown adipose tissue. Cell Death Dis. (2020) 11:51. doi: 10.1038/s41419-020-2253-2
71. La Rosa P, Petrillo S, Turchi R, Berardinelli F, Schirinzi T, Vasco G, et al. The Nrf2 induction prevents ferroptosis in Friedreich’s Ataxia. Redox Biol. (2021) 38:101791. doi: 10.1016/j.redox.2020.101791
72. Drysdale J, Arosio P, Invernizzi R, Cazzola M, Volz A, Corsi B, et al. Mitochondrial ferritin: a new player in iron metabolism. Blood Cells Mol Dis. (2002) 29:376–83. doi: 10.1006/bcmd.2002.0577
73. Levi S, Ripamonti M, Dardi M, Cozzi A, Santambrogio P. Mitochondrial ferritin: its role in physiological and pathological conditions. Cells. (2021) 10:1969. doi: 10.3390/cells10081969
74. Maccarinelli F, Gammella E, Asperti M, Regoni M, Biasiotto G, Turco E, et al. Mice lacking mitochondrial ferritin are more sensitive to doxorubicin-mediated cardiotoxicity. J Mol Med (Berl). (2014) 92:859–69. doi: 10.1007/s00109-014-1147-0
75. Wu W, Chang S, Wu Q, Xu Z, Wang P, Li Y, et al. Mitochondrial ferritin protects the murine myocardium from acute exhaustive exercise injury. Cell Death Dis. (2016) 7:e2475. doi: 10.1038/cddis.2016.372
76. Wang X, Ma H, Sun J, Zheng T, Zhao P, Li H, et al. Mitochondrial ferritin deficiency promotes osteoblastic ferroptosis via mitophagy in type 2 diabetic osteoporosis. Biol Trace Elem Res. (2022) 200:298–307. doi: 10.1007/s12011-021-02627-z
77. Yu LM, Dong X, Xue XD, Xu S, Zhang X, Xu YL, et al. Melatonin attenuates diabetic cardiomyopathy and reduces myocardial vulnerability to ischemia-reperfusion injury by improving mitochondrial quality control: Role of SIRT6. J Pineal Res. (2021) 70:e12698. doi: 10.1111/jpi.12698
78. Tong M, Saito T, Zhai P, Oka SI, Mizushima W, Nakamura M, et al. Mitophagy is essential for maintaining cardiac function during high fat diet-induced diabetic cardiomyopathy. Circ Res. (2019) 124:1360–71. doi: 10.1161/CIRCRESAHA.118.314607
79. Li Y, Wang X, Huang Z, Zhou Y, Xia J, Hu W, et al. CISD3 inhibition drives cystine-deprivation induced ferroptosis. Cell Death Dis. (2021) 12:839. doi: 10.1038/s41419-021-04128-2
80. Honarmand Ebrahimi K, Hagedoorn PL, Hagen WR. Unity in the biochemistry of the iron-storage proteins ferritin and bacterioferritin. Chem Rev. (2015) 115:295–326. doi: 10.1021/cr5004908
81. Masaldan S, Bush AI, Devos D, Rolland AS, Moreau C. Striking while the iron is hot: Iron metabolism and ferroptosis in neurodegeneration. Free Radic Biol Med. (2019) 133:221–33. doi: 10.1016/j.freeradbiomed.2018.09.033
82. Ajoolabady A, Aslkhodapasandhokmabad H, Libby P, Tuomilehto J, Lip GYH, Penninger JM, et al. Ferritinophagy and ferroptosis in the management of metabolic diseases. Trends Endocrinol Metab. (2021) 32:444–62. doi: 10.1016/j.tem.2021.04.010
83. Santana-Codina N, Mancias JD. The role of NCOA4-mediated ferritinophagy in health and disease. Pharm (Basel). (2018) 11:114. doi: 10.3390/ph11040114
84. Hou W, Xie Y, Song X, Sun X, Lotze MT, Zeh HJ 3rd, et al. Autophagy promotes ferroptosis by degradation of ferritin. Autophagy. (2016) 12:1425–8. doi: 10.1080/15548627.2016.1187366
85. Fang Y, Chen X, Tan Q, Zhou H, Xu J, Gu Q. Inhibiting ferroptosis through disrupting the NCOA4-FTH1 interaction: A new mechanism of action. ACS Cent Sci. (2021) 7:980–9. doi: 10.1021/acscentsci.0c01592
86. Sun J, Xu J, Liu Y, Lin Y, Wang F, Han Y, et al. Exogenous spermidine alleviates diabetic cardiomyopathy via suppressing reactive oxygen species, endoplasmic reticulum stress, and Pannexin-1-mediated ferroptosis. Biomol BioMed. (2023) 23:825–37. doi: 10.17305/bb.2022.8846
87. Zhang X, Dong X, Jie H, Li S, Li H, Su Y, et al. Downregulation of the (pro)renin receptor alleviates ferroptosis-associated cardiac pathological changes via the NCOA 4-mediated ferritinophagy pathway in diabetic cardiomyopathy. Int Immunopharmacol. (2024) 138:112605. doi: 10.1016/j.intimp.2024.112605
88. Yang Z, Wang M, Zhang Y, Cai F, Jiang B, Zha W, et al. Metformin ameliorates diabetic cardiomyopathy by activating the PK2/PKR pathway. Front Physiol. (2020) 11:425. doi: 10.3389/fphys.2020.00425
89. Yang F, Qin Y, Wang Y, Meng S, Xian H, Che H, et al. Metformin inhibits the NLRP3 inflammasome via AMPK/mTOR-dependent effects in diabetic cardiomyopathy. Int J Biol Sci. (2019) 15:1010–9. doi: 10.7150/ijbs.29680
90. Dawood AF, Alzamil NM, Hewett PW, Momenah MA, Dallak M, Kamar SS, et al. Metformin Protects against Diabetic Cardiomyopathy: An Association between Desmin-Sarcomere Injury and the iNOS/mTOR/TIMP-1 Fibrosis Axis. Biomedicines. (2022) 10:984. doi: 10.3390/biomedicines10050984
91. Wu Z, Bai Y, Qi Y, Chang C, Jiao Y, Bai Y, et al. Metformin ameliorates ferroptosis in cardiac ischemia and reperfusion by reducing NOX4 expression via promoting AMPKα. Pharm Biol. (2023) 61:886–96. doi: 10.1080/13880209.2023.2212700
92. Bizino MB, Jazet IM, Westenberg JJM, van Eyk HJ, Paiman EHM, Smit JWA, et al. Effect of liraglutide on cardiac function in patients with type 2 diabetes mellitus: randomized placebo-controlled trial. Cardiovasc Diabetol. (2019) 18:55. doi: 10.1186/s12933-019-0857-6
93. Ji Y, Zhao Z, Cai T, Yang P, Cheng M. Liraglutide alleviates diabetic cardiomyopathy by blocking CHOP-triggered apoptosis via the inhibition of the IRE-α pathway. Mol Med Rep. (2014) 9:1254–8. doi: 10.3892/mmr.2014.1956
94. An JR, Su JN, Sun GY, Wang QF, Fan YD, Jiang N, et al. Liraglutide alleviates cognitive deficit in db/db mice: involvement in oxidative stress, iron overload, and ferroptosis. Neurochem Res. (2022) 47:279–94. doi: 10.1007/s11064-021-03442-7
95. Song JX, An JR, Chen Q, Yang XY, Jia CL, Xu S, et al. Liraglutide attenuates hepatic iron levels and ferroptosis in db/db mice. Bioengineered. (2022) 13:8334–48. doi: 10.1080/21655979.2022.2051858
96. Bain SC, Carstensen B, Hyveled L, Seremetis S, Flindt Kreiner F, Amadid H, et al. Glucagon-like peptide-1 receptor agonist use is associated with lower blood ferritin levels in people with type 2 diabetes and hemochromatosis: a nationwide register-based study. BMJ Open Diabetes Res Care. (2023) 11:e003300. doi: 10.1136/bmjdrc-2022-003300
97. Li C, Zhang J, Xue M, Li X, Han F, Liu X, et al. SGLT2 inhibition with empagliflozin attenuates myocardial oxidative stress and fibrosis in diabetic mice heart. Cardiovasc Diabetol. (2019) 18:15. doi: 10.1186/s12933-019-0816-2
98. Xing YJ, Liu BH, Wan SJ, Cheng Y, Zhou SM, Sun Y, et al. A SGLT2 Inhibitor Dapagliflozin Alleviates Diabetic Cardiomyopathy by Suppressing High Glucose-Induced Oxidative Stress in vivo and in vitro. Front Pharmacol. (2021) 12:708177. doi: 10.3389/fphar.2021.708177
99. Han JX, Luo LL, Wang YC, Miyagishi M, Kasim V, Wu SR. SGLT2 inhibitor empagliflozin promotes revascularization in diabetic mouse hindlimb ischemia by inhibiting ferroptosis. Acta Pharmacol Sin. (2023) 44:1161–74. doi: 10.1038/s41401-022-01031-0
100. Lu Q, Yang L, Xiao JJ, Liu Q, Ni L, Hu JW, et al. Empagliflozin attenuates the renal tubular ferroptosis in diabetic kidney disease through AMPK/NRF2 pathway. Free Radic Biol Med. (2023) 195:89–102. doi: 10.1016/j.freeradbiomed.2022.12.088
101. Du S, Shi H, Xiong L, Wang P, Shi Y. Canagliflozin mitigates ferroptosis and improves myocardial oxidative stress in mice with diabetic cardiomyopathy. Front Endocrinol (Lausanne). (2022) 13:1011669. doi: 10.3389/fendo.2022.1011669
102. Zhang W, Lu J, Wang Y, Sun P, Gao T, Xu N, et al. Canagliflozin attenuates lipotoxicity in cardiomyocytes by inhibiting inflammation and ferroptosis through activating AMPK pathway. Int J Mol Sci. (2023) 24:858. doi: 10.3390/ijms24010858
103. Al Zoubi S, Chen J, Murphy C, Martin L, Chiazza F, Collotta D, et al. Linagliptin attenuates the cardiac dysfunction associated with experimental sepsis in mice with pre-existing type 2 diabetes by inhibiting NF-κB. Front Immunol. (2018) 9:2996. doi: 10.3389/fimmu.2018.02996
104. Birnbaum Y, Tran D, Bajaj M, Ye Y. DPP-4 inhibition by linagliptin prevents cardiac dysfunction and inflammation by targeting the Nlrp3/ASC inflammasome. Basic Res Cardiol. (2019) 114:35. doi: 10.1007/s00395-019-0743-0
105. Al-Damry NT, Attia HA, Al-Rasheed NM, Al-Rasheed NM, Mohamad RA, Al-Amin MA, et al. Sitagliptin attenuates myocardial apoptosis via activating LKB-1/AMPK/Akt pathway and suppressing the activity of GSK-3β and p38α/MAPK in a rat model of diabetic cardiomyopathy. BioMed Pharmacother. (2018) 107:347–58. doi: 10.1016/j.biopha.2018.07.126
106. Al-Rasheed NM, Al-Rasheed NM, Hasan IH, Al-Amin MA, Al-Ajmi HN, Mahmoud AM. Sitagliptin attenuates cardiomyopathy by modulating the JAK/STAT signaling pathway in experimental diabetic rats. Drug Des Devel Ther. (2016) 10:2095–107. doi: 10.2147/DDDT.S109287
107. Zhou Y, Wang H, Man F, Guo Z, Xu J, Yan W, et al. Sitagliptin protects cardiac function by reducing nitroxidative stress and promoting autophagy in zucker diabetic fatty (ZDF) rats. Cardiovasc Drugs Ther. (2018) 32:541–52. doi: 10.1007/s10557-018-6831-9
108. Zhang X, Zhang Z, Yang Y, Suo Y, Liu R, Qiu J, et al. Alogliptin prevents diastolic dysfunction and preserves left ventricular mitochondrial function in diabetic rabbits. Cardiovasc Diabetol. (2018) 17:160. doi: 10.1186/s12933-018-0803-z
109. Zhang Y, Zhang X, Wee Yong V, Xue M. Vildagliptin improves neurological function by inhibiting apoptosis and ferroptosis following intracerebral hemorrhage in mice. Neurosci Lett. (2022) 776:136579. doi: 10.1016/j.neulet.2022.136579
