- 1Brain Health Research Institute and Department of Biological Sciences, Kent State University, Kent, OH, United States
- 2School of Biomedical Sciences, Kent State University, Kent, OH, United States
Background: The neuroendocrine control of ovulation is orchestrated by neuronal circuits that ultimately drive the release of gonadotropin-releasing hormone (GnRH) from the hypothalamus to trigger the preovulatory surge in luteinizing hormone (LH) secretion. While estrogen feedback signals are determinant in triggering activation of GnRH neurons, through stimulation of afferent kisspeptin neurons in the rostral periventricular area of the third ventricle (RP3VKISS1 neurons), many neuropeptidergic and classical neurotransmitter systems have been shown to regulate the LH surge. Among these, several lines of evidence indicate that the monoamine neurotransmitter serotonin (5-HT) has an excitatory, permissive, influence over the generation of the surge, via activation of type 2 5-HT (5-HT2) receptors. The mechanisms through which this occurs, however, are not well understood. We hypothesized that 5-HT exerts its influence on the surge by stimulating RP3VKISS1 neurons in a 5-HT2 receptor-dependent manner.
Methods: We tested this using kisspeptin neuron-specific calcium imaging and electrophysiology in brain slices obtained from male and female mice.
Results: We show that exogenous 5-HT reversibly increases the activity of the majority of RP3VKISS1 neurons. This effect is more prominent in females than in males, is likely mediated directly at RP3VKISS1 neurons and requires activation of 5-HT2 receptors. The functional impact of 5-HT on RP3VKISS1 neurons, however, does not significantly vary during the estrous cycle.
Conclusion: Taken together, these data suggest that 5-HT2 receptor-mediated stimulation of RP3VKISS1 neuron activity might be involved in mediating the influence of 5-HT on the preovulatory LH surge.
1 Introduction
Fertility in all mammals is ultimately controlled by gonadotropin-releasing hormone (GnRH) neurons, which drive the release of anterior pituitary gland gonadotropins luteinizing hormone (LH) and follicle-stimulating hormone. In females of spontaneously ovulating species, the rise in circulating estrogen (E) concentration stimulates the mid-cycle surge in GnRH and LH secretion that causes ovulation (1). Because GnRH neurons do not express E receptor α (ERα), the receptor required for the preovulatory surge (2–6), these cells rely on afferent ERα-expressing neurons, the GnRH neuronal network, to relay E feedback information (7). An important part of this network for mediating preovulatory E positive feedback is the preoptic area (POA) subpopulation of kisspeptin (KISS1) neurons found in two contiguous nuclei – the anteroventral periventricular nucleus and the periventricular nucleus – referred to together as the rostral periventricular area of the third ventricle (RP3V) (8, 9). RP3VKISS1 neurons express ERα, undergo physiological changes in response to E positive feedback, project to GnRH neurons and can drive GnRH neuron action potential firing and surge-like LH secretion [reviewed in (10–12)].
Although E positive feedback is an obligatory determinant of the preovulatory surge in spontaneous ovulators (1), many other cues regulate this neuroendocrine event through actions of neuropeptides and neurotransmitters on the GnRH neuronal network [for reviews see (7, 13–22)]. Among these, many lines of evidence indicate that the monoamine neurotransmitter serotonin (5-hydroxytryptamine, 5-HT) plays a role in the LH surge in rodents. Lesions of the dorsal raphe nucleus (DR), which comprises 5-HT-producing neurons, inhibit the LH surge (23–26). Further suggesting a role for endogenously released 5-HT, depletion of 5-HT and 5-HT receptor antagonism both suppress the LH surge (24, 27–31). Moreover, blockade of type 2 5-HT (5-HT2) receptors prevents the surge (32–34) while activation of 5-HT2 receptors is sufficient to restore the surge in animals with a DR lesion (26), indicating that 5-HT2 receptors might play a central role.
The mechanisms through which 5-HT might regulate the LH surge, however, are not fully understood. While evidence suggests that 5-HT neurons might influence the surge via indirect mechanisms (35–37), a direct influence on the GnRH neuronal network may also be at play. Indeed, 5-HT neuronal fibers are found in the RP3V (38–40) as well as in the vicinity of GnRH neurons in the POA (41), some of which originate in the DR (42). These projections might be important seeing that 5-HT release in the hypothalamus, including the POA, increases during the surge and that neurotoxic lesion of 5-HT neuron fibers in the POA prevents the surge (28, 43). At the cellular level, 5-HT can increase or decrease action potential firing and GnRH release via activation of multiple 5-HT receptors in immortalized GnRH neurons maintained in culture (44, 45). Some of these effects are seen in native GnRH neurons recorded in brain slices, where, curiously, most (≈75%) female GnRH neurons are inhibited by 5-HT, via a 5-HT1 receptor-mediated mechanism, whereas only a subset exhibits 5-HT2 receptor-mediated increases in activity (46). The impact of 5-HT neurotransmission on the LH surge, therefore, cannot be fully accounted for by effects of this neurotransmitter on GnRH neurons.
We hypothesized here that the impact of 5-HT on the LH surge is mediated, at least in part, through regulation of RP3VKISS1 neurons upstream of GnRH neurons. Using GCaMP-based calcium imaging and electrophysiology in brain slices, we investigated the effect of 5-HT on RP3VKISS1 neuron activity and the role of 5-HT2 receptors therein.
2 Materials and methods
2.1 Animals
Mice expressing the genetically encoded calcium indicator GCaMP6f (47) in kisspeptin neurons were generated by crossing mice that express the Cre recombinase enzyme (Cre) in kisspeptin cells (Kiss1-Cre; Jackson laboratory stock #023426) (48) with mice that express Cre-dependent GCaMP6f at the ROSA26 locus (flox-STOP-GCaMP6f; Jackson laboratory stock #028865) (49). Male and female offspring heterozygous for the Kiss1-Cre and flox-STOP-GCaMP6f alleles (Kiss1-Cre::GCaMP6f) were used in calcium imaging experiments. Female mice expressing the humanized renilla green fluorescent protein (hrGFP) in kisspeptin neurons (Kiss1-hrGFP; Jackson laboratory stock #023426) (50) were used in electrophysiology experiments. All experimental mice were adults (2-6 months). Female estrous cycle stage was determined by vaginal lavage (5 µL H2O) taken between zeitgeber time (ZT) 1 and 3 or post-mortem (ZT2.5-5.5). Aqueous vaginal smears were stained with methylene blue and cytology examined under light microscopy to assess estrous cycle stage (51). Mice were group-housed with littermates under controlled temperature (23 ± 2°C) and lighting (12h light/dark) conditions with ad libitum access to food and water. Mice were assigned to experiments based on their genotype, sex, and on their estrous cycle stage as needed. All experiments were approved by Kent State University’s Institutional Animal Care and Use Committee.
2.2 Fixed brain slice preparation and imaging
To visualize the distribution of GCaMP6f-expressing neurons in the RP3V of male and female mice, two male and two female (one diestrus and one proestrus) Kiss1-Cre::GCaMP6f mice were deeply anesthetized via intraperitoneal injection of 3 mg/mL pentobarbital before being perfused through the heart with 4% paraformaldehyde (PFA). The brains were then dissected out and placed in 4% PFA for 1 hour before being transferred to 20% sucrose in 0.1 M phosphate-buffered saline. The brains were then cut in 3 series of coronal sections (30 μm thick) using a freezing microtome. The tissue was mounted onto superfrost charged slices, air dried, and coverslipped using an aqueous mounting medium (ProLong™ Gold antifade mountant, Thermofisher). Epifluorescence images were taken in the RP3V region using a DMR microscope (Leica Microsystems) equipped with an ORCA-FLASH 4.0 V3 Digital CMOS (Hamamatsu, Japan), controlled by the MicroBrightField Neurolucida Software (MBF Bioscience).
2.3 Live brain slice preparation
Brain slices were prepared as previously described (52, 53). Briefly, mice were decapitated following isoflurane anesthesia, and their brains quickly removed. Coronal brain slices (200 μm thick) containing the RP3V were cut using a vibrating blade microtome (HM650V, Microm International GmbH) in an ice-cold solution containing (in mM): 87 NaCl, 2.5 KCl, 25 NaHCO3, 1.25 NaH2PO4, 0.5 CaCl2, 6 MgCl2, 25 glucose and 75 sucrose. Brain slices were left to incubate at 30-34°C for at least 1 hour in artificial cerebrospinal fluid (aCSF) containing (in mM): 125 NaCl, 2.5 KCl, 26 NaHCO3, 1.25 NaH2PO4, 2.5 CaCl2, 1.2 MgCl2 and 11 glucose. All solutions were equilibrated to pH 7.4 with a mixture of 95% O2/5% CO2. Female mice were killed between ZT2.5 and 5.5 and males between ZT2 and 4.5.
2.4 Calcium imaging and electrophysiology
Individual brain slices obtained from Kiss1-Cre::GCaMP6f or Kiss1-hrGFP mice were placed under an upright epifluorescence microscope (either Scientifica, UK or Prior Scientific, UK) and constantly perfused (1.5 mL/min) with warm (32-34°C) aCSF.
Variations in intracellular calcium concentration ([Ca2+]i) in RP3VKISS1 neurons were estimated by measuring fluorescence changes in individual GCaMP6f-expressing RP3V neurons in slices from Kiss1-Cre::GCaMP6f mice. Slices were illuminated through a 40x immersion objective, using a light-emitting diode light source (pE300ultra LED; CoolLED, UK) filtered for blue light excitation (460-487 nm; Semrock, USA). Epifluorescence (emission 500-546 nm; Semrock, USA) was collected using an ORCA-FLASH 4.0 LT+ CMOS camera (Hamamatsu). LED and camera were controlled and synchronized with the μ-manager 1.4 software (54). After a ≥15-minute stabilization period in the recording chamber, a focal plane including several fluorescent cell bodies was chosen and acquisitions (100 ms light exposure at 2 Hz for 10-15 minutes) started. Low intensity LED illumination was used (≈0.1 to 0.8 mW) to minimize GCaMP6f photobleaching.
