- 1Laboratory of Neurochemistry and Molecular Neurobiology, Division of Molecular Biology, Ruđer Bošković Institute, Zagreb, Croatia
- 2Department of Obstetrics and Gynecology, Medical University of Graz, Graz, Austria
The biogenic monoamine serotonin (5-hydroxytryptamine, 5-HT) is a chemical messenger widely distributed in the brain and various other organs. Its homeostasis is maintained by the coordinated activity of a variety of proteins, including enzymes of serotonin metabolism, transmembrane transporters of serotonin, and serotonin receptors. The serotonin system has been identified also in the placenta in rodent models as a key component of placental physiology. However, serotonin pathways in the human placenta are far from well understood. Their alterations may have long-lasting consequences for the fetus that can manifest later in life. In this review, we summarize information on the location of the components of the serotonin system in the human placenta, their regulation, function, and alterations in pathological pregnancies. We highlight current controversies and discuss important topics for future research.
1. Introduction
The placenta is a temporary fetal organ that develops early in pregnancy. It acts as a barrier/facilitator for transport of gases, nutrients, and waste between maternal and fetal blood. In addition, the placenta produces and secretes a variety of hormones, cytokines, and growth factors that are essential for proper placental and fetal development and for facilitating maternal adaptation to and maintenance of pregnancy (1). Human placental development begins shortly after the blastocyst implants into the uterine wall, when cytotrophoblast cells begin to proliferate and fuse into a multinucleate syncytiotrophoblast. During the first trimester of pregnancy, as the cytotrophoblasts continue to invade the uterine wall, protrusions and fetal vessels form, eventually giving rise to chorionic villi. At the end of the first trimester, flow of fully oxygenated maternal blood is established enabling maternal blood to fill the lacunae formed within the syncytiotrophoblast (2). At the sites where the chorionic villi anchor into the maternal decidua (anchoring villi), a subpopulation of trophoblasts, the extravillous cytotrophoblasts, remodels the maternal spiral arteries by transiently partially replacing the endothelial cells lining the arteries and colonizing the inner layers of the myometrium (1, 3). Finally, the placental barrier formed consists of feto-placental endothelial cells lining the fetal capillaries, a mesenchymal core (Hofbauer cells, fibroblasts, and collagenous stroma), and cytotrophoblast cells overlaid by a continuous layer of syncytiotrophoblast (4). Although essential to the life of the fetus, the placenta is in many aspects still a rather mysterious organ.
Primary biogenic monoamines, such as dopamine, epinephrine, norepinephrine and serotonin, are widely used chemical messengers that act as neurotransmitters, hormones and autacoids. As low weight molecules with an amino group attached to an aromatic ring, all are synthesized from aromatic L-amino acids and inactivated by removal of the amino group. They play various regulatory roles in the nervous system and other organ systems. Serotonin not only regulates functions in the mature organism, but also acts as a significant growth factor during development and regulates various developmental processes, including nervous system development. Homeostasis of serotonin signaling during both development and adulthood is maintained by the coordinated activity of a variety of serotonin-regulating proteins, including its metabolic enzymes, membrane transporters and receptors. These proteins are widely distributed in the brain and various other organs, including the placenta.
Available evidence from clinical and animal studies suggests that placental serotonin system regulates placental development and functions and plays a role in proper embryo/fetal development. Its alterations may have long-lasting consequences for the fetus, which may manifest later in life like reverberations of the original effect. Despite the emerging evidence of the important role of serotonin for the feto-placental unit, its pathways in the human placenta are still far from being understood.
Here, we summarize the current data on the presence, regulation and function of serotonin system components in the human placenta, as well as on their putative role in normal and pathological pregnancy. We also highlight gaps and controversies in current knowledge and discuss directions for future research.
2. Serotonin system and its components in general
Serotonin (5-hydroxytryptamine, 5-HT), originally called enteramine, is a biogenic monoamine first isolated in 1937 from enterochromaffin cells of the intestine, and shown to induce intestinal contractions (5). In the 1940s, the same substance was identified as a “tone-modifier” present in serum and therefore named serotonin (6). Soon thereafter, its synthesis was discovered in the brain and more recently in several other peripheral sites (cf. below).
Today, serotonin is best known for its neurotransmitter role in the brain, but it acts also as a hormone and autocrine/paracrine messenger in various other organs (7). Many of its roles are highly conserved in a variety of animal species (8). In vertebrates, it modulates brain functions such as mood, emotion, cognition, sleep/wake rhythm, appetite, sexual behavior, pain perception, and response to stress (9). In addition, it regulates and fine tunes numerous other physiological processes, including hemostasis and vascular tone, gastrointestinal functions, immune response, reproductive functions, bone remodeling, and energy balance (7, 10, 11). Moreover, it contributes to regulating organ development and regeneration, by controlling basic cellular processes such as proliferation, differentiation, and migration (12, 13). In the developing fetal brain, it acts as a neurotrophic factor for various neuronal populations and also regulates the maturation of its own neurons (14, 15). Less well known are the antioxidant effects of serotonin in scavenging reactive oxygen species and inhibiting lipid peroxidation (16).
The effects of serotonin are primarily mediated by its interaction with plasma membrane receptors. In humans, 14 different serotonin receptor subtypes were identified and classified into 7 families (HTR1 to HTR7) based on structural, pharmacological, and signal transduction properties. With the exception of HTR3, a ligand-gated cation channel, all other subtypes are G protein-coupled receptors that activate various intracellular signaling pathways (17, 18). Serotonin receptors are widely distributed in the brain and various peripheral organs. During development, several serotonin receptor subtypes emerge in the developing brain prior to enzymes for serotonin synthesis, suggesting an extra-embryonic source of serotonin in the early stages of neurodevelopment (19).
Serotonin can also act in a receptor-independent manner by covalently binding to glutamine residues of various extracellular, cytoplasmic or nuclear proteins, in a process known as serotonylation. Serotonylation has been implicated in the regulation of platelet functions (20, 21), insulin secretion (22) and gene expression regulation (23).
Most of serotonin in the human body is produced and stored in the gastrointestinal tract. Some of the intestine-derived serotonin is secreted into the blood, where it is rapidly internalized in platelets and only a tiny fraction (< 1%) remains free in plasma (24). There are other niches of local serotonin synthesis in the human body, including serotonergic neurons, pinealocytes, pulmonary artery endothelial cells, mammary epithelial cells, mast cells, pancreatic beta cells, adipocytes, hepatocytes, osteoclasts, melanocytes, keratinocytes, and fibroblasts (25–27).
Serotonin biosynthesis uses essential amino acid L-tryptophan (L-trp) as precursor and involves two enzymatic steps (Figure 1). The first step, conversion of L-trp to 5-hydroxytryptophan (5-HTP), is catalyzed by rate-limiting tryptophan hydroxylase (TPH) and subsequent decarboxylation of 5-hydroxytryptophan to serotonin, is catalyzed by aromatic acid decarboxylase. There are two TPH isoforms, TPH1 and TPH2, encoded by genes located on human chromosome 11 and 12, respectively. The two TPH isoforms have different tissue expression – TPH1 (peripheral) is abundant in enterochromaffin cells of the intestine and in other peripheral tissues, whereas TPH2 (neuronal) is found mainly in serotonergic neurons of the brain (28).
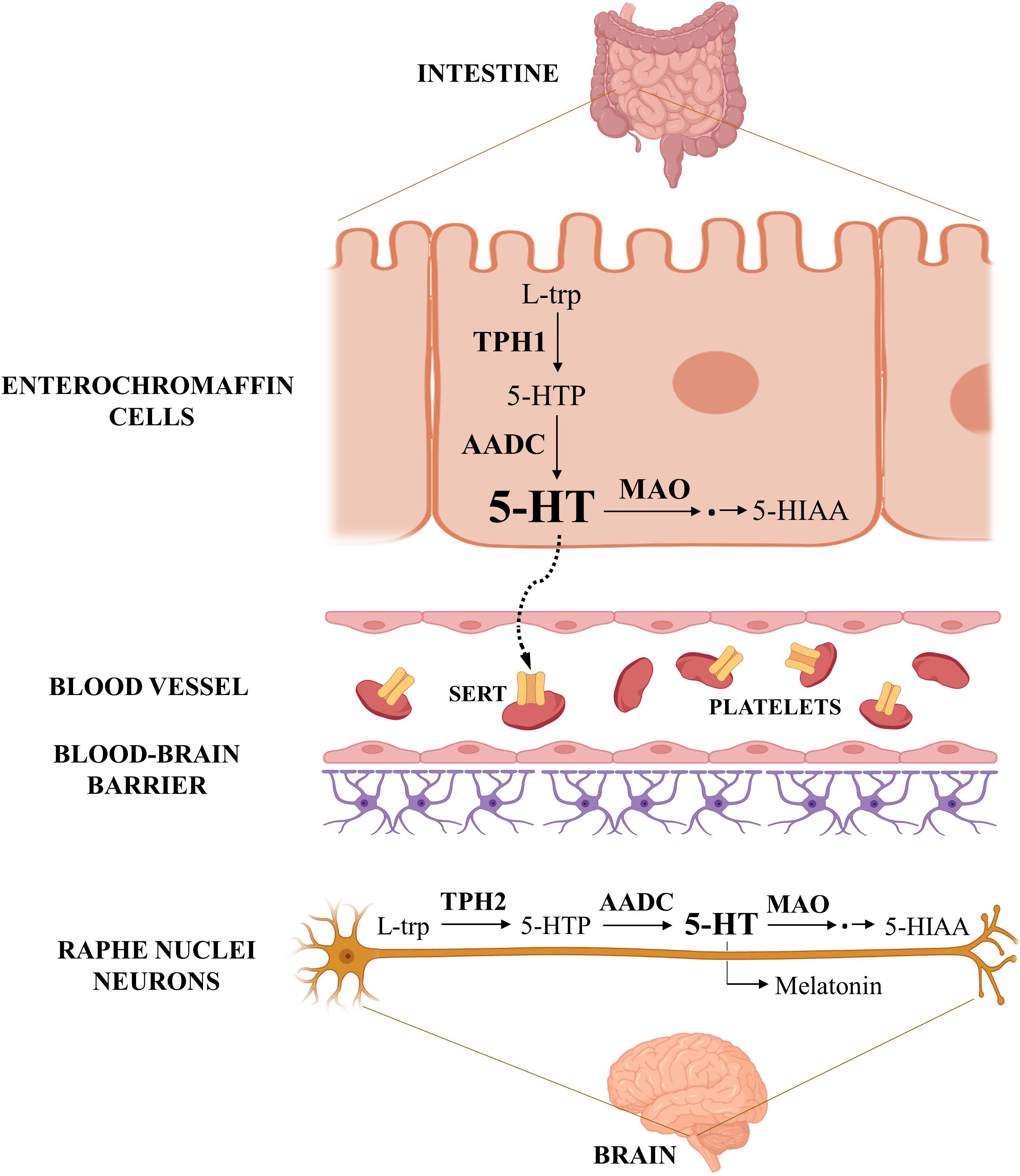
Figure 1 Serotonin metabolism. The figure shows the serotonin pathways in the gastrointestinal tract and in the central nervous system, the main sites of its synthesis in the human body. Serotonin synthesized in the enterochromaffin cells of the intestine is released into the portal circulation, taken up into platelets, and distributed to various other organs. Created with BioRender.com.
Serotonin is catabolized mainly to the final metabolite 5-hydroxyindoleacetic acid (5-HIAA), which can be excreted by the kidneys. The first step in this catabolic pathway, oxidative deamination, is catalyzed by the outer mitochondrial membrane enzyme monoamine oxidase (MAO). Its two isoforms, MAOA and MAOB, are encoded by distinct genes on the human X chromosome (29). Both isoforms catalyze oxidative deamination of serotonin and various other endogenous and dietary monoamines, with MAOA having preferential affinity for 5-HT over other substrates (30). Serotonin can also be converted into melatonin via two enzymatic steps.
As a hydrophilic substance, serotonin cannot freely pass through the phospholipid bilayer of the plasma membranes but is transported by specialized transmembrane proteins. These plasma membrane transporters mediate the uptake of extracellular serotonin into cells, which is the key mechanism responsible for terminating receptor-mediated serotonin signaling. There are two plasma membrane transport systems for serotonin, with fundamentally different kinetic properties: the high-affinity/low capacity (uptake-1) system and the low-affinity/high capacity (uptake-2) system. The uptake-1 system is represented by the serotonin transporter (SERT, also known as 5-HTT) (31), whereas the uptake-2 system includes the plasma membrane monoamine transporter (PMAT) and organic cation transporters (OCTs) 1, 2 and 3 (32). SERT is highly selective for serotonin while PMAT and OCTs can transport both serotonin and other monoamines.
Once inside the cell, another group of transmembrane transporters, the vesicular monoamine transporters (VMATs), mediate the transfer of cytosolic serotonin into secretory/storage organelles such as dense granules in platelets, synaptic vesicles in neurons, and secretory granules in enterochromaffin cells, pancreatic beta cells, mastocytes, and adipocytes (33–35). The storage of serotonin in intracellular organelles protects it from degradation by MAO and enables its release via exocytosis. There are two closely related VMATs, VMAT1 and VMAT2, with different pharmacological properties and tissue distribution (36).
Export of serotonin from cells storing the amine in secretory/storage organelles occurs via calcium-stimulated exocytosis (37–39). The export mechanism(s) from cells where it is not stored in intracellular vesicles are much less well understood. One possible efflux pathway could be via OCT2 (40) or OCT3 (41), which transport organic cations in both directions across the plasma membrane. It has been observed that SERT also reverses the direction of transport in the presence of some exogenous substrates (42), but it is unknown whether this phenomenon occurs under physiological conditions. Serotonin “leakage” via passive diffusion is generally considered insignificant because of its hydrophilic properties, but ability of serotonin to bind to lipid membranes (43) suggests that this may be more important than assumed. Nevertheless, a thorough investigation of more efficient and regulated, carrier-mediated efflux mechanisms for serotonin is warranted.
The activity of serotonin metabolizing enzymes, receptors, and transmembrane transporters is regulated by multiple mechanisms. In general, transcription of serotonin-related genes is modulated by genetic (44, 45) and epigenetic factors (45–47) and transcripts are further processed by prominent, tissue-specific alternative splicing and RNA editing (48, 49). In addition, the activity of serotonin-related proteins is controlled by post-translational modifications such as palmitoylation, phosphorylation, glycosylation, serotonylation, and disulfide bond formation, as well as by membrane trafficking, cell-surface localization and interactions with other proteins (50–55).
An interesting feature of the serotonin system, observed in both rodents and humans, is the presence of sex differences in some of its components resulting in functional differences between males and females. In humans, sex differences have been found in the concentration of serotonin and its metabolite 5-HIAA (56, 57) and in the rate of serotonin synthesis (58) in the central nervous system. The function/density of SERT and certain serotonin receptors in the human brain also differs between men and women (59, 60). In rodent models, pharmacological (61, 62) or genetic (63) manipulation of the serotonergic system causes sex-dependent behavioral and biochemical changes.
Sex differences in the serotonin system occur early in development. Studies in animal models have shown that the developing serotonergic system of males and females is differentially affected by various prenatal and perinatal factors including the microbiome (64), maternal overnutrition (65), and traumatic experiences such as parental separation (66). In humans, prenatal exposure to selective serotonin reuptake inhibitor (SSRI) antidepressants had sex-dependent effects on neonatal brain microstructure (67). Sexually dimorphic features of the human serotonergic system during the prenatal period were also evidenced by a positive correlation between placental and brain serotonin levels found only in male but not female human fetuses (65).
3. Serotonin system and its components in the human placenta
3.1. Localization of serotonin system components in the human placenta
The human placenta expresses many components of the serotonin system, including multiple serotonin receptor subtypes and several other proteins responsible for handling serotonin (Figure 2). The results of studies that examined the expression and/or localization of serotonin-related genes in human placenta are shown in Table 1 (studies in placental tissue homogenates), Table 2 (studies in placental tissue sections) and Table 3 (studies in isolated placental cells). Several studies have also compared the expression of serotonin-related genes in the human placenta between different stages of pregnancy (Table 4).
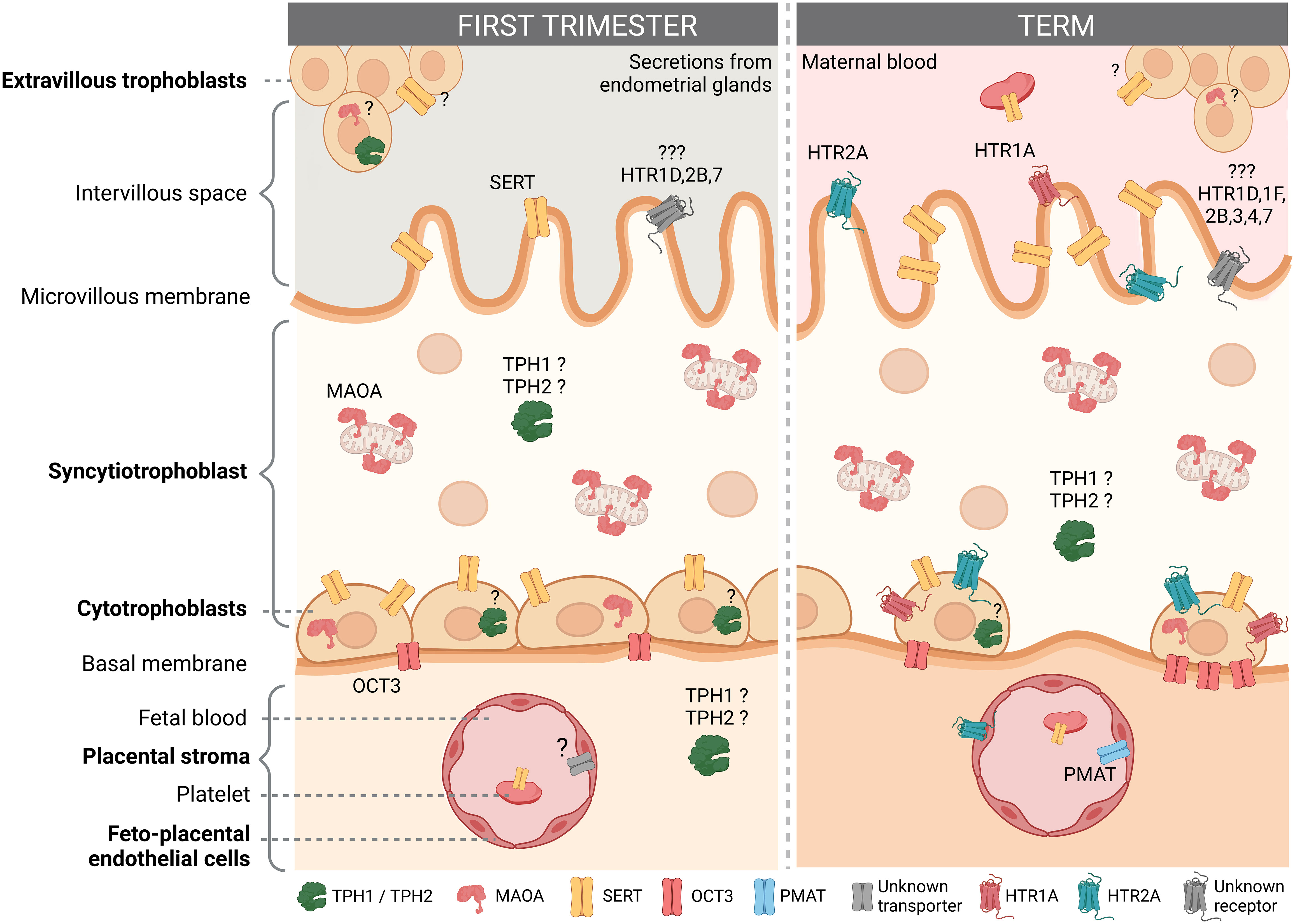
Figure 2 Localization of the serotonin system components in the human placenta according to gestational age. The figure shows the presence and localization of the components of the serotonin system in the human first trimester and term placenta according to the available literature. For details see main text. Components for which the results of different studies do not agree or which are reported only at the mRNA level are indicated with a question mark (?). Cell types not studied to date, such as mast cells, Hofbauer cells and other stromal cells are not shown. Created with BioRender.com.
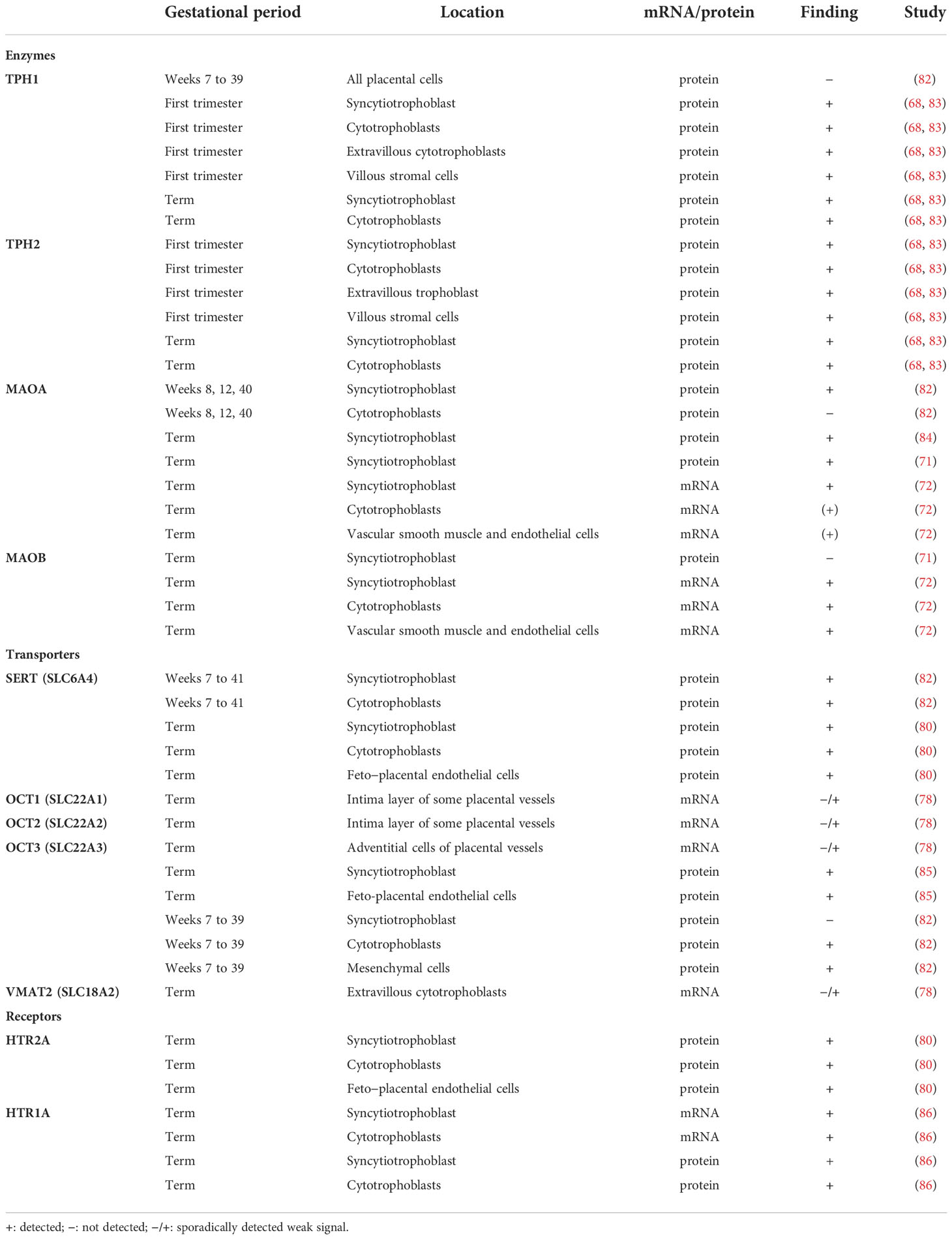
Table 2 Results on the localization of mRNAs and proteins of serotonin-related genes in human placenta obtained by in situ hybridization (ISH) and by immunohistochemical analysis (ISH), respectively.
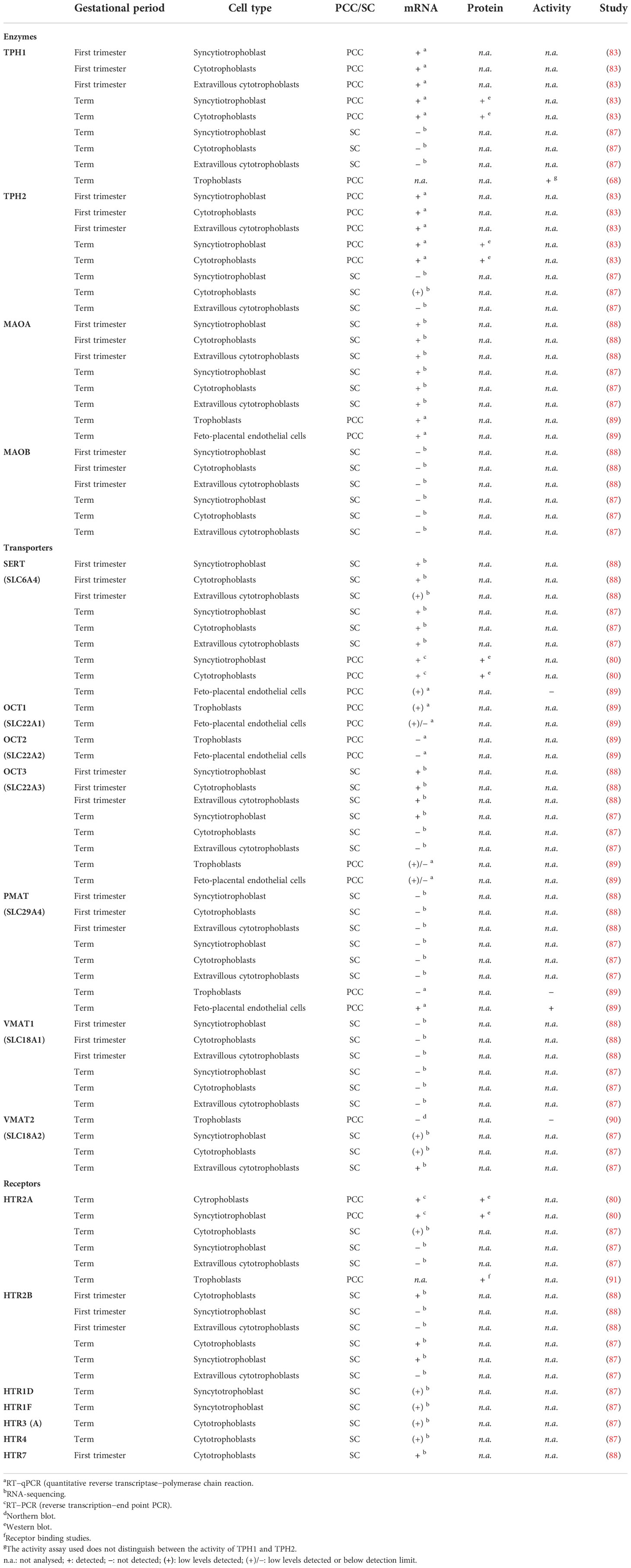
Table 3 Results on the presence of components of the serotonin system in human primary cell cultures (PCC) or single cells (SC).
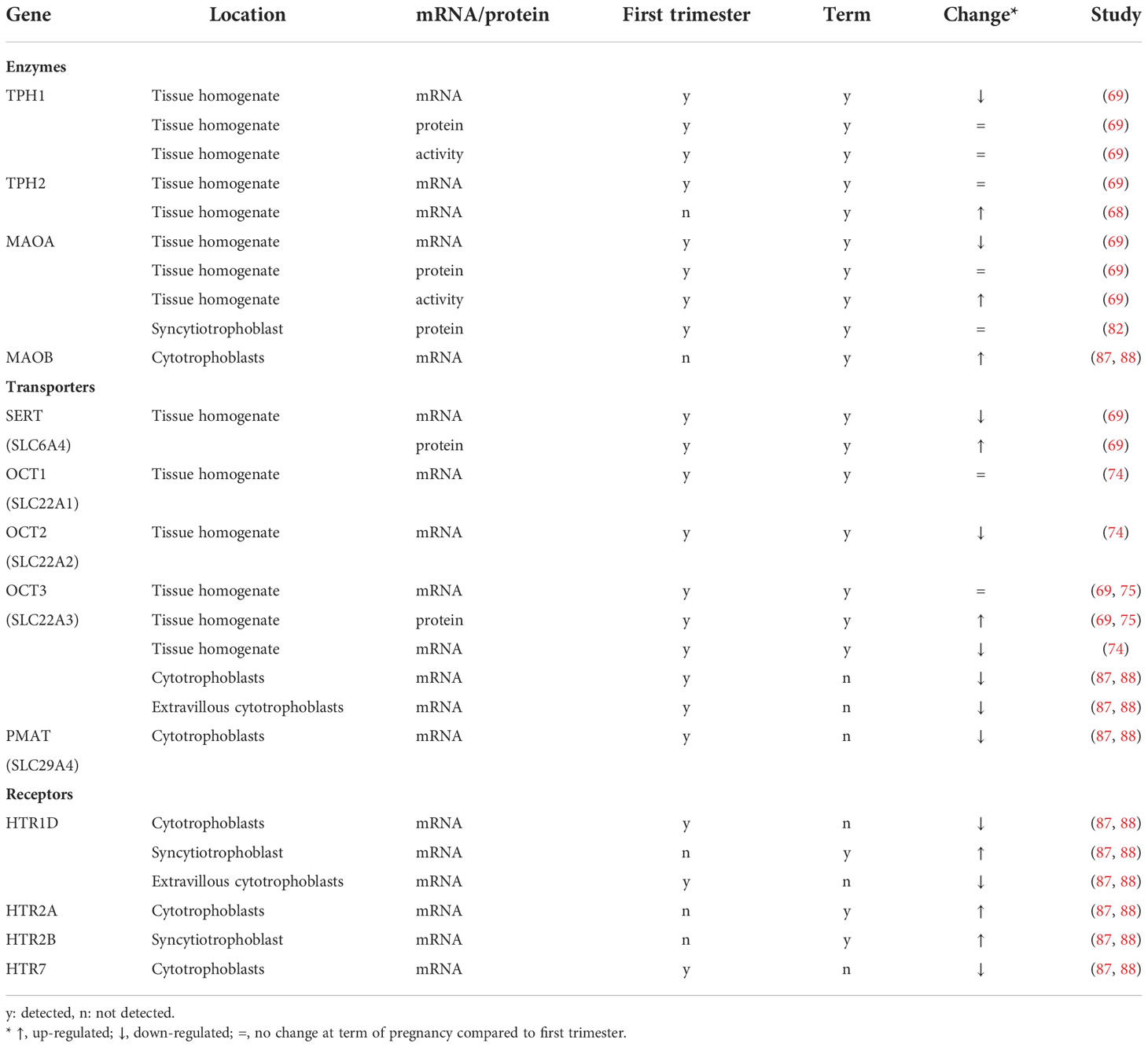
Table 4 Changes in the expression of serotonin components in human placenta in the third trimester compared with the first trimester of pregnancy.
3.1.1. Serotonin
Evidence on the presence of serotonin itself in the human placental cells is yet inconclusive. An early immunohistochemical (IHC) study of the human term placenta reported the presence of serotonin in syncytiotrophoblast, stromal cells, and capillary endothelium (86). However, recent IHC study of the human term placenta detected serotonin only in platelets in the chorionic villus vessels and maternal intervillous space, but not in untreated syncytiotrophoblast and cytotrophoblasts (82). Similarly, in the human first and second trimester placentas, platelets were strongly stained for serotonin, while only traces of serotonin were seen in untreated trophoblast cells (82). In the presence of exogenously added serotonin, both cytoplasmic and nuclear compartments of the cytotrophoblast stained for serotonin, but only nuclei in the syncytiotrophoblast stained for serotonin, whereas cytoplasmic serotonin staining in the syncytiotrophoblast was observed only after pharmacological blockade of serotonin catabolism (82). This suggests that serotonin levels in the syncytiotrophoblast are tightly controlled by rapid enzymatic degradation.
3.1.2. Serotonin-synthesizing enzymes
Studies also disagree about the presence of serotonin synthesis and expression of serotonin-synthesizing enzymes in the placenta. It has long been assumed that all serotonin affecting peripheral organ functions is synthesized in the intestine and distributed throughout the body by circulating platelets (Figure 1). This classical view of serotonin as a gut-derived hormone has been extended by the discovery of local sources of serotonin in various organs expressing TPH1, a peripheral isoform of the rate-limiting enzyme in serotonin synthesis (26). As for the placenta, an initial study demonstrated a lack of serotonin synthesis in the mouse placenta (92). However, Bonnin et al. (93) found evidence for serotonin synthesis in both mouse and human placenta. They demonstrated that levels of both serotonin and its immediate precursor (5-HTP) increase in homogenates of human first trimester placenta incubated with L-tryptophan (L-trp) and tetrahydrobiopterin (BH4, cofactor for TPH1 and TPH2 activity) (93). Conversion of L-trp to 5-HTP or serotonin in homogenates of human first trimester placenta has been replicated in independent studies and has also been demonstrated in homogenates of human term placenta (68, 69) and in cultured human term syncytiotrophoblast (83).
In addition, the presence of TPH1 and TPH2 mRNAs (encoding the peripheral and neuronal isoforms, respectively, of the rate-limiting enzyme of serotonin synthesis) and the presence of TPH1 and TPH2 proteins was demonstrated in primary syncytiotrophoblast and cytotrophoblasts isolated from human first trimester and term placentas (83). Studies in human placental homogenates reported that TPH1 mRNA was the predominant isoform throughout pregnancy (69) or the only one detected in the first trimester (68). In IHC analysis, TPH1 and TPH2 proteins were localized in syncytiotrophoblast, cytotrophoblasts, and some stromal cells of human first trimester and term placentas (68, 83).
In contrast to the above results, another IHC study failed to find TPH1 protein in human placentas from the first and second trimesters of pregnancy, while rare TPH1 signals were observed in human term placentas, differing from strong signals in human appendix (82). In addition, single-cell transcriptome analysis by RNA-sequencing (RNA-seq) did not detect TPH1 mRNAs in human term syncytiotrophoblast and cytotrophoblasts, whereas TPH2 mRNAs were detected only in term cytotrophoblasts, but at very low levels (87).
3.1.3. Serotonin-catabolizing enzymes
Serotonin catabolizing isoenzyme MAOA is abundant in the human placenta throughout pregnancy, as demonstrated by IHC (82) and enzyme activity studies (69). Known sites of MAOA expression in human placenta appear to be syncytiotrophoblast (29, 72, 82, 84), cytotrophoblasts (72, 87, 88) and feto-placental endothelial cells (89). Single cell transcriptomic data show that syncytiotrophoblast as compared with cytotrophoblasts contain much higher levels of MAOA mRNA (about 10-fold higher in the first trimester, and about 2-fold higher at term) (87, 88). mRNA encoding MAOB, MAO isoform with a lower affinity for serotonin, was detected at very low levels in term placenta (70, 87) and was absent in first trimester placenta (88).
3.1.4. Serotonin receptors
Ten serotonin receptor subtypes (HTR1A, HTR1D, HTR1E, HTR1F, HTR2A, HTR2B, HTR3 (subunit HTR3A), HTR4, HTR5A and HTR5B) have been reported to be expressed in the human term placenta (68, 79–81, 86, 87, 91). HTR2A has been localized in syncytiotrophoblast, cytotrophoblasts and fetal capillary endothelium of human term placentas (80). HTR2B, the most abundant subtype according to single cell transcriptomic analyses, is also expressed in both syncytiotrophoblast and cytotrophoblasts (81, 87). In contrast, HTR1D and HTR1F mRNAs were detected only in syncytiotrophoblast, whereas HTR3A and HTR4 mRNAs were found at low levels only in cytotrophoblasts (87) of human term placentas. So far, only HTR1D, HTR2B and HTR7 mRNAs have been detected in human first trimester placentas (specifically in cytotrophoblasts) (88).
However, it should be emphasized that the expression of most serotonin receptor subtypes in human placenta (with the exception of HTR2A (79, 80, 91) and HTR1A (86)) has been detected only at the mRNA level, so the presence and location of their proteins and their functions in human placenta require further investigation.
3.1.5. Vesicular transporters
Transcripts encoding VMAT2 were not detected in cultured human term trophoblasts by Northern blot analysis, and functional analysis demonstrated the absence of VMAT activity in the human trophoblast cell line JAR (90). Furthermore, in situ hybridization (ISH) did not detect VMAT2 mRNA in human villous trophoblasts, while only a weak signal was occasionally seen in extravillous trophoblasts within the uterine wall; very low levels were detected in placental homogenates by the more sensitive RT-PCR analysis (78), which may also have targeted platelet mRNA content. Single-cell transcriptome (RNA-seq) analyses reported that VMAT1 mRNA was not found in trophoblasts throughout pregnancy, while only very low levels of VMAT2 mRNA were detected in term trophoblasts (87, 88). Taken together, these results indicate that serotonin in trophoblasts is not stored in intracellular vesicles.
3.1.6. Plasma membrane transporters
Human placenta expresses several plasma membrane transporters for serotonin. Activity of the high-affinity SERT has been shown in plasma membrane vesicles (70, 94) and primary trophoblasts (89) isolated from human term placenta. SERT mRNA levels were lower in term compared to first trimester placentas, while the opposite was found for SERT protein levels (69). IHC analyses localized SERT protein to both syncytiotrophoblast and cytotrophoblasts (80, 82). Single-cell transcriptome analyses showed lower SERT mRNA levels in syncytiotrophoblast and cytotrophoblasts, both in the first trimester (88) and at term (87). On the other hand, recent study found that SERT mRNA levels were upregulated during spontaneous syncytialization of human primary trophoblasts, but SERT protein and activity levels were downregulated by syncytialization (95). Low levels of SERT mRNA and SERT protein were also detected in feto-placental endothelial cells (80, 82, 89), but functional analysis did not support significant SERT activity in these cells (89).
Low-affinity, polyspecific transporters OCT1 and OCT2 have been reported to be absent (77) or expressed at very low levels (74–76, 78, 89) in human first trimester and term placentas. This is consistent with the absence of their mRNAs in single cell transcriptome analyses (87, 88).
OCT3 is the most prominent member of OCT family and abundant in the human placenta throughout pregnancy (76, 77), with slightly lower mRNA and protein levels found in the first trimester than at term (69, 75). OCT3 protein and activity were detected in membrane vesicles isolated from the fetus-facing (basal) side, but not from the maternal-facing (microvillous) side of the human term placenta (70, 77). However, the exact cell type(s) harboring this transporter are not entirely clear. Thus, in one IHC study, prominent OCT3 staining was found on basolateral surface and in cytoplasm of cytotrophoblasts, while it was absent in syncytiotrophoblast in all three trimesters of pregnancy (82). This is consistent with OCT3 protein levels being down-regulated during spontaneous syncytialization of human term primary trophoblasts (95). However, in another study, OCT3 was localized to the basal (fetal-facing), but not the apical (maternal-facing) membrane of term syncytiotrophoblast (85). Weak OCT3 staining was occasionally observed in fetal capillaries (82, 85), but OCT3 mRNA was not detected in primary feto-placental endothelial cells (89). Single-cell transcriptome analysis by RNA-Seq demonstrated weak OCT3 mRNA signal in all types of first trimester trophoblasts (88), while at the end of pregnancy, OCT3 transcripts were detected only in syncytiotrophoblast (87).
Low levels of PMAT mRNA, encoding another low-affinity serotonin transporter, were detected in human term placental tissue (75). PMAT mRNA was absent in human first trimester (88) and term trophoblasts (87, 89), but was detected in human term feto-placental endothelial cells (89). In addition, efficient low-affinity serotonin uptake activity was detected in feto-placental endothelial cells, most likely mediated by PMAT (89).
3.2. Functions of serotonin system components in the human placenta
Serotonin is a potent vasoactive agent. Therefore, it was recognized early on that the placental serotonin system may contribute to the regulation of umbilico-placental blood flow. Serotonin induced a strong contractile effect on human umbilical and placental arteries and veins in tissue explants (96–98). Ketanserin, a known antagonist of the HTR2A receptor, significantly decreased the contractile response to serotonin in chorionic artery and vein segments from human placentas, suggesting that HTR2A is likely the receptor subtype mediating the vasoconstrictive effects of serotonin (98). At the cellular level, several studies have aimed to understanding the function and regulation of the serotonin system in the human placenta using cell culture models. Some of these studies have been performed on the human trophoblast-like cell lines such as BeWo, JEG-3 and JAR. These choriocarcinoma-derived cells are commonly used in vitro models in human placenta research (99). Recently, however, concerns have been raised about their suitability for studying serotonin pathways (70, 89, 95). Suitable in vitro models for future studies would be primary trophoblasts, placental explants, and organoid trophoblast cultures (100), as well as primary feto-placental endothelial cells, Hofbauer cells, and other cell types. Also, in vitro studies should preferably be conducted at physiological oxygen tension as TPH1 and SERT have been shown to be oxygen-regulated in pulmonary endothelial and smooth muscle cells, respectively (101, 102).
Thus, serotonin has been shown to stimulate proliferