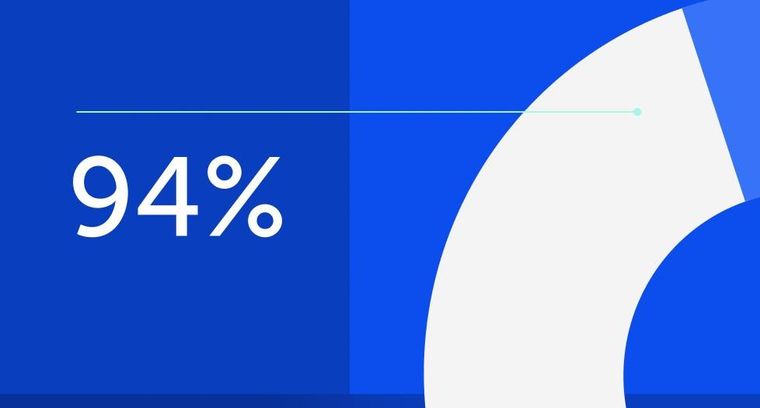
94% of researchers rate our articles as excellent or good
Learn more about the work of our research integrity team to safeguard the quality of each article we publish.
Find out more
REVIEW article
Front. Endocrinol., 08 July 2024
Sec. Molecular and Structural Endocrinology
Volume 15 - 2024 | https://doi.org/10.3389/fendo.2024.1401120
This article is part of the Research TopicEndocrine Disruptors in Molecular and Structural EndocrinologyView all 5 articles
Parts of this article's content have been modified or rectified in:
Erratum: Addressing chemically-induced obesogenic metabolic disruption: selection of chemicals for in vitro human PPARα, PPARγ transactivation, and adipogenesis test methods
Whilst western diet and sedentary lifestyles heavily contribute to the global obesity epidemic, it is likely that chemical exposure may also contribute. A substantial body of literature implicates a variety of suspected environmental chemicals in metabolic disruption and obesogenic mechanisms. Chemically induced obesogenic metabolic disruption is not yet considered in regulatory testing paradigms or regulations, but this is an internationally recognised human health regulatory development need. An early step in the development of relevant regulatory test methods is to derive appropriate minimum chemical selection lists for the target endpoint and its key mechanisms, such that the test method can be suitably optimised and validated. Independently collated and reviewed reference and proficiency chemicals relevant for the regulatory chemical universe that they are intended to serve, assist regulatory test method development and validation, particularly in relation to the OECD Test Guidelines Programme. To address obesogenic mechanisms and modes of action for chemical hazard assessment, key initiating mechanisms include molecular-level Peroxisome Proliferator-Activated Receptor (PPAR) α and γ agonism and the tissue/organ-level key event of perturbation of the adipogenesis process that may lead to excess white adipose tissue. Here we present a critical literature review, analysis and evaluation of chemicals suitable for the development, optimisation and validation of human PPARα and PPARγ agonism and human white adipose tissue adipogenesis test methods. The chemical lists have been derived with consideration of essential criteria needed for understanding the strengths and limitations of the test methods. With a weight of evidence approach, this has been combined with practical and applied aspects required for the integration and combination of relevant candidate test methods into test batteries, as part of an Integrated Approach to Testing and Assessment for metabolic disruption. The proposed proficiency and reference chemical list includes a long list of negatives and positives (20 chemicals for PPARα, 21 for PPARγ, and 11 for adipogenesis) from which a (pre-)validation proficiency chemicals list has been derived.
The global incidence of obesity and metabolic disorders is growing dramatically (1, 2). Noncommunicable diseases, including metabolic syndrome, are estimated to account for 74% of all deaths globally (3). The increasing incidence of obesity, type 2 diabetes, insulin resistance and hypertension are commonly considered to be a consequence of lifestyle, particularly high dietary intakes of sugar, processed food, and trans-unsaturated fatty acids, large portion sizes, and decreased physical activity (4). An association with socioeconomic status, age, sex and ethnicity is often reported, but environmental and industrial contaminants are also considered to play a role in altering metabolism in humans and contributing to the epidemic of non-communicable diseases including metabolic syndrome. Metabolic disruption, in the context of energy metabolism, is a complex process involving multiple tissues, organs, and relevant molecular targets. This disruption can manifest in various forms, including dysregulated glucose metabolism, impaired lipid metabolism, altered hormonal signalling, and dysfunctional mitochondrial function (5).
Indeed, the roles of chemical and environmental factors are increasingly acknowledged (1, 2, 6, 7), in addition to the established nutrition and lifestyle causative factors (8). These factors, especially exposure to environmental chemicals, contribute to the complexity of metabolic disorders and highlight the need for a comprehensive understanding of their underlying mechanisms in order to develop regulatory relevant test methods to adequately assess the hazards of MDCs. At the molecular level, metabolic disruption may involve abnormal activity or expression of enzymes, receptors, transporters, and signalling molecules, all of which can be perturbed by chemical exposure.
The economic impact of chemically-induced metabolic disruption for the three groups of chemicals for which the weight of evidence of causing metabolic disruption in humans is strongest (p,p’-dichlorodiphenyldichloroethylene (p,p’-DDE), phthalates, bisphenol A (BPA)) is estimated to exceed €18 billion (with moderate probability) in Europe (6, 9). Developing appropriate human-relevant test methods to adequately assess these putative chemical hazards is needed for front-end public health protection, to reduce the contribution that chemical hazards may make towards metabolic disruption. This will also support greener more sustainable chemistry development, reducing potentially regrettable chemical substitutions.
To address the need for elucidating and identifying metabolic disruption chemicals, the European Commission has dedicated funding to the development and (pre-)validation of test methods and Integrated Approaches to Testing and Assessment (IATA) under the Horizon 2020 Research and Innovation framework, to three projects of the EURION cluster: EDCMET (10), GOLIATH (7), and OBERON (11). To this end, the EU-funded Horizon 2020 GOLIATH project (7) (https://beatinggoliath.eu/; https://cordis.europa.eu/project/id/825489) aims to address the lack of methods for testing EDCs that disrupt metabolism and metabolic functions. These chemicals collectively referred to as “metabolism disrupting compounds” (MDCs) are natural and anthropogenic chemicals that can promote metabolic changes that can ultimately contribute to the development of obesity, diabetes, and/or fatty liver in humans. The project has focused on the main cellular targets of metabolic disruption: Peroxisome proliferator activated receptor (PPAR) test methods, hepatocytes, pancreatic endocrine cells, myocytes and adipocytes-and using a mechanistic and mode of action or adverse outcome pathway (AOP) type framework, has generated key information on MDC-related modes of action (7). The project committed to take forward between three to five test methods towards pre-validation, depending upon the test method optimisation and test method readiness to enter the OECD Test Guideline Programme. To support in vitro test method development and validation, careful and relevant chemical selection needs to be conducted for each endpoint, and with clear understanding of the test methods, including their potential limitations.
Here we report on the selection of recommended reference chemicals in relation to human white adipose tissue adipogenesis, PPARα and PPARγ agonism, to support the (pre-)validation of these test methods within the GOLIATH project. Related chemical selection reviews previously published from the same project include the chemical selection for steatosis (12) and CYP induction chemical selection augmentation (13).
There are several types of fat tissue in the human body, each with its own characteristics and functions. The two main types of fat are white adipose tissue (WAT) and brown adipose tissue (BAT), also there is a transitional type known as beige or brite adipose tissue. Brown fat cells have a significant number of mitochondria, which play a central role in energy production, compared to white fat cells (14). Activation of brown fat may contribute to increased energy expenditure, which may play a role in preventing or managing obesity. Brown fat has the ability to burn calories to produce heat, and individuals with higher amounts of active brown fat may have an increased capability to resist weight gain. This is important in the context of preventing obesity-related metabolic diseases (15). However, the focus of this chemical selection review is specific to the mechanistic understanding in relation to an increase in WAT in particular via the well understood Molecular Initiating Events (MIEs) of PPARγ activation, but also the role PPARα. PPAR agonists are known to help alleviate the underlying metabolic dysregulation observed in metabolic syndrome and insulin resistance by targeting different aspects of metabolism. They improve lipid profile, enhance insulin sensitivity, reduce inflammation, and promote metabolic homeostasis (16). Although relevant to metabolic disruption, chemical selection regarding BAT will be conducted separately, and is not part of the scope of this paper.
Nutritionally, the PPARs evolved as a fatty acid-activated nuclear receptors, where long chain fatty acids are the key molecular targets, to effect regulation of signal transduction processes and gene regulation, which in turn dictate their roles in health and disease (17–19). Fatty acids are important players in the development of the pathology of cardiovascular and endocrine diseases, thus understanding of the roles of fatty acids is useful for identifying chemical characteristics that contribute to the potential to interact with the PPARs. PPARα activation results in biological mechanisms leading to fatty acid oxidation in the liver cells as a response e.g., to extended fasting and/or energy deprivation. PPARγ activation, on the other hand, stimulates storage of fatty acids, including in the adipose tissues (20). This has essentially been the basis for the development of pharmaceuticals such as the thiazolidinediones for PPARγ, and fibrates for PPARα (21, 22), to lower triglyceride or glucose levels.
In the literature, a number of chemicals have been implicated in relation to obesity, including bisphenols, pesticides, phthalates, metals, and perfluorinated compounds. Here we present a more thorough analysis of chemicals that may (or may not) be implicated in the mode of action of adipogenesis. From the MIE(s) of PPARα and PPARγ activation, the later key event (KE) of WAT adipogenesis stimulation, with the intention that it can be a basis for developing and optimizing human adipogenesis-relevant in vitro test methods that can be integrated together as a test method battery, as part of an IATA for metabolic disruption.
The PPARs are a well understood family of orphan nuclear receptors within a family of transcription factors involved in gene regulation of key physiological processes, with fundamental roles in regulating energy balance. Three closely related receptors: PPARα, β/δ, and γ, are variously expressed in the liver, kidney, heart, hematopoietic and adipose tissue. With a central role in hepatogenesis, PPARα is expressed primarily in liver, but also kidney, heart and muscle. PPARδ is ubiquitous and has a fundamental role in embryo development, it allows normal cells to better cope with adverse nutrient and energy situations, it has a therapeutic role in burning fat, although if over-expressed it can promote inflammation and tumorigenesis (23). Selective ligands for PPARδ are lacking (24), and PPARδ is not the focus of this chemical selection objective.
A number of prevalent metabolic disorders such as obesity, atherosclerosis and type 2 diabetes mellitus are associated with a shift in this balance, so PPARα and PPARγ in particular, are of interest pharmacologically (25). Model agonists include xenobiotics that elicit increases in the number and size of peroxisomes when administered to rodents, and also can induce hepatocellular carcinoma development via a non-genotoxic mechanism (26).
PPARγ has a central regulatory role in adipogenesis, lipogenesis and lipid storage in the liver and adipose tissue, and adipokine production and is pivotal for whole body insulin sensitivity primarily in the muscle (25, 27). It is found in adipocytes, the large intestine, and monocyte lineage cells.
PPARα regulates key steps in lipid and fibrate metabolism, fatty acid oxidation and fasting response. It is the molecular target for naturally occurring plant fatty acids and peroxisome proliferators, including pharmaceuticals, phthalates and pesticides (28, 29). PPARγ ligands include fatty acids, prostaglandins and pharmaceuticals such as the group of antidiabetic drugs, thiazolidinediones (glitazones) (25, 30, 31). As with other ligand-activated nuclear receptors, the PPARs bind to their cognate DNA elements as heterodimers with the Retinoid X Receptor (RXR), and then activate the transcription of downstream genes, including e.g., Cytochrome P450 (CYP)-mediated metabolism CYP4A genes. This can lead to enhanced CYP metabolism of the ligand as reviewed previously (31), and activation of genes involved in the regulation of energy homeostasis (32).
Activation of PPARα induces the expression of CYP enzymes involved in the ω-oxidation pathway, such as CYP4A, which catalyzes the oxidation of fatty acids (29). PPARγ also regulates the expression of certain CYP enzymes, particularly in adipose tissue, with potential regulatory effects in the expression of Cyp2f2 (33).
As RXR is relevant for the PPAR MIE, the scope of the chemical selection review, took this into consideration. Figure 1 gives an overview of the PPARs and their roles in adipogenesis.
Figure 1. The role of the PPARs in fat and glucose metabolism (modified from Evans et al., 2004, organ illustrations from Biorender.com and Microsoft PowerPoint). FAO, Fatty acid oxidation; WAT, White adipose tissue.
Adipogenesis is the molecular and cellular process by which undifferentiated cells, typically mesenchymal stem cells, differentiate and develop into mature adipocytes (fat cells) capable of storing and releasing lipids (34). This process plays a fundamental role in the development and maintenance of adipose tissue, which is responsible for storing energy in the form of fat. It is also crucial for energy homoeostasis; adipocytes store excess energy, releasing it during times of need. If adipocytes become hypertrophic, owing e.g., to chronic energy excess, they also can become insulin-resistant. They lose their ability to appropriately respond to physiological levels of insulin and other mediators of energy metabolism/expenditure, such that energy expenditure is impaired, and lipid storage becomes dysfunctional, leading to excess accumulation of (visceral) body fat. Dysfunctional adipocytes are unable to respond adequately to physiological levels of insulin and other mediators of energy metabolism/expenditure. Fatty acids are released into the circulation and accumulate in other organs and are stored as body fat. The dysregulated secretion of endocrine factors from hypertrophic and dysfunctional adipocytes contributes to the development of systemic inflammation, insulin resistance, and metabolic disruption, leading to pathological changes in various organs and tissues implicated in obesity-related metabolic disorders (35).
Adipocytes originate from mesenchymal stem cells (MSCs), which are multipotent cells that can differentiate into various cell types, including adipocytes, osteoblasts, and chondrocytes through a complex and multi-step process comprising a network of transcription factors (36, 37). Adipogenic signalling pathways (e.g. Wnt, BMP or Hedgehog signalling) and transcription factors such as PPARγ, C/EBPs, sterol regulatory element-binding protein (SREBP), and glucocorticoid receptor (GR) are considered as master regulators of genes in adipogenesis (38) and their differential expression/activation determines the adipocytic phenotype (i.e., WAT, BAT, or beige adipocytes).
Adipocyte differentiation can be induced in cell culture by the stimulation of MSCs with isobutylmethylxanthine (IBMX), dexamethasone, and insulin, in particular for the murine 3T3-L1 cell line (39). Multipotent human MSCs are reported to require a PPARγ agonist to effectively promote differentiation (40). The 3T3-L1 cell line is a well-established model for studying adipogenesis and has been extensively used to elucidate the transcriptional cascade and molecular mechanisms underlying adipocyte differentiation. Greater details on the relative merits of the murine and human cell systems are reviewed in Kassotis et al., 2022 (41) and references therein. Stimulation by IBMX inhibits phosphodiesterases, leading to increased intracellular levels of cyclic AMP (cAMP) and activation of C/EBP-δ. Dexamethasone induces expression of C/EBP-α, C/EBP-β and -δ, in turn, these induce the expression of C/EBP-α and PPARγ2. Insulin stimulates both adipogenesis and lipogenesis through induction of SREBP-1c and other transcription factors, in addition to directly inhibiting lipolysis (41). Whilst GR is therefore highly relevant to PPARγ with respect to the promotion of cellular differentiation (42, 43), and therefore consideration of GR ligands is included in this chemical selection, chemical selection and pre-validation specifically focused upon the GR was not included in the original GOLIATH proposal, and is currently being addressed by the PEPPER platform (L’association Pepper (ed-pepper.eu)).
Adipogenesis can be assessed and quantified in vitro by measuring endpoints such as the degree of intracellular lipid accumulation, the expression of adipogenic genes and their corresponding protein products. This is therefore the basis of in vitro test method development, that, when ready, can be proposed for test guideline development, as part of the obesity endpoint aspect of an IATA for metabolic disruption.
Currently, there are no existing test guidelines, approaches or testing strategies for metabolic disrupting chemicals, but the need for such methods has been expressed in a number of international and European reports (44–46), and the intention to develop and optimise such test methods was part of the European Commission Horizon 2020 call that the GOLIATH consortium has striven to address.