110. Kim JH, Lewin TM, Coleman RA. Expression and characterization of recombinant rat Acyl-CoA synthetases 1, 4, and 5. Selective inhibition by triacsin C and thiazolidinediones. J Biol Chem. (2001) 276:24667–73. doi: 10.1074/jbc.M010793200
111. Wang Y, Zhang M, Bi R, Su Y, Quan F, Lin Y, et al. ACSL4 deficiency confers protection against ferroptosis-mediated acute kidney injury. Redox Biol. (2022) 51:102262. doi: 10.1016/j.redox.2022.102262
112. Doll S, Proneth B, Tyurina YY, Panzilius E, Kobayashi S, Ingold I, et al. ACSL4 dictates ferroptosis sensitivity by shaping cellular lipid composition. Nat Chem Biol. (2017) 13:91–8. doi: 10.1038/nchembio.2239
113. Xu Y, Li X, Cheng Y, Yang M, Wang R. Inhibition of ACSL4 attenuates ferroptotic damage after pulmonary ischemia-reperfusion. FASEB J. (2020) 34:16262–75. doi: 10.1096/fj.202001758R
114. Dai Y, Chen Y, Mo D, Jin R, Huang Y, Zhang L, et al. Inhibition of ACSL4 ameliorates tubular ferroptotic cell death and protects against fibrotic kidney disease. Commun Biol. (2023) 6:907. doi: 10.1038/s42003-023-05272-5
115. Iqbal S, Jabeen F, Kahwa I, Omara T. Suberosin alleviates thiazolidinedione-induced cardiomyopathy in diabetic rats by inhibiting ferroptosis via modulation of ACSL4-LPCAT3 and PI3K-AKT signaling pathways. Cardiovasc Toxicol. (2023) 23:295–304. doi: 10.1007/s12012-023-09804-7
116. Fang WJ, Wang CJ, He Y, Zhou YL, Peng XD, Liu SK. Resveratrol alleviates diabetic cardiomyopathy in rats by improving mitochondrial function through PGC-1α deacetylation. Acta Pharmacol Sin. (2018) 39:59–73. doi: 10.1038/aps.2017.50
117. Tan YY, Chen LX, Fang L, Zhang Q. Cardioprotective effects of polydatin against myocardial injury in diabetic rats via inhibition of NADPH oxidase and NF-κB activities. BMC Complement Med Ther. (2020) 20:378. doi: 10.1186/s12906-020-03177-y
118. Li T, Tan Y, Ouyang S, He J, Liu L. Resveratrol protects against myocardial ischemia-reperfusion injury via attenuating ferroptosis. Gene. (2022) 808:145968. doi: 10.1016/j.gene.2021.145968
119. Hu T, Zou HX, Zhang ZY, Wang YC, Hu FJ, Huang WX, et al. Resveratrol protects cardiomyocytes against ischemia/reperfusion-induced ferroptosis via inhibition of the VDAC1/GPX4 pathway. Eur J Pharmacol. (2024) 971:176524. doi: 10.1016/j.ejphar.2024.176524
120. Wang X, Li Y, Han L, Li J, Liu C, Sun C. Role of flavonoids in the treatment of iron overload. Front Cell Dev Biol. (2021) 9:685364. doi: 10.3389/fcell.2021.685364
121. Jubaidi FF, Zainalabidin S, Taib IS, Hamid ZA, Budin SB. The potential role of flavonoids in ameliorating diabetic cardiomyopathy via alleviation of cardiac oxidative stress, inflammation and apoptosis. Int J Mol Sci. (2021) 22:5094. doi: 10.3390/ijms22105094
122. Guan F, Du H, Li J, Ren H, Dong A. Quercetin alleviates LPS-stimulated myocardial injury through regulating ALOX5/PI3K/AKT pathway in sepsis. Cardiovasc Toxicol. (2024) 24:1116–24. doi: 10.1007/s12012-024-09901-1
123. Wang IC, Lin JH, Lee WS, Liu CH, Lin TY, Yang KT. Baicalein and luteolin inhibit ischemia/reperfusion-induced ferroptosis in rat cardiomyocytes. Int J Cardiol. (2023) 375:74–86. doi: 10.1016/j.ijcard.2022.12.018