RP3VKISS1 neuron spontaneous action potential firing was recorded in brain slices from Kiss1-hrGFP mice. GFP-expressing RP3V neurons were visualized using brief LED illumination (excitation and emission as above) and subsequently approached with glass recording micropipettes using infrared differential interference contrast illumination. Recording micropipettes (tip resistance: 3-6 MΩ) were made with borosilicate glass (cat. #BF150-110-7.5, Sutter Instruments, USA), pulled using a Model P-1000 micropipette puller (Sutter Instruments). Action potential firing was recorded in voltage-clamp mode (no holding potential applied) in the minimally invasive cell-attached configuration (12-30 MΩ initial seal resistance). Glass micropipettes were filled with aCSF and the recording configuration was achieved by applying the lowest amount of suction required to detect spontaneous, fast, downward deflections in the current trace (spikes), which correspond to single action potentials (55). Electrical signals were recorded, filtered at 2 kHz, and digitized at 10 to 20 kHz using a double integrated patch amplifier (Sutter Instruments, USA), and acquired with the SutterPatch software (Sutter Instruments, USA).
All calcium imaging and electrophysiology experiments were performed between ZT3.5 and 10.
2.5 Drug applications
All drugs were dissolved to the appropriate stock concentration in water or in DMSO, aliquoted and stored at -20°C. Stock were diluted to working concentrations in aCSF prior to performing experiments. Final DMSO concentration never exceeded 0.1%. All drugs were bath-applied. Agonists were applied for one or two minutes after a ≥ three-minute baseline period in electrophysiology and in calcium imaging experiments. Antagonists and blockers were applied continuously to the slice for ≥ five minutes before a recording started and for the duration of the recording. In calcium imaging experiments, cell viability was routinely tested at the end of the experiments by applying the glutamate receptor agonists (S)-α-Amino-3-hydroxy-5-methyl-4-isoxazolepropionic acid (AMPA; 10-20 μM) or L-glutamate (100 μM), or by raising extracellular [K+] (+ 10 mM KCl).
AMPA (cat. #0254), the 5-HT2 receptor antagonists 6-[2-[4-[Bis(4-fluorophenyl)methylene]-1-piperidinyl]ethyl]-7-methyl-5H-thiazolo[3,2-a]pyrimidin-5-one (ritanserin; cat. #1955) and 1,2,3,4,10,14b-Hexahydro-2-methyldibenzo[c,f]pyrazino[1,2-a]azepine hydrochloride (mianserin; cat. #0997), the 5-HT2A receptor agonist (4-Bromo-3,6-dimethoxybenzocyclobuten-1-yl)methylamine hydrobromide (TCB-2; cat. #2592), the 5-HT2C receptor agonist 8,9-Dichloro-2,3,4,4a-tetrahydro-1H-pyrazino[1,2-a]quinoxalin-5(6H)-one hydrochloride (WAY161503; cat. #1801), the 5-HT3 receptor agonist 1-(6-Chloro-2-pyridinyl)-4-piperidinamine hydrochloride (SR57227; cat. #1205), the 5-HT4 receptor agonist (±)-4-Amino-5-chloro-N-[1-[(3R*,4S*)-3-(4-fluorophenoxy)propyl]-3-methoxy-4-piperidinyl]-2-methoxybenzamide (cisapride; cat. #1695), the 5-HT6 receptor agonist 3-[(-3-Fluorophenyl)sulfonyl]-N,N-dimethyl-1H-pyrrolo[2,3-b]pyridine-1-ethanamine dihydrochloride (WAY208466; cat. #3904), the 5-HT7 receptor agonist (2S)-5-(1,3,5-Trimethylpyrazol-4-yl)-2-(dimethylamino)tetralin (AS19; cat. #1968) and 5-HT hydrochloride (cat. #3547) were purchased from Tocris (Bio-techne, USA). L-glutamate (cat. #HB0383) was purchased from HelloBio (USA) and tetrodotoxin citrate (cat. #T-550) from Alomone labs (Israel).
2.6 Analysis
For calcium imaging, GCaMP6f fluorescence image time-series were processed in the FIJI software (56). Regions of interest (ROIs) were drawn around individual, in-focus fluorescent somata [12.1 ± 0.5 (ranging 4 to 24) and 9.2 ± 1.0 (ranging 7 to 14) ROIs per slice in females and males, respectively]. Mean fluorescence intensity within each ROI was measured in each frame. Fluorescence intensity data were analyzed using scripts written in R (http://www.r-project.org/). For each ROI, normalized fluorescence was calculated as , where F is the baseline fluorescence intensity calculated as the mean fluorescence intensity over a one-minute period preceding agonist applications and Ft is the fluorescence measured at any time point. Time-dependent bleaching was corrected by subtracting the normalized fluorescence obtained in an ROI that did not contain a fluorescent cell within the RP3V (empty ROI). This approach could not be used for recordings of the effect of AMPA, L-glutamate or KCl as these treatments induced very large increases in fluorescence that contaminated the empty ROI, thereby causing unacceptable distortions of traces upon subtraction. ROIs in which normalized fluorescence traces reversibly increased from baseline for a period of at least 2 minutes, around the time of agonist application, were recorded as being excited. For illustration and statistical comparisons, agonist effects were calculated as the mean normalized fluorescence over 30 seconds at the peak of the effect minus that over 30 seconds of baseline.
For electrophysiology, spikes were detected using the threshold crossing method. Spike time stamps were organized into ten-second bins and the mean firing rate calculated for each bin. To determine if 5-HT affected the spontaneous firing of RP3VKISS1 neurons, baseline firing was first measured as the mean firing rate during the two minutes preceding 5-HT application. Recordings in which the mean firing rate changed by greater than twice the standard deviation of baseline (2 × SD) during the five minutes after 5-HT first entered the bath were recorded as displaying a response to 5-HT. For statistical comparisons, firing rates were averaged over a one-minute period during baseline and at the peak of 5-HT effect.
In total, 800 GCaMP6f-expressing cells in 64 slices from 45 female mice and 55 GCaMP6f-expressing cells in 6 slices from 5 male mice were analyzed in calcium imaging experiments, while 17 GFP-expressing cells in 11 slices from 7 female mice were analyzed in electrophysiology experiments. These numbers are broken down by experiments in the results section.
Statistical analyses were performed using Prism 9.0 (GraphPad, USA). Data are reported in the text and tables as mean ± SEM, and in figures as mean ± SEM or ± 95% confidence intervals. Comparisons between two independent groups were made using the Mann-Whitney test and those between two paired groups using the paired t-test or the Wilcoxon signed rank test, as appropriate. Comparisons between multiple groups were made with the Kruskal-Wallis test with Dunn’s post-tests. Comparisons of proportions were made using Fisher’s exact tests. Differences were considered statistically significant for p< 0.05.
3 Results
3.1 5-HT stimulates RP3VKISS1 neuron activity in a sex-dependent, but not estrous cycle-dependent, manner
The effects of 5-HT on RP3VKISS1 neuron activity were assessed using calcium imaging in brain slices from male and female Kiss1-Cre::GCaMP6f mice (Figures 1A, B). In slices from female mice, two-minute bath applications of 5-HT (10 μM) resulted in transient increases in GCaMP6f fluorescence in the majority of RP3VKISS1 neurons (Figures 1C, E). In slices obtained from male Kiss1-Cre::GCaMP6f mice, 5-HT-induced increases in fluorescence could also be observed (Figures 1D, F), but these responses were seen much less frequently (see below).
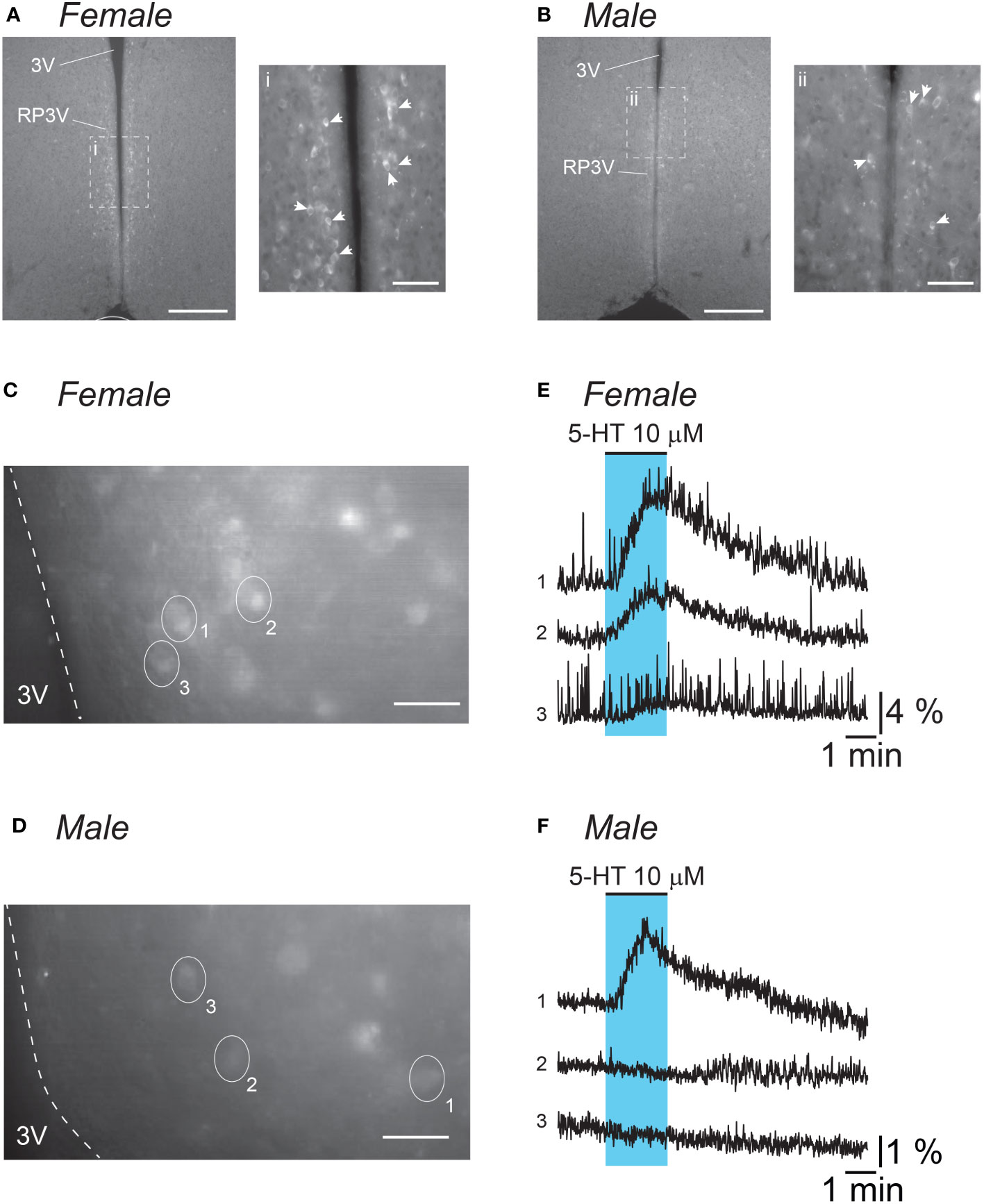
Figure 1 5-HT stimulates RP3VKISS1 neuron activity. (A, B) Low magnification (10X) epifluorescence images of endogenous GCaMP6f fluorescence in fixed coronal slices (30 μm thick) including the RP3V from a diestrous female (A) and a male (B) mouse. Insets i and ii are 20X magnification images of areas framed (dashed lines) in the low magnification images. Similar GCaMP6f-expressing cell distribution was observed in two different animals of each sex. White arrowheads indicate individual GCaMP6f-expressing neurons. 3V: third ventricle. Scale bars are 200 μm (50 μm in i and ii). (C, D) Epifluorescence images (40X magnification, average projection of 240 frames) of endogenous GCaMP6f fluorescence in acute coronal slices (200 μm thick) including the RP3V obtained from an estrous female (C) and a male (D) mouse. Ovals and numbers indicate individual regions of interest (ROIs) each including a single cell. Dashed lines delineate the border of the 3V. Scale bars 25 μm. (E, F) Normalized fluorescence traces for the ROIs delineated in C and D. Blue shading indicates the timing of 5-HT bath applications. Please note the difference in scale for normalized fluorescence in female (E) versus male traces (F).
Because 5-HT signaling may be involved in the generation of the LH surge, we examined RP3VKISS1 neuron responses to 5-HT across the estrous cycle. As illustrated in Figure 2A, 5-HT-induced increases in RP3VKISS1 neuron fluorescence could be seen in slices from diestrous, proestrous and estrous mice and were larger than those seen in slices from male mice. The proportions of RP3VKISS1 neurons excited by 5-HT were similar at all cycle stages (diestrus: 85.3%, proestrus: 87.5%, estrus: 82.0%; p > 0.18, Fisher’s exact tests) and were significantly higher than in males (29.1%; p < 0.001, Fisher’s exact tests; Figure 2B and Table 1). The magnitude of RP3VKISS1 neuron responses to 5-HT significantly varied across these groups, with male responses significantly smaller than those in females at any estrous cycle stage, but no statistical differences across the estrous cycle (Table 1; Figure 2C). Smaller responses to 5-HT in males versus females could not be explained by a reduced ability of male RP3VKISS1 neurons to mount changes in [Ca2+]i, as responses to the glutamate receptor agonist AMPA were, in fact, larger in males than in females in those slices that were tested in this manner (Table 2).
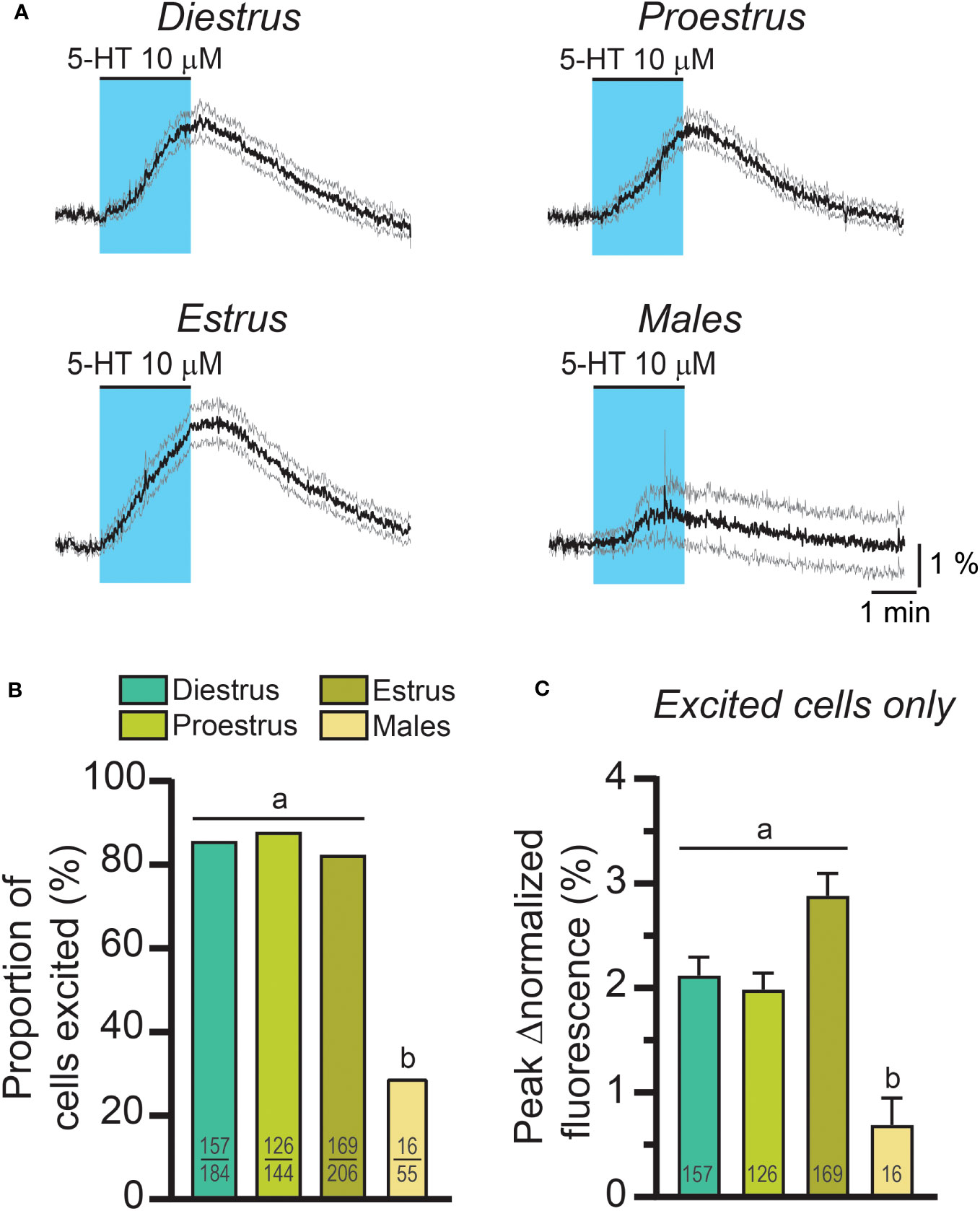
Figure 2 The effect of exogenous 5-HT on RP3VKISS1 neuron activity across the estrous cycle and in males. (A) 5-HT-induced changes in RP3VKISS1 neuron normalized fluorescence across the estrous cycle and in males. Traces are means (black) ± 95% confidence intervals (grey). (B) Similar proportions of RP3VKISS1 neurons were stimulated by 5-HT across the estrous cycle. This proportion was significantly lower in males. Numbers in bars are sample sizes (excited cells/total cells). (C) Peak 5-HT effect did not vary as a function of estrous cycle stage but was significantly smaller in males. Numbers in bars are sample sizes. Letters indicate results of statistical tests [Fisher’s exact tests in (B) Kruskal-Wallis and Dunn’s post-tests in (C)]. Bars with different letters are significantly different (p < 0.05; see Table 1 for details).
Together, these observations indicate that RP3VKISS1 neuron responses to exogenous 5-HT are – to some extent – sex-dependent, being more prominent in females. Female responses, however, are not affected by the estrous cycle. The remainder of the study was conducted in brain slices from females.
3.2 5-HT increases female RP3VKISS1 neuron action potential firing
We then investigated if the effect of 5-HT was associated with changes in electrical activity in RP3VKISS1 neurons. In slices from female Kiss1-hrGFP mice, we recorded individual RP3V GFP-expressing neurons in the cell-attached configuration. Bath applications of 5-HT (one minute, 50 μM) increased action potential firing in just over half (52.9%) of RP3VKISS1 neurons (9 out of 17 neurons in 8 slices from 5 mice [1 di- and 4 estrus]; Figures 3A, C), decreased it in a few cells (2 cells [11.8% of total] in 2 slices from 2 mice [1 di- and 1 estrus]; Figures 3B, C), or did not affect firing (6 neurons [35.3% of total] in 5 slices from 4 mice [1 pro-, 1 di- and 2 estrus]). In those RP3VKISS1 neurons that were stimulated by 5-HT, action potential firing increased from 2.20 ± 0.42 to 6.33 ± 1.40 Hz (p < 0.01, t = 3.66, df = 8, paired t-test; Figure 3D). In the two cells inhibited by 5-HT, spontaneous firing (0.73 and 0.12 Hz) was transiently silenced in both cases.
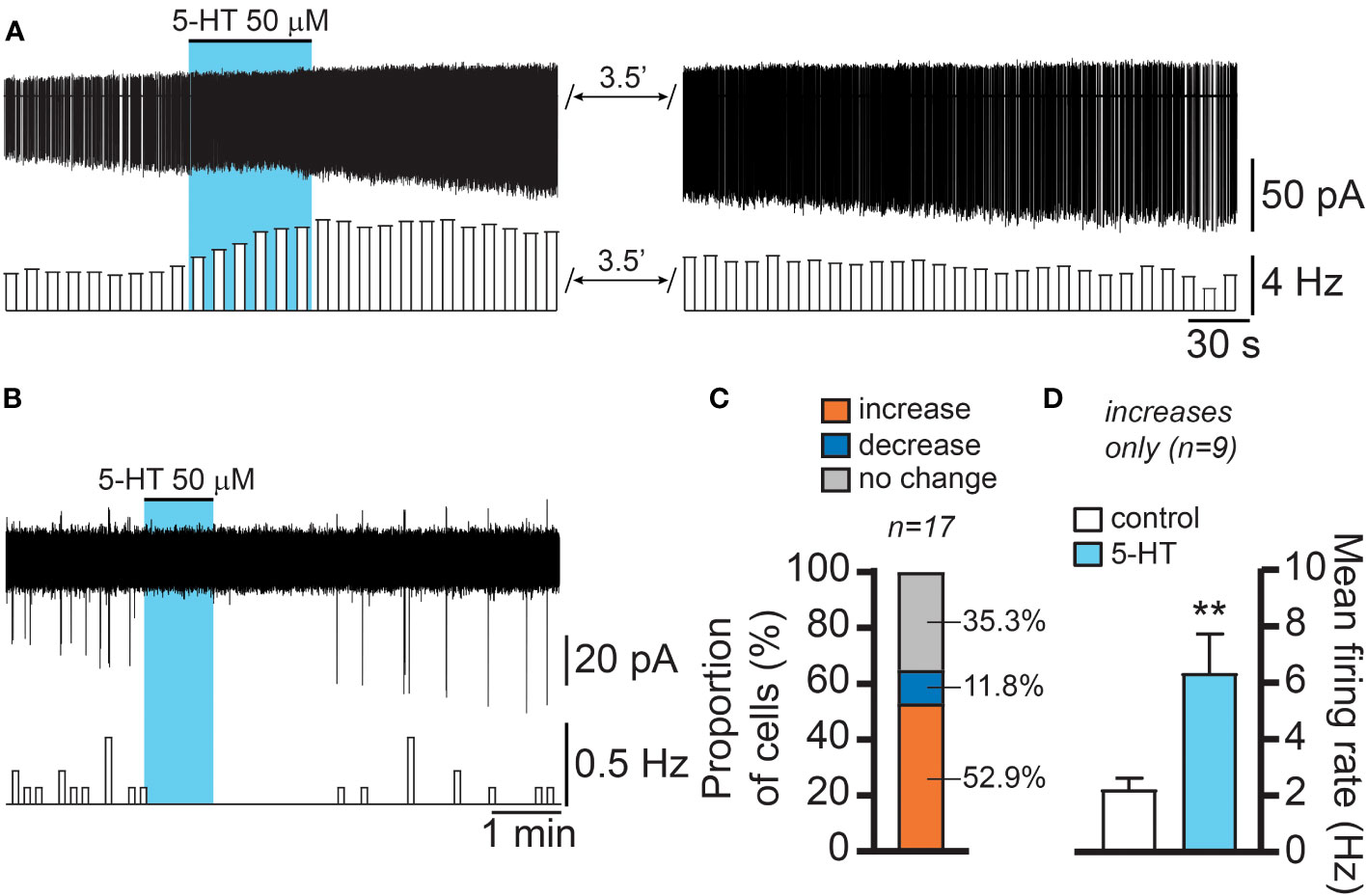
Figure 3 5-HT effects on female RP3VKISS1 neuron action potential firing. (A, B) Example traces and corresponding rate-meters illustrating the excitatory (A) and inhibitory (B) effects of 5-HT on female RP3VKISS1 neuron firing. Blue shading indicates the timing of 5-HT bath applications. (C) Proportions of RP3VKISS1 neurons that showed increased, decreased or no change in firing in response to 5-HT. (D) In those cells that were excited by 5-HT, the increase in firing was statistically significant. **p < 0.01 paired t-test. Numbers above bars are sample sizes.
These observations indicate that 5-HT primarily stimulates RP3VKISS1 neuron action potential firing. While 5-HT could also suppress firing in RP3VKISS1 neurons, this was seen much less frequently.
3.3 The effect of 5-HT on female RP3VKISS1 neuron [Ca2+]i is direct
We next tested whether the effect of 5-HT is direct or mediated through the release of an intermediary factor by cells within the slice. To do this, we used a protocol in which slices from Kiss1-Cre::GCaMP6f females were exposed to 5-HT (two minutes, 10 μM) twice at a 15- to 20-minute interval, the second 5-HT application being carried out in the presence of the voltage-gated sodium channel inhibitor tetrodotoxin (TTX; 0.5 μM) to block electrical activity in the slice. This concentration of TTX is sufficient to inhibit action potential generation in ex vivo preparations (57–59). As illustrated in Figure 4A, 5-HT-induced increases in RP3VKISS1 neuron fluorescence persisted in the presence of TTX. In control conditions, 57 out of 68 cells (83.8%) were stimulated by 5-HT. In the presence of TTX, 53 of these neurons (77.9% of the total; p = 0.51, Fisher’s exact test; Figure 4B) displayed 5-HT-induced increases in normalized fluorescence. On average, 5-HT increased RP3VKISS1 neuron normalized fluorescence to a similar extent whether in the absence (2.72 ± 0.37%) or in the presence of TTX (2.34 ± 0.37%; n = 57 from 7 slices in 4 mice [2 pro-, 1 di- and 1 estrus]; p = 0.24, Wilcoxon test; Figure 4C).
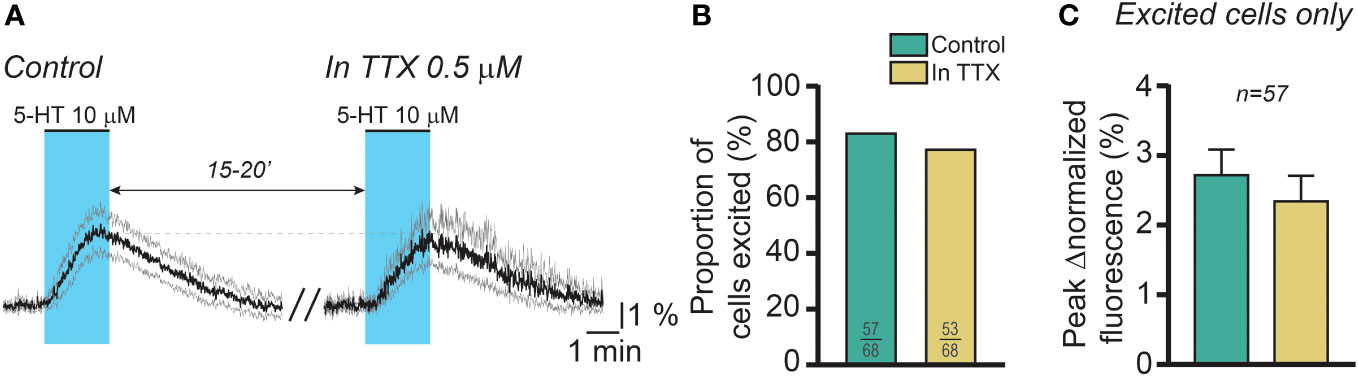
Figure 4 The effect of 5-HT on female RP3VKISS1 neuron activity is direct. (A) 5-HT was applied twice to brain slices from female mice, at 15-to-20-minute intervals. The first application (control; left) was carried out in the absence of drugs whereas the second was conducted in the continuous presence of tetrodotoxin (TTX; right) to block action potentials. Traces are means (black) ± 95% confidence intervals (grey) and include those RP3VKISS1 neurons that were stimulated by 5-HT upon the first application (n = 57). Blue shading indicates the timing of 5-HT bath applications. (B) Similar proportions of RP3VKISS1 neurons were stimulated by 5-HT in the presence and in the absence of TTX. Numbers in bars are sample sizes (excited cells/total cells). (C) The peak magnitude of the 5-HT effect was similar in the presence and in the absence of TTX. Only RP3VKISS1 neurons excited by 5-HT upon the first application were included in this analysis. The number above the bars is the sample size.
This finding indicates that the effect of 5-HT on RP3VKISS1 neuron activity is likely direct.
3.4 Activation of 5-HT2 receptors mimics the effect of 5-HT in female RP3VKISS1 neurons
Because female RP3VKISS1 neurons express genes for multiple stimulatory 5-HT receptor subtypes, including htr2a, htr2c, htr3a, htr4, htr6 and htr7 (60), we next examined if activating these 5-HT receptors could increase [Ca2+]i in female RP3VKISS1 neurons. Increases in RP3VKISS1 neuron activity could be seen in response to bath-applications of the agonists TCB-2 (1 μM; 5-HT2A receptors; 2 di-, 1 pro- and 1 estrous mice), WAY161503 (10 μM; 5-HT2C receptors; 3 diestrous mice), SR57227 (1 μM; 5-HT3 receptors; 4 diestrous mice), cisapride (1 μM; 5-HT4 receptors; 3 diestrous mice), WAY208466 (10 μM; 5-HT6 receptors; 3 diestrous mice) and AS19 (10 μM; 5-HT7 receptors; 1 di-, 3 pro- and 1 estrous mice) (Figures 5A, B and Table 3). However, activating 5-HT2A and 5-HT2C receptors resulted in greater proportions of stimulated RP3VKISS1 neurons (≈55-70%) than activating other receptor subtypes (Figure 5C and Table 3). In addition, activating 5-HT2C receptors resulted in significantly larger responses than activating other 5-HT receptors, whereas responses to 5-HT2A receptor activation were rather moderate in magnitude (Figure 5D and Table 3).
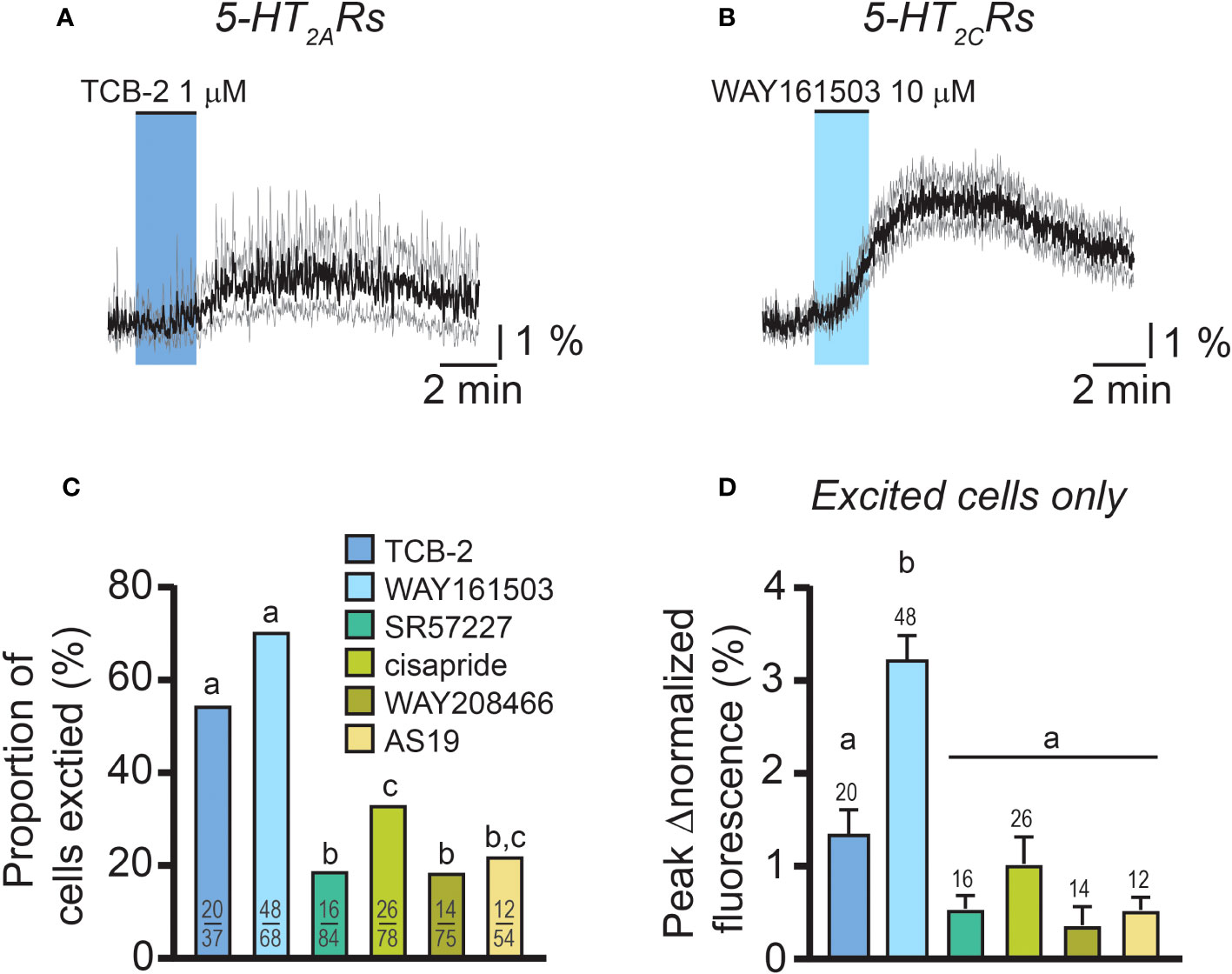
Figure 5 Responses of female RP3VKISS1 neurons to excitatory 5-HT receptor agonists. (A, B) Average responses of female RP3VKISS1 neurons stimulated by 5-HT2A (A) and 5-HT2C (B) receptor agonists. Colored shading indicates the timing of agonist applications. Traces are means (black) ± 95% confidence intervals (grey). (C) Proportions of female RP3VKISS1 neurons stimulated by 5-HT receptor agonists. Numbers in bars are sample sizes (excited cells/total cells). (D) Magnitude of the effect of different 5-HT receptor agonists. Only RP3VKISS1 neurons that responded to agonists were included in this analysis. Numbers above bars are sample sizes. Letters above bars represent the results of statistical tests [Fisher’s exact tests in (C) and Kruskal-Wallis and Dunn’s post-tests in (D)]. Bars that do not share a letter are significantly different (p < 0.05, see Table 3 for details).