For regulatory purposes, test methods need to demonstrate that they are able to address the intended chemical applicability domain and to classify chemicals correctly (47–50). In vitro test method tools can provide mechanistic information to elucidate the modes of action leading to apical adverse outcomes, thereby contributing to the reduction of in vivo testing (51), especially when combined within IATAs (50). To facilitate the development of IATAs, to improve human health relevance and better utilise in vivo data, new approach methodologies (NAMs) can also provide concentration-response information. Historically, for many OECD in vitro Test Guidelines (TGs), the initial focus has been on (dichotomous) hazard identification particularly for classification and labelling needs, but also based upon the extent to which the results from the validation study can be reliably interpreted. However, to enable in vitro test methods to be used beyond prioritisation for subsequent testing in animal studies, to develop utility within IATAs, it is of value if a test method can also provide continuous (quantitative) data to inform upon the potency of a chemical (47, 52). This will enable the characterisation of the influence of physico-chemical properties on variability across the concentration response being generated (52).
Here we report on a minimum set of tentative provisional proficiency chemicals for test method development, optimisation and (pre-)validation testing for molecular and tissue-level effects with relevance towards metabolic disruption. Specifically, chemicals were selected to probe molecular transactivation of the human nuclear receptors PPARα and PPARγ and also adipogenesis (for the latter, commitment of human mesenchymal stem cells to the adipose lineage, and promotion of lipid accumulation in WAT adipocytes is key).
The selection of chemicals, suitable for the industrial chemical applicability domain and associated test method regulatory purposes was based upon the following criteria: i) structural diversity to address the physico-chemical properties applicability domain of the industrial chemical universe (e.g. biocides, pesticides, plasticizers, flame retardants, industrial coatings, pharmaceuticals etc.), for which the test method is intended to predict endpoint-specific toxicity (i.e., PPARα, PPARγ, adipogenesis), ii) receptor cross-talk in lipid homeostasis, and iii) be structurally relevant for the biological role of the endpoint, as shown for example with known natural or endogenous ligands, and in PPAR molecular modelling receptor ligand binding studies, as often conducted in drug discovery (31, 53–58).
At the outset of the project, in 2020, a targeted literature review was performed, for each endpoint, utilising expert knowledge in the fields of nuclear receptor activation, adipogenesis, nutrition and metabolic disruption, together with (guidance from) highly relevant well-documented reviews, to focus and retrieve pertinent chemical and target endpoint literature evidence. The literature search was later updated and supplemented in relation to PPAR and adipogenesis obesity key events in late 2023, to include relevant recently published articles for each chemical and test method. Details regarding the literature search are provided in Supplementary Material 1: Literature search.
The data were primarily prioritised and evaluated according to greatest human relevance and data reproducibility, with support from data generated within the GOLIATH project, e.g. Garoche et al. (59), for candidate proficiency chemicals. Based on this initial expert input, key candidate chemicals were identified, and the Scopus and PubMed databases were iteratively queried for additional, related chemicals with substantial literature support. Where identified, prototypical chemicals from literature review articles were included in the preliminary candidate list. Chemicals with the highest assessment with a reasonably well documented weight of supporting evidence, relevance towards metabolic disruption mechanisms, and relevance towards OECD test method development, were prioritized and are proposed as tentative chemicals for test method development, optimisation, proficiency, and (pre-)validation testing. These are summarised in Table 1, together with concentration information, CAS Number and 2D structures, and are itemised for PPARα, PPARγ and adipogenesis in, Tables 2A–C respectively, with the detailed review provided in Supplementary Material 2: Table 1. Structural diversity was assessed by structural examination in combination, with an understanding of known ligands and molecular pharmacophore and docking studies for the PPARs (31, 54–56, 58, 225).
Table 1. Summary chemical selection table for the pre-validation of hPPARα and hPPARγ transactivation, and hMSC adipogenesis test methods.
As documented in Supplementary Material 2: Table 1: The Weight of Evidence (WoE) approach undertaken to evaluate the literature obtained first examined the scope of each paper, describing the study or review undertaken, the range of concentrations/doses tested where given, and collated the uncertainties and limitations of each paper reviewed, to arrive at a summary of the literature for each chemical, in relation to the specific endpoints under scrutiny.
Chemical selection coverage considered specific reference chemicals for the hPPARα and hPPARγ transactivation test methods and the adipogenesis test method individually. It also included chemicals that are in common with PPARα and PPARγ, PPARα but not PPARγ, and then PPARγ and not PPARα. And then also in combination with the (hMSC) adipogenesis test method evaluates the capacity of chemicals to stimulate the formation of (in vitro) human adipocytes. Additionally, the PPAR heterodimerisation partner RXR was also considered, as studies have shown that activation of RXRα without direct binding of PPARγ can lead to increased lipid accumulation in hMSCs. This has been shown for example for 2,4-di-tert-butylphenol (2,4-DTBP) (226) and for tributyltin (TBT) (227) and this mechanism is considered perturb the development of WAT.
The aim was also to have as broad a coverage as realistically possible within a minimum number of chemicals for which there is robust evidence, to address and probe the chemical applicability domain that the metabolic disruption IATA for obesity needs to address. As indicated for the development of testing strategies, the chemical applicability domain needs to reflect the chemical sector universe for which the test method will be utilised, and the chemicals selected and subsequently tested can start to gauge the relative points of departure for the mechanism and/or endpoints under investigation.
In common with, and expanding upon the primary considerations for the selection of chemicals (48) and as indicated in previous publications (12, 13), the following aspects also guided the selection and prioritization of candidate chemicals: Availability and international considerations; cost; human mechanistic relevance; reproducibility; range of activity; inclusion of metabolites and avoidance of undefined chemical mixtures.
The chemicals were selected to accommodate national and international limitations on use, as the pre-validation reference chemicals will ultimately be intended for use in OECD TGs, with global distribution and use. Consequently, chemicals listed under the Stockholm Convention on Persistent Organic Pollutants (228) or being proposed to be added, were generally avoided, with respect to validation and subsequent TG development.
However, some chemical classes are of high current interest, and a relatively rich amount of information was available for these, particularly the perfluorinated chemicals (189–191, 229). Whilst it is very useful to learn more about the toxicity of these chemicals and confirm test method performance in the development/optimisation phase, for (pre-)validation purposes, it is preferred to keep the reference and proficiency chemicals that also are on the POPs list, to an absolute minimum, as, in addition to the disposal issues, this may lead to practical difficulties in running the tests and acceptance for some OECD member countries.
Additional chemicals to be excluded are those that have (variable) global restrictions with respect to substance abuse, such as anabolic steroids, and (illegal) drugs such as cannabinoids. Thus, while there are interesting data available for anabolic steroids and cannabinoid chemical classes, with potency for the endpoints of interest (230–233), these were not suitable for test method validation purposes.
Chemicals that are rare, difficult to obtain and costly are generally not be prioritised unless there are no alternatives. Ultimately the components of a validated test method that is intended for OECD (TG) adoption needs to be globally and financially accessible for all OECD stakeholders. There also needs to be consideration of longer-term production and availability of the proficiency chemicals as far as reasonable. Specific issues in this regard for these chemicals were checked and concerns were not identified.
Chemicals were selected based on high relevance to humans, wherever possible. This is because the test methods are being developed to address human relevant mechanisms/endpoints. Species specific differences are well known for PPAR transactivation and metabolism studies (57, 59), although for both human and mouse mesenchymal stem cells, cannabidiol for example has been shown to activate mouse and human PPARγ, but not its heterodimeric partner RXR. As a consequence of PPARγ activation, increased lipid accumulation and the expression of adipogenic genes was shown for both species in vitro (230).
Human MSC data are considered to be more human relevant than murine 3T3-L1 data, as discussed further in the following section. Whilst the 3T3-L1 mouse pre-adipocyte cell line is extensively utilised for identifying adipogenic chemicals, and it is easier to use a cell line, rather than primary cells, the species relevance with respect to induction of human adipogenesis is weaker. This cell line is already induced/committed to the (pre-)adipocyte lineage, so data generated are indicating the potential for promotion but not initiation of adipogenesis. For this particular cell line, data often differ for certain chemicals, as compared to a more human relevant model using primary hMSCs (41) and pre-validation (Hoffmann et al., manuscript in preparation) and lineage derived cells (201), and thus greater weight in the literature review was given to data generated using in vitro test systems that better address initiation of adipogenesis.
With test method validation in mind, chemicals need to be selected on the basis of reproducible data, not a single literature report. Where data have been reported to not be reproducible, as for example with the PPAR activity data from Tox21 (40, 160), these data were not used. Janesick et al. (160) report that in trying to reproduce the ToxCast data only 5/21 of the top scoring ToxCast™ PPARγ activators were activators, three were PPARγ antagonists, the remainder were inactive. The authentic PPARγ activators identified induced adipogenesis in 3T3-L1 cells and murine MSCs. Only 7 of the 17 chemicals predicted to be active by the ToxCast™ ToxPi promoted adipogenesis, one inhibited adipogenesis, and two of the 7 predicted negatives were also adipogenic. Of these 9 adipogenic chemicals, three activated PPARγ, and one activated RXRα. It can therefore be concluded that ToxCast™ PPARγ and RXRα assays and some of the computational tool predictions available from the US EPA Chemical Dashboard, are suitable for high throughput screening (HTS) in the identification of potential hazards and prioritisation of chemicals from large chemical libraries that could not otherwise be screened in lower-throughput model systems. However, for the test method applications intended here, the lack of correlation with laboratory measurements of PPARγ and RXR meant that the data were not sufficiently robust to support the chemical selection. Therefore, taken in isolation, this set of 3T3-L1 data was considered to be unreliable for identifying new PPAR and adipogenesis reference chemicals, but was useful in contributing to the WoE. Notably, the 3T3-L1 cell line is derived from mouse embryonic fibroblasts, and while it shares some similarities with human adipocytes, there are significant species-specific differences in gene expression, metabolism, and response to stimuli. As with many and indeed most cell lines that haven’t been validated, there can be variability in the differentiation efficiency of 3T3-L1 cells between different laboratories and experimental conditions, as well as strain drift. Factors such as passage number, cell confluency, and the composition of differentiation media can influence the extent and kinetics of adipocyte differentiation in the cell line, leading to inconsistencies in experimental results. Due to the limitations and variability associated with 3T3-L1 cell-based assays, hazard assessment for regulatory purposes needs to be cautious if relying solely on data generated from these models in regulatory decisions. However, such data does have utility as supporting information, with value for future applications, as data robustness and reliability is improved.
Independently, Filer et al. (174) assessed the discrepancies and uncertainty of ToxPi predictions using the different ToxCast datasets, focusing on the accuracy to predict activity of chemicals inducing adipogenesis and metabolic disruption in murine 3T3-L1 preadipocytes (174). Poor predictive performance was considered to be a consequence of a number of factors such as reliance on a single model, the development phase/stage of ToxCast, the cytotoxicity scoring and correction system, the weighting approaches and chemical selection utilised (174). While an adjusted prediction model yielded a balanced accuracy of 0.97 in predicting adipogenesis of a ToxCast chemical subset, the authors recommend that an extended verification of the predictive capacity with more chemicals (38 in training set, 30 in test set) is needed to better cover the diverse chemical space. Examining the models developed by Filer et al., for the purposes of this chemical selection, it was noted that the focus was more on semi-volatile chemicals and use of the 3T3-L1 cell line. Use of semi-volatile chemicals, without further technical adjustments to prevent chemical cross-contamination (“chemical creep”), such as using adhesive seals on the testing plates is a confounding hazard that requires strict control, volatile chemicals generally need to be avoided in validation studies. Chemical data from Filer et al. (174) were therefore included where relevant, to support the chemicals selected (and this is noted in Supplementary Material 2: Table 1). Further, it has also been recognised that expression levels in cell sources of 3T3-L1 cells can vary and this can influence the classification of (adipogenic) chemicals. Gene expression assays have shown that PPARγ expression is higher in OP9 cells compared to ATCC 3T3-L1 cells. This could partially account for the lower relative fold induction observed in these cells. However, Zenbio 3T3-L1 cells exhibited the highest relative fold inductions, showing no difference with those from ATCC 3T3-L1 (86). Furthermore reported reproducibility issues and variation in 3T3-L1 preadipocytes laboratory strains (86) and different protocol induction/exposure periods and plasticware also substantially affected the results generated, as also noted above. For example, in some protocols, there was a distinction between the induction period (with “induction cocktail” or similar, often containing e.g., IBMX, insulin, dexamethasone) and exposure to test chemicals (in medium with/without induction chemicals).
Finally, while PPARγ activation is considered necessary and sufficient for adipogenesis in humans and cell culture systems, including mouse models and cell lines such as 3T3-L1, the fundamental mechanisms of adipogenesis are highly conserved across species, including humans. Activation of the signalling pathway may occur through mechanisms other than or in addition to receptor activation (25, 234).
Pharmaceutical chemicals with a defined mode of action are included, so that there is sufficient coverage of mechanistically well understood weak-moderate actives, such that target specificity is addressed as well as reasonably possible. Principally, GW3965 is included as a weak PPARγ agonist and weak adipogenesis inducer, and tesaglitazar as a weak PPARα agonist.
Chemicals selected should preferably have a range of activity that spans negative, low/weak, moderate, and high/strong activity in the test method, as compared to the positive control, but this may not always be feasible, - depending upon the quality and weight of evidence of the supporting literature. To verify the selected potency bands (negative, weak, moderate and strong) and to ensure their validity and applicability towards the target in vitro test method, subject matter experts reviewed the literature for information relating to concentration and dose -response, consistency and biological relevance and (potential) uncertainties. From this review (Supplementary Material 2: Table 1) putative bands of activity ranging from negative, weak to moderate and strong were determined and are provided in Tables 1 and 2A–C. These potency bands will be confirmed following (pre-)validation of the respective test methods.
At least 25% of the chemicals in the full reference set should be negatives, ideally more, up to 50%. While a lower share of negatives can be acceptable during test method development, optimisation and early proficiency testing, recommendations should be improved as confidence in the test method is gained aiming at ultimately achieving 25–50% negative proficiency chemicals for (pre-)validation studies. The use of tools to identify and exclude chemicals with potentially promiscuous activity was an addition to the chemical selection process, and it will be important to continue to look for clusters of in vitro assays that may indicate non-specific activity through a common molecular target. Therefore, all chemicals included here were also assessed with respect to their potential to deliver false-positive results in screening assays due to non-specific reactive chemistry interference, using the “pan assay interference compounds (PAINS) remover” tool (version Demo 0.99) (235). None of the structures were identified as active/positive for non-specific reactive chemistry interference using this filtering tool.
Tables 1, 2A–C and the Supplementary Material 2: Table 1. provide summary and full review details respectively.
Some chemical metabolites may have increased or reduced toxicity. OECD expert group recommendations are to include some active metabolites where possible (159, 236, 237). If a respective model has limited metabolic capacity, the inclusion of both the parent chemical and the active metabolite(s) is relevant.
To ensure clear attribution of effects observed with the endpoint in question, when characterising both a chemical and a candidate test method, single and pure chemicals need to be used. Purity needs to be documented. Undefined isomeric and racemic mixtures of a specific chemical should be avoided. Isomeric mixtures of the same chemical are variable and consequently can result in highly variable results. This has been observed for nonylphenol in some early optimisation and validation efforts for ER binding, for example (Jacobs personal communication), and so is best avoided, unless the isomer distribution can be clearly and consistently quantified, isomers can be tested individually, and there is additional justification for using such a chemical.
As indicated in the methods section 2, this chemical selection was developed to support the design and development of a conceptual IATA for metabolic disruption, with specific reference chemicals for each PPARα and PPARγ transactivation test method, and the WAT adipogenesis test method, but also to selectively probe each one of the three test methods, as well as consideration of the heterodimerisation partner RXR. Thus, the chemicals were selected to provide some overlap, and thus strengthen the mechanistic evidence linking molecular and adverse organ/tissue-level effects and support the development of both Adverse Outcome Pathways (AOPs) and IATAs for metabolic disruption. This approach to chemical selection is being recommended to enhance regulatory relevant development of IATAs and the design of validation studies for the NAMs addressing the IATA KEs (238).
