- Department of Molecular, Cellular and Developmental Biology, University of Michigan, Ann Arbor, MI, United States
Stanniocalcin 1 (Stc1) is well known for its role in regulating calcium uptake in fish by acting on ionocytes or NaR cells. A hallmark of NaR cells is the expression of Trpv6, a constitutively open calcium channel. Recent studies in zebrafish suggest that genetical deletion of Stc1a and Trpv6 individually both increases IGF signaling and NaR cell proliferation. While trpv6-/- fish suffered from calcium deficiency and died prematurely, stc1a-/- fish had elevated body calcium levels but also died prematurely. The relationship between Stc1a, Trpv6, and IGF signaling in regulating calcium homeostasis and organismal survival is unclear. Here we report that loss of Stc1a increases Trpv6 expression in NaR cells in an IGF signaling-dependent manner. Treatment with CdCl2, a Trpv6 inhibitor, reduced NaR cell number in stc1a-/- fish to the sibling levels. Genetic and biochemical analysis results suggest that Stc1a and Trpv6 regulate NaR cell proliferation via the same IGF pathway. Alizarin red staining detected abnormal calcium deposits in the yolk sac region and kidney stone-like structures in stc1a-/- fish. Double knockout or pharmacological inhibition of Trpv6 alleviated these phenotypes, suggesting that Stc1a inhibit epithelial Ca2+ uptake by regulating Trpv6 expression and activity. stc1a-/- mutant fish developed cardiac edema, body swelling, and died prematurely. Treatment of stc1a-/- fish with CdCl2 or double knockout of Trpv6 alleviated these phenotypes. These results provide evidence that Stc1a regulates calcium homeostasis and organismal survival by suppressing Trpv6 expression and inhibiting IGF signaling in ionocytes.
Introduction
Stanniocalcins (Stcs) are evolutionarily conserved glycoproteins. The first Stc protein was discovered from the Corpuscles of Stannius (CS), an endocrine organ unique to bony fish (1, 2). Surgical removal of CS resulted in elevated blood calcium levels and the appearance of kidney stones (3–5). Injection of CS extracts or purified Stc1 reversed these effects (6). In cultured rainbow trout CSs, secretion of Stc1 was found to be regulated by external Ca2+ levels ([Ca2+]) (6). High [Ca2+] increased Stc1 secretion via the calcium sensing receptor (7). In vivo, zebrafish embryos raised in high [Ca2+] media showed elevated stc1 mRNA levels (8, 9). Morpholino-based knockdown of Stc1a increased Ca2+ uptake and forced expression of Stc1a decreased Ca2+ uptake (10, 11). These and other findings have led to the notion that Stc1 is a hypocalcemic hormone in fish (2, 4, 6).
For several decades, Stc1 was considered a fish-specific hormone and even once called teleocalcin (2). Recent advances in genomics, however, have revealed that two STC genes are present in humans and other mammals. Human STC1 shares 61% sequence identity with fish Stc1 (12). In addition to STC1, there is a related protein (STC2), which shares ~30% identity in amino acid sequence with STC1 and contains a histidine cluster in the C-terminal region (2). Subsequent studies show that many teleost fish including zebrafish have 4 distinct stc genes, including stc1a, stc1b, stc2a, and stc2b (13), consistent with the notion that many teleost fish genomes underwent an additional round of genome-wide duplication (14). Published results suggest that mammalian STCs regulate somatic growth by inhibiting the insulin-like growth factor (IGF) signaling locally (15–17). IGFs act by binding to the IGF1 receptor and activating the downstream signaling cascades, including the PI3K-AKT-mTOR pathway and the RAS/RAF-MAP kinase pathway (18). In extracellular environments, IGFs are found in complexes with six types of IGF binding proteins (IGFBPs). These IGFBPs bind to IGF with an equal or greater affinity than the IGF1 receptor and therefore regulates IGF availability and biological activity (19). An important regulatory mechanism of the IGF signaling is proteolytic degradation of IGFBPs (16). Two structurally related metalloproteinases, pregnancy-associated plasma protein-a (PAPP-A) and PAPP-A2, have been shown to cleave IGFBPs and release IGFs from the IGFBP-IGF complex for IGF1 receptor binding (20). In vivo and biochemical studies suggest that human STC1 and STC2 function as potent inhibitors of PAPP-A and PAPP-A2 (21–23).
Recent genetic studies in zebrafish suggest that Stc1a is essential for life (24). stc1a-/- zebrafish developed cardiac edema around 4-5 days post fertilization (dpf). This was followed by whole body swelling and premature death (24). In zebrafish, calcium uptake is mainly carried out by Na+/H+-ATPase-rich (NaR) cells, one of the five types of ionocytes (25). stc1a-/- mutant larvae had significantly more NaR cells due to elevated NaR cell proliferation (24). Mechanistic analysis results show that Stc1a suppresses local IGF signaling by inhibiting Papp-aa mediated degradation of IGF binding protein 5a (Igfbp5a) in NaR cells (24, 26). A loss of Stc1a liberates IGFs from the Igfbp5a/IGF complex and increases bioavailable IGFs for IGF1 receptor binding (24, 26, 27). Addition of fish IGF-1 in excess was sufficient to increase NaR cell proliferation (26, 28). These findings suggest that the Stc1a-Papp-aa-Igfbp5a-IGF axis regulates NaR cell number and density.
A hallmark of NaR cells is the expression of Trpv6 (previously known as epithelial calcium channel or ECaC) (10, 29). Trpv6 is a constitutively open channel and it mediates continuous Ca2+ influx and maintains high cytoplasmic [Ca2+] levels (29). We have previously shown that genetic deletion of trpv6 not only reduces calcium influx but also increases NaR cell proliferation (29). While trpv6-/- fish suffered from calcium deficiency and died prematurely, stc1a-/- fish had elevated body calcium levels but also died prematurely (24, 29). The relationship between Stc1a, Trpv6, and IGF signaling in regulating NaR cell proliferation and calcium uptake is unclear. In the current study, we provide evidence that both Stc1a and Trpv6 inhibits NaR cell proliferation by suppressing IGF signaling. Genetic deletion of Stc1a increases trpv6 mRNA levels and results in abnormal calcium deposits in the yolk sac and kidney stones. These phenotypes were rescued by inhibiting Trpv6 channel activity and by double knockout of Trpv6. Additional evidence suggests a crosstalk between Trpv6-mediated calcium signaling and IGF signaling in NaR cells and they work together to maintain calcium homeostasis and organismal survival.
Results and discussion
Stc1a is synthesized and secreted from CS (10) (Figure 1A). As previously reported, genetic deletion of Stc1a resulted in a significant increase in NaR cells (24) (Figures 1B, C). Whether this action of Stc1a is specific to NaR cells was not clear. In this study, we determined the number of H+-ATPase-rich (HR) cells and Na+/Cl_ cotransporter (NCC) cells, two other ionocyte types responsible for Na+ uptake and Cl- uptake (25). No significant difference was detected in either HR cells or NCC cells (Figures 1D–G) between stc1a-/- larvae and their siblings, suggesting the action of Stc1a is specific to NaR cells. This result is consistent with previous studies showing that Igfbp5a is specifically expressed in NaR cells, but not in other ionocyte types (30–32).
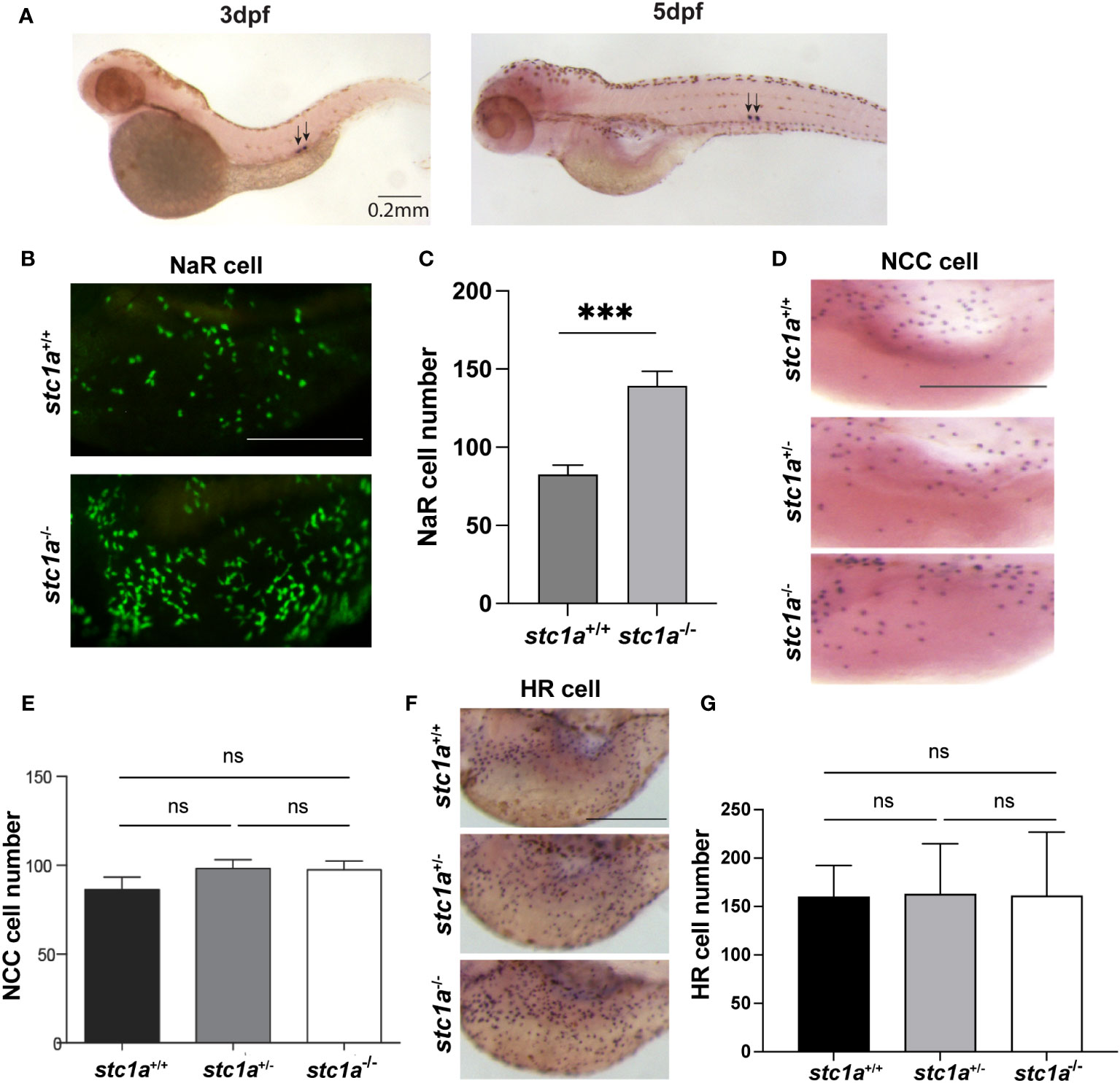
Figure 1 CS-derived Stc1a regulates the proliferation of NaR cells, but not other ionocyte types. (A) In situ hybridization analysis of stc1a mRNA expression in 3 and 5 days post fertilization (pdf) larvae. Arrows indicate the corpuscles of Stannius. (B, C) Loss of Stc1a increases NaR cell proliferation. stc1a+/+;Tg(igfbp5a:GFP), stc1a-/-;Tg(igfbp5a:GFP) embryos were raised in E3 embryo medium to 5 day post fertilization (dpf) and analyzed. Representative views are shown in (B). Scale bar = 0.2 mm. The NaR cell numbers were quantified and shown in (C). n = 16-19 larvae/group ***, P < 0.001. (D, E) NCC cells. Larvae (4 dpf) of the indicated genotypes were analyzed by in situ hybridization for slc12a10.2 mRNA expression. Representative views are shown in (D) and quantified data in (E). Scale bar = 0.2 mm. n = 4~13. ns, not statistically significant. (F, G) HR cells. Larvae (4 dpf) of the indicated genotypes were analyzed by in situ hybridization for atpv61al mRNA expression. Representative views are shown in (F) and quantified data in (G). Scale bar = 0.2 mm. n = 10~15 larvae/group. ns, not statistically significant. Images shown here and in all following figures are lateral views of the yolk sac region. Anterior to the left and dorsal up. Data shown are Mean ± SEM.
To test whether Trpv6 is involved in the increased NaR cell proliferation observed in stc1a-/- mutant fish, we measured trpv6 mRNA levels by qRT-PCR in Tg(igfbp5a:GFP) fish. In Tg(igfbp5a:GFP) fish, NaR cells are genetically labeled by GFP expression (32), allowing quantification of NaR cells in live larvae. Compared to the siblings, stc1a-/- fish had significantly greater levels of trpv6 mRNA (Figure 2A). To ascertain that the increased trpv6 mRNA levels are not a result of increased NaR cell number in stc1a-/- fish (24), GFP-positive NaR cells were quantified and used to normalize trpv6 mRNA levels. The trpv6 mRNA levels/NaR cell in stc1a-/- were also significantly greater than the siblings (Figure 2C). Our finding is consistent with in vitro studies reporting that si/shRNA-mediated knockdown of STC1 increases TRPV6 protein levels in human CaCo2, Hela, and Caski cells (33, 34). Next, we measured stc1a mRNA levels in trpv6-/- fish and siblings. As shown in Figure 2B, stc1a mRNA levels were significantly lower in trpv6-/- mutant fish, suggesting that Stc1a and Trpv6 are interconnected.
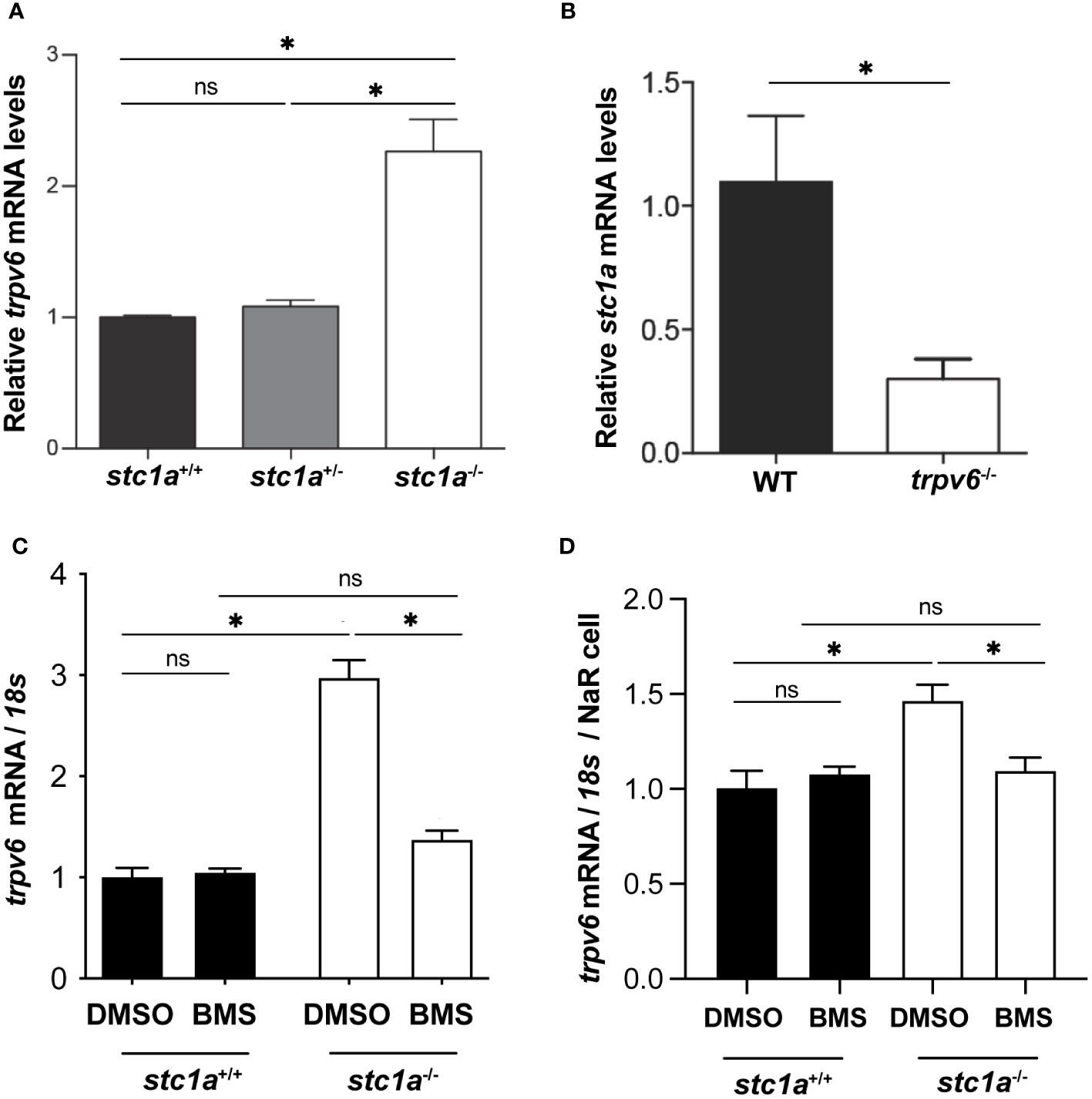
Figure 2 Stc1a and Trpv6 regulate each other’s expression. (A) Loss of Stc1a increases trpv6 mRNA levels. Fish of the indicated genotypes were raised in E3 embryo medium. At 5 dpf, the trpv6 mRNA levels were measured and normalized by 18S RNA levels. n = 15~17. *, P < 0.05. (B) Loss of Trpv6 reduces stc1a mRNA levels. Embryos of the indicated genotypes were raised in E3 embryo medium. At 5 dpf, the stc1a mRNA levels were measured and normalized by 18S RNA levels. n = 15~17. *, P < 0.05. (C, D) IGF signaling is critical in increasing Trpv6 expression in stc1a-/- fish. Larvae (4 pdf) of the indicated genotypes were treated with DMSO or BMS-754807 for one day and the trpv6 mRNA levels were measured and normalized by 18S rRNA (C). The data were further normalized by NaR cell numbers and shown in (D). Data shown are from 3 independent experiments, each containing 15 larvae/group. *, P < 0.05. ns, not statistically significant.
Previous studies have shown that loss of Stc1a increases IGF-Akt-Tor signaling in NaR cells (24). Does the elevated IGF-Akt-Tor signaling play any role in the increase of trpv6 mRNA expression in stc1a-/- fish? This idea was tested by treating stc1a-/- fish and siblings with BMS-754807, an IGF1-R inhibitor (31). As shown in Figures 2C, D, BMS-754807 reduced trpv6 mRNA levels to the sibling levels, suggesting that loss of Stc1a increases Trpv6 expression via an IGF signaling-dependent mechanism. Recently, we have discovered that serum- and glucocorticoid-regulated kinase 1 (Sgk1) acts downstream in the IGF-Akt-Tor signaling pathway in NaR cells (35, 36). Studies in culture mammalian cells suggest that SGK1 up-regulates the expression of several ion channels and transporters, including the epithelial Ca2+ channels TRPV5 and TRPV6 (37). SGK1 influences transcription factors such as NF-κB, p53, CREB, AP-1 and FOXO3a. Future studies are needed to clarify whether Sgk1 plays a role in regulating trpv6 expression.
The functional role of increased trpv6 expression was investigated using CdCl2, a Trpv6 inhibitor (29). CdCl2 treatment reduced NaR cell number in stc1a-/- fish to the sibling group levels (Figure 3A), indicating that Stc1a suppresses NaR cell proliferation by acting through Trpv6. If this were correct, then double deletion of Stc1a and Trpv6 should phenocopy each other. Indeed, compared to the siblings, the NaR cell number of trpv6-/-; Tg(igfbp5a:GFP) fish was significantly higher. Double deletion of Stc1a and Trvp6 did not cause any further increase (Figures 3B, C), indicating that Stc1a and Trpv6 act via the same pathway. Akt is a downstream effector of IGF signaling and has been used as a proxy of IGF signaling in NaR cells due to the lack of antibodies to detected phospho-IGF1 receptors (31). To determine whether IGF signaling is involved, we measured phosphorylated Akt levels. Few Phospho-Akt positive cells were detected in wild-type and heterozygous siblings (Figures 3D, E). In comparison, a robust increase in Phospho-Akt positive NaR cells was detected in trpv6-/- larvae (Figures 3D, E). The double stc1a-/-; trpv6-/- mutant fish had a similar level of increase in Akt signaling as trpv6-/- mutant fish (Figures 3D, E), suggesting that Stc1a and Trpv6 inhibit NaR cell proliferation via the same IGF signaling. It is worthy to point out the difference in the two approaches used to inhibit Trpv6 function/activity in this study. In Figure 3A, CdCl2 treatment was carried out in stc1a-/- fish. These fish have a functional Trpv6 and at elevated levels. In this setting, CdCl2 treatment inhibited Trpv6-mediated calcium influx and resulted in reduced NaR cell proliferation, supporting the conclusion that Stc1a acts via Trpv6 to suppress NaR cell proliferation. In comparison, the experiment shown in Figures 3B, C used trpv6-/- mutant larvae. In this genetic deletion model, there is no functional Trpv6 (29). Loss of Stc1a did not cause a further increase in NaR cell proliferation in the absence of a functional Trpv6. This result is in agreement with our conclusion.
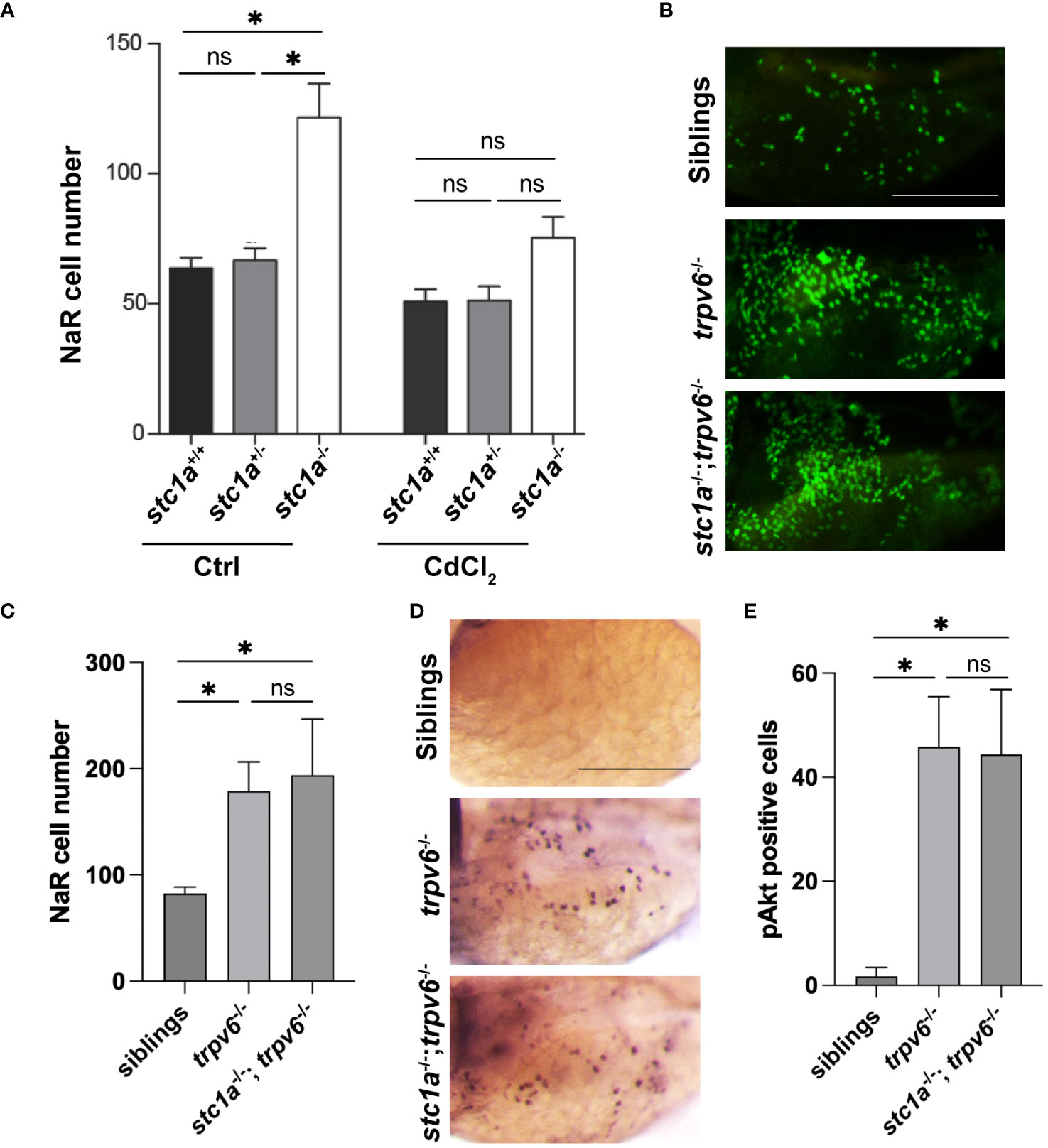
Figure 3 Stc1a and Trpv6 suppress NaR cell proliferation via the same IGF signaling pathway. (A) Inhibition of Trpv6 abolishes the elevated NaR cell proliferation in stc1a-/- larvae. Larvae (3 dpf) of the indicated genotypes were treated with DMSO or 10 μg/L CdCl2 for 2 days. GFP-labeled NaR cells were quantified and shown. n = 4~19 fish/group. *, P < 0.05. ns, not statistically significant. (B, C) stc1a-/-; trpv6-/- double mutants phenocopy trpv6-/- fish. Progeny of stc1a+/-; trpv6+/- in the Tg(igfbp5a:GFP) background were raised in E3 medium. At 5dpf, NaR cells were quantified and shown. These larvae were genotyped individually Representative images are shown in (B) and quantified data in (C). n = 4~19 larvae/group. Scale bar = 0.2 mm. (D, E) Progenies of stc1a+/-; trpv6+/- intercrosses were raised in E3 medium. They were subjected to whole mount immunohistochemistry using an anti-phospho-Akt antibody. Phospho-Akt positive cells in the yolk sac region were quantified. The larvae were genotyped individually afterwards. Representative images are shown in (D) and quantified data in (E). n = 5~14 larvae/group. Scale bar = 0.2 mm.
It has been documented half a century ago that removal of CS resulted in increased body calcium contents and the appearance of kidney stones (4). This has been attributed to the loss of Stc1. This notion, however, has not been tested genetically due to the lack of a stable genetic mutant. We visited this issue using the stc1a-/- mutant fish. Compared to their wild-type and heterozygous siblings, abnormal calcium deposits were observed in the yolk sac region where NaR cells are located (Figure 4A). Highly calcified stone-like structures were also observed in the renal tube (Figure 4A). In a previous report, we have quantified the calcium levels in stc1a-/- mutants and sibling embryos and found that stc1a-/- fish had significantly elevated calcium levels (24). Taken together, these data suggest that a permanent loss of Stc1a results in calcium imbalance and the development of kidney stones, essentially recapitulating the classical experiment results reported by Pang in the 1970s (4) using molecular genetics in zebrafish. Are these abnormal calcium deposits and kidney stones observed in stc1a-/- larvae related to the increased trpv6 gene expression (Figure 2)? To address this question, we treated the fish with CdCl2. CdCl2 markedly reduced the calcified structures in the yolk sac region and in the renal tubes (Figure 4A). This was investigated further using double mutant fish. Alizarin red staining showed that the abnormal calcified structures were not observed in the stc1a-/-; trpv6-/- double mutant fish. trpv6-/- fish had markedly reduced staining as well (Figure 4B). These results suggest that Stc1a inhibits epithelial Ca2+ uptake by regulating Trpv6 expression and activity.
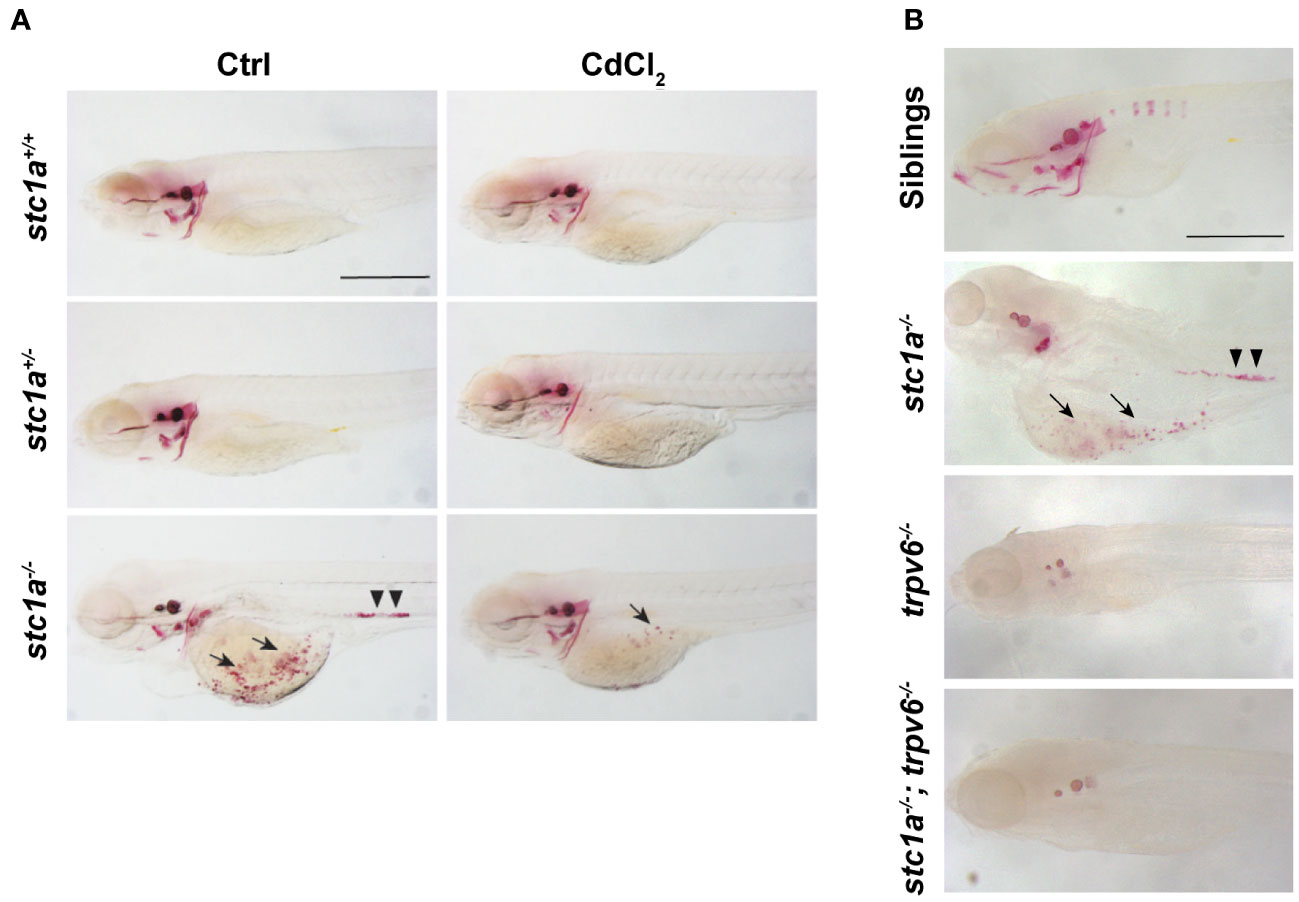
Figure 4 Loss of Stc1a results in abnormal calcium deposits in a Trpv6-depndent manner. (A) Larvae (3 dpf) of the indicated genotypes treated with or without 10 μg/L CdCl2 for 2 days. They were subjected to Alizarin red staining at 5 dpf. Representative images are shown. Note the ectopic calcified structures in the yolk sac region (arrow) and kidney stones (arrow heads) in the mutant fish. Scale bar = 0.5 mm. (B) Alizarin red staining analysis of 7 dpf zebrafish larvae of the indicated genotypes. Representative images are shown. Note the ectopic calcified structures in the yolk sac region (arrow) and kidney stones (arrow heads) in the mutant fish. Scale bar = 0.2 mm.
At 4-5 dpf, stc1a-/- mutants developed cardiac edema and this was followed by whole body swelling and premature death (24). In this study, we detected a significant reduction in heart rates (Figure 5A). These phenotypes are very different from the mouse model. Stc1-/- null mice grew normally with no notable anatomical abnormalities (38). These differences among species may relate to their distinct physiology and different habitats. Zebrafish Stc1a is expressed and secreted from CS glands in a calcium concentration-regulated manner (10, 24, 39). Mice, however, do not have CS glands and Stc1 gene is expressed in many tissues and likely acts locally as a PAPP-A/PAPP-A2 inhibitor (2, 17). Mouse Stc1 does not appear to affect calcium homeostasis because Stc1 knockout mice had normal circulating calcium levels and normal Vitamin D3 response (38). Mice and other territorial animals take up Ca2+ from food and drinks. Zebrafish live in freshwater, a hypoosmotic aquatic environment (40). Zebrafish actively regulate their body osmolarity by maintaining ion water balance. They use ionocytes to uptake salts. At the same time, zebrafish remove the excess osmotic water by producing and excreting large volumes of diluted urine and reabsorbing ions in the kidney (39, 40). Although zebrafish nephrons begin to form, efficient glomerular filtration and ion re-absorption begin around 4-5 dpf (39, 41, 42). The cardiac edema and body swelling phenotypes observed in stc1a-/- mutant fish begin to manifest around 4-5 dpf. These led us to speculate that elevated epithelial Ca2+ uptake and impaired renal function may result in the accumulation of osmotic water, which lead to the progressive development of edema and swelling. If this were correct, then pharmacological or genetic blockade of Trpv6-mediated Ca2+ uptake should rescue the stc1a mutant fish. Indeed, treatment of stc1a-/- fish with CdCl2 alleviated the edema and body swelling phenotype (Figure 5B). While stc1a-/- fish died between 6 to 10 dpf, there was no death in the CdCl2 treated group until 10 dpf (Figure 5C). The role of Trvp6-mediated epithelial Ca2+ uptake was tested further by double knocking out stc1a and trpv6. As shown in Figures 5D, E, no cardiac edema or body swelling was observed in stc1a-/-; trpv6-/- double mutant larvae. All stc1a mutant larvae lacked inflated swimming bladders (Figure 5D). This phenotype was rescued by CdCl2 treatment (Figure 5A) but not by double deletion of stc1a-/- and trpv6-/- (Figure 5C). The reason is not clear at this time. We have reported that the premature death can be rescued by reducing NaR cell number via pharmacological inhibition of the IGF1 receptor and Tor or by double deletion of igfbp5a or papp-aa in the stc1a-/- background (24). Since Stc1a and Trpv6 inhibit NaR cell number via the same IGF signaling, we tested the possible role of Trpv6 in zebrafish survival. While many stc1a-/- fish died between 7 to 10 dpf, no death was detected in stc1a-/-; trpv6-/- fish, trpv6-/- or siblings until 10 dpf (Figures 5D, E). These data suggest that the increased calcium uptake due to the combinatory effects of more NaR cells and great Trpv6 expression/NaR cell may cause ion water imbalance and premature death of stc1a-/- fish.
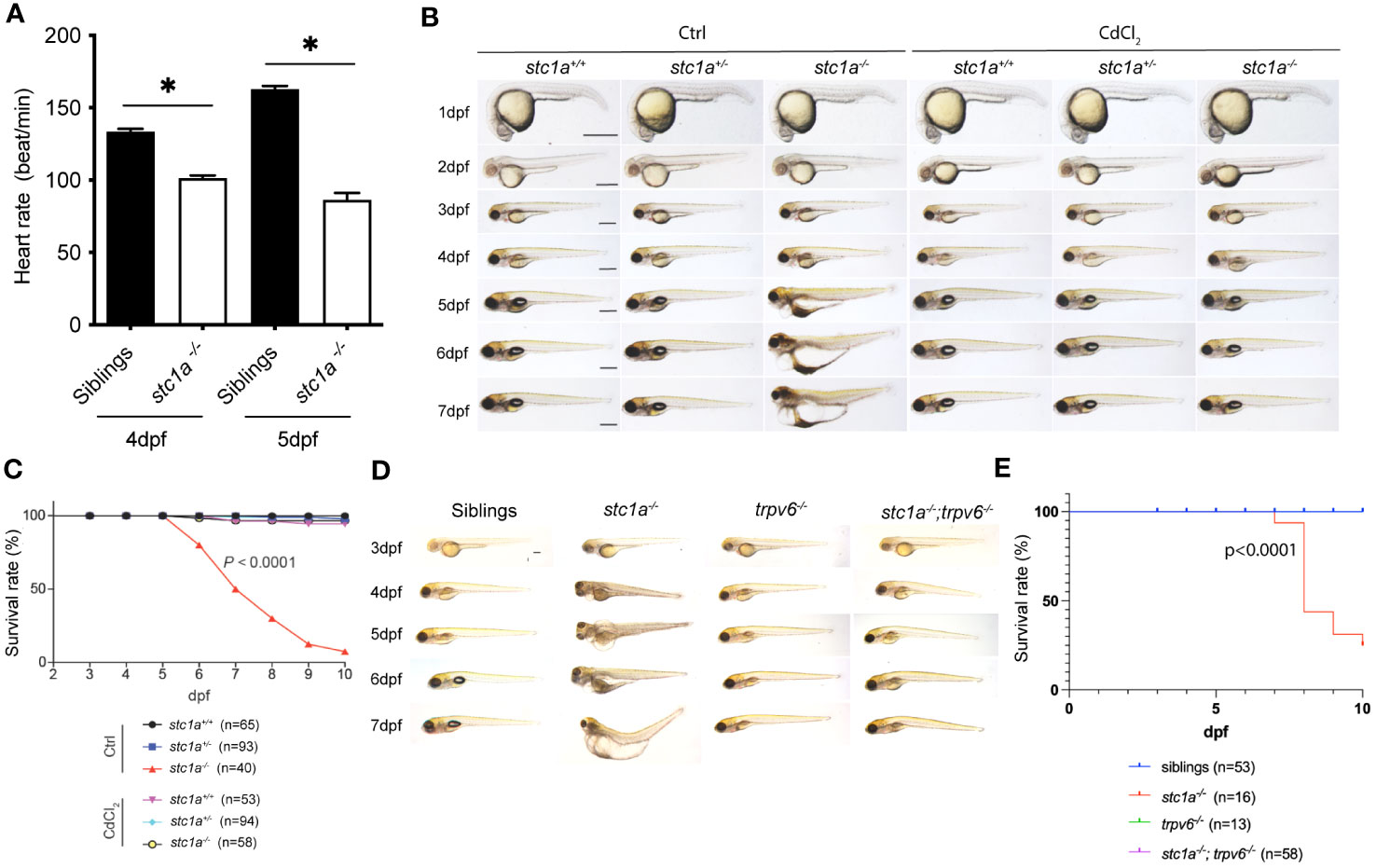
Figure 5 Pharmacological inhibition and double deletion of Trpv6 rescues cardiac edema and body swelling, and delays premature death of stc1a-/- fish. (A) Loss of Stc1a reduces heartbeat rate. Heartbeat rate stc1a-/- and siblings was determined and shown. *, P < 0.05. n = 9~17. (B) Gross morphology of fish of the indicated genotypes at the indicated time. Progeny of stc1a+/- intercrosses were raised in E3 embryo medium and treated with or without 10 μg/L CdCl2 from 3 dpf until the indicated time. Fish were genotyped individually. Representative views of the indicated genotypes at the indicated stages are shown and survival curve shown in (C). Scale bar = 0.5 mm. P < 0.0001 by log-rank test. (D, E) Gross morphology of fish of the indicated genotypes at the indicated time. Representative views at the indicated stages are shown and survival curve shown in (E). Scale bar = 0.2 mm. P < 0.0001 by log-rank test.
In summary, the results of this study have provided genetic and biochemical evidence that Stc1a regulates calcium homeostasis and organismal survival by playing dual roles in ionocytes (Figure 6). Stc1a suppresses NaR cell proliferation via its reported role in inhibiting Papp-aa-mediated local Igfbp5a degradation (24, 26). Stc1a also inhibits Trpv6 expression and/or Trpv6-mediated calcium uptake (Figure 6). These two functions are linked. While Trpv6-mediated calcium uptake inhibits IGF signaling, IGF signaling upregulates Trpv6 expression and stimulates NaR cell proliferation (Figure 6). A loss of Stc1a results in a reactivation of IGF-PI3 kinase-Akt-Tor signaling in NaR cells, which stimulates NaR cell proliferation and increase NaR cell number and calcium uptake. In addition, loss of Stc1a also increases Trpv6 expression and Trpv6-mediated calcium uptake. These changes contribute to abnormal calcium deposits in the yolk sac region and in the kidney, the development of edema, body swelling, and premature death (Figure 6). The current study also reveals a feedback loop from Trpv6 to Stc1a. While loss of Stc1a increases Trpv6 expression in NaR cells, loss of Trpv6 expression decreases Stc1a expression in CS. These findings provide new insights into our understanding of Stc1/STC1. At present, the biochemical pathways that lead to the formation of ectopic calcium deposits in the yolk sac region and in renal tubes found in the stc1a-/- mutant fish are not clear. In the adult stages, NaR cells are distributed mainly in the gills and kidney. Because stc1a-/- mutant fish die prematurely, the function of Stc1a in adult physiology is not clear. A conditional knockout fish model will be needed to elucidate Stc1a’s actions in the adult gills, kidney, and intestine. In addition to stc1a, zebrafish have 3 other stc genes. Future studies will be needed to elucidate their functions and the relationship among these genes.
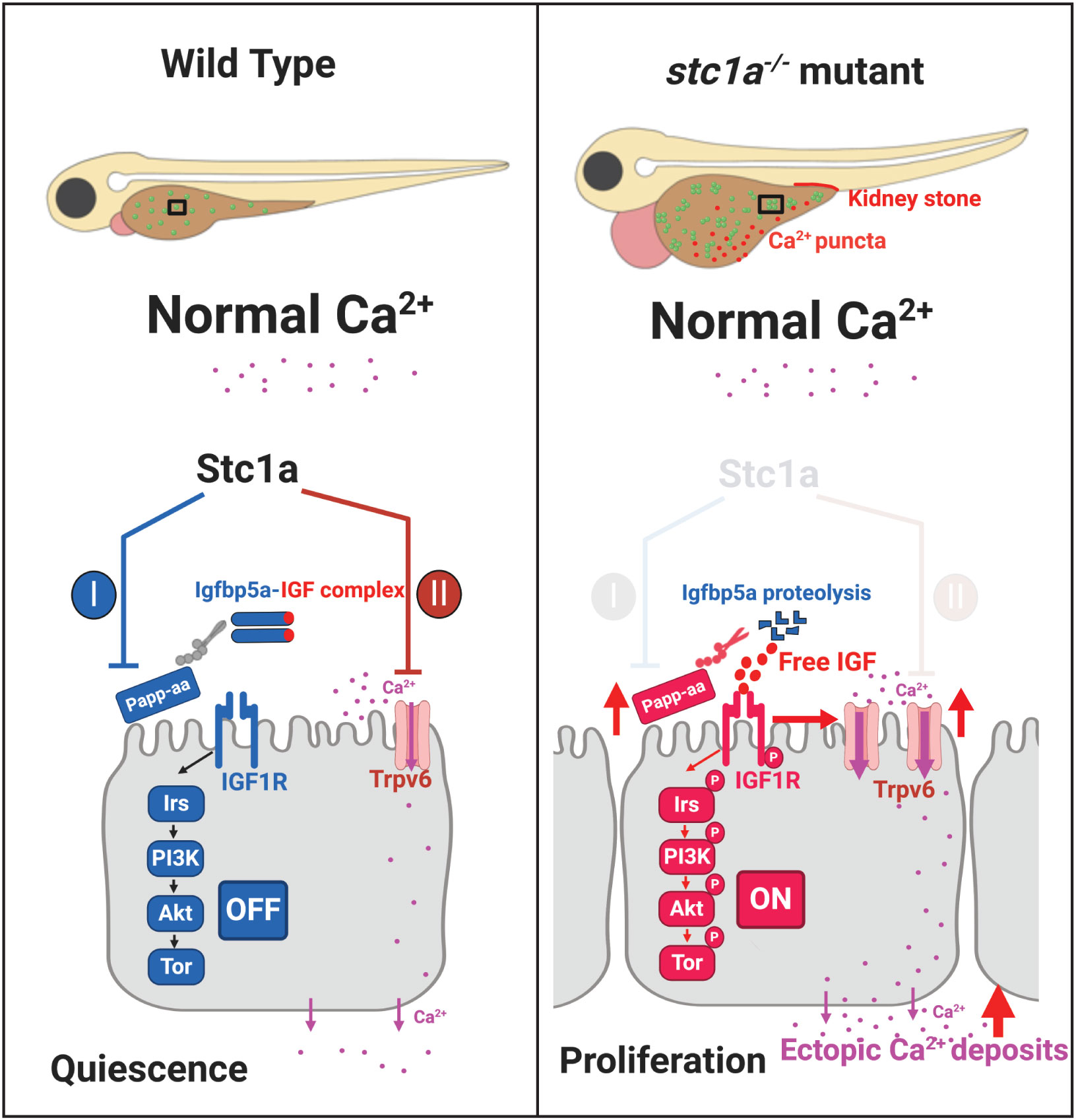
Figure 6 A proposed model. Stc1a plays dual roles in ionocytes. Stc1a suppresses local IGF signaling and inhibits NaR cell proliferation by inhibiting Papp-aa-mediated Igfbp5a degradation. Stc1a also inhibits Trpv6 expression and activities. These two functions are linked. Trpv6-mediated calcium signaling inhibits IGF signaling, while IGF signaling upregulates Trpv6 expression. A loss of Stc1a reactivates IGF-PI3 kinase-Akt-Tor signaling in NaR cells and increased NaR cell proliferation. In addition, Trpv6 expression and Trpv6-mediated calcium uptake in each NaR cell are elevated in the stc1a-/- mutant fish. These changes contribute to abnormal calcium deposits in the yolk sac region and kidney and to the developemnt of cardiac edema, body swelling, and premature death phenotypes.
Materials and methods
Zebrafish
The experiments were conducted in accordance with the guidelines approved by the Institutional Committee on the Use and Care of Animals, University of Michigan. Zebrafish were raised, maintained, crossed, and staged in accordance with the standard zebrafish husbandry guidelines (43). Embryos and larvae were raised at ~28°C in the standard E3 embryo medium. To inhibit pigmentation, 0.003% (w/v) N-phenylthiourea was added to these medium. The Tg(igfbp5a:GFP) fish line, Tg(ifbp5a:GFP);stc1a+/-, and Tg(igfbp5a:GFP);trpv6+/- fish line were generated in previous studies (24, 26, 27, 29). Double mutant fish were generated by crossing these lines.
Genotyping
Fish larvae or adult fish fin were digested in 100 μl SZL buffer (50 mM KCl, 2.5 mM MgCl2, 10 mM tris-HCl (pH 8.3), 0.45% NP-40, 0.45% Tween 20, 0.01% gelatine) and proteinase K (100 μg/ml) at 60°C for 2 hours. The reaction was stopped by 15-minute heat treatment (95°C). The genotyping was performed by PCR using the digestion mixture as a template as previously reported (24, 29).
Morphological analysis and heart rate
Heat rate was determined by counting heartbeat manually under a stereomicroscopy. For morphology imaging, embryos and larvae were briefly anesthetized with Tricaine and mounted in 1.5% agarose and imaged. Bright field images were acquired using a stereomicroscope (Leica MZ16F, Leica, Wetzlar, Germany) equipped with a QImaging QICAM camera (QImaging, Surrey, BC, Canada). After imaging, embryos and larvae were washed and returned to the E3 embryo medium.
Immunostaining, in situ hybridization, and Alizarin red staining
Immunostaining of phospho-Akt was performed as previously described (31, 44). Briefly, zebrafish larvae were fixed overnight in 4% paraformaldehyde. They were dehydrated in methanol for two hours at -20°C and washed with PBST (Triton 0.1%). After incubated with in PBST containing 5% horse serum for 1.5 hours at 4°C. The larvae were rinsed and incubated overnight with an antibody against phospho-Akt at 4°C. They were washed with PBST and 5% HS in PBST. The larvae were incubated with an anti-rabbit HRP antibody (Jackson ImmunoResearch, West Grove, PA, USA) for 3 hours at room temperature and visualized by nickel-diaminobenzidine staining. Whole mount in situ hybridization was performed as previously reported (30, 31, 45). Calcified tissues were detected by Alizarin red staining as reported previously (27).
qRT-PCR
Total RNA was extracted from pooled zebrafish embryos and larvae as reported (46). RNA was reverse transcribed to cDNA using oligo(dT)18 primer and M-MLV (Promega). qPCR was performed using SYBR Green (Bio-Rad) on a StepONEPLUS real-time thermocycler (Applied Biosystems). The expression level of a target gene transcript was normalized by 18S RNA level. The following primers were used: trpv6-qPCR-F: 5’- GGACCCTACGTCATTGTGATAC-3’, trpv6-qPCR-R: 5’-GGTACTGCGGAAGTGCTAAG-3’, 18s-qPCR-F: 5’-AATCGCATTTGCCATCACCG-3’, and 18s-qPCR-R: 5’-TCACCACCCTCTCAACCTCA-3’.
Drug treatment
All drugs were dissolved in DMSO and further diluted in double deionized water as previously reported (24, 31). Drug solutions were changed daily.
Statistical analysis
Statistical tests were determined using GraphPad Prism 8 software (GraphPad Software, Inc.,San Diego, CA). Values are shown as means ± SEM. Unpaired two-tailed t-test, Chi-square test, log-rank test and one-way ANOVA followed by Tukey’s multiple comparison test were used to determine statistical significance of experimental groups. A p-value less than 0.05 was accepted as statistically significant.
Data availability statement
The original contributions presented in the study are included in the article/supplementary material. Further inquiries can be directed to the corresponding author.
Ethics statement
The animal study was approved by Institutional Committee on the Use and Care of Animals, University of Michigan. The study was conducted in accordance with the local legislation and institutional requirements.
Author contributions
SL: Writing – review & editing, Data curation, Formal Analysis, Investigation, Visualization. HL: Data curation, Formal Analysis, Investigation, Visualization, Writing – review & editing. ZW: Data curation, Formal Analysis, Investigation, Visualization, Writing – review & editing, Validation. CD: Writing – review & editing, Conceptualization, Funding acquisition, Project administration, Resources, Supervision, Writing – original draft.
Funding
The author(s) declare financial support was received for the research, authorship, and/or publication of this article. This work was supported by NSF IOS-1755268 to CD. The funders had no role in study design, data collection and analysis, decision to publish, or preparation of the manuscript.
Acknowledgments
We thank Dr. Chenggong Liu, Dr. Yi Xin, and other Duan lab members for discussions and technical help.
Conflict of interest
The authors declare that the research was conducted in the absence of any commercial or financial relationships that could be construed as a potential conflict of interest.
The author(s) declared that they were an editorial board member of Frontiers, at the time of submission. This had no impact on the peer review process and the final decision.
Publisher’s note
All claims expressed in this article are solely those of the authors and do not necessarily represent those of their affiliated organizations, or those of the publisher, the editors and the reviewers. Any product that may be evaluated in this article, or claim that may be made by its manufacturer, is not guaranteed or endorsed by the publisher.
References
1. Pang PK, Pang RK, Sawyer WH. Effects of environmental calcium and replacement therapy on the killifish, Fundulus heteroclitus, after the surgical removal of the corpuscles of Stannius. Endocrinol (1973) 93(3):705–10. doi: 10.1210/endo-93-3-705
2. Yeung BH, Law AY, Wong CK. Evolution and roles of stanniocalcin. Mol Cell Endocrinol (2012) 349(2):272–80. doi: 10.1016/j.mce.2011.11.007
3. Fontaine M. [Stannius' Corpuscles and ionic (Ca, K, na) of the interior environment of the eel (Anguilla Anguilla L.)]. C R Hebd Seances Acad Sci (1964) 259:875–8.
4. Pang PK. The relationship between corpuscles of stannius and serum electrolyte regulation in killifish, Fundulus heteroclitus. J Exp Zool (1971) 178(1):1–8. doi: 10.1002/jez.1401780102
5. Fenwick JC, So YP. A perfusion study of the effect of stanniectomy on the net influx of calcium 45 across an isolated eel gill (1). J Exp Zool (1974) 188(1):125–31. doi: 10.1002/jez.1401880112
6. Ellis TJ, Wagner GF. Post-transcriptional regulation of the stanniocalcin gene by calcium. J Biol Chem (1995) 270(4):1960–5. doi: 10.1074/jbc.270.4.1960
7. Radman DP, McCudden C, James K, Nemeth EM, Wagner GF. Evidence for calcium-sensing receptor mediated stanniocalcin secretion in fish. Mol Cell Endocrinol (2002) 186(1):111–9. doi: 10.1016/S0303-7207(01)00643-8
8. Kwong RW, Auprix D, Perry SF. Involvement of the calcium-sensing receptor in calcium homeostasis in larval zebrafish exposed to low environmental calcium. Am J Physiol Regul Integr Comp Physiol (2014) 306(4):R211–21. doi: 10.1152/ajpregu.00350.2013
9. Lin CH, Su CH, Hwang PP. Calcium-sensing receptor mediates Ca(2+) homeostasis by modulating expression of PTH and stanniocalcin. Endocrinol (2014) 155(1):56–67. doi: 10.1210/en.2013-1608
10. Tseng DY, Chou MY, Tseng YC, Hsiao CD, Huang CJ, Kaneko T, et al. Effects of stanniocalcin 1 on calcium uptake in zebrafish (Danio rerio) embryo. Am J Physiol Regul Integr Comp Physiol (2009) 296(3):R549–57. doi: 10.1152/ajpregu.90742.2008
11. Chou MY, Lin CH, Chao PL, Hung JC, Cruz SA, Hwang PP. Stanniocalcin-1 controls ion regulation functions of ion-transporting epithelium other than calcium balance. Int J Biol Sci (2015) 11(2):122–32. doi: 10.7150/ijbs.10773
12. Chang AC, Dunham MA, Jeffrey KJ, Reddel RR. Molecular cloning and characterization of mouse stanniocalcin cDNA. Mol Cell Endocrinol (1996) 124(1-2):185–7. doi: 10.1016/S0303-7207(96)03929-9
13. Schein V, Cardoso JC, Pinto PI, Anjos L, Silva N, Power DM, et al. Four stanniocalcin genes in teleost fish: structure, phylogenetic analysis, tissue distribution and expression during hypercalcemic challenge. Gen Comp Endocrinol (2012) 175(2):344–56. doi: 10.1016/j.ygcen.2011.11.033
14. Taylor JS, Braasch I, Frickey T, Meyer A, Van de Peer Y. Genome duplication, a trait shared by 22000 species of ray-finned fish. Genome Res (2003) 13(3):382–90. doi: 10.1101/gr.640303
15. Marouli E, Graff M, Medina-Gomez C, Lo KS, Wood AR, Kjaer TR, et al. Rare and low-frequency coding variants alter human adult height. Nat (2017) 542(7640):186–90. doi: 10.1038/nature21039
16. Duan C, Allard JB. Insulin-like growth factor binding protein-5 in physiology and disease. Front Endocrinol (Lausanne) (2020) 11:100. doi: 10.3389/fendo.2020.00100
17. Oxvig C, Conover CA. The stanniocalcin-PAPP-A-IGFBP-IGF axis. J Clin Endocrinol Metab (2023) 4–6. doi: 10.1210/clinem/dgad053
18. Hakuno F, Takahashi SI. IGF1 receptor signaling pathways. J Mol Endocrinol (2018) 61(1):T69–86. doi: 10.1530/JME-17-0311
19. Allard JB, Duan C. IGF-binding proteins: why do they exist and why are there so many? Front Endocrinol (Lausanne) (2018) 9:117. doi: 10.3389/fendo.2018.00117
20. Oxvig C. The role of PAPP-A in the IGF system: location, location, location. J Cell Commun Signal (2015) 9(2):177–87. doi: 10.1007/s12079-015-0259-9
21. Kloverpris S, Mikkelsen JH, Pedersen JH, Jepsen MR, Laursen LS, Petersen SV, et al. Stanniocalcin-1 potently inhibits the proteolytic activity of the metalloproteinase pregnancy-associated plasma protein-A. J Biol Chem (2015) 290(36):21915–24. doi: 10.1074/jbc.M115.650143
22. Jepsen MR, Kloverpris S, Mikkelsen JH, Pedersen JH, Fuchtbauer EM, Laursen LS, et al. Stanniocalcin-2 inhibits mammalian growth by proteolytic inhibition of the insulin-like growth factor axis. J Biol Chem (2015) 290(6):3430–9. doi: 10.1074/jbc.M114.611665
23. Argente J, Chowen JA, Perez-Jurado LA, Frystyk J, Oxvig C. One level up: abnormal proteolytic regulation of IGF activity plays a role in human pathophysiology. EMBO Mol Med (2017) 9(10):1338–45. doi: 10.15252/emmm.201707950
24. Li S, Liu C, Goldstein A, Xin Y, Ke C, Duan C. Calcium state-dependent regulation of epithelial cell quiescence by stanniocalcin 1a. Front Cell Dev Biol (2021) 9:662915. doi: 10.3389/fcell.2021.662915
25. Hwang PP, Chou MY. Zebrafish as an animal model to study ion homeostasis. Pflugers Arch (2013) 465(9):1233–47. doi: 10.1007/s00424-013-1269-1
26. Liu C, Li S, Noer PR, Kjaer-Sorensen K, Juhl AK, Goldstein A, et al. The metalloproteinase Papp-aa controls epithelial cell quiescence-proliferation transition. Elife (2020) 9:1–20. doi: 10.7554/eLife.52322
27. Liu C, Xin Y, Bai Y, Lewin G, He G, Mai K, et al. Ca(2+) concentration-dependent premature death of igfbp5a(-/-) fish reveals a critical role of IGF signaling in adaptive epithelial growth. Sci Signal (2018) 11(548):1–10. doi: 10.1126/scisignal.aat2231
28. Moriyama S, Duguay SJ, Conlon JM, Duan C, Dickhoff WW, Plisetskaya EM. Recombinant coho salmon insulin-like growth factor I. Expression in Escherichia coli, purification and characterization. Eur J Biochem (1993) 218(1):205–11. doi: 10.1111/j.1432-1033.1993.tb18366.x
29. Xin Y, Malick A, Hu M, Liu C, Batah H, Xu H, et al. Cell-autonomous regulation of epithelial cell quiescence by calcium channel Trpv6. Elife (2019) 8:1–21. doi: 10.7554/eLife.48003
30. Dai W, Kamei H, Zhao Y, Ding J, Du Z, Duan C. Duplicated zebrafish insulin-like growth factor binding protein-5 genes with split functional domains: evidence for evolutionarily conserved IGF binding, nuclear localization, and transactivation activity. FASEB J (2010) 24(6):2020–9. doi: 10.1096/fj.09-149435
31. Dai W, Bai Y, Hebda L, Zhong X, Liu J, Kao J, et al. Calcium deficiency-induced and TRP channel-regulated IGF1R-PI3K-Akt signaling regulates abnormal epithelial cell proliferation. Cell Death Differ (2014) 21(4):568–81. doi: 10.1038/cdd.2013.177
32. Liu C, Dai W, Bai Y, Chi C, Xin Y, He G, et al. Development of a whole organism platform for phenotype-based analysis of IGF1R-PI3K-akt-tor action. Sci Rep (2017) 7(1):1994. doi: 10.1038/s41598-017-01687-3
33. Xiang J, Guo R, Wan C, Wu L, Yang S, Guo D. Regulation of intestinal epithelial calcium transport proteins by stanniocalcin-1 in caco2 cells. Int J Mol Sci (2016) 17(7). doi: 10.3390/ijms17071095
34. Liu JH, Cao YM, Rong ZP, Ding J, Pan X. Trichostatin A induces autophagy in cervical cancer cells by regulating the PRMT5-STC1-TRPV6-JNK pathway. Pharmacol (2021) 106(1-2):60–9. doi: 10.1159/000507937
35. Li Y, Liu C, Rolling L, Sikora V, Chen Z, Gurwin J, et al. ROS signaling-induced mitochondrial Sgk1 expression regulates epithelial cell renewal. Proc Natl Acad Sci U S A (2023) 120(24):e2216310120. doi: 10.1073/pnas.2216310120
36. Li Y, Liu C, Bai X, Li M, Duan C. FK506-binding protein 5 regulates cell quiescence-proliferation decision in zebrafish epithelium. FEBS Lett (2023) 597(14):1868–79. doi: 10.1002/1873-3468.14670
37. Lang F, Stournaras C, Zacharopoulou N, Voelkl J, Alesutan I. Serum- and glucocorticoid-inducible kinase 1 and the response to cell stress. Cell Stress (2018) 3(1):1–8. doi: 10.15698/cst2019.01.170
38. Chang AC, Cha J, Koentgen F, Reddel RR. The murine stanniocalcin 1 gene is not essential for growth and development. Mol Cell Biol (2005) 25(23):10604–10. doi: 10.1128/MCB.25.23.10604-10610.2005
39. Shu Y, Lou Q, Dai Z, Dai X, He J, Hu W, et al. The basal function of teleost prolactin as a key regulator on ion uptake identified with zebrafish knockout models. Sci Rep (2016) 6:18597. doi: 10.1038/srep18597
40. Evans DH. Teleost fish osmoregulation: what have we learned since August Krogh, Homer Smith, and Ancel Keys. Am J Physiol Regul Integr Comp Physiol (2008) 295(2):R704–13. doi: 10.1152/ajpregu.90337.2008
41. Hentschel DM, Mengel M, Boehme L, Liebsch F, Albertin C, Bonventre JV, et al. Rapid screening of glomerular slit diaphragm integrity in larval zebrafish. Am J Physiol Renal Physiol (2007) 293(5):F1746–50. doi: 10.1152/ajprenal.00009.2007
42. Rider SA, Tucker CS, del-Pozo J, Rose KN, MacRae CA, Bailey MA, et al. Techniques for the in vivo assessment of cardio-renal function in zebrafish (Danio rerio) larvae. J Physiol (2012) 590(8):1803–9. doi: 10.1113/jphysiol.2011.224352
43. Westerfield M ZFIN. The zebrafish book : a guide for the laboratory use of zebrafish Danio (Brachydanio) rerio, 4th ed. Eugene, OR.
44. Schlueter PJ, Sang X, Duan C, Wood AW. Insulin-like growth factor receptor 1b is required for zebrafish primordial germ cell migration and survival. Dev Biol (2007) 305(1):377–87. doi: 10.1016/j.ydbio.2007.02.015
45. Zhang P, Lu L, Yao Q, Li Y, Zhou J, Liu Y, et al. Molecular, functional, and gene expression analysis of zebrafish hypoxia-inducible factor-3alpha. Am J Physiol Regul Integr Comp Physiol (2012) 303(11):R1165–74. doi: 10.1152/ajpregu.00340.2012
Keywords: Stc1a, IGF signaling, Trvp6, calcium uptake, ionocyte
Citation: Li S, Li H, Wang Z and Duan C (2023) Stanniocalcin 1a regulates organismal calcium balance and survival by suppressing Trpv6 expression and inhibiting IGF signaling in zebrafish. Front. Endocrinol. 14:1276348. doi: 10.3389/fendo.2023.1276348
Received: 11 August 2023; Accepted: 09 October 2023;
Published: 26 October 2023.
Edited by:
Wei Ge, University of Macau, ChinaReviewed by:
Shaojun Jim Du, University of Maryland, United StatesJianzhen Li, Northwest Normal University, China
Copyright © 2023 Li, Li, Wang and Duan. This is an open-access article distributed under the terms of the Creative Commons Attribution License (CC BY). The use, distribution or reproduction in other forums is permitted, provided the original author(s) and the copyright owner(s) are credited and that the original publication in this journal is cited, in accordance with accepted academic practice. No use, distribution or reproduction is permitted which does not comply with these terms.
*Correspondence: Cunming Duan, cduan@umich.edu
†Present address: Shuang Li, School of Marine Science and Technology, Zhejiang Ocean University, Zhoushan, China
‡These authors have contributed equally to this work