Taken together, these observations indicate that the female RP3VKISS1 neuron population expresses multiple functional excitatory 5-HT receptor subtypes. However, of these, activation of 5-HT2 receptors, in particular 5-HT2C, most closely mimics the effect of 5-HT on female RP3VKISS1 neurons.
3.5 5-HT stimulates female RP3VKISS1 neuron activity via activation of 5-HT2 receptors
Based on these findings, we then sought to determine the role of 5-HT2 receptors in mediating the effect of 5-HT on RP3VKISS1 neuron activity. Using a dual 5-HT application protocol like that described above, we tested the impact of 5-HT2 receptor antagonists on RP3VKISS1 neuron responses to 5-HT. As illustrated in Figure 6A, the antagonist ritanserin (5 μM), which prevents the LH surge in rats (34), almost completely blocked the effect of 5-HT in RP3VKISS1 neurons. 69 out of 85 cells (81.2%) were stimulated by 5-HT in control conditions. In the presence of ritanserin, 31 of these (36.5% of the total; p < 0.001, Fisher’s exact test) had 5-HT-induced increases in normalized fluorescence (Figure 6B). On average, 5-HT-induced changes in RP3VKISS1 neuron normalized fluorescence were substantially reduced by ritanserin (0.29 ± 0.10% versus 3.37 ± 0.32% in the absence of antagonist; n = 69 in 5 slices from 5 mice [2 pro-, 2 di- and 1 estrus]; p < 0.001, sum of signed ranks (W) = -2387, Wilcoxon test; Figure 6C). The same held true when only those cells that were excited by 5-HT in the presence of ritanserin were considered (Table 4). Similar results were obtained with the antagonist mianserin (10 μM). In its presence, only 8 out of 75 cells (10.7% versus 80.0% [60 out of 75] in control; p < 0.001, Fisher’s exact test; Figure 6D) were excited by 5-HT. Moreover, 5-HT-induced increases in normalized fluorescence were significantly suppressed by mianserin (-0.53 ± 0.22% versus 2.61 ± 0.42% in the absence of antagonist; n = 60 in 6 slices from 5 mice [1 pro-, 2 di- and 2 estrus]; p < 0.001, sum of signed ranks (W) = -1718, Wilcoxon test; Figure 6E), even when only cells stimulated by 5-HT in the presence of the antagonist were considered (Table 4).
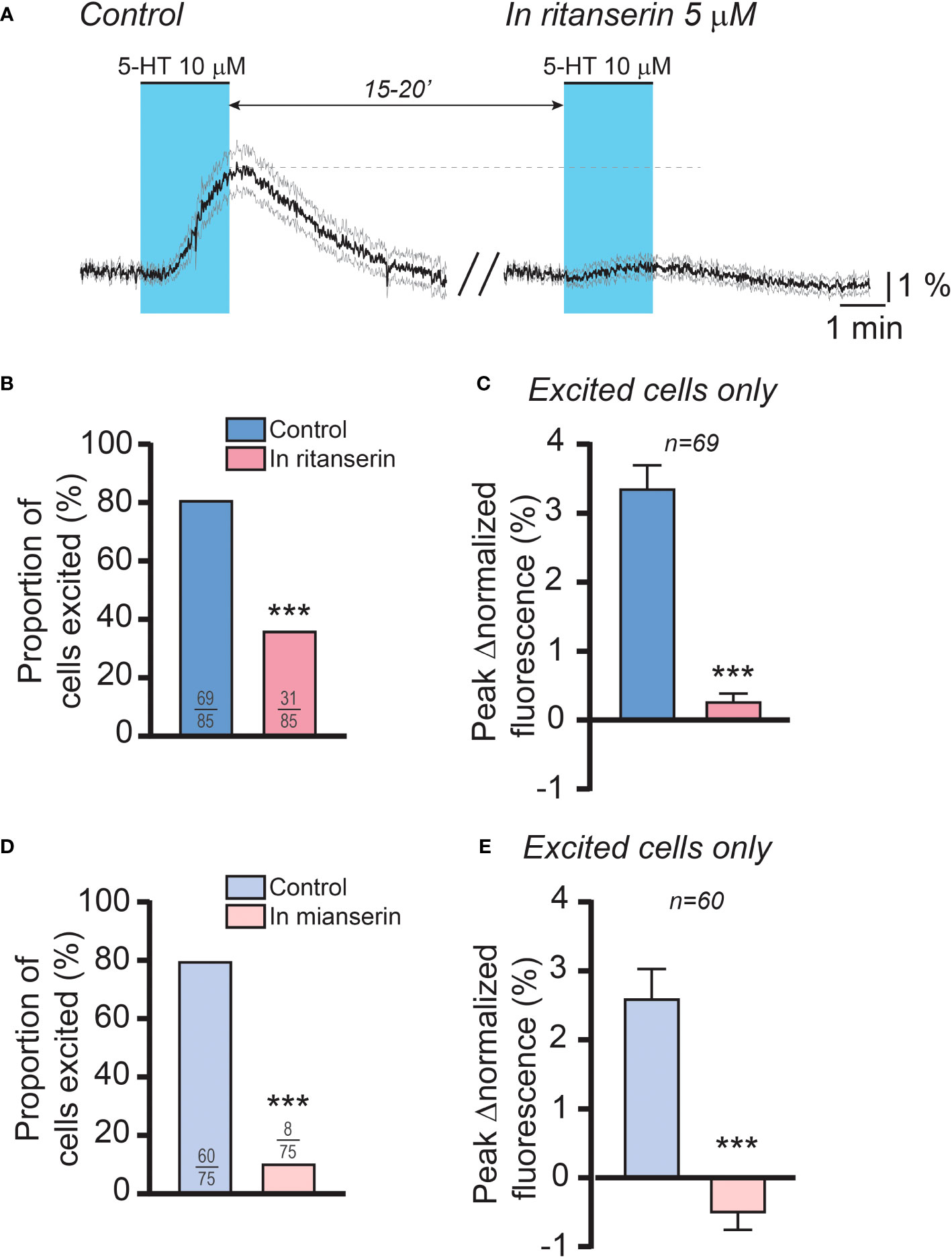
Figure 6 5-HT2 receptors mediate the effect of 5-HT on female RP3VKISS1 neuron activity. (A) 5-HT was applied twice to female brain slices, at 15-to-20-minute intervals. The first application (control; left) was carried out in the absence of drugs whereas the second was conducted in the continuous presence of ritanserin (right), a 5-HT2 receptor antagonist. Light blue shading indicates the timing of 5-HT bath applications. Traces are means (black) ± 95% confidence intervals (grey). (B) Proportions of RP3VKISS1 neurons stimulated by 5-HT significantly decreased in the presence of ritanserin. ***p < 0.001, Fisher’s exact test. Numbers in bars are sample sizes (excited cells/total cells). (C) Ritanserin significantly decreased the peak magnitude of the 5-HT effect. ***p < 0.001, Wilcoxon signed rank test. Number above bars is the sample size. (D) Mianserin significantly reduced the proportions of RP3VKISS1 neurons stimulated by 5-HT. ***p < 0.001, Fisher’s exact test. Numbers in bars are sample sizes (excited cells/total cells). (E) The peak magnitude of the 5-HT effect was significantly suppressed by mianserin. ***p < 0.001, Wilcoxon signed rank test. Number above bars is the sample size. Only RP3VKISS1 neurons that responded to 5-HT upon the first bath application were included in the analyses displayed in (C, E).

Table 4 Excitatory effects of 5-HT in the presence of 5-HT2 receptor antagonists ritanserin or mianserin.
Together with the results obtained using 5-HT receptor agonists, these data indicate that the effect of 5-HT on RP3VKISS1 neurons is – for the most part – mediated by 5-HT2 receptors, likely through the combined effect of 5-HT2A and 5-HT2C receptor activation.
4 Discussion
We report here using GCaMP-based [Ca2+]i imaging and electrophysiology that exogenous 5-HT stimulates the activity of a majority of RP3VKISS1 neurons in female mice. This effect is observed in higher proportions of RP3VKISS1 neurons – and with larger magnitudes – in females than in males. In females, however, the effect of 5-HT does not significantly vary between estrous cycle stages. Lastly, we find that 5-HT-induced excitations are likely mediated directly at RP3VKISS1 neurons and that these effects require activation of 5-HT2 receptors. Together, these observations suggest that stimulation of RP3VKISS1 neurons might be a mechanism through which 5-HT influences the LH surge in rodents.
A large proportion of female RP3VKISS1 neurons (> 80%) exhibited increases in [Ca2+]i in response to 5-HT in brain slices from Kiss1-Cre::GCaMP6f mice. In slices from Kiss1-hrGFP mice, 5-HT caused an increase in action potential firing in > 50% of RP3VKISS1 neurons. Increases in [Ca2+]i induced by 5-HT likely result from opening of voltage-gated Ca2+ channels in response to action potential firing and/or to subthreshold membrane depolarization, from G-protein-dependent Ca2+ release from internal stores, or from a combination thereof (10, 61). As the cell-attached patch-clamp recording configuration does not give access to subthreshold membrane potential fluctuations – nor to changes in [Ca2+]i – the proportions of neurons that responded to 5-HT applications with elevations in [Ca2+]i and in firing rates cannot be directly compared. Interestingly, 5-HT suppressed firing in a small subset (≈ 10%) of RP3VKISS1 neurons in electrophysiology experiments. It is possible that such responses were not seen with our [Ca2+]i imaging because, at least under our recording conditions, this approach does not effectively resolve inhibitions in those cells that display low resting activity levels (10, 61). Nevertheless, our observation that 5-HT stimulated activity in a majority of RP3VKISS1 neurons is in line with a recent report that 5-HT increases firing in arcuate kisspeptin (ARCKISS1) neurons (62), suggesting that stimulation by 5-HT is a common feature of hypothalamic kisspeptin neurons. Somewhat contrastingly, only a small proportion of adult female GnRH neurons (< 40%) are stimulated by 5-HT, whereas most (≈ 75%) are inhibited (46).
The stimulatory effect of 5-HT on RP3VKISS1 neuron [Ca2+]i was largely resistant to blockade of action potential firing, suggesting that responses to 5-HT were mediated primarily through action potential-independent VGCC opening and/or mobilization of intracellular Ca2+ stores. This also reveals that RP3VKISS1 neuron [Ca2+]i responses to 5-HT were independent on electrical activity within the brain slice and, therefore, resulted from direct 5-HT actions at the kisspeptin neurons. Moreover, RP3VKISS1 neurons displayed excitatory responses to 5-HT2A, 5-HT2C, 5-HT3, 5-HT4, 5-HT6 and 5-HT7 receptor activation. This reveals that RP3VKISS1 neurons may express multiple functional 5-HT receptor subtypes. However, activation of 5-HT2C and – to a lesser extent – 5-HT2A receptors most closely mimicked the stimulatory effect of 5-HT. Importantly, the stimulatory effect of 5-HT was blocked by the 5-HT2 receptor antagonists ritanserin and mianserin. Together, these observations indicate that 5-HT stimulates RP3VKISS1 neuron activity by acting at 5-HT2 receptors, including 5-HT2A and 5-HT2C receptors. 5-HT is also reported to stimulate the activity of ARCKISS1 neurons, but this is mostly mediated by 5-HT4 receptors (62). The two main populations of hypothalamic kisspeptin neurons may, therefore, have different functional 5-HT receptor make-ups. Nevertheless, our observations in RP3VKISS1 neurons are in line with previous reports that ritanserin prevents the LH surge in rats (33, 34), and that activation of 5-HT2A and 5-HT2C receptors restores the LH surge in DR-lesioned female rats (26). Seeing the pattern and pharmacology of RP3VKISS1 neuron responses to 5-HT – and, as discussed above, that 5-HT may primarily inhibit female GnRH neurons (46) – we propose that the stimulatory effect of 5-HT we report here might be involved in mediating the previously reported excitatory influence of 5-HT neurotransmission on the LH surge (27–30, 32–34).
5-HT responses were found in a substantially and significantly lower proportion of RP3VKISS1 neurons in males (≈ 35%) than in females. Further, those male RP3VKISS1 neurons that were stimulated by 5-HT displayed much smaller responses than their female counterparts. Although we cannot fully rule out that a proportion of the GCaMP6f-expressing cells that we recorded in slices from male mice were RP3V neurons that do not, in fact, express Kiss1 (10, 63–65), this suggests that 5-HT excitatory effects in RP3VKISS1 neurons display some degree of sex-dependence. As the LH surge mechanism, including many aspects of the RP3VKISS1 → GnRH neural circuit, is sexually differentiated in rodents (66–69), this observation suggests that 5-HT signaling in RP3VKISS1 neurons might be part of the mechanism activating these cells for the surge. On the other hand, we find that the impact of 5-HT on RP3VKISS1 neuron activity did not significantly change between estrous cycle stages, with similar proportions of neurons stimulated and similar response magnitude. This suggests that regulation of 5-HT2 receptor function in RP3VKISS1 neurons might not be a mechanism through which these cells are specifically activated for the proestrous surge. Rather, this suggests that 5-HT might have a permissive effect on RP3VKISS1 neuron activity as it does on the surge in vivo (15). On the other hand, 5-HT content and turnover in the hypothalamus, including the POA, might fluctuate around the time of the proestrous surge in a diurnal manner (28, 70, 71). Whether 5-HT release in the vicinity of RP3VKISS1 neurons fluctuates in a time- and estrous cycle-dependent manner is unknown but would be anticipated to affect RP3VKISS1 neuron activity.
5-HT-containing fibers are detected within the RP3V in male and female rats (38–40), but it is unknown whether these fibers are in the vicinity of kisspeptin neurons. The origin of these fibers is also unknown. They may originate in the DR as lesions of this area, more so than lesions of the medial raphe nucleus, prevent the LH surge (23–26). Curiously, electrical stimulation of the DR fails at altering the LH surge in proestrous rats (24), whereas this manipulation can evoke LH secretion in E-replaced OVX rats (72) and result in a subtle prolongation of LH secretion evoked by stimulation of the POA (73). DR neurons project directly to POA GnRH neurons (42); whether they project to RP3VKISS1 neurons will need to be established by tract-tracing experiments. It should be noted that 5-HT transporter-immunoreactive fibers are seen in close apposition to ARCKISS1 neurons (62). As ARCKISS1 neurons might be involved in regulating the LH surge (74–78), it is possible that these fibers contribute to the influence of 5-HT on the surge. As discussed above, the pharmacology of ARCKISS1 neuron responses to 5-HT does not match that of the influence of 5-HT on the surge, however.
Lastly, the type of information that 5-HT neurons relay to the GnRH neuronal network to regulate the surge is unknown. 5-HT may mediate the permissive effect of the metabolic hormone leptin on the hypothalamic-pituitary-gonadal axis (79) and, indeed, the DR is involved in many aspects of energy balance (80). Additionally, 5-HT signaling may be involved in regulating circadian rhythms and responses to stressors (81–83), which also affect LH secretion and the surge (20, 21). Further studies will be required to determine the precise contribution of changes in 5-HT neurotransmission in regulating the surge and whether this involves RP3VKISS1 neurons.
In conclusion, the findings reported here regarding the stimulatory effect of 5-HT signaling on RP3VKISS1 neuron activity contribute to our understanding of the brain circuits that control ovulation in females.
Data availability statement
The raw data supporting the conclusions of this article will be made available by the authors, without undue reservation.
Ethics statement
The animal study was approved by Kent State University Institutional Animal Care and Use Committee. The study was conducted in accordance with the local legislation and institutional requirements.
Author contributions
RP designed the research. CB, RB, AN, AA and JD carried out the experiments. CB, RB, AN, AA, and RP analyzed the data. RP wrote the manuscript. All authors contributed to the article and approved the submitted version.
Funding
This work was supported by NICHD grant R01HD109337 to RP. Publication of this study was supported by the Department of Biological Sciences and the Open Access Publishing Fund at Kent State University.
Acknowledgments
We thank Drs. Bradley Jamieson and Aleisha Moore as well as members of the Piet laboratory for their comments on an earlier draft of this manuscript, Conor McDevitt for technical assistance and Dr. Aleisha Moore for helping with taking pictures of GCaMP6f endogenous fluorescence in fixed tissue.
Conflict of interest
The authors declare that the research was conducted in the absence of any commercial or financial relationships that could be construed as a potential conflict of interest.
Publisher’s note
All claims expressed in this article are solely those of the authors and do not necessarily represent those of their affiliated organizations, or those of the publisher, the editors and the reviewers. Any product that may be evaluated in this article, or claim that may be made by its manufacturer, is not guaranteed or endorsed by the publisher.
References
1. Goodman RL, Herbison AE, Lehman MN, Navarro VM. Neuroendocrine control of gonadotropin-releasing hormone: pulsatile and surge modes of secretion. J Neuroendocrinol (2022) 34(5):e13094. doi: 10.1111/jne.13094
2. Herbison AE, Robinson JE, Skinner DC. Distribution of estrogen receptor-immunoreactive cells in the preoptic area of the ewe: co-localization with glutamic acid decarboxylase but not luteinizing hormone-releasing hormone. Neuroendocrinology (1993) 57(4):751–9. doi: 10.1159/000126433
3. Herbison AE, Theodosis DT. Localization of oestrogen receptors in preoptic neurons containing neurotensin but not tyrosine hydroxylase, cholecystokinin or luteinizing hormone-releasing hormone in the male and female rat. Neuroscience (1992) 50(2):283–98. doi: 10.1016/0306-4522(92)90423-y
4. Lehman MN, Ebling FJ, Moenter SM, Karsch FJ. Distribution of estrogen receptor-immunoreactive cells in the sheep brain. Endocrinology (1993) 133(2):876–86. doi: 10.1210/endo.133.2.8344223
5. Wintermantel TM, Campbell RE, Porteous R, Bock D, Grone HJ, Todman MG, et al. Definition of estrogen receptor pathway critical for estrogen positive feedback to gonadotropin-releasing hormone neurons and fertility. Neuron (2006) 52(2):271–80. doi: 10.1016/j.neuron.2006.07.023
6. Glidewell-Kenney C, Hurley LA, Pfaff L, Weiss J, Levine JE, Jameson JL. Nonclassical estrogen receptor alpha signaling mediates negative feedback in the female mouse reproductive axis. Proc Natl Acad Sci U.S.A. (2007) 104(19):8173–7. doi: 10.1073/pnas.0611514104
7. Herbison AE. Physiology of the Adult Gonadotropin-Releasing Hormone Neuronal Network. In: Plant TM, Zeleznik AJ, editors. Knobil and Neill’s Physiology of Reproduction. San Diego: Academic Press (2015). p. 399–467.
8. Clarkson J, Herbison AE. Oestrogen, kisspeptin, gpr54 and the pre-ovulatory luteinising hormone surge. J Neuroendocrinol (2009) 21(4):305–11. doi: 10.1111/j.1365-2826.2009.01835.x
9. Herbison AE. Estrogen positive feedback to gonadotropin-releasing hormone (Gnrh) neurons in the rodent: the case for the rostral periventricular area of the third ventricle (Rp3v). Brain Res Rev (2008) 57(2):277–87. doi: 10.1016/j.brainresrev.2007.05.006
10. Jamieson BB, Piet R. Kisspeptin neuron electrophysiology: intrinsic properties, hormonal modulation, and regulation of homeostatic circuits. Front Neuroendocrinol (2022) 66:101006. doi: 10.1016/j.yfrne.2022.101006
11. Kauffman AS. Neuroendocrine mechanisms underlying estrogen positive feedback and the lh surge. Front Neurosci (2022) 16:953252. doi: 10.3389/fnins.2022.953252
12. Starrett JR, Moenter SM. Hypothalamic kisspeptin neurons as potential mediators of estradiol negative and positive feedback. Peptides (2023) 163:170963. doi: 10.1016/j.peptides.2023.170963
13. Kordon C, Glowinski J. Role of hypothalamic monoaminergic neurones in the gonadotrophin release-regulating mechanisms. Neuropharmacology (1972) 11(2):153–62. doi: 10.1016/0028-3908(72)90088-3
14. Kalra SP, Kalra PS. Neural regulation of luteinizing hormone secretion in the rat. Endocr Rev (1983) 4(4):311–51. doi: 10.1210/edrv-4-4-311
15. Vitale ML, Chiocchio SR. Serotonin, a neurotransmitter involved in the regulation of luteinizing hormone release. Endocr Rev (1993) 14(4):480–93. doi: 10.1210/edrv-14-4-480
16. Herbison AE. Noradrenergic regulation of cyclic gnrh secretion. Rev Reprod (1997) 2(1):1–6. doi: 10.1530/ror.0.0020001
17. Spergel DJ. Modulation of gonadotropin-releasing hormone neuron activity and secretion in mice by non-peptide neurotransmitters, gasotransmitters, and gliotransmitters. Front Endocrinol (Lausanne) (2019) 10:329. doi: 10.3389/fendo.2019.00329
18. Spergel DJ. Neuropeptidergic modulation of gnrh neuronal activity and gnrh secretion controlling reproduction: insights from recent mouse studies. Cell Tissue Res (2019) 375(1):179–91. doi: 10.1007/s00441-018-2893-z
19. Navarro VM. Metabolic regulation of kisspeptin - the link between energy balance and reproduction. Nat Rev Endocrinol (2020) 16(8):407–20. doi: 10.1038/s41574-020-0363-7
20. McCosh RB, O’Bryne KT, Karsch FJ, Breen KM. Regulation of the gonadotropin-releasing hormone neuron during stress. J Neuroendocrinol (2022) 34(5):e13098. doi: 10.1111/jne.13098
21. Piet R. Circadian and kisspeptin regulation of the preovulatory surge. Peptides (2023) 163:170981. doi: 10.1016/j.peptides.2023.170981
22. Iremonger KJ, Constantin S, Liu X, Herbison AE. Glutamate regulation of gnrh neuron excitability. Brain Res (2010) 1364(10 December 2010):35–43. doi: 10.1016/j.brainres.2010.08.071
23. Hery M, Laplante E. Kordon C. Participation of serotonin in the phasic release of luteinizing hormone. Ii. Effects of lesions of serotonin-containing pathways in the central nervous system. Endocrinology (1978) 102(4):1019–25. doi: 10.1210/endo-102-4-1019
24. Morello H, Taleisnik S. Changes of the release of luteinizing hormone (Lh) on the day of proestrus after lesions or stimulation of the raphe nuclei in rats. Brain Res (1985) 360(1-2):311–7. doi: 10.1016/0006-8993(85)91247-8
25. Vitale ML, Villar MJ, Chiocchio SR, Tramezzani JH. Dorsal raphe lesion alters the estrous cycle and the preovulatory gonadotropin release. Neuroendocrinology (1987) 46(3):252–7. doi: 10.1159/000124828
26. Maekawa F, Tsukahara S, Tsukamura H, Maeda KI, Yamanouchi K. Prevention of inhibitory effect of dorsal raphe nucleus lesions on ovulation and lh surge by 5-ht 2a/2c receptor agonists in female rats. Neurosci Res (1999) 35(4):291–8. doi: 10.1016/s0168-0102(99)00090-5
27. Hery M, Laplante E. Kordon C. Participation of serotonin in the phasic release of lh. I. Evidence from pharmacological experiments. Endocrinology (1976) 99(2):496–503. doi: 10.1210/endo-99-2-496
28. Walker RF. Serotonin neuroleptics change patterns of preovulatory secretion of luteinizing hormone in rats. Life Sci (1980) 27(12):1063–8. doi: 10.1016/0024-3205(80)90030-2
29. Horn AM, Fink G. Parachlorophenylalanine blocks the spontaneous pro-oestrous surge of prolactin as well as lh and affects the secretion of oestradiol-17 beta. J Endocrinol (1985) 104(3):415–8. doi: 10.1677/joe.0.1040415
30. Meyer DC, Eadens DJ. The role of endogenous serotonin in phasic lh release. Brain Res Bull (1985) 15(3):283–6. doi: 10.1016/0361-9230(85)90152-2
31. Burri R, Petersen SL, Barraclough CA. Effects of P-chlorophenylalanine on hypothalamic indoleamine levels and the associated changes which occur in catecholamine dynamics and lh surges in estrogen-treated ovariectomized rats. Brain Res (1987) 416(2):267–76. doi: 10.1016/0006-8993(87)90906-1
32. Tanaka E, Baba N, Toshida K, Suzuki K. Evidence for 5-ht2 receptor involvement in the stimulation of preovulatory lh and prolactin release and ovulation in normal cycling rats. Life Sci (1993) 52(7):669–76. doi: 10.1016/0024-3205(93)90459-g
33. Dow RC, Williams BC, Bennie J, Carroll S, Fink G. A central 5-ht2 receptor mechanism plays a key role in the proestrous surge of luteinizing hormone in the rat. Psychoneuroendocrinology (1994) 19(4):395–9. doi: 10.1016/0306-4530(94)90019-1
34. Fink G, Dow RC, McQueen JK, Bennie JG, Carroll SM. Serotonergic 5-ht2a receptors important for the oestradiol-induced surge of luteinising hormone-releasing hormone in the rat. J Neuroendocrinol (1999) 11(1):63–9. doi: 10.1046/j.1365-2826.1999.00299.x
35. Morello H, Taleisnik S. The inhibition of proestrous lh surge and ovulation in rats bearing lesions of the dorsal raphe nucleus is mediated by the locus coeruleus. Brain Res (1988) 440(2):227–31. doi: 10.1016/0006-8993(88)90990-0
36. Morello H, Caligaris L, Haymal B, Taleisnik S. Inhibition of proestrous lh surge and ovulation in rats evoked by stimulation of the medial raphe nucleus involves a gaba-mediated mechanism. Neuroendocrinology (1989) 50(1):81–7. doi: 10.1159/000125205
37. Morello H, Caligaris L, Haymal B, Taleisnik S. The pineal gland mediates the inhibition of proestrous luteinizing hormone surge and ovulation in rats resulting from stimulation of the medial raphe nucleus or injection of 5-ht into the third ventricle. J Neuroendocrinol (1989) 1(3):195–7. doi: 10.1111/j.1365-2826.1989.tb00102.x
38. Simerly RB, Swanson LW. The distribution of neurotransmitter-specific cells and fibers in the anteroventral periventricular nucleus: implications for the control of gonadotropin secretion in the rat. Brain Res (1987) 400(1):11–34. doi: 10.1016/0006-8993(87)90649-4
39. Simerly RB, Swanson LW, Gorski RA. The distribution of monoaminergic cells and fibers in a periventricular preoptic nucleus involved in the control of gonadotropin release: immunohistochemical evidence for a dopaminergic sexual dimorphism. Brain Res (1985) 330(1):55–64. doi: 10.1016/0006-8993(85)90007-1
40. Awasthi JR, Tamada K, Overton ETN, Takumi T. Comprehensive topographical map of the serotonergic fibers in the male mouse brain. J Comp Neurol (2021) 529(7):1391–429. doi: 10.1002/cne.25027
41. Kiss J, Halasz B. Demonstration of serotoninergic axons terminating on luteinizing hormone-releasing hormone neurons in the preoptic area of the rat using a combination of immunocytochemistry and high resolution autoradiography. Neuroscience (1985) 14(1):69–78. doi: 10.1016/0306-4522(85)90164-2
42. Campbell RE, Herbison AE. Definition of brainstem afferents to gonadotropin-releasing hormone neurons in the mouse using conditional viral tract tracing. Endocrinology (2007) 148(12):5884–90. doi: 10.1210/en.2007-0854
43. Johnson MD, Crowley WR. Role of central serotonin systems in the stimulatory effects of ovarian hormones and naloxone on luteinizing hormone release in female rats. Endocrinology (1986) 118(3):1180–6. doi: 10.1210/endo-118-3-1180
44. Hery M, Francois-Bellan AM, Hery F, Deprez P, Becquet D. Serotonin directly stimulates luteinizing hormone-releasing hormone release from gt1 cells via 5-ht7 receptors. Endocrine (1997) 7(2):261–5. doi: 10.1007/BF02778149
45. Wada K, Hu L, Mores N, Navarro CE, Fuda H, Krsmanovic LZ, et al. Serotonin (5-ht) receptor subtypes mediate specific modes of 5-ht-induced signaling and regulation of neurosecretion in gonadotropin-releasing hormone neurons. Mol Endocrinol (2006) 20(1):125–35. doi: 10.1210/me.2005-0109
46. Bhattarai JP, Roa J, Herbison AE, Han SK. Serotonin acts through 5-ht1 and 5-ht2 receptors to exert biphasic actions on gnrh neuron excitability in the mouse. Endocrinology (2014) 155(2):513–24. doi: 10.1210/en.2013-1692
47. Chen TW, Wardill TJ, Sun Y, Pulver SR, Renninger SL, Baohan A, et al. Ultrasensitive fluorescent proteins for imaging neuronal activity. Nature (2013) 499(7458):295–300. doi: 10.1038/nature12354
48. Cravo RM, Margatho LO, Osborne-Lawrence S, Donato J Jr., Atkin S, Bookout AL, et al. Characterization of kiss1 neurons using transgenic mouse models. Neuroscience (2011) 173:37–56. doi: 10.1016/j.neuroscience.2010.11.022
49. Madisen L, Garner AR, Shimaoka D, Chuong AS, Klapoetke NC, Li L, et al. Transgenic mice for intersectional targeting of neural sensors and effectors with high specificity and performance. Neuron (2015) 85(5):942–58. doi: 10.1016/j.neuron.2015.02.022
50. Cravo RM, Frazao R, Perello M, Osborne-Lawrence S, Williams KW, Zigman JM, et al. Leptin signaling in kiss1 neurons arises after pubertal development. PloS One (2013) 8(3):e58698. doi: 10.1371/journal.pone.0058698
51. Caligioni CS. Assessing reproductive status/stages in mice. Curr Protoc Neurosci (2009) Appendix 4:Appendix 4I. doi: 10.1002/0471142301.nsa04is48
52. Jamieson BB, Bouwer GT, Campbell RE, Piet R. Estrous cycle plasticity in the central clock output to kisspeptin neurons: implications for the preovulatory surge. Endocrinology (2021) 162(6):bqab071. doi: 10.1210/endocr/bqab071
53. Jamieson BB, Moore AM, Lohr DB, Thomas SX, Coolen LM, Lehman MN, et al. Prenatal androgen treatment impairs the suprachiasmatic nucleus arginine-vasopressin to kisspeptin neuron circuit in female mice. Front Endocrinol (Lausanne) (2022) 13:951344. doi: 10.3389/fendo.2022.951344
54. Edelstein A, Amodaj N, Hoover K, Vale R, Stuurman N. Computer control of microscopes using micromanager. Curr Protoc Mol Biol (2010) Chapter 14:Unit14 20. doi: 10.1002/0471142727.mb1420s92
55. Perkins KL. Cell-attached voltage-clamp and current-clamp recording and stimulation techniques in brain slices. J Neurosci Methods (2006) 154(1-2):1–18. doi: 10.1016/j.jneumeth.2006.02.010
56. Schindelin J, Arganda-Carreras I, Frise E, Kaynig V, Longair M, Pietzsch T, et al. Fiji: an open-source platform for biological-image analysis. Nat Methods (2012) 9(7):676–82. doi: 10.1038/nmeth.2019
57. Narahashi T, Moore JW, Scott WR. Tetrodotoxin blockage of sodium conductance increase in lobster giant axons. J Gen Physiol (1964) 47(5):965–74. doi: 10.1085/jgp.47.5.965
58. Narahashi T. Tetrodotoxin: A brief history. Proc Jpn Acad Ser B Phys Biol Sci (2008) 84(5):147–54. doi: 10.2183/pjab.84.147
59. Piet R, Boehm U, Herbison AE. Estrous cycle plasticity in the hyperpolarization-activated current ih is mediated by circulating 17beta-estradiol in preoptic area kisspeptin neurons. J Neurosci (2013) 33(26):10828–39. doi: 10.1523/JNEUROSCI.1021-13.2013
60. Stephens SBZ, Kauffman AS. Estrogen regulation of the molecular phenotype and active translatome of avpv kisspeptin neurons. Endocrinology (2021) 162(9):bqab080. doi: 10.1210/endocr/bqab080
61. Han SY, Clarkson J, Piet R, Herbison AE. Optical approaches for interrogating neural circuits controlling hormone secretion. Endocrinology (2018) 159(11):3822–33. doi: 10.1210/en.2018-00594
62. Gocz B, Rumpler E, Sarvari M, Skrapits K, Takacs S, Farkas I, et al. Transcriptome profiling of kisspeptin neurons from the mouse arcuate nucleus reveals new mechanisms in estrogenic control of fertility. Proc Natl Acad Sci U.S.A. (2022) 119(27):e2113749119. doi: 10.1073/pnas.2113749119
63. de Croft S, Piet R, Mayer C, Mai O, Boehm U, Herbison AE. Spontaneous kisspeptin neuron firing in the adult mouse reveals marked sex and brain region differences but no support for a direct role in negative feedback. Endocrinology (2012) 153(11):5384–93. doi: 10.1210/en.2012-1616
64. Yeo SH, Kyle V, Morris PG, Jackman S, Sinnett-Smith LC, Schacker M, et al. Visualisation of kiss1 neurone distribution using a kiss1-cre transgenic mouse. J Neuroendocrinol (2016) 28(11):10.1111/jne.12435. doi: 10.1111/jne.12435
65. Sanz E, Bean JC, Palmiter RD, Quintana A, McKnight GS. Activation of kiss1 neurons in the preoptic hypothalamus stimulates testosterone synthesis in adult male mice. bioRxiv (2020). doi: 10.1101/2020.12.03.410878
66. Dorner G, Gotz F, Rohde W. On the evocability of a positive oestrogen feedback action on lh secretion in female and male rats. Endokrinologie (1975) 66(3):369–72.
67. Clarkson J, Herbison AE. Postnatal development of kisspeptin neurons in mouse hypothalamus; sexual dimorphism and projections to gonadotropin-releasing hormone neurons. Endocrinology (2006) 147(12):5817–25. doi: 10.1210/en.2006-0787
68. Kauffman AS, Gottsch ML, Roa J, Byquist AC, Crown A, Clifton DK, et al. Sexual differentiation of kiss1 gene expression in the brain of the rat. Endocrinology (2007) 148(4):1774–83. doi: 10.1210/en.2006-1540
69. Poling MC, Luo EY, Kauffman AS. Sex differences in steroid receptor coexpression and circadian-timed activation of kisspeptin and rfrp-3 neurons may contribute to the sexually dimorphic basis of the lh surge. Endocrinology (2017) 158(10):3565–78. doi: 10.1210/en.2017-00405
70. Kueng W, Wirz-Justice A, Menzi R, Chappuis-Arndt E. Regional brain variations of tryptophan, monoamines, monoamine oxidase activity, plasma free and total tryptophan during the estrous cycle of the rat. Neuroendocrinology (1976) 21(4):289–96. doi: 10.1159/000122536
71. Cohen IR, Wise PM. Effects of estradiol on the diurnal rhythm of serotonin activity in microdissected brain areas of ovariectomized rats. Endocrinology (1988) 122(6):2619–25. doi: 10.1210/endo-122-6-2619
72. Kitts CS, Johnson JH. Reversal by estrogen of the effect of dorsal raphe stimulation on release of lh but not prolactin. J Neurosci Res (1986) 15(2):253–9. doi: 10.1002/jnr.490150214
73. Petersen SL, Hartman RD, Barraclough CA. An analysis of serotonin secretion in hypothalamic regions based on 5-hydroxytryptophan accumulation or push-pull perfusion. Effects of mesencephalic raphe or locus coeruleus stimulation and correlated changes in plasma luteinizing hormone. Brain Res (1989) 495(1):9–19. doi: 10.1016/0006-8993(89)91213-4
74. Helena CV, Toporikova N, Kalil B, Stathopoulos AM, Pogrebna VV, Carolino RO, et al. Kndy neurons modulate the magnitude of the steroid-induced luteinizing hormone surges in ovariectomized rats. Endocrinology (2015) 156(11):4200–13. doi: 10.1210/en.2015-1070
75. Mittelman-Smith MA, Krajewski-Hall SJ, McMullen NT, Rance NE. Ablation of kndy neurons results in hypogonadotropic hypogonadism and amplifies the steroid-induced lh surge in female rats. Endocrinology (2016) 157(5):2015–27. doi: 10.1210/en.2015-1740
76. Hu MH, Li XF, McCausland B, Li SY, Gresham R, Kinsey-Jones JS, et al. Relative importance of the arcuate and anteroventral periventricular kisspeptin neurons in control of puberty and reproductive function in female rats. Endocrinology (2015) 156(7):2619–31. doi: 10.1210/en.2014-1655
77. Lin XH, Lass G, Kong LS, Wang H, Li XF, Huang HF, et al. Optogenetic activation of arcuate kisspeptin neurons generates a luteinizing hormone surge-like secretion in an estradiol-dependent manner. Front Endocrinol (Lausanne) (2021) 12:775233. doi: 10.3389/fendo.2021.775233
78. Shen X, Liu Y, Li XF, Long H, Wang L, Lyu Q, et al. Optogenetic stimulation of kiss1(Arc) terminals in the avpv induces surge-like luteinizing hormone secretion via glutamate release in mice. Front Endocrinol (Lausanne) (2022) 13:1036235. doi: 10.3389/fendo.2022.1036235
79. Sullivan SD, Howard LC, Clayton AH, Moenter SM. Serotonergic activation rescues reproductive function in fasted mice: does serotonin mediate the metabolic effects of leptin on reproduction? Biol Reprod (2002) 66(6):1702–6. doi: 10.1095/biolreprod66.6.1702
80. Bhave VM, Nectow AR. The dorsal raphe nucleus in the control of energy balance. Trends Neurosci (2021) 44(12):946–60. doi: 10.1016/j.tins.2021.09.004
81. Morin LP, Allen CN. The circadian visual system, 2005. Brain Res Rev (2006) 51(1):1–60. doi: 10.1016/j.brainresrev.2005.08.003
82. Lowry CA. Functional subsets of serotonergic neurones: implications for control of the hypothalamic-pituitary-adrenal axis. J Neuroendocrinol (2002) 14(11):911–23. doi: 10.1046/j.1365-2826.2002.00861.x
Keywords: hypothalamus, GnRH neuronal network, preovulatory surge, GCaMP-based calcium imaging, action potential firing
Citation: Buo C, Bearss RJ, Novak AG, Anello AE, Dakin JJ and Piet R (2023) Serotonin stimulates female preoptic area kisspeptin neurons via activation of type 2 serotonin receptors in mice. Front. Endocrinol. 14:1212854. doi: 10.3389/fendo.2023.1212854
Received: 27 April 2023; Accepted: 19 September 2023;
Published: 12 October 2023.
Edited by:
Nandini Vasudevan, University of Reading, United KingdomReviewed by:
Susan Wray, National Institute of Neurological Disorders and Stroke (NIH), United StatesShunji Yamada, Kyoto Prefectural University of Medicine, Japan
Copyright © 2023 Buo, Bearss, Novak, Anello, Dakin and Piet. This is an open-access article distributed under the terms of the Creative Commons Attribution License (CC BY). The use, distribution or reproduction in other forums is permitted, provided the original author(s) and the copyright owner(s) are credited and that the original publication in this journal is cited, in accordance with accepted academic practice. No use, distribution or reproduction is permitted which does not comply with these terms.
*Correspondence: Richard Piet, cnBpZXRAa2VudC5lZHU=