As diabetes and metabolic diseases are often associated with high blood glucose and lipid levels, drugs that activate both PPARα/γ are included. In addition to the environmental contaminants and pharmaceuticals known to perturb this receptor, inclusion of natural ligands will assist in developing the predictive models for PPAR transactivation test methods.
Learnings from medicinal chemistry developments and molecular docking studies (7, 59, 239–243), contribute to the selection, especially in relation to physico-chemical properties. For example, the addition of fluorine to the headsets of experimental PPAR ligands, increase potency and persistence, and can indicate the likelihood of respective PPAR ligand binding, as seen in vitro, for example, with the PFAS class of chemicals (59).
Looking at the PPARs heterodimerisation partner RXR, RXR ligands such as CD3264, TBT, or 9-cis retinoic acid are reported to interfere in reporter cell lines such as the HG5LN GAL4-PPAR, by decreasing the signal induced by the PPAR ligands according to their Kd for RXRs (59). Therefore, the RXR ligand TBT is included in the chemical selection for an adipogenesis test method, and relevant RXR interactions reported in the literature are captured.
Whilst inclusion of more chemicals with antagonistic activity would be desirable for mechanistic understanding and complete characterisation of a chemical’s activity towards the respective receptor, such information was scarce, and support from human health-relevant studies (i.e., human in vivo information) was lacking. Therefore, the tentative preliminary proficiency chemicals proposed herein focus on PPAR agonist binding/activity modalities.
The hMSC-derived in vitro adipogenesis test method is the prototypical method that was considered as a potentially suitable candidate for human-relevant WAT adipogenesis (230). While the US EPA Tox21 screening battery utilises the murine 3T3-L1 preadipocyte cell line for identifying (environmental) obesogens with recently reported more satisfactory accuracy (174), the data did not satisfy the objectives here, with respect to greater accuracy of human relevance. The aim of this chemical selection was to identify chemicals with highest relevance towards the perturbation of initiation and promotion of human adipogenesis, and for a candidate test method to ideally capture both, the commitment of precursor cells such as MSCs, to the (WAT) adipocyte lineage, and the subsequent promotion of intracellular lipid accumulation.
Building upon the chemicals selected for PPARα and PPARγ, the chemicals to be utilised for this test method could also include modulators of the Wnt and other critical signalling pathways with a demonstrated role in adipogenesis (244–247), and also in relation to the PPAR heterodimerization partner RXR. With respect to the latter, pharmacological rexinoids such as targretin (170), and natural ligands e.g. retinoic acids (248) that have also been shown to directly interact with PPAR receptors (249, 250) were also considered. The putative potency bands derived were negatives and positives, in addition to the positive control (weak, weak to moderate, moderate and strong activity).
In summary, 20 chemicals, including back-up chemicals were identified as a source list for PPARα preliminary proficiency testing. Of these, 10 are negatives: BPA, TPP, pp’-DDE, TCS, ROSI, CPF, PFHXA, MBX-102, TBBPA and targretin; and 10 are positives: phytanic acid, clofibrate and AGN194204 (3 weak agonists); MEHP, EPA, tesaglitazar, and clofibric acid (4 weak-moderate agonists); PFOA, pristanic acid, DHA (3 strong agonists), plus the positive control chemical: GW7647. For PPARγ proficiency testing 21 chemicals were identified. Of which 5 are negatives: BPA, pp’-DDE, TCS, PFHXA and targretin; and 16 are positives: CPF, clofibrate, phytanic acid, MBX-102 and GW3965 hydrochloride (5 weak agonists); MEHP, GW7647, EPA, clofibric acid, and pristanic acid (5 moderate agonists), TPP, DHA, TBBPA, PFOA, 15d-PGJ2 and tesaglitazar (6 strong agonists), plus the positive control chemical: rosiglitazone. Fludioxonil was categorised as unknown for PPARγ, however there are some negative results.
For the hMSC adipogenesis test method we propose 11 proficiency chemicals. The following 4 negatives were identified: TCS, TTNPB, pp’-DDE, and CPF. 7 positives were identified, PFOA (1 weak), GW3965 hydrochloride, TBBPA, MEHP, TPP (4 moderate), fludioxonil and TBT chloride (2 strong). Rosiglitazone is the positive control for candidate adipogenesis test methods.
Phytanic acid, a natural PPAR agonist, regulates glucose metabolism in rat primary hepatocytes (251). Whilst phytanic acid induces beige adipocyte differentiation, (but does not for brown fat), this differentiation has been shown to be mediated by PPARα (252). It is also a natural RXR agonist at physiological concentrations and it mediates the favourable effects of phytol derivatives on BAT adipocyte differentiation, and also induces differentiation of UCP1 in mouse under in vivo and in vitro conditions, postulated to be via RXR especially in presence of other PPARγ activators (193). 50 μM phytanic acid treatment induced differentiation in 70% of the 3T3-L1 preadipocytes assessed by lipid droplet accumulation and aP2 mRNA induction (194), and in similar experiments this was considered to probably be mediated by RXR (195). However, adipocyte differentiation in mouse embryo fibroblast (C3H10T1/2) cells was reported to be very low, as few cells were differentiated following 50 μM phytanic acid treatment (193, 251). Thus, whilst phytanic acid has been implicated in the normal biological process of adipogenesis, further research is needed to elucidate its precise mechanisms of action and its role in adipose tissue biology including whether it is able to commit MSCs to the (WAT) adipocyte lineage, or rather promotes lipid accumulation and maturation in committed (pre-)adipocytes. Therefore, phytanic acid has been categorised in category unknown, and is currently a lower priority chemical for (initial) test method characterisation and (pre-)validation.
A summary of high priority chemicals is given in Table 1, and extensive background information is provided in Supplementary Material 2: Tables 1 and 2. Other RXR relevant chemicals are BPA, TBT, phytanic acid, calcitriol, targretin, AGN194204, fludioxonil and 2,4-DTBP. Studies have indicated that they can interact with RXR and affect its activity, potentially disrupting signalling pathways mediated by RXR-containing heterodimers (84, 87, 88, 160, 170, 194, 195, 202, 226, 253, 254).
Consideration of mechanistic and mode of action interactions of some prototypical reference/proficiency chemicals, within a putative natural history mechanistic model for overall increased adipogenesis are shown schematically in Figure 2. Perturbed PPAR activation is a primary MIE, that is an initial and early adaptive stimulus towards adipogenesis, with a subsequent increase in weight gain leading to obesity.
Figure 2. A schematic simplified overview model of the natural history leading to excess white adipose tissue with associated prototypical positive ligands indicated principally for the mechanisms mediated by the PPARs, RXR and adipogenesis. When heterodimerised with RXR, PPARα and PPARγ are the molecular initiating event (MIEs) gene regulators of PPAR response elements (PPREs), which maintain metabolic homeostasis, and when activated have multiple roles in adiposity and can lead to reduced insulin sensitivity and increased insulin resistance. (The heterodimerisation partner of the PPARs is also the heterodimerisation partner for a number of other nuclear receptors (NRs), of which LXR, VDR and GR are particularly relevant here.) In relation to adiposity, these include the induction of CYP4A gene transcription factors for lipid metabolism for PPARα to adipocyte differentiation, glucose homeostasis, and the expression and transcription of adipose tissue-secreted factors for PPARγ. C/EBPα, CCAAT/enhancer-binding protein α; STAT1, STAT5A and STAT5B, signal transducer and activator of transcription 1, 5A and 5B, respectively; aP2, fatty acid binding protein 2; ACBP, acyl-CoA–binding protein; LPL, lipoprotein lipase; CD36, cluster of differentiation 36; PEPCK, phosphoenolpyruvate carboxykinase; ACS, acyl-CoA synthetase; GyK, glycerol kinase; Glut4, glucose transporter 4; PI3K, phosphoinositide 3 kinase; IRS-1 and IRS-2, insulin receptor substrate 1 and 2, respectively. Pink octagons indicate prototypical ligands for the respective receptors that they are attached to, more potent ligands are indicated with the black border. MDCs are in bold, endogenous and natural ligands in italics, and pharmaceuticals in normal font. Negatives and weak chemicals from Tables 1 and 2 are not included in this figure.
Here we sought reference chemicals specific to unique targets within specified molecular pathways, whilst also considering the broader IATA development that is critical for regulatory acceptance of these assays. Due to the higher complexity of the hMSC functional assay compared to molecular-level nuclear receptor transactivation assays, the relatively longer duration, and associated higher implementation cost, we recommend a smaller set of well-characterised prototypical chemicals, including limited pharmaceuticals, as a first tier of adipogenesis test method optimisation and proficiency testing, to demonstrate the robust performance of an assay. Subsequently, a larger chemical set, including more borderline or weakly active chemicals is introduced once the test method is more mature, as a second tier of test method optimisation and in preparation for (pre-)validation testing.
Such an approach is becoming increasingly advocated, given the recent regulatory acceptability difficulties for validated in vitro assays that do not have an IATA or guidance as to how to use them (c.f. in vitro CYP induction test method (13)), and EU regulatory concerns with respect to industry dossier submissions considering peroxisome proliferation and putative lack of relevance to human toxicity (229, 255, 256), including liver tumour formation (257). Some aspects of this, in relation to cell proliferation can already be ascertained in standard in vivo toxicity testing for pesticides and pharmaceuticals (e.g., in OECD TG 407/408) (258).
The provision of this minimum set of literature-supported proficiency chemicals will facilitate the development of regulatory relevant test methods for metabolic disruption in relation to obesity. The methodological considerations, in accordance with OECD Good In Vitro Method Practices (4), for the selection of chemicals will also support the chemical selection for other (in vitro) test methods, related to adverse outcomes consequent to metabolic disruption.
Here we have described the evidence-based selection of suitable chemicals for the development and validation of PPARα and PPARγ as molecular-level events, and white adipose tissue adipogenesis as an apical organ/tissue-level adverse effect for obesity. In order to facilitate further characterisation and proficiency testing of three relevant in vitro test methods that can assess the MIE of the hPPARα and hPPARγ transactivation (luciferase-based receptor transactivation test methods using HG5LN GAL4-PPAR cell lines (59, 259) towards perturbed adiposity (hMSC primary cell test method). Understanding adipocyte biology is essential for understanding the pathophysiological basis of obesity and related metabolic diseases (such as type 2 diabetes). From this understanding, one can elucidate and support the development of suitable and more human relevant in vitro test methods towards become validated Test Guidelines.
By moving these test methods further along the road towards validation, the intention is to ultimately support regulatory applications in the assessment of chemical hazards towards the adverse health outcome of obesity/metabolic disruption, and to facilitate progress towards test guideline and IATA development. The initial list of proposed proficiency chemicals was further supported with review by international experts in regulatory test method development. However, it is acknowledged that while the PPARα to a lesser degree, and PPARγ in particular are key coordinators in the adipogenesis metabolic axis, this is not the entire mechanistic picture towards adverse adipogenicity leading to obesity (260–262). White adipocytes play essential roles in energy storage, insulation, and hormone regulation. Mature white adipocytes serve as the primary site for energy storage in the form of triglycerides. There is little cellular turnover, they expand, and they are very long-lived. A proliferating pool of MSCs and preadipocytes are the source of differentiated adipocytes that need to be created in a balanced manner to accommodate both reduced and excess energy intake. Effective means to regulate the differentiation of new adipocytes so that the number of cells balance the lipid storage need is essential, and C/EBPβ has been identified to be a critical regulatory switch controlling the transcriptional activation potential of preadipocytes and fibroblasts that can be stimulated to differentiate into mature adipocytes (25, 263). Abdou et al. (264) describe how the commitment to differentiation occurs stochastically within different cell cultures, with increasing strength of adipogenic stimulus leading to the commitment of increasing numbers of preadipocytes to differentiation and maturation. Members of the C/EBP transcription factor family are transcriptionally involved in the role of PPARγ as a primary effector of adipogenesis (25). There are further intricate mechanistic observations that are influential in capturing adversity in the mode of action landscape of the development of obesity. For example, the interactive pathways in relation to the GR (265), the liver-X receptor (LXR) and the heterodimerisation partner RXR, hepatic fibrosis, cholestasis and insulin are influential. Inflammatory cytokines (266), epigenetics and hormonal appetite control and satiety are also integral in the development and progression of obesity and metabolic syndrome. Epigenetic mechanisms, particularly histone modifications for example via HDAC1 and cofactors on C/EBPβ functions directly prior to the onset of commitment, play a contributory role in the initiation of the transcription of the commitment factors C/EBPα and PPARγ. Threshold-driven, cross-regulation of C/EBPα and PPARγ lead the cells towards terminal differentiation. In some cases, PPARγ is not the mechanistic trigger towards adipogenesis at all, as reported for example for the flame retardant dechlorane plus (267).
For another example, the antioxidant 2, 4-Di-tert-butylphenol (2,4-DTBP) increased lipid accumulation in hMSCs by activating the PPARγ-RXR heterodimer via RXRα but not directly binding to PPARγ, confirmatory evidence was shown in crystal studies of bound RXR (226). Indeed, other examples are also mediated by RXR, such as TBT, and others require further elucidation, such as phytanic acid. Receptors that also heterodimerise with RXR, such as VDR, can also be affected by RXR binding, and receptor competition for RXR heterodimerisation within a given tissue, is a broader consideration (31).
In relation to the cellular and tissue level, for adipogenesis test method models, whilst the weight of evidence appears to be stronger for the promotion of lipid accumulation in murine in vitro cell models (especially in 3T3-L1 cells), there is greater uncertainty in humans/human in vitro cell models. The adipogenicity of BPA appears to be particularly contentious. Whilst on balance, BPA is clearly positive in 3T3-L1 cells, in human primary pre-adipocytes, it is not a PPARγ agonist. Whilst BPA and BPS both induce adipogenesis, the results from Boucher et al. (88), show that BPS affects adipose specific transcriptional changes earlier than seen for BPA, and alters the expression of genes specifically related to adipogenesis and lipid metabolism (88).
Importantly, one study (89) found that in murine 3T3-derived cells, lipid accumulation was not observed without application of the “induction cocktail” in vitro (this was not investigated in other studies). Indeed Longo et al. and Kossotis et al. (41, 89), suggest that overall, the murine 3T3-L1 cell line is a useful but less relevant model for human adipogenesis, as mechanistically, they are already initiated/committed to the adipocyte lineage, and there is variability across batches and reproducibility issues between laboratory strains.
With respect to regulatory relevance for human health, for the evaluation of potential obesity related hazard(s), the essential points for clarification include the differences reported for different adipogenesis cell models in particular. These are most likely also explained by differences in initiated states and different protocols and induction methods. Independent HTS assessments of data generated by ToxCast and Tox21 in relation to metabolic disruption have found these to be inadequate with respect to reliability (160), and whilst there is great utility in HTS for the purposes of prescreening, for (pre)validation purposes with respect to the objective of the current exercise, they were not considered to contribute substantially to the WoE. However subsequent independent assessments are reported to improve ToxPi predictability (174), but as explained in section 2.4 this 3T3-L1 data could only be utilised as supporting information (160).
This exercise has been one of the more challenging with respect to recommendations for the selection of chemicals for test methods that are intended for a metabolic disruption IATA, here in relation to obesity, compared to single endpoints. Overall, a reasonable number of negatives and potential reference/proficiency chemicals with a range of activity have been elucidated from the literature and recommendations for (pre-)validation purposes of the individual test methods can be made, but also with consideration of their inclusion in an IATA. To that end, chemicals from the list generated herein are being selected and implemented in pre-validation studies of hPPARα and hPPARγ transactivation assays (59) and hMSC cells in the GOLIATH project. Further work is being taken up within other related projects, such as PARC, in relation to for example the testing of the proposed chemicals in a higher throughput 96 well format for the hMSC test method, and will be proposed in relevant follow-up projects, also with the intention of contributing to the OECD detailed review paper on metabolic disruption underway. Other relevant mechanisms, such as retinoid and PPARδ-dependent neurite outgrowth assay and hGR activation, are being (pre) validated by the PEPPER platform. The intention is that these test methods can ultimately be proposed as draft TGs, to be included in IATAs and ultimately define approaches, when fully validated in the future.
Collectively this work will contribute to the development of the OECD TG NAM toolbox to assess the potential chemical hazards from obesogenic chemicals. By contributing to a better mechanistic understanding of the contribution of chemical toxicity to the global obesity epidemic, we will also be able to support the development of safer-by-design chemicals and the future development of relevant endocrine modality chemical regulations, to better protect public health.
EO: Data curation, Investigation, Writing – original draft, Writing – review & editing. BK: Data curation, Investigation, Methodology, Writing – original draft, Writing – review & editing. MJ: Conceptualization, Data curation, Funding acquisition, Investigation, Methodology, Project administration, Resources, Supervision, Validation, Visualization, Writing – original draft, Writing – review & editing.
The author(s) declare financial support was received for the research, authorship, and/or publication of this article. This project has received funding from the European Union’s Horizon 2020 research and innovation programme under grant agreement No. 825489 "GOLIATH project". The views presented in this publication reflect only the author’s view and the European Commission/funding source is not responsible for any use that may be made of the information it contains.
Constructive comments and feedback on the initial chemical selection from Susan Laws (US EPA, retired), and Michael G. Wade (Health Canada) are gratefully acknowledged.
The authors declare that the research was conducted in the absence of any commercial or financial relationships that could be construed as a potential conflict of interest.
All claims expressed in this article are solely those of the authors and do not necessarily represent those of their affiliated organizations, or those of the publisher, the editors and the reviewers. Any product that may be evaluated in this article, or claim that may be made by its manufacturer, is not guaranteed or endorsed by the publisher.
The Supplementary Material for this article can be found online at: https://www.frontiersin.org/articles/10.3389/fendo.2024.1401120/full#supplementary-material.
Supplementary Material 1: Table 1. | Details on PubMed literature search and its results (date: 2020 and Dec 2023).
Supplementary Material 2: Table 1. | Detailed overview of the long list of literature considered with respect to the chemical selection for the pre-validation of the hPPARα, hPPARγ transactivation and hMSC adipogenesis test methods.
Supplementary Material 2: Table 2. | Summary of other cell types and non-selected chemicals for the pre-validation of the hPPARα, hPPARγ transactivation, and hMSC adipogenesis test methods.
1. Boutari C, Mantzoros CS. A 2022 update on the epidemiology of obesity and a call to action: as its twin COVID-19 pandemic appears to be receding, the obesity and dysmetabolism pandemic continues to rage on. Metabolism. (2022) 133:155217. doi: 10.1016/j.metabol.2022.155217
2. Heindel JJ, Lustig RH, Howard S, Corkey BE. Obesogens: a unifying theory for the global rise in obesity. Int J Obes (Lond). (2024) 48:449–60. doi: 10.1038/s41366-024-01460-3
3. WHO. Noncommunicable diseases 2023. Available online at: https://www.who.int/news-room/fact-sheets/detail/noncommunicable-diseases (Accessed 16 September 2023).
4. Jacobs MN, Colacci A, Corvi R, Vaccari M, Aguila MC, Corvaro M, et al. Chemical carcinogen safety testing: OECD expert group international consensus on the development of an integrated approach for the testing and assessment of chemical non-genotoxic carcinogens. Arch Toxicol. (2020) 94:2899–923. doi: 10.1007/s00204-020-02784-5
5. Heindel JJ, Blumberg B, Cave M, Machtinger R, Mantovani A, Mendez MA, et al. Metabolism disrupting chemicals and metabolic disorders. Reprod Toxicol. (2017) 68:3–33. doi: 10.1016/j.reprotox.2016.10.001
6. Legler J, Fletcher T, Govarts E, Porta M, Blumberg B, Heindel JJ, et al. Obesity, diabetes, and associated costs of exposure to endocrine-disrupting chemicals in the European Union. J Clin Endocrinol Metab. (2015) 100:1278–88. doi: 10.1210/jc.2014-4326
7. Legler J, Zalko D, Jourdan F, Jacobs M, Fromenty B, Balaguer P, et al. The GOLIATH project: towards an internationally harmonised approach for testing metabolism disrupting compounds. Int J Mol Sci. (2020) 21. doi: 10.3390/ijms21103480
8. Heath L, Jebb SA, Aveyard P, Piernas C. Obesity, metabolic risk and adherence to healthy lifestyle behaviours: prospective cohort study in the UK Biobank. BMC Med. (2022) 20:65. doi: 10.1186/s12916-022-02236-0
9. Trasande L, Zoeller RT, Hass U, Kortenkamp A, Grandjean P, Myers JP, et al. Estimating burden and disease costs of exposure to endocrine-disrupting chemicals in the European union. J Clin Endocrinol Metab. (2015) 100:1245–55. doi: 10.1210/jc.2014-4324
10. Küblbeck J, Vuorio T, Niskanen J, Fortino V, Braeuning A, Abass K, et al. The EDCMET project: metabolic effects of endocrine disruptors. Int J Mol Sci. (2020) 21. doi: 10.3390/ijms21083021
11. Audouze K, Sarigiannis D, Alonso-Magdalena P, Brochot C, Casas M, Vrijheid M, et al. Integrative strategy of testing systems for identification of endocrine disruptors inducing metabolic disorders-an introduction to the OBERON project. Int J Mol Sci. (2020) 21. doi: 10.3390/ijms21082988
12. Kubickova B, Jacobs MN. Development of a reference and proficiency chemical list for human steatosis endpoints in vitro. Front Endocrinol (Lausanne). (2023) 14:1126880. doi: 10.3389/fendo.2023.1126880
13. Jacobs MN, Kubickova B, Boshoff E. Candidate proficiency test chemicals to address industrial chemical applicability domains for in vitro human cytochrome P450 enzyme induction. Front Toxicol. (2022) 4:880818. doi: 10.3389/ftox.2022.880818
14. Singh R, Barrios A, Dirakvand G, Pervin S. Human brown adipose tissue and metabolic health: potential for therapeutic avenues. Cells. (2021) 10. doi: 10.20944/preprints202110.0005.v1
15. Seale P, Lazar MA. Brown fat in humans: turning up the heat on obesity. Diabetes. (2009) 58:1482–4. doi: 10.2337/db09-0622
16. Wang L, Waltenberger B, Pferschy-Wenzig EM, Blunder M, Liu X, Malainer C, et al. Natural product agonists of peroxisome proliferator-activated receptor gamma (PPARγ): a review. Biochem Pharmacol. (2014) 92:73–89. doi: 10.1016/j.bcp.2014.07.018
17. Chawla A, Repa JJ, Evans RM, Mangelsdorf DJ. Nuclear receptors and lipid physiology: opening the X-files. Science. (2001) 294:1866–70. doi: 10.1126/science.294.5548.1866
18. Kliewer SA, Lehmann JM, Milburn MV, Willson TM. The PPARs and PXRs: nuclear xenobiotic receptors that define novel hormone signaling pathways. Recent Prog Horm Res. (1999) 54:345–67; discussion 67–8.
19. Uauy R, Mena P, Rojas C. Essential fatty acids in early life: structural and functional role. Proc Nutr Soc. (2000) 59:3–15. doi: 10.1017/S0029665100000021
20. Bar-Tana J. Peroxisome proliferator-activated receptor gamma (PPARgamma) activation and its consequences in humans. Toxicol Lett. (2001) 120:9–19. doi: 10.1016/S0378-4274(01)00302-2
21. Kato M, Kusumi T, Tsuchida S, Tanaka M, Sasaki M, Kudo H. Induction of differentiation and peroxisome proliferator-activated receptor gamma expression in colon cancer cell lines by troglitazone. J Cancer Res Clin Oncol. (2004) 130:73–9. doi: 10.1007/s00432-003-0510-2
22. Memon RA, Tecott LH, Nonogaki K, Beigneux A, Moser AH, Grunfeld C, et al. Up-regulation of peroxisome proliferator-activated receptors (PPAR-alpha) and PPAR-gamma messenger ribonucleic acid expression in the liver in murine obesity: troglitazone induces expression of PPAR-gamma-responsive adipose tissue-specific genes in the liver of obese diabetic mice. Endocrinology. (2000) 141:4021–31. doi: 10.1210/en.141.11.4021
23. Liu Y, Colby JK, Zuo X, Jaoude J, Wei D, Shureiqi I. The role of PPAR-δ in metabolism, inflammation, and cancer: many characters of a critical transcription factor. Int J Mol Sci. (2018) 19. doi: 10.3390/ijms19113339
24. Da'adoosh B, Marcus D, Rayan A, King F, Che J, Goldblum A. Discovering highly selective and diverse PPAR-delta agonists by ligand based machine learning and structural modeling. Sci Rep. (2019) 9:1106. doi: 10.1038/s41598-019-38508-8
25. Evans RM, Barish GD, Wang YX. PPARs and the complex journey to obesity. Nat Med. (2004) 10:355–61. doi: 10.1038/nm1025
26. Jacobs MN, Colacci A, Louekari K, Luijten M, Hakkert BC, Paparella M, et al. International regulatory needs for development of an IATA for non-genotoxic carcinogenic chemical substances. Altex. (2016) 33:359–92. doi: 10.14573/altex
27. Ahmadian M, Suh JM, Hah N, Liddle C, Atkins AR, Downes M, et al. PPARγ signaling and metabolism: the good, the bad and the future. Nat Med. (2013) 19:557–66. doi: 10.1038/nm.3159
28. Forman BM, Chen J, Evans RM. Hypolipidemic drugs, polyunsaturated fatty acids, and eicosanoids are ligands for peroxisome proliferator-activated receptors alpha and delta. Proc Natl Acad Sci U S A. (1997) 94:4312–7. doi: 10.1073/pnas.94.9.4312
29. Kersten S. Integrated physiology and systems biology of PPARα. Mol Metab. (2014) 3:354–71. doi: 10.1016/j.molmet.2014.02.002
30. Grygiel-Górniak B. Peroxisome proliferator-activated receptors and their ligands: nutritional and clinical implications–a review. Nutr J. (2014) 13:17. doi: 10.1186/1475-2891-13-17
31. Jacobs MN, Lewis DF. Steroid hormone receptors and dietary ligands: a selected review. Proc Nutr Soc. (2002) 61:105–22. doi: 10.1079/PNS2001140
32. McMullen PD, Bhattacharya S, Woods CG, Sun B, Yarborough K, Ross SM, et al. A map of the PPARα transcription regulatory network for primary human hepatocytes. Chem Biol Interact. (2014) 209:14–24. doi: 10.1016/j.cbi.2013.11.006
33. Kroker AJ, Bruning JB. Review of the structural and dynamic mechanisms of PPARγ Partial agonism. PPAR Res. (2015) 2015:816856. doi: 10.1155/2015/816856
34. Bahmad HF, Daouk R, Azar J, Sapudom J, Teo JCM, Abou-Kheir W, et al. Modeling adipogenesis: current and future perspective. Cells. (2020) 9. doi: 10.3390/cells9102326
35. Scheja L, Heeren J. The endocrine function of adipose tissues in health and cardiometabolic disease. Nat Rev Endocrinol. (2019) 15:507–24. doi: 10.1038/s41574-019-0230-6
36. Hu L, Yin C, Zhao F, Ali A, Ma J, Qian A. Mesenchymal stem cells: cell fate decision to osteoblast or adipocyte and application in osteoporosis treatment. Int J Mol Sci. (2018) 19. doi: 10.3390/ijms19020360
37. Matsushita K, Dzau VJ. Mesenchymal stem cells in obesity: insights for translational applications. Lab Invest. (2017) 97:1158–66. doi: 10.1038/labinvest.2017.42
38. Jakab J, Miškić B, Mikšić Š, Juranić B, Ćosić V, Schwarz D, et al. Adipogenesis as a potential anti-obesity target: A review of pharmacological treatment and natural products. Diabetes Metab Syndr Obes. (2021) 14:67–83. doi: 10.2147/DMSO.S281186
39. Zhao X, Hu H, Wang C, Bai L, Wang Y, Wang W, et al. A comparison of methods for effective differentiation of the frozen-thawed 3T3-L1 cells. Anal Biochem. (2019) 568:57–64. doi: 10.1016/j.ab.2018.12.020
40. Foley B, Doheny DL, Black MB, Pendse SN, Wetmore BA, Clewell RA, et al. Editor's highlight: screening toxCast prioritized chemicals for PPARG function in a human adipose-derived stem cell model of adipogenesis. Toxicol Sci. (2017) 155:85–100. doi: 10.1093/toxsci/kfw186
41. Kassotis CD, Vom Saal FS, Babin PJ, Lagadic-Gossmann D, Le Mentec H, Blumberg B, et al. Obesity III: Obesogen assays: Limitations, strengths, and new directions. Biochem Pharmacol. (2022) 199:115014. doi: 10.1016/j.bcp.2022.115014
42. Asada M, Rauch A, Shimizu H, Maruyama H, Miyaki S, Shibamori M, et al. DNA binding-dependent glucocorticoid receptor activity promotes adipogenesis via Krüppel-like factor 15 gene expression. Lab Invest. (2011) 91:203–15. doi: 10.1038/labinvest.2010.170
43. Nicolaides NC, Chrousos G, Kino T. Glucocorticoid receptor. In: Feingold KR, Anawalt B, Blackman MR, Boyce A, Chrousos G, Corpas E, et al, editors. Endotext [Internet]. South Dartmouth (MA): MDText.com, Inc. (2020).
44. OECD. Detailed Review Paper on the State of the Science on Novel In Vitro and In Vivo Screening and Testing Methods and Endpoints for Evaluating Endocrine Disruptors 2012. Available online at: https://www.oecd-ilibrary.org/content/publication/9789264221352-en.
45. Bopp S, Nepelska M, Halder M, Munn S. Expert survey on identification of gaps in available test methods for evaluation of endocrine disruptors. Luxembourg: JRC Technical Reports European Union (2017).
46. European C, Directorate-General for E. Setting priorities for further development and validation of test methods and testing approaches for evaluating endocrine disruptors – Final report: Publications Office. Luxembourg, Publications Office of the European Union (2018).
47. Jacobs MN, Ezendam J, Hakkert B, Oelgeschlaeger M. Potential of concentration-response data to broaden regulatory application of in vitro test guidelines. Altex. (2022) 39:315–21. doi: 10.14573/altex
48. OECD. Guidance document on the validation and international acceptance of new or updated test methods for hazard assessment (2005). Available online at: https://ntp.niehs.nih.gov/sites/default/files/iccvam/suppdocs/feddocs/oecd/oecd-gd34.pdf.
49. OECD. Guidance Document on Good In Vitro Method Practices (GIVIMP) (2018). Available online at: https://www.oecd-ilibrary.org/content/publication/9789264304796-en.
50. OECD. Report of the Workshop on a Framework for the Development and Use Of Integrated Approaches to Testing and Assessment (2017). Available online at: https://www.oecd-ilibrary.org/content/publication/9789264274747-en.
51. Zuang V, Dura A, Ahs Lopez E, Barroso J, Batista Leite S, Berggren E, et al. Non-animal Methods in Science and Regulation. Luxembourg: Publications Office of the European Union (2022).
52. Jacobs MN, Hoffmann S, Hollnagel HM, Kern P, Kolle SN, Natsch A, et al. Avoiding a reproducibility crisis in regulatory toxicology-on the fundamental role of ring trials. Arch Toxicol. (2024) 98:2047–63. doi: 10.1007/s00204-024-03736-z
53. Wang M, Winneroski LL, Ardecky RJ, Babine RE, Brooks DA, Etgen GJ, et al. Conversion of human-selective PPARalpha agonists to human/mouse dual agonists: a molecular modeling analysis. Bioorg Med Chem Lett. (2004) 14:6113–6. doi: 10.1016/j.bmcl.2004.09.031
54. Ding TT, Liu YY, Zhang LM, Shi JR, Xu WR, Li SY, et al. Exploring dual agonists for PPARα/γ Receptors using pharmacophore modeling, docking analysis and molecule dynamics simulation. Comb Chem High Throughput Screen. (2022) 25:1450–61. doi: 10.2174/1386207324666210628114216
55. Li Y, Ks N, Byran G, Krishnamurthy PT. Identification of selective PPAR-γ Modulators by combining pharmacophore modeling, molecular docking, and adipogenesis assay. Appl Biochem Biotechnol. (2023) 195:1014–41. doi: 10.1007/s12010-022-04190-2
56. Al Sharif M, Tsakovska I, Alov P, Vitcheva V, Diukendjieva A, Pajeva I. Molecular modeling approach to study the PPARγ-ligand interactions. Methods Mol Biol. (2019) 1966:261–89. doi: 10.1007/978-1-4939-9195-2_22
57. Lewis DF, Jacobs MN, Dickins M, Lake BG. Molecular modelling of the peroxisome proliferator-activated receptor alpha (PPAR alpha) from human, rat and mouse, based on homology with the human PPAR gamma crystal structure. Toxicol In Vitro. (2002) 16:275–80. doi: 10.1016/S0278-6915(02)00049-2
58. Tsakovska I, Al Sharif M, Alov P, Diukendjieva A, Fioravanzo E, Cronin MT, et al. Molecular modelling study of the PPARγ receptor in relation to the mode of action/adverse outcome pathway framework for liver steatosis. Int J Mol Sci. (2014) 15:7651–66. doi: 10.3390/ijms15057651
59. Garoche C, Boulahtouf A, Grimaldi M, Chiavarina B, Toporova L, den Broeder MJ, et al. Interspecies differences in activation of peroxisome proliferator-activated receptor γ by pharmaceutical and environmental chemicals. Environ Sci Technol. (2021) 55:16489–501. doi: 10.1021/acs.est.1c04318
60. Chandalia A, Clarke HJ, Clemens LE, Pandey B, Vicena V, Lee P, et al. MBX-102/JNJ39659100, a novel non-TZD selective partial PPAR-γ agonist lowers triglyceride independently of PPAR-α activation. PPAR Res. (2009) 2009:706852. doi: 10.1155/2009/706852
61. Forman BM, Tontonoz P, Chen J, Brun RP, Spiegelman BM, Evans RM. 15-Deoxy-delta 12, 14-prostaglandin J2 is a ligand for the adipocyte determination factor PPAR gamma. Cell. (1995) 83:803–12. doi: 10.1016/0092-8674(95)90193-0
62. Kim S, Reed E, Monti S, Schlezinger JJ. A data-driven transcriptional taxonomy of adipogenic chemicals to identify white and brite adipogens. Environ Health Perspect. (2021) 129:77006. doi: 10.1289/EHP6886
63. Wang WL, Welsh J, Tenniswood M. 1,25-Dihydroxyvitamin D3 modulates lipid metabolism in prostate cancer cells through miRNA mediated regulation of PPARA. J Steroid Biochem Mol Biol. (2013) 136:247–51. doi: 10.1016/j.jsbmb.2012.09.033
64. Salehpour A, Hosseinpanah F, Shidfar F, Vafa M, Razaghi M, Dehghani S, et al. A 12-week double-blind randomized clinical trial of vitamin D3 supplementation on body fat mass in healthy overweight and obese women. Nutr J. (2012) 11:78. doi: 10.1186/1475-2891-11-78
65. Pannu PK, Zhao Y, Soares MJ. Reductions in body weight and percent fat mass increase the vitamin D status of obese subjects: a systematic review and metaregression analysis. Nutr Res. (2016) 36:201–13. doi: 10.1016/j.nutres.2015.11.013
66. Rock CL, Emond JA, Flatt SW, Heath DD, Karanja N, Pakiz B, et al. Weight loss is associated with increased serum 25-hydroxyvitamin D in overweight or obese women. Obes (Silver Spring). (2012) 20:2296–301. doi: 10.1038/oby.2012.57
67. LeBlanc ES, Rizzo JH, Pedula KL, Ensrud KE, Cauley J, Hochberg M, et al. Associations between 25-hydroxyvitamin D and weight gain in elderly women. J Womens Health (Larchmt). (2012) 21:1066–73. doi: 10.1089/jwh.2012.3506
68. Chang E, Kim Y. Vitamin D decreases adipocyte lipid storage and increases NAD-SIRT1 pathway in 3T3-L1 adipocytes. Nutrition. (2016) 32:702–8. doi: 10.1016/j.nut.2015.12.032
69. Kim JH, Kang S, Jung YN, Choi HS. Cholecalciferol inhibits lipid accumulation by regulating early adipogenesis in cultured adipocytes and zebrafish. Biochem Biophys Res Commun. (2016) 469:646–53. doi: 10.1016/j.bbrc.2015.12.049
70. Szymczak-Pajor I, Miazek K, Selmi A, Balcerczyk A, Śliwińska A. The action of vitamin D in adipose tissue: is there the link between vitamin D deficiency and adipose tissue-related metabolic disorders? Int J Mol Sci. (2022) 23. doi: 10.3390/ijms23020956
71. Marino M, Venturi S, Del Bo C, Møller P, Riso P, Porrini M. Vitamin D counteracts lipid accumulation, augments free fatty acid-induced ABCA1 and CPT-1A expression while reducing CD36 and C/EBPβ Protein levels in monocyte-derived macrophages. Biomedicines. (2022) 10. doi: 10.3390/biomedicines10040775
72. Park CY, Han SN. The role of vitamin D in adipose tissue biology: adipocyte differentiation, energy metabolism, and inflammation. J Lipid Atheroscler. (2021) 10:130–44. doi: 10.12997/jla.2021.10.2.130
73. Mutt SJ, Hyppönen E, Saarnio J, Järvelin M-R, Herzig K-H. Vitamin D and adipose tissue—more than storage. Front Physiol. (2014) 5. doi: 10.3389/fphys.2014.00228
74. Salehpour A, Hedayati M, Shidfar F, Neshatbini Tehrani A, Farshad AA, Mohammadi S. 1,25-Dihydroxyvitamin D3 modulates adipogenesis of human adipose-derived mesenchymal stem cells dose-dependently. Nutr Metab (Lond). (2021) 18:29. doi: 10.1186/s12986-021-00561-4
75. Dantas JR, Araújo DB, Silva KR, Souto DL, de Fátima Carvalho Pereira M, Luiz RR, et al. Adipose tissue-derived stromal/stem cells + cholecalciferol: a pilot study in recent-onset type 1 diabetes patients. Arch Endocrinol Metab. (2021) 65:342–51. doi: 10.20945/2359-3997000000368
76. Zoico E, Franceschetti G, Chirumbolo S, Rossi AP, Mazzali G, Rizzatti V, et al. Phenotypic shift of adipocytes by cholecalciferol and 1α,25 dihydroxycholecalciferol in relation to inflammatory status and calcium content. Endocrinology. (2014) 155:4178–88. doi: 10.1210/en.2013-1969
77. Bonnet L, Karkeni E, Couturier C, Astier J, Dalifard J, Defoort C, et al. Gene expression pattern in response to cholecalciferol supplementation highlights cubilin as a major protein of 25(OH)D uptake in adipocytes and male mice white adipose tissue. Endocrinology. (2018) 159:957–66. doi: 10.1210/en.2017-00650
78. Sneve M, Figenschau Y, Jorde R. Supplementation with cholecalciferol does not result in weight reduction in overweight and obese subjects. Eur J Endocrinol. (2008) 159:675–84. doi: 10.1530/EJE-08-0339
79. Dirtu AC, Roosens L, Geens T, Gheorghe A, Neels H, Covaci A. Simultaneous determination of bisphenol A, triclosan, and tetrabromobisphenol A in human serum using solid-phase extraction and gas chromatography-electron capture negative-ionization mass spectrometry. Anal Bioanal Chem. (2008) 391:1175–81. doi: 10.1007/s00216-007-1807-9
80. Kim UJ, Oh JE. Tetrabromobisphenol A and hexabromocyclododecane flame retardants in infant-mother paired serum samples, and their relationships with thyroid hormones and environmental factors. Environ pollut. (2014) 184:193–200. doi: 10.1016/j.envpol.2013.08.034
81. Riu A, Grimaldi M, le Maire A, Bey G, Phillips K, Boulahtouf A, et al. Peroxisome proliferator-activated receptor γ is a target for halogenated analogs of bisphenol A. Environ Health Perspect. (2011) 119:1227–32. doi: 10.1289/ehp.1003328
82. Riu A, McCollum CW, Pinto CL, Grimaldi M, Hillenweck A, Perdu E, et al. Halogenated bisphenol-A analogs act as obesogens in zebrafish larvae (Danio rerio). Toxicol Sci. (2014) 139:48–58. doi: 10.1093/toxsci/kfu036
83. Routti H, Berg MK, Lille-Langøy R, Øygarden L, Harju M, Dietz R, et al. Environmental contaminants modulate the transcriptional activity of polar bear (Ursus maritimus) and human peroxisome proliferator-activated receptor alpha (PPARA). Sci Rep. (2019) 9:6918. doi: 10.1038/s41598-019-43337-w
84. Crowe DL, Chandraratna RA. A retinoid X receptor (RXR)-selective retinoid reveals that RXR-alpha is potentially a therapeutic target in breast cancer cell lines, and that it potentiates antiproliferative and apoptotic responses to peroxisome proliferator-activated receptor ligands. Breast Cancer Res. (2004) 6:R546–55. doi: 10.1186/bcr913
85. Wang J, Bi W, Zhao W, Varghese M, Koch RJ, Walker RH, et al. Selective brain penetrable Nurr1 transactivator for treating Parkinson's disease. Oncotarget. (2016) 7:7469–79. doi: 10.18632/oncotarget.v7i7
86. Kassotis CD, Masse L, Kim S, Schlezinger JJ, Webster TF, Stapleton HM. Characterization of adipogenic chemicals in three different cell culture systems: implications for reproducibility based on cell source and handling. Sci Rep. (2017) 7:42104. doi: 10.1038/srep42104
87. Sharma S, Ahmad S, Khan MF, Parvez S, Raisuddin S. In silico molecular interaction of bisphenol analogues with human nuclear receptors reveals their stronger affinity vs. classical bisphenol A. Toxicol Mech Methods. (2018) 28:660–9. doi: 10.1080/15376516.2018.1491663
88. Boucher JG, Gagné R, Rowan-Carroll A, Boudreau A, Yauk CL, Atlas E. Bisphenol A and bisphenol S induce distinct transcriptional profiles in differentiating human primary preadipocytes. PloS One. (2016) 11:e0163318. doi: 10.1371/journal.pone.0163318
89. Longo M, Zatterale F, Naderi J, Nigro C, Oriente F, Formisano P, et al. Low-dose bisphenol-A promotes epigenetic changes at pparγ Promoter in adipose precursor cells. Nutrients. (2020) 12. doi: 10.3390/nu12113498
90. Drobna Z, Talarovicova A, Schrader HE, Fennell TR, Snyder RW, Rissman EF. Bisphenol F has different effects on preadipocytes differentiation and weight gain in adult mice as compared with Bisphenol A and S. Toxicology. (2019) 420:66–72. doi: 10.1016/j.tox.2019.03.016
91. Pomatto V, Cottone E, Cocci P, Mozzicafreddo M, Mosconi G, Nelson ER, et al. Plasticizers used in food-contact materials affect adipogenesis in 3T3-L1 cells. J Steroid Biochem Mol Biol. (2018) 178:322–32. doi: 10.1016/j.jsbmb.2018.01.014
92. Sargis RM, Johnson DN, Choudhury RA, Brady MJ. Environmental endocrine disruptors promote adipogenesis in the 3T3-L1 cell line through glucocorticoid receptor activation. Obes (Silver Spring). (2010) 18:1283–8. doi: 10.1038/oby.2009.419
93. Taxvig C, Dreisig K, Boberg J, Nellemann C, Schelde AB, Pedersen D, et al. Differential effects of environmental chemicals and food contaminants on adipogenesis, biomarker release and PPARγ activation. Mol Cell Endocrinol. (2012) 361:106–15. doi: 10.1016/j.mce.2012.03.021
94. Wada K, Sakamoto H, Nishikawa K, Sakuma S, Nakajima A, Fujimoto Y, et al. Life style-related diseases of the digestive system: endocrine disruptors stimulate lipid accumulation in target cells related to metabolic syndrome. J Pharmacol Sci. (2007) 105:133–7. doi: 10.1254/jphs.FM0070034
95. Ahmed S, Atlas E. Bisphenol S- and bisphenol A-induced adipogenesis of murine preadipocytes occurs through direct peroxisome proliferator-activated receptor gamma activation. Int J Obes (Lond). (2016) 40:1566–73. doi: 10.1038/ijo.2016.95
96. Angle BM, Do RP, Ponzi D, Stahlhut RW, Drury BE, Nagel SC, et al. Metabolic disruption in male mice due to fetal exposure to low but not high doses of bisphenol A (BPA): evidence for effects on body weight, food intake, adipocytes, leptin, adiponectin, insulin and glucose regulation. Reprod Toxicol. (2013) 42:256–68. doi: 10.1016/j.reprotox.2013.07.017
97. ANSES (French Agency for Food EaOHS. OPINION of the French Agency for Food, Environmental and Occupational Health & Safety in response to the consultation of the European Food Safety Authority on its draft Opinion regarding the assessment of risks to human health related to dietary exposure to Bisphenol A 2014. Available online at: https://www.anses.fr/en/system/files/SUBSTANCES2014sa0033EN.pdf.
98. Ariemma F, D'Esposito V, Liguoro D, Oriente F, Cabaro S, Liotti A, et al. Low-dose bisphenol-A impairs adipogenesis and generates dysfunctional 3T3-L1 adipocytes. PloS One. (2016) 11:e0150762. doi: 10.1371/journal.pone.0150762
99. Atlas E, Pope L, Wade MG, Kawata A, Boudreau A, Boucher JG. Bisphenol A increases aP2 expression in 3T3L1 by enhancing the transcriptional activity of nuclear receptors at the promoter. Adipocyte. (2014) 3:170–9. doi: 10.4161/adip.28436
100. Bastos Sales L, Kamstra JH, Cenijn PH, van Rijt LS, Hamers T, Legler J. Effects of endocrine disrupting chemicals on in vitro global DNA methylation and adipocyte differentiation. Toxicol In Vitro. (2013) 27:1634–43. doi: 10.1016/j.tiv.2013.04.005
101. Biasiotto G, Zanella I, Masserdotti A, Pedrazzani R, Papa M, Caimi L, et al. Municipal wastewater affects adipose deposition in male mice and increases 3T3-L1 cell differentiation. Toxicol Appl Pharmacol. (2016) 297:32–40. doi: 10.1016/j.taap.2016.02.023
102. Biemann R, Navarrete Santos A, Navarrete Santos A, Riemann D, Knelangen J, Blüher M, et al. Endocrine disrupting chemicals affect the adipogenic differentiation of mesenchymal stem cells in distinct ontogenetic windows. Biochem Biophys Res Commun. (2012) 417:747–52. doi: 10.1016/j.bbrc.2011.12.028
103. Boucher JG, Boudreau A, Ahmed S, Atlas E. In vitro effects of bisphenol A β-D-glucuronide (BPA-G) on adipogenesis in human and murine preadipocytes. Environ Health Perspect. (2015) 123:1287–93. doi: 10.1289/ehp.1409143
104. Burkhardt P, Palma-Duran SA, Tuck ARR, Norgren K, Li X, Nikiforova V, et al. Environmental chemicals change extracellular lipidome of mature human white adipocytes. Chemosphere. (2024) 349:140852. doi: 10.1016/j.chemosphere.2023.140852
105. Carwile JL, Michels KB. Urinary bisphenol A and obesity: NHANES 2003–2006. Environ Res. (2011) 111:825–30. doi: 10.1016/j.envres.2011.05.014
106. Chamorro-García R, Kirchner S, Li X, Janesick A, Casey SC, Chow C, et al. Bisphenol A diglycidyl ether induces adipogenic differentiation of multipotent stromal stem cells through a peroxisome proliferator-activated receptor gamma-independent mechanism. Environ Health Perspect. (2012) 120:984–9. doi: 10.1289/ehp.1205063
107. Cimmino I, Fiory F, Perruolo G, Miele C, Beguinot F, Formisano P, et al. Potential mechanisms of bisphenol A (BPA) contributing to human disease. Int J Mol Sci. (2020) 21. doi: 10.3390/ijms21165761
108. Do MT, Chang VC, Mendez MA, de Groh M. Urinary bisphenol A and obesity in adults: results from the Canadian Health Measures Survey. Health Promot Chronic Dis Prev Can. (2017) 37:403–12. doi: 10.24095/hpcdp.37.12.02
109. Dunder L, Lejonklou MH, Lind PM, Lind L. Urinary bisphenol A and serum lipids: a meta-analysis of six NHANES examination cycles (2003–2014). J Epidemiol Community Health. (2019) 73:1012–9. doi: 10.1136/jech-2019-212555
110. EFSA Panel on Food Contact Materials E, Flavourings, Aids P. Scientific Opinion on the risks to public health related to the presence of bisphenol A (BPA) in foodstuffs. EFSA J. (2015) 13:3978. doi: 10.2903/j.efsa.2015.3978
111. Gao P, Wang L, Yang N, Wen J, Zhao M, Su G, et al. Peroxisome proliferator-activated receptor gamma (PPARγ) activation and metabolism disturbance induced by bisphenol A and its replacement analog bisphenol S using in vitro macrophages and in vivo mouse models. Environ Int. (2020) 134:105328. doi: 10.1016/j.envint.2019.105328
112. Heindel JJ, Howard S, Agay-Shay K, Arrebola JP, Audouze K, Babin PJ, et al. Obesity II: Establishing causal links between chemical exposures and obesity. Biochem Pharmacol. (2022) 199:115015. doi: 10.1016/j.bcp.2022.115015
113. Héliès-Toussaint C, Peyre L, Costanzo C, Chagnon MC, Rahmani R. Is bisphenol S a safe substitute for bisphenol A in terms of metabolic function? An in vitro study. Toxicol Appl Pharmacol. (2014) 280:224–35. doi: 10.1016/j.taap.2014.07.025
114. Kassotis CD, Hoffman K, Völker J, Pu Y, Veiga-Lopez A, Kim SM, et al. Reproducibility of adipogenic responses to metabolism disrupting chemicals in the 3T3-L1 pre-adipocyte model system: An interlaboratory study. Toxicology. (2021) 461:152900. doi: 10.1016/j.tox.2021.152900
115. Ko A, Hwang MS, Park JH, Kang HS, Lee HS, Hong JH. Association between urinary bisphenol A and waist circumference in korean adults. Toxicol Res. (2014) 30:39–44. doi: 10.5487/TR.2014.30.1.039
116. Marmugi A, Ducheix S, Lasserre F, Polizzi A, Paris A, Priymenko N, et al. Low doses of bisphenol a induce gene expression related to lipid synthesis and trigger triglyceride accumulation in adult mouse liver. Hepatology. (2012) 55:395–407. doi: 10.1002/hep.24685
117. Menale C, Piccolo MT, Cirillo G, Calogero RA, Papparella A, Mita L, et al. Bisphenol A effects on gene expression in adipocytes from children: association with metabolic disorders. J Mol Endocrinol. (2015) 54:289–303. doi: 10.1530/JME-14-0282
118. Norgren K, Tuck A, Vieira Silva A, Burkhardt P, Öberg M, Munic Kos V. High throughput screening of bisphenols and their mixtures under conditions of low-intensity adipogenesis of human mesenchymal stem cells (hMSCs). Food Chem Toxicol. (2022) 161:112842. doi: 10.1016/j.fct.2022.112842
119. Pereira-Fernandes A, Demaegdt H, Vandermeiren K, Hectors TL, Jorens PG, Blust R, et al. Evaluation of a screening system for obesogenic compounds: screening of endocrine disrupting compounds and evaluation of the PPAR dependency of the effect. PloS One. (2013) 8:e77481. doi: 10.1371/journal.pone.0077481
120. Savastano S, Tarantino G, D'Esposito V, Passaretti F, Cabaro S, Liotti A, et al. Bisphenol-A plasma levels are related to inflammatory markers, visceral obesity and insulin-resistance: a cross-sectional study on adult male population. J Transl Med. (2015) 13:169. doi: 10.1186/s12967-015-0532-y
121. Schaffert A, Krieg L, Weiner J, Schlichting R, Ueberham E, Karkossa I, et al. Alternatives for the worse: Molecular insights into adverse effects of bisphenol a and substitutes during human adipocyte differentiation. Environ Int. (2021) 156:106730. doi: 10.1016/j.envint.2021.106730
122. Shankar A, Teppala S, Sabanayagam C. Urinary bisphenol a levels and measures of obesity: results from the national health and nutrition examination survey 2003–2008. ISRN Endocrinol. (2012) 2012:965243. doi: 10.5402/2012/965243
123. Teppala S, Madhavan S, Shankar A. Bisphenol A and metabolic syndrome: results from NHANES. Int J Endocrinol. (2012) 2012:598180. doi: 10.1155/2012/598180
124. Valentino R, D'Esposito V, Passaretti F, Liotti A, Cabaro S, Longo M, et al. Bisphenol-A impairs insulin action and up-regulates inflammatory pathways in human subcutaneous adipocytes and 3T3-L1 cells. PloS One. (2013) 8:e82099. doi: 10.1371/journal.pone.0082099
125. Veiga-Lopez A, Kannan K, Liao C, Ye W, Domino SE, Padmanabhan V. Gender-specific effects on gestational length and birth weight by early pregnancy BPA exposure. J Clin Endocrinol Metab. (2015) 100:E1394–403. doi: 10.1210/jc.2015-1724
126. Verbanck M, Canouil M, Leloire A, Dhennin V, Coumoul X, Yengo L, et al. Low-dose exposure to bisphenols A, F and S of human primary adipocyte impacts coding and non-coding RNA profiles. PloS One. (2017) 12:e0179583. doi: 10.1371/journal.pone.0179583
127. Wang L, Asimakopoulos AG, Kannan K. Accumulation of 19 environmental phenolic and xenobiotic heterocyclic aromatic compounds in human adipose tissue. Environ Int. (2015) 78:45–50. doi: 10.1016/j.envint.2015.02.015
128. Eskenazi B, Harley K, Bradman A, Weltzien E, Jewell NP, Barr DB, et al. Association of in utero organophosphate pesticide exposure and fetal growth and length of gestation in an agricultural population. Environ Health Perspect. (2004) 112:1116–24. doi: 10.1289/ehp.6789
129. Hoogduijn MJ, Rakonczay Z, Genever PG. The effects of anticholinergic insecticides on human mesenchymal stem cells. Toxicol Sci. (2006) 94:342–50. doi: 10.1093/toxsci/kfl101
130. Li J, Ren F, Li Y, Luo J, Pang G. Chlorpyrifos induces metabolic disruption by altering levels of reproductive hormones. J Agric Food Chem. (2019) 67:10553–62. doi: 10.1021/acs.jafc.9b03602
131. Trunnelle KJ, Bennett DH, Tulve NS, Clifton MS, Davis MD, Calafat AM, et al. Urinary pyrethroid and chlorpyrifos metabolite concentrations in Northern California families and their relationship to indoor residential insecticide levels, part of the Study of Use of Products and Exposure Related Behavior (SUPERB). Environ Sci Technol. (2014) 48:1931–9. doi: 10.1021/es403661a
132. Prueitt RL, Goodman JE, Bailey LA, Rhomberg LR. Hypothesis-based weight-of-evidence evaluation of the neurodevelopmental effects of chlorpyrifos. Crit Rev Toxicology. (2011) 41:822–903. doi: 10.3109/10408444.2011.616877
133. WHO. Joint FAO/WHO Meeting on Pesticide Residues, Pesticide residues in food 2006: WHO Food and Agriculture Organization of the United Nations (2006). Available online at: https://www.fao.org/fileadmin/templates/agphome/documents/Pests_Pesticides/JMPR/report2006jmpr.pdf.
134. Persistent Organic Pollutants Review Committee (POPRC). Call for information and follow-up to the eighteenth meeting of the Persistent Organic Pollutants Review Committee (2022). Available online at: https://www.pops.int/TheConvention/POPsReviewCommittee/Meetings/POPRC18/POPRC18Followup/tabid/9352/Default.aspx.
135. EFSA (European Food Safety Authority). Statement on the availableoutcomes of the human health assessment in the context of the pesticides peer review of the activesubstance chlorpyrifos. EFSA Journal (2019) 17:5809. doi: 10.2903/j.efsa.2019.5809
136. Mehendale HM. PPAR-alpha: a key to the mechanism of hepatoprotection by clofibrate. Toxicol Sci. (2000) 57:187–90. doi: 10.1093/toxsci/57.2.187
137. Tahri-Joutey M, Andreoletti P, Surapureddi S, Nasser B, Cherkaoui-Malki M, Latruffe N. Mechanisms mediating the regulation of peroxisomal fatty acid beta-oxidation by PPARα. Int J Mol Sci. (2021) 22. doi: 10.3390/ijms22168969
138. Qiu YY, Zhang J, Zeng FY, Zhu YZ. Roles of the peroxisome proliferator-activated receptors (PPARs) in the pathogenesis of nonalcoholic fatty liver disease (NAFLD). Pharmacol Res. (2023) 192:106786. doi: 10.1016/j.phrs.2023.106786
139. Lalloyer F, Staels B. Fibrates, glitazones, and peroxisome proliferator-activated receptors. Arterioscler Thromb Vasc Biol. (2010) 30:894–9. doi: 10.1161/ATVBAHA.108.179689
140. Bougarne N, Weyers B, Desmet SJ, Deckers J, Ray DW, Staels B, et al. Molecular actions of PPARα in lipid metabolism and inflammation. Endocr Rev. (2018) 39:760–802. doi: 10.1210/er.2018-00064
141. Oliver M. The clofibrate saga: a retrospective commentary. Br J Clin Pharmacol. (2012) 74:907–10. doi: 10.1111/j.1365-2125.2012.04282.x
142. Grundy SM, Ahrens EH Jr., Salen G, Schreibman PH, Nestel PJ. Mechanisms of action of clofibrate on cholesterol metabolism in patients with hyperlipidemia. J Lipid Res. (1972) 13:531–51. doi: 10.1016/S0022-2275(20)39388-3
143. Staels B, Dallongeville J, Auwerx J, Schoonjans K, Leitersdorf E, Fruchart JC. Mechanism of action of fibrates on lipid and lipoprotein metabolism. Circulation. (1998) 98:2088–93. doi: 10.1161/01.CIR.98.19.2088
144. Yokoyama Y, Xin B, Shigeto T, Umemoto M, Kasai-Sakamoto A, Futagami M, et al. Clofibric acid, a peroxisome proliferator-activated receptor alpha ligand, inhibits growth of human ovarian cancer. Mol Cancer Ther. (2007) 6:1379–86. doi: 10.1158/1535-7163.MCT-06-0722
145. Kim J, Sun Q, Yue Y, Yoon KS, Whang KY, Marshall Clark J, et al. 4,4'-Dichlorodiphenyltrichloroethane (DDT) and 4,4'-dichlorodiphenyldichloroethylene (DDE) promote adipogenesis in 3T3-L1 adipocyte cell culture. Pestic Biochem Physiol. (2016) 131:40–5. doi: 10.1016/j.pestbp.2016.01.005
146. Mangum LH, Howell GE 3rd, Chambers JE. Exposure to p,p'-DDE enhances differentiation of 3T3-L1 preadipocytes in a model of sub-optimal differentiation. Toxicol Lett. (2015) 238:65–71. doi: 10.1016/j.toxlet.2015.07.009
147. Burns JS, Williams PL, Korrick SA, Hauser R, Sergeyev O, Revich B, et al. Association between chlorinated pesticides in the serum of prepubertal Russian boys and longitudinal biomarkers of metabolic function. Am J Epidemiol. (2014) 180:909–19. doi: 10.1093/aje/kwu212
148. Liu Q, Wang Q, Xu C, Shao W, Zhang C, Liu H, et al. Organochloride pesticides impaired mitochondrial function in hepatocytes and aggravated disorders of fatty acid metabolism. Sci Rep. (2017) 7:46339. doi: 10.1038/srep46339
149. Cai A, Portengen L, Govarts E, Martin LR, Schoeters G, Legler J, et al. Prenatal exposure to persistent organic pollutants and changes in infant growth and childhood growth trajectories. Chemosphere. (2023) 314:137695. doi: 10.1016/j.chemosphere.2022.137695
150. Pesta M, Cedikova M, Dvorak P, Dvorakova J, Kulda V, Srbecka K, et al. Trends in gene expression changes during adipogenesis in human adipose derived mesenchymal stem cells under dichlorodiphenyldichloroethylene exposure. Mol Cell Toxicology. (2018) 14:369–79. doi: 10.1007/s13273-018-0041-1
151. Jugan J, Lind PM, Salihovic S, Stubleski J, Kärrman A, Lind L, et al. The associations between p,p'-DDE levels and plasma levels of lipoproteins and their subclasses in an elderly population determined by analysis of lipoprotein content. Lipids Health Dis. (2020) 19:249. doi: 10.1186/s12944-020-01417-1
152. Salihovic S, Ganna A, Fall T, Broeckling CD, Prenni JE, van Bavel B, et al. The metabolic fingerprint of p,p′-DDE and HCB exposure in humans. Environ Int. (2016) 88:60–6. doi: 10.1016/j.envint.2015.12.015
153. Rolle-Kampczyk U, Gebauer S, Haange SB, Schubert K, Kern M, Moulla Y, et al. Accumulation of distinct persistent organic pollutants is associated with adipose tissue inflammation. Sci Total Environ. (2020) 748:142458. doi: 10.1016/j.scitotenv.2020.142458
154. Liang Y, Liu D, Zhan J, Luo M, Han J, Wang P, et al. New insight into the mechanism of POP-induced obesity: Evidence from DDE-altered microbiota. Chemosphere. (2020) 244:125123. doi: 10.1016/j.chemosphere.2019.125123
155. Vannice G, Rasmussen H. Position of the academy of nutrition and dietetics: dietary fatty acids for healthy adults. J Acad Nutr Diet. (2014) 114:136–53. doi: 10.1016/j.jand.2013.11.001
156. Kim HK, Della-Fera M, Lin J, Baile CA. Docosahexaenoic acid inhibits adipocyte differentiation and induces apoptosis in 3T3-L1 preadipocytes. J Nutr. (2006) 136:2965–9. doi: 10.1093/jn/136.12.2965
157. Félix-Soriano E, Sáinz N, Fernández-Galilea M, Gil-Iturbe E, Celay J, Martínez-Climent JA, et al. Chronic docosahexaenoic acid supplementation improves metabolic plasticity in subcutaneous adipose tissue of aged obese female mice. J Nutr Biochem. (2023) 111:109153. doi: 10.1016/j.jnutbio.2022.109153
158. Neff LM, Culiner J, Cunningham-Rundles S, Seidman C, Meehan D, Maturi J, et al. Algal docosahexaenoic acid affects plasma lipoprotein particle size distribution in overweight and obese adults. J Nutr. (2011) 141:207–13. doi: 10.3945/jn.110.130021
159. Jacobs MN, Janssens W, Bernauer U, Brandon E, Coecke S, Combes R, et al. The use of metabolising systems for in vitro testing of endocrine disruptors. Curr Drug Metab. (2008) 9:796–826. doi: 10.2174/138920008786049294
160. Janesick AS, Dimastrogiovanni G, Vanek L, Boulos C, Chamorro-García R, Tang W, et al. On the utility of toxCast™ and toxPi as methods for identifying new obesogens. Environ Health Perspect. (2016) 124:1214–26. doi: 10.1289/ehp.1510352
161. Briand F, Tréguier M, André A, Grillot D, Issandou M, Ouguerram K, et al. Liver X receptor activation promotes macrophage-to-feces reverse cholesterol transport in a dyslipidemic hamster model. J Lipid Res. (2010) 51:763–70. doi: 10.1194/jlr.M001552
162. Archer A, Stolarczyk E, Doria ML, Helguero L, Domingues R, Howard JK, et al. LXR activation by GW3965 alters fat tissue distribution and adipose tissue inflammation in ob/ob female mice. J Lipid Res. (2013) 54:1300–11. doi: 10.1194/jlr.M033977
163. Santinha D, Klopot A, Marques I, Ellis E, Jorns C, Johansson H, et al. Lipidomic analysis of human primary hepatocytes following LXR activation with GW3965 identifies AGXT2L1 as a main target associated to changes in phosphatidylethanolamine. J Steroid Biochem Mol Biol. (2020) 198:105558. doi: 10.1016/j.jsbmb.2019.105558
164. Shoucri BM, Martinez ES, Abreo TJ, Hung VT, Moosova Z, Shioda T, et al. Retinoid X receptor activation alters the chromatin landscape to commit mesenchymal stem cells to the adipose lineage. Endocrinology. (2017) 158:3109–25. doi: 10.1210/en.2017-00348
165. Zheng F, Zhang S, Lu W, Wu F, Yin X, Yu D, et al. Regulation of insulin resistance and adiponectin signaling in adipose tissue by liver X receptor activation highlights a cross-talk with PPARγ. PloS One. (2014) 9:e101269. doi: 10.1371/journal.pone.0101269
166. Mitro N, Vargas L, Romeo R, Koder A, Saez E. T0901317 is a potent PXR ligand: implications for the biology ascribed to LXR. FEBS Lett. (2007) 581:1721–6. doi: 10.1016/j.febslet.2007.03.047
167. Hamblin M, Chang L, Fan Y, Zhang J, Chen YE. PPARs and the cardiovascular system. Antioxid Redox Signal. (2009) 11:1415–52. doi: 10.1089/ars.2008.2280
168. Seimandi M, Lemaire G, Pillon A, Perrin A, Carlavan I, Voegel JJ, et al. Differential responses of PPARα, PPARδ, and PPARγ reporter cell lines to selective PPAR synthetic ligands. Analytical Biochem. (2005) 344:8–15. doi: 10.1016/j.ab.2005.06.010
169. McMullen PD, Bhattacharya S, Woods CG, Pendse SN, McBride MT, Soldatow VY, et al. Identifying qualitative differences in PPARα signaling networks in human and rat hepatocytes and their significance for next generation chemical risk assessment methods. Toxicol Vitro. (2020) 64:104463. doi: 10.1016/j.tiv.2019.02.017
170. Schierle S, Merk D. Therapeutic modulation of retinoid X receptors - SAR and therapeutic potential of RXR ligands and recent patents. Expert Opin Ther Pat. (2019) 29:605–21. doi: 10.1080/13543776.2019.1643322
171. Zhang F, Cao RL, Liu P, Chi TY, Ji XF, Zheng ZH, et al. The bexarotene derivative OAB-14 ameliorates cognitive decline in APP/PS1 transgenic mice by suppressing microglia-mediated neuroinflammation through the PPAR-γ pathway. Int Immunopharmacol. (2023) 124:110911. doi: 10.1016/j.intimp.2023.110911
172. Agarwal VR, Bischoff ED, Hermann T, Lamph WW. Induction of adipocyte-specific gene expression is correlated with mammary tumor regression by the retinoid X receptor-ligand LGD1069 (targretin). Cancer Res. (2000) 60:6033–8.
173. Hacioglu C, Kar F, Kanbak G. Ex vivo investigation of bexarotene and nicotinamide function as a protectıve agent on rat synaptosomes treated with Aβ(1–42). Neurochem Res. (2021) 46:804–18. doi: 10.1007/s11064-020-03216-7
174. Filer DL, Hoffman K, Sargis RM, Trasande L, Kassotis CD. On the utility of toxCast-based predictive models to evaluate potential metabolic disruption by environmental chemicals. Environ Health Perspect. (2022) 130:57005. doi: 10.1289/EHP6779
175. Feige JN, Gerber A, Casals-Casas C, Yang Q, Winkler C, Bedu E, et al. The pollutant diethylhexyl phthalate regulates hepatic energy metabolism via species-specific PPARalpha-dependent mechanisms. Environ Health Perspect. (2010) 118:234–41. doi: 10.1289/ehp.0901217
176. Radke EG, Braun JM, Meeker JD, Cooper GS. Phthalate exposure and male reproductive outcomes: A systematic review of the human epidemiological evidence. Environ Int. (2018) 121:764–93. doi: 10.1016/j.envint.2018.07.029
177. Zamora AN, Jansen EC, Tamayo-Ortiz M, Goodrich JM, Sánchez BN, Watkins DJ, et al. Exposure to phenols, phthalates, and parabens and development of metabolic syndrome among mexican women in midlife. Front Public Health. (2021) 9:620769. doi: 10.3389/fpubh.2021.620769
178. Desvergne B, Feige JN, Casals-Casas C. PPAR-mediated activity of phthalates: A link to the obesity epidemic? Mol Cell Endocrinol. (2009) 304:43–8. doi: 10.1016/j.mce.2009.02.017
179. Feige JN, Gelman L, Rossi D, Zoete V, Métivier R, Tudor C, et al. The endocrine disruptor monoethyl-hexyl-phthalate is a selective peroxisome proliferator-activated receptor gamma modulator that promotes adipogenesis. J Biol Chem. (2007) 282:19152–66. doi: 10.1074/jbc.M702724200
180. Zoete V, Grosdidier A, Michielin O. Peroxisome proliferator-activated receptor structures: ligand specificity, molecular switch and interactions with regulators. Biochim Biophys Acta. (2007) 1771:915–25. doi: 10.1016/j.bbalip.2007.01.007
181. Kratochvil I, Hofmann T, Rother S, Schlichting R, Moretti R, Scharnweber D, et al. Mono(2-ethylhexyl) phthalate (MEHP) and mono(2-ethyl-5-oxohexyl) phthalate (MEOHP) but not di(2-ethylhexyl) phthalate (DEHP) bind productively to the peroxisome proliferator-activated receptor γ. Rapid Commun Mass Spectrom. (2019) 33 Suppl 1:75–85. doi: 10.1002/rcm.8258
182. Yaghjyan L, Sites S, Ruan Y, Chang SH. Associations of urinary phthalates with body mass index, waist circumference and serum lipids among females: National Health and Nutrition Examination Survey 1999–2004. Int J Obes (Lond). (2015) 39:994–1000. doi: 10.1038/ijo.2015.8
183. Waits A, Chen HC, Kuo PL, Wang CW, Huang HB, Chang WH, et al. Urinary phthalate metabolites are associated with biomarkers of DNA damage and lipid peroxidation in pregnant women - Tainan Birth Cohort Study (TBCS). Environ Res. (2020) 188:109863. doi: 10.1016/j.envres.2020.109863
184. Trasande L, Attina TM, Sathyanarayana S, Spanier AJ, Blustein J. Race/ethnicity-specific associations of urinary phthalates with childhood body mass in a nationally representative sample. Environ Health Perspect. (2013) 121:501–6. doi: 10.1289/ehp.1205526
185. Sugeng EJ, Symeonides C, O'Hely M, Vuillermin P, Sly PD, Vijayasarathy S, et al. Predictors with regard to ingestion, inhalation and dermal absorption of estimated phthalate daily intakes in pregnant women: The Barwon infant study. Environ Int. (2020) 139:105700. doi: 10.1016/j.envint.2020.105700
186. Sol CM, Santos S, Duijts L, Asimakopoulos AG, Martinez-Moral MP, Kannan K, et al. Fetal exposure to phthalates and bisphenols and childhood general and organ fat. A population-based prospective cohort study. Int J Obes (Lond). (2020) 44:2225–35. doi: 10.1038/s41366-020-00672-7
187. Wassenaar PNH, Legler J. Systematic review and meta-analysis of early life exposure to di(2-ethylhexyl) phthalate and obesity related outcomes in rodents. Chemosphere. (2017) 188:174–81. doi: 10.1016/j.chemosphere.2017.08.165
188. Zomer AW, van der Burg B, Jansen GA, Wanders RJ, Poll-The BT, van der Saag PT. Pristanic acid and phytanic acid: naturally occurring ligands for the nuclear receptor peroxisome proliferator-activated receptor alpha. J Lipid Res. (2000) 41:1801–7. doi: 10.1016/S0022-2275(20)31973-8
189. ECHA. Proposed restriction on undecafluorohexanoic acid (PFHxA), its salts and related substances (2020). Available online at: https://www.google.com/url?sa=t&rct=j&q=&esrc=s&source=web&cd=&ved=2ahUKEwiqh-b5_a-DAxV0UkEAHd0NDvwQFnoECA0QAQ&url=https%3A%2F%2Fwww.echa.europa.eu%2Fdocuments%2F10162%2F036bf592-cf6d-6063–8f30-b1f89ba4cf0f&usg=AOvVaw2UA0aBgnE_MU-inlF8F12T&opi=89978449.
190. Spratlen MJ, Perera FP, Lederman SA, Robinson M, Kannan K, Herbstman J, et al. The association between perfluoroalkyl substances and lipids in cord blood. J Clin Endocrinol Metab. (2020) 105:43–54. doi: 10.1210/clinem/dgz024
191. Watkins AM, Wood CR, Lin MT, Abbott BD. The effects of perfluorinated chemicals on adipocyte differentiation in vitro. Mol Cell Endocrinol. (2015) 400:90–101. doi: 10.1016/j.mce.2014.10.020
192. Koshy TT, Attina TM, Ghassabian A, Gilbert J, Burdine LK, Marmor M, et al. Serum perfluoroalkyl substances and cardiometabolic consequences in adolescents exposed to the World Trade Center disaster and a matched comparison group. Environ Int. (2017) 109:128–35. doi: 10.1016/j.envint.2017.08.003
193. Schluter A, Giralt M, Iglesias R, Villarroya F. Phytanic acid, but not pristanic acid, mediates the positive effects of phytol derivatives on brown adipocyte differentiation. FEBS Lett. (2002) 517:83–6. doi: 10.1016/S0014-5793(02)02583-8
194. Schlüter A, Yubero P, Iglesias R, Giralt M, Villarroya F. The chlorophyll-derived metabolite phytanic acid induces white adipocyte differentiation. Int J Obes Relat Metab Disord. (2002) 26:1277–80. doi: 10.1038/sj.ijo.0802068
195. Hellgren LI. Phytanic acid–an overlooked bioactive fatty acid in dairy fat? Ann N Y Acad Sci. (2010) 1190:42–9. doi: 10.1111/j.1749-6632.2009.05254.x
196. García-Rojas P, Antaramian A, González-Dávalos L, Villarroya F, Shimada A, Varela-Echavarría A, et al. Induction of peroxisomal proliferator-activated receptor gamma and peroxisomal proliferator-activated receptor gamma coactivator 1 by unsaturated fatty acids, retinoic acid, and carotenoids in preadipocytes obtained from bovine white adipose tissue1,2. J Anim Sci. (2010) 88:1801–8. doi: 10.2527/jas.2009-2579
197. Werner LB, Hellgren LI, Raff M, Jensen SK, Petersen RA, Drachmann T, et al. Effect of dairy fat on plasma phytanic acid in healthy volunteers - a randomized controlled study. Lipids Health Disease. (2011) 10:95. doi: 10.1186/1476-511X-10-95
198. Kitareewan S, Burka LT, Tomer KB, Parker CE, Deterding LJ, Stevens RD, et al. Phytol metabolites are circulating dietary factors that activate the nuclear receptor RXR. Mol Biol Cell. (1996) 7:1153–66. doi: 10.1091/mbc.7.8.1153
199. Cronet P, Petersen JF, Folmer R, Blomberg N, Sjöblom K, Karlsson U, et al. Structure of the PPARalpha and -gamma ligand binding domain in complex with AZ 242; ligand selectivity and agonist activation in the PPAR family. Structure. (2001) 9:699–706. doi: 10.1016/S0969-2126(01)00634-7
200. Kalliora C, Kyriazis ID, Oka SI, Lieu MJ, Yue Y, Area-Gomez E, et al. Dual peroxisome-proliferator-activated-receptor-α/γ activation inhibits SIRT1-PGC1α axis and causes cardiac dysfunction. JCI Insight. (2019) 5. doi: 10.1172/jci.insight.129556
201. Chang RC, Joloya EM, Li Z, Shoucri BM, Shioda T, Blumberg B. miR-223 plays a key role in obesogen-enhanced adipogenesis in mesenchymal stem cells and in transgenerational obesity. Endocrinology. (2023) 164. doi: 10.1210/endocr/bqad027
202. Grün F, Watanabe H, Zamanian Z, Maeda L, Arima K, Cubacha R, et al. Endocrine-disrupting organotin compounds are potent inducers of adipogenesis in vertebrates. Mol Endocrinol. (2006) 20:2141–55. doi: 10.1210/me.2005-0367
203. Kanayama T, Kobayashi N, Mamiya S, Nakanishi T, Nishikawa J. Organotin compounds promote adipocyte differentiation as agonists of the peroxisome proliferator-activated receptor gamma/retinoid X receptor pathway. Mol Pharmacol. (2005) 67:766–74. doi: 10.1124/mol.104.008409
204. Bertuloso BD, Podratz PL, Merlo E, de Araújo JF, Lima LC, de Miguel EC, et al. Tributyltin chloride leads to adiposity and impairs metabolic functions in the rat liver and pancreas. Toxicol Lett. (2015) 235:45–59. doi: 10.1016/j.toxlet.2015.03.009
205. Ceotto Freitas-Lima L, Merlo E, Campos Zicker M, Navia-Pelaez JM, de Oliveira M, Dos Santos Aggum Capettini L, et al. Tributyltin impacts in metabolic syndrome development through disruption of angiotensin II receptor signaling pathways in white adipose tissue from adult female rats. Toxicol Lett. (2018) 299:21–31. doi: 10.1016/j.toxlet.2018.08.018
206. Grün F, Blumberg B. Environmental obesogens: organotins and endocrine disruption via nuclear receptor signaling. Endocrinology. (2006) 147:S50–5. doi: 10.1210/en.2005-1129
207. Grün F. Chapter Eleven - The Obesogen Tributyltin. In: Litwack G, editor. Vitamins & Hormones, vol. 94 . Academic Press, California (2014). p. 277–325.
208. Pereira-Fernandes A, Vanparys C, Vergauwen L, Knapen D, Jorens PG, Blust R. Toxicogenomics in the 3T3-L1 cell line, a new approach for screening of obesogenic compounds. Toxicol Sci. (2014) 140:352–63. doi: 10.1093/toxsci/kfu092
209. Farinetti A, Marraudino M, Ponti G, Panzica G, Gotti S. Chronic treatment with tributyltin induces sexually dimorphic alterations in the hypothalamic POMC system of adult mice. Cell Tissue Res. (2018) 374:587–94. doi: 10.1007/s00441-018-2896-9
210. Lan XR, Li YW, Chen QL, Shen YJ, Liu ZH. Tributyltin impaired spermatogenesis and reproductive behavior in male zebrafish. Aquat Toxicol. (2020) 224:105503. doi: 10.1016/j.aquatox.2020.105503
211. Ho JCH, Hsiao CD, Kawakami K, Tse WKF. Triclosan (TCS) exposure impairs lipid metabolism in zebrafish embryos. Aquat Toxicol. (2016) 173:29–35. doi: 10.1016/j.aquatox.2016.01.001
212. Regnault C, Usal M, Veyrenc S, Couturier K, Batandier C, Bulteau AL, et al. Unexpected metabolic disorders induced by endocrine disruptors in Xenopus tropicalis provide new lead for understanding amphibian decline. Proc Natl Acad Sci U S A. (2018) 115:E4416–e25. doi: 10.1073/pnas.1721267115
213. Husøy T, Andreassen M, Hjertholm H, Carlsen MH, Norberg N, Sprong C, et al. The Norwegian biomonitoring study from the EU project EuroMix: Levels of phenols and phthalates in 24-hour urine samples and exposure sources from food and personal care products. Environ Int. (2019) 132:105103. doi: 10.1016/j.envint.2019.105103
214. Nasab H, Rajabi S, Mirzaee M, Hashemi M. Association of urinary triclosan, methyl triclosan, triclocarban, and 2,4-dichlorophenol levels with anthropometric and demographic parameters in children and adolescents in 2020 (case study: Kerman, Iran). Environ Sci pollut Res Int. (2022) 29:30754–63. doi: 10.1007/s11356-021-18466-3
215. Liu J, Chen D, Huang Y, Bigambo FM, Chen T, Wang X. Effect of maternal triclosan exposure on neonatal birth weight and children triclosan exposure on children's BMI: A meta-analysis. Front Public Health. (2021) 9:648196. doi: 10.3389/fpubh.2021.648196
216. Kalloo G, Calafat AM, Chen A, Yolton K, Lanphear BP, Braun JM. Early life Triclosan exposure and child adiposity at 8 Years of age: a prospective cohort study. Environ Health. (2018) 17:24. doi: 10.1186/s12940-018-0366-1
217. Hu Y, Ding G, Lv C, Zhang Q, Zhang Y, Yuan T, et al. Association between triclosan exposure and obesity measures among 7-year-old children in northern China. Ecotoxicol Environ Saf. (2022) 239:113610. doi: 10.1016/j.ecoenv.2022.113610
218. Lankester J, Patel C, Cullen MR, Ley C, Parsonnet J. Urinary triclosan is associated with elevated body mass index in NHANES. PloS One. (2013) 8:e80057. doi: 10.1371/journal.pone.0080057
219. Alfhili MA, Lee MH. Triclosan: an update on biochemical and molecular mechanisms. Oxid Med Cell Longev. (2019) 2019:1607304. doi: 10.1155/2019/1607304
220. Wu Y, Wu Q, Beland FA, Ge P, Manjanatha MG, Fang JL. Differential effects of triclosan on the activation of mouse and human peroxisome proliferator-activated receptor alpha. Toxicol Lett. (2014) 231:17–28. doi: 10.1016/j.toxlet.2014.09.001
221. Yueh M-F, He F, Chen C, Vu C, Tripathi A, Knight R, et al. Triclosan leads to dysregulation of the metabolic regulator FGF21 exacerbating high fat diet-induced nonalcoholic fatty liver disease. Proc Natl Acad Sci. (2020) 117:31259–66. doi: 10.1073/pnas.2017129117
222. SCCP. Scientific Committee on Consumer Products, Opinion on Triclosan, SCCP/1192/08 (2009). Available online at: https://ec.europa.eu/health/ph_risk/committees/04_sccp/docs/sccp_o_166.pdf.
223. Cano-Sancho G, Smith A, La Merrill MA. Triphenyl phosphate enhances adipogenic differentiation, glucose uptake and lipolysis via endocrine and noradrenergic mechanisms. Toxicol In Vitro. (2017) 40:280–8. doi: 10.1016/j.tiv.2017.01.021
224. Li X, Hansen PA, Xi L, Chandraratna RA, Burant CF. Distinct mechanisms of glucose lowering by specific agonists for peroxisomal proliferator activated receptor gamma and retinoic acid X receptors. J Biol Chem. (2005) 280:38317–27. doi: 10.1074/jbc.M505853200
225. Chen R, Wan J, Song J, Qian Y, Liu Y, Gu S. Rational screening of peroxisome proliferator-activated receptor-γ agonists from natural products: potential therapeutics for heart failure. Pharm Biol. (2017) 55:503–9. doi: 10.1080/13880209.2016.1255648
226. Ren XM, Chang RC, Huang Y, Amorim Amato A, Carivenc C, Grimaldi M, et al. 2,4-di-tert-butylphenol induces adipogenesis in human mesenchymal stem cells by activating retinoid X receptors. Endocrinology. (2023) 164. doi: 10.1210/endocr/bqad021
227. Shoucri BM, Hung VT, Chamorro-García R, Shioda T, Blumberg B. Retinoid X receptor activation during adipogenesis of female mesenchymal stem cells programs a dysfunctional adipocyte. Endocrinology. (2018) 159:2863–83. doi: 10.1210/en.2018-00056
228. UNEP. United Nations Environment Programme, Stockholm Convention on Persistent Organic Pollutants (POPs) (2019). Available online at: https://chm.pops.int/Portals/0/download.aspx?d=UNEP-POPS-COP-CONVTEXT-2021.English.pdf.
229. Bjork JA, Wallace KB. Structure-activity relationships and human relevance for perfluoroalkyl acid-induced transcriptional activation of peroxisome proliferation in liver cell cultures. Toxicol Sci. (2009) 111:89–99. doi: 10.1093/toxsci/kfp093
230. Chang RC, Thangavelu CS, Joloya EM, Kuo A, Li Z, Blumberg B. Cannabidiol promotes adipogenesis of human and mouse mesenchymal stem cells via PPARγ by inducing lipogenesis but not lipolysis. Biochem Pharmacol. (2022) 197:114910. doi: 10.1016/j.bcp.2022.114910
231. O'Sullivan SE, Kendall DA. Cannabinoid activation of peroxisome proliferator-activated receptors: potential for modulation of inflammatory disease. Immunobiology. (2010) 215:611–6. doi: 10.1016/j.imbio.2009.09.007
232. Sun Y, Bennett A. Cannabinoids: a new group of agonists of PPARs. PPAR Res. (2007) 2007:23513. doi: 10.1155/2007/23513
233. Takeda S, Ikeda E, Su S, Harada M, Okazaki H, Yoshioka Y, et al. Δ(9)-THC modulation of fatty acid 2-hydroxylase (FA2H) gene expression: possible involvement of induced levels of PPARα in MDA-MB-231 breast cancer cells. Toxicology. (2014) 326:18–24. doi: 10.1016/j.tox.2014.09.011
234. Kassotis CD, Stapleton HM. Endocrine-mediated mechanisms of metabolic disruption and new approaches to examine the public health threat. Front Endocrinol (Lausanne). (2019) 10:39. doi: 10.3389/fendo.2019.00039
235. Baell JB, Holloway GA. New substructure filters for removal of pan assay interference compounds (PAINS) from screening libraries and for their exclusion in bioassays. J Med Chem. (2010) 53:2719–40. doi: 10.1021/jm901137j
236. Jacobs MN, Laws SC, Willett K, Schmieder P, Odum J, Bovee TF. In vitro metabolism and bioavailability tests for endocrine active substances: what is needed next for regulatory purposes? Altex. (2013) 30:331–51. doi: 10.14573/altex
237. OECD. Detailed Review Paper on the Use of Metabolising Systems for in Vitro Testing of Endocrine Disruptors. Paris: OECD Publishing (2008). Available at: https://one.oecd.org/document/env/jm/mono(2008)24/en/pdf.
238. Louekari K, Jacobs MN. A modular strategy for the testing and assessment of non-genotoxic carcinogens. Arch Toxicol. (2024) Online ahead of print. doi: 10.1007/s00204-024-03753-y
239. Jacobs MN, Dickins M, Lewis DF. Homology modelling of the nuclear receptors: human oestrogen receptorbeta (hERbeta), the human pregnane-X-receptor (PXR), the Ah receptor (AhR) and the constitutive androstane receptor (CAR) ligand binding domains from the human oestrogen receptor alpha (hERalpha) crystal structure, and the human peroxisome proliferator activated receptor alpha (PPARalpha) ligand binding domain from the human PPARgamma crystal structure. J Steroid Biochem Mol Biol. (2003) 84:117–32. doi: 10.1016/S0960-0760(03)00021-9
240. Lewis DF. Quantitative structure-activity relationships (QSARs) within the cytochrome P450 system: QSARs describing substrate binding, inhibition and induction of P450s. Inflammopharmacology. (2003) 11:43–73. doi: 10.1163/156856003321547112
241. Lewis DF, Jacobs MN, Dickins M, Lake BG. Quantitative structure–activity relationships for inducers of cytochromes P450 and nuclear receptor ligands involved in P450 regulation within the CYP1, CYP2, CYP3 and CYP4 families. Toxicology. (2002) 176:51–7. doi: 10.1016/S0300-483X(02)00135-X
242. Lill MA, Dobler M, Vedani A. In silico prediction of receptor-mediated environmental toxic phenomena-application to endocrine disruption. SAR QSAR Environ Res. (2005) 16:149–69. doi: 10.1080/10629360412331319826
243. Schneider M, Pons JL, Bourguet W, Labesse G. Towards accurate high-throughput ligand affinity prediction by exploiting structural ensembles, docking metrics and ligand similarity. Bioinformatics. (2020) 36:160–8. doi: 10.1093/bioinformatics/btz538
244. Bennett CN, Ross SE, Longo KA, Bajnok L, Hemati N, Johnson KW, et al. Regulation of Wnt signaling during adipogenesis. J Biol Chem. (2002) 277:30998–1004. doi: 10.1074/jbc.M204527200
245. de Winter TJJ, Nusse R. Running against the wnt: how wnt/β-catenin suppresses adipogenesis. Front Cell Dev Biol. (2021) 9. doi: 10.3389/fcell.2021.627429
246. Prestwich TC, Macdougald OA. Wnt/beta-catenin signaling in adipogenesis and metabolism. Curr Opin Cell Biol. (2007) 19:612–7. doi: 10.1016/j.ceb.2007.09.014
247. Xu C, Wang J, Zhu T, Shen Y, Tang X, Fang L, et al. Cross-talking between PPAR and WNT signaling and its regulation in mesenchymal stem cell differentiation. Curr Stem Cell Res Ther. (2016) 11:247–54. doi: 10.2174/1574888X10666150723145707
248. Feng S, Reuss L, Wang Y. Potential of Natural Products in the Inhibition of Adipogenesis through Regulation of PPARγ Expression and/or Its Transcriptional Activity. Molecules. (2016) 21. doi: 10.3390/molecules21101278
249. Al Tanoury Z, Piskunov A, Rochette-Egly C. Vitamin A and retinoid signaling: genomic and nongenomic effects. J Lipid Res. (2013) 54:1761–75. doi: 10.1194/jlr.R030833
250. Schug TT, Berry DC, Shaw NS, Travis SN, Noy N. Opposing effects of retinoic acid on cell growth result from alternate activation of two different nuclear receptors. Cell. (2007) 129:723–33. doi: 10.1016/j.cell.2007.02.050
251. Heim M, Johnson J, Boess F, Bendik I, Weber P, Hunziker W, et al. Phytanic acid, a natural peroxisome proliferator-activated receptor (PPAR) agonist, regulates glucose metabolism in rat primary hepatocytes. FASEB J. (2002) 16:718–20. doi: 10.1096/fj.01-0816fje
252. Wang H, Mao X, Du M. Phytanic acid activates PPARα to promote beige adipogenic differentiation of preadipocytes. J Nutr Biochem. (2019) 67:201–11. doi: 10.1016/j.jnutbio.2019.02.013
253. Stossi F, Dandekar RD, Johnson H, Lavere P, Foulds CE, Mancini MG, et al. Tributyltin chloride (TBT) induces RXRA down-regulation and lipid accumulation in human liver cells. PloS One. (2019) 14:e0224405. doi: 10.1371/journal.pone.0224405
254. Haussler MR, Haussler CA, Jurutka PW. Genomically anchored vitamin D receptor mediates an abundance of bioprotective actions elicited by its 1,25-dihydroxyvitamin D hormonal ligand. Vitam Horm. (2023) 123:313–83. doi: 10.1016/bs.vh.2022.12.008
255. Bentley P, Calder I, Elcombe C, Grasso P, Stringer D, Wiegand HJ. Hepatic peroxisome proliferation in rodents and its significance for humans. Food Chem Toxicol. (1993) 31:857–907. doi: 10.1016/0278-6915(93)90225-N
256. IARC. Peroxisome proliferation and its role in Carcinogenesis, IARC Technical Publication No. 24 IARC Press (1995). Available online at: https://publications.iarc.fr/_publications/media/download/3480/c38962057a869b362b367a431e0c451e1e1272c3.pdf.
257. van Kesteren P, Pronk M, Heusinkveld H, Luijten M, Hakkert B. titled "Critical evaluation of the human relevance of the mode of action for rodent liver tumor formation by activators of the constitutive androstane receptor (CAR)". Crit Rev Toxicol. (2022) 52:397–8. Letter to the editor regarding the review article by Yamada et al. doi: 10.1080/10408444.2022.2115875
258. Strupp C, Corvaro M, Cohen SM, Corton JC, Ogawa K, Richert L, et al. Increased cell proliferation as a key event in chemical carcinogenesis: application in an integrated approach for the testing and assessment of non-genotoxic carcinogenesis. Int J Mol Sci. (2023) 24. doi: 10.3390/ijms241713246
259. Grimaldi M, Boulahtouf A, Delfosse V, Thouennon E, Bourguet W, Balaguer P. Reporter cell lines for the characterization of the interactions between human nuclear receptors and endocrine disruptors. Front Endocrinol (Lausanne). (2015) 6:62. doi: 10.3389/fendo.2015.00062
260. Egusa G, Ohno H, Nagano G, Sagawa J, Shinjo H, Yamamoto Y, et al. Selective activation of PPARα maintains thermogenic capacity of beige adipocytes. iScience. (2023) 26:107143. doi: 10.1016/j.isci.2023.107143
261. Goto T, Lee JY, Teraminami A, Kim YI, Hirai S, Uemura T, et al. Activation of peroxisome proliferator-activated receptor-alpha stimulates both differentiation and fatty acid oxidation in adipocytes. J Lipid Res. (2011) 52:873–84. doi: 10.1194/jlr.M011320
262. Corrales P, Vidal-Puig A, Medina-Gómez G. PPARs and metabolic disorders associated with challenged adipose tissue plasticity. Int J Mol Sci. (2018) 19. doi: 10.3390/ijms19072124
263. Abdou HS, Atlas E, Haché RJ. A positive regulatory domain in CCAAT/enhancer binding protein β (C/EBPΒ) is required for the glucocorticoid-mediated displacement of histone deacetylase 1 (HDAC1) from the C/ebpα promoter and maximum adipogenesis. Endocrinology. (2013) 154:1454–64. doi: 10.1074/jbc.M110.211540
264. Abdou HS, Atlas E, Haché RJ. Liver-enriched inhibitory protein (LIP) actively inhibits preadipocyte differentiation through histone deacetylase 1 (HDAC1). J Biol Chem. (2011) 286:21488–99. doi: 10.1074/jbc.M110.211540
265. Tomlinson JJ, Boudreau A, Wu D, Abdou Salem H, Carrigan A, Gagnon A, et al. Insulin sensitization of human preadipocytes through glucocorticoid hormone induction of forkhead transcription factors. Mol Endocrinol. (2010) 24:104–13. doi: 10.1210/me.2009-0091
266. Bapat SP, Whitty C, Mowery CT, Liang Y, Yoo A, Jiang Z, et al. Obesity alters pathology and treatment response in inflammatory disease. Nature. (2022) 604:337–42. doi: 10.1038/s41586-022-04536-0
Keywords: adipogenesis, obesogen, peroxisome proliferator-activated receptor, metabolic disruption, integrated testing strategy, test guideline, validation
Citation: Ozcagli E, Kubickova B and Jacobs MN (2024) Addressing chemically-induced obesogenic metabolic disruption: selection of chemicals for in vitro human PPARα, PPARγ transactivation, and adipogenesis test methods. Front. Endocrinol. 15:1401120. doi: 10.3389/fendo.2024.1401120
Received: 14 March 2024; Accepted: 10 June 2024;
Published: 08 July 2024.
Edited by:
Anna-Maria Andersson, Department of Growth and Reproduction, Rigshospitalet, DenmarkReviewed by:
Chad Deisenroth, United States Environmental Protection Agency (EPA), United StatesCopyright © 2024 Ozcagli, Kubickova and Jacobs. This is an open-access article distributed under the terms of the Creative Commons Attribution License (CC BY). The use, distribution or reproduction in other forums is permitted, provided the original author(s) and the copyright owner(s) are credited and that the original publication in this journal is cited, in accordance with accepted academic practice. No use, distribution or reproduction is permitted which does not comply with these terms.
*Correspondence: Miriam N. Jacobs, TWlyaWFtLkphY29ic0B1a2hzYS5nb3YudWs=
†ORCID: Eren Ozcagli, orcid.org/0000-0003-3472-1693
Barbara Kubickova, orcid.org/0000-0002-5044-7180
Miriam N. Jacobs, orcid.org/0000-0002-4858-0118
Disclaimer: All claims expressed in this article are solely those of the authors and do not necessarily represent those of their affiliated organizations, or those of the publisher, the editors and the reviewers. Any product that may be evaluated in this article or claim that may be made by its manufacturer is not guaranteed or endorsed by the publisher.
Research integrity at Frontiers
Learn more about the work of our research integrity team to safeguard the quality of each article we publish.