124. Yang M, Xia L, Song J, Hu H, Zang N, Yang J, et al. Puerarin ameliorates metabolic dysfunction-associated fatty liver disease by inhibiting ferroptosis and inflammation. Lipids Health Dis. (2023) 22:202. doi: 10.1186/s12944-023-01969-y
125. Wang Y, Xu Y, Wang Q, Guo F, Song Y, Fan X, et al. Sulforaphane ameliorated podocyte injury according to regulation of the Nrf2/PINK1 pathway for mitophagy in diabetic kidney disease. Eur J Pharmacol. (2023) 958:176042. doi: 10.1016/j.ejphar.2023.176042
126. Li S, Yang H, Chen X. Protective effects of sulforaphane on diabetic retinopathy: activation of the Nrf2 pathway and inhibition of NLRP3 inflammasome formation. Exp Anim. (2019) 68:221–31. doi: 10.1538/expanim.18-0146
127. Tian H, Xiong Y, Zhang Y, Leng Y, Tao J, Li L, et al. Activation of NRF2/FPN1 pathway attenuates myocardial ischemia-reperfusion injury in diabetic rats by regulating iron homeostasis and ferroptosis. Cell Stress Chaperones. (2021) 27:149–64. doi: 10.1007/s12192-022-01257-15
128. Wang J, Wang S, Wang W, Chen J, Zhang Z, Zheng Q, et al. Protection against diabetic cardiomyopathy is achieved using a combination of sulforaphane and zinc in type 1 diabetic OVE26 mice. J Cell Mol Med. (2019) 23:6319–30. doi: 10.1111/jcmm.14520
129. Bai Y, Cui W, Xin Y, Miao X, Barati MT, Zhang C, et al. Prevention by sulforaphane of diabetic cardiomyopathy is associated with up-regulation of Nrf2 expression and transcription activation. J Mol Cell Cardiol. (2013) 57:82–95. doi: 10.1016/j.yjmcc.2013.01.008
130. Sun Y, Zhou S, Guo H, Zhang J, Ma T, Zheng Y, et al. Protective effects of sulforaphane on type 2 diabetes-induced cardiomyopathy via AMPK-mediated activation of lipid metabolic pathways and NRF2 function. Metabolism. (2020) 102:154002. doi: 10.1016/j.metabol.2019.154002
131. Gu J, Cheng Y, Wu H, Kong L, Wang S, Xu Z, et al. Metallothionein is downstream of nrf2 and partially mediates sulforaphane prevention of diabetic cardiomyopathy. Diabetes. (2017) 66:529–42. doi: 10.2337/db15-1274
132. Savic N, Markelic M, Stancic A, Velickovic K, Grigorov I, Vucetic M, et al. Sulforaphane prevents diabetes-induced hepatic ferroptosis by activating Nrf2 signaling axis. Biofactors. (2024) 50:810–27. doi: 10.1002/biof.v50.4
133. Zheng Z, Xu J, Mao Y, Mei Z, Zhu J, Lan P, et al. Sulforaphane improves post-resuscitation myocardial dysfunction by inhibiting cardiomyocytes ferroptosis via the Nrf2/IRF1/GPX4 pathway. BioMed Pharmacother. (2024) 179:117408. doi: 10.1016/j.biopha.2024.117408
134. Ren BC, Zhang YF, Liu SS, Cheng XJ, Yang X, Cui XG, et al. Curcumin alleviates oxidative stress and inhibits apoptosis in diabetic cardiomyopathy via Sirt1-Foxo1 and PI3K-Akt signalling pathways. J Cell Mol Med. (2020) 24:12355–67. doi: 10.1111/jcmm.v24.21
135. Wu X, Zhou X, Lai S, Liu J, Qi J. Curcumin activates Nrf2/HO-1 signaling to relieve diabetic cardiomyopathy injury by reducing ROS in vitro and in vivo. FASEB J. (2022) 36:e22505. doi: 10.1096/fj.202200543RRR
136. Wang M, Jin L, Zhang Q, Zhu W, He H, Lou S, et al. Curcumin analog JM-2 alleviates diabetic cardiomyopathy inflammation and remodeling by inhibiting the NF-κB pathway. BioMed Pharmacother. (2022) 154:113590. doi: 10.1016/j.biopha.2022.113590
137. Sheng W, Li A, Yue Y, Wang Q, Yu F, Weng J, et al. A novel curcumin-loaded nanoplatform alleviates osteoarthritis by inhibiting chondrocyte ferroptosis. Macromol Rapid Commun. (2024):e2400495. doi: 10.1002/marc.202400495
138. Feng Y, Madungwe NB, Imam Aliagan AD, Tombo N, Bopassa JC. Liproxstatin-1 protects the mouse myocardium against ischemia/reperfusion injury by decreasing VDAC1 levels and restoring GPX4 levels. Biochem Biophys Res Commun. (2019) 520:606–11. doi: 10.1016/j.bbrc.2019.10.006
139. Tang X, Li Z, Yu Z, Li J, Zhang J, Wan N, et al. Effect of curcumin on lung epithelial injury and ferroptosis induced by cigarette smoke. Hum Exp Toxicol. (2021) 40:S753–62. doi: 10.1177/09603271211059497
140. Chang W, Zhang M, Meng Z, Yu Y, Yao F, Hatch GM, et al. Berberine treatment prevents cardiac dysfunction and remodeling through activation of 5’-adenosine monophosphate-activated protein kinase in type 2 diabetic rats and in palmitate-induced hypertrophic H9c2 cells. Eur J Pharmacol. (2015) 769:55–63. doi: 10.1016/j.ejphar.2015.10.043
141. Li G, Xing W, Zhang M, Geng F, Yang H, Zhang H, et al. Antifibrotic cardioprotection of berberine via downregulating myocardial IGF-1 receptor-regulated MMP-2/MMP-9 expression in diabetic rats. Am J Physiol Heart Circ Physiol. (2018) 315:H802–h813. doi: 10.1152/ajpheart.00093.2018
142. Dong S, Zhang S, Chen Z, Zhang R, Tian L, Cheng L, et al. Berberine could ameliorate cardiac dysfunction via interfering myocardial lipidomic profiles in the rat model of diabetic cardiomyopathy. Front Physiol. (2018) 9:1042. doi: 10.3389/fphys.2018.01042
143. Zhong C, Xie Y, Wang H, Chen W, Yang Z, Zhang L, et al. Berberine inhibits NLRP3 inflammasome activation by regulating mTOR/mtROS axis to alleviate diabetic cardiomyopathy. Eur J Pharmacol. (2024) 964:176253. doi: 10.1016/j.ejphar.2023.176253
144. Yang L, Cheng CF, Li ZF, Huang XJ, Cai SQ, Ye SY, et al. Berberine blocks inflammasome activation and alleviates diabetic cardiomyopathy via the miR−18a−3p/Gsdmd pathway. Int J Mol Med. (2023) 51:49. doi: 10.3892/ijmm.2023.5252
145. Bao L, Jin Y, Han J, Wang W, Qian L, Wu W. Berberine regulates GPX4 to inhibit ferroptosis of islet beta cells. Planta Med. (2023) 89:254–61. doi: 10.1055/a-1939-7417
146. Wang S, Wang Y, Qin Q, Li J, Chen Q, Zhang Y, et al. Berberine protects against dihydrotestosterone-induced human ovarian granulosa cell injury and ferroptosis by regulating the circ_0097636/miR-186-5p/SIRT3 pathway. Appl Biochem Biotechnol. (2024) 196:5265–82. doi: 10.1007/s12010-023-04825-y
147. Wang W, Yu R, Wu C, Li Q, Chen J, Xiao Y, et al. Berberine alleviates contrast-induced nephropathy by activating Akt/Foxo3a/Nrf2 signalling pathway. J Cell Mol Med. (2024) 28:e18016. doi: 10.1111/jcmm.18016
148. Gu S, Wang J, Yu S, Zhang S, Gao T, Yan D, et al. Berberine ameliorates nonalcoholic fatty liver disease-induced bone loss by inhibiting ferroptosis. Bone. (2024) 185:117114. doi: 10.1016/j.bone.2024.117114
149. Fan BY, Pang YL, Li WX, Zhao CX, Zhang Y, Wang X, et al. Liproxstatin-1 is an effective inhibitor of oligodendrocyte ferroptosis induced by inhibition of glutathione peroxidase 4. Neural Regener Res. (2021) 16:561–6. doi: 10.4103/1673-5374.293157
150. Xiao Z, Kong B, Fang J, Qin T, Dai C, Shuai W, et al. Ferrostatin-1 alleviates lipopolysaccharide-induced cardiac dysfunction. Bioengineered. (2021) 12:9367–76. doi: 10.1080/21655979.2021.2001913
151. Sun C, Peng F, Li J, Cui X, Qiao X, Zhu W. Ferroptosis-specific inhibitor ferrostatin-1 relieves H(2)O(2)-induced redox imbalance in primary cardiomyocytes through the nrf2/ARE pathway. Dis Markers. (2022) 2022:4539932. doi: 10.1155/2022/4539932
152. Chen Y, Guo X, Zeng Y, Mo X, Hong S, He H, et al. Ferroptosis contributes to catecholamine-induced cardiotoxicity and pathological remodeling. Free Radic Biol Med. (2023) 207:227–38. doi: 10.1016/j.freeradbiomed.2023.07.025
153. Li D, Song C, Zhang J, Zhao X. Resveratrol alleviated 5-FU-induced cardiotoxicity by attenuating GPX4 dependent ferroptosis. J Nutr Biochem. (2023) 112:109241. doi: 10.1016/j.jnutbio.2022.109241
154. Yu W, Chen C, Xu C, Xie D, Wang Q, Liu W, et al. Activation of p62-NRF2 Axis Protects against Doxorubicin-Induced Ferroptosis in Cardiomyocytes: A Novel Role and Molecular Mechanism of Resveratrol. Am J Chin Med. (2022) 50:2103–23. doi: 10.1142/S0192415X22500902
155. Chen D, Geng Y, Deng Z, Li P, Xue S, Xu T, et al. Inhibition of TLR4 alleviates heat stroke-induced cardiomyocyte injury by down-regulating inflammation and ferroptosis. Molecules. (2023) 28:2297. doi: 10.3390/molecules28052297
Keywords: diabetic cardiomyopathy, iron metabolism, ferroptosis, mitochondria, therapy
Citation: Tian M, Huang X, Li M, Lou P, Ma H, Jiang X, Zhou Y and Liu Y (2024) Ferroptosis in diabetic cardiomyopathy: from its mechanisms to therapeutic strategies. Front. Endocrinol. 15:1421838. doi: 10.3389/fendo.2024.1421838
Received: 23 April 2024; Accepted: 15 October 2024;
Published: 11 November 2024.
Edited by:
Ying Xin, Jilin University, ChinaReviewed by:
Weijian Hang, Huazhong University of Science and Technology, ChinaCadiele Oliana Reichert, Federal University of Santa Catarina, Brazil
Yanbo Shi, Jiaxing Traditional Chinese Medicine Hospital Affiliated to Zhejiang Chinese Medical University, China
Tao He, Hanze University of Applied Sciences, Netherlands
Copyright © 2024 Tian, Huang, Li, Lou, Ma, Jiang, Zhou and Liu. This is an open-access article distributed under the terms of the Creative Commons Attribution License (CC BY). The use, distribution or reproduction in other forums is permitted, provided the original author(s) and the copyright owner(s) are credited and that the original publication in this journal is cited, in accordance with accepted academic practice. No use, distribution or reproduction is permitted which does not comply with these terms.
*Correspondence: Yaru Zhou, enlyeWRzeUBoZWJtdS5lZHUuY24=; Yan Liu, bGl1eWFuQGhlYm11LmVkdS5jbg==
†These authors have contributed equally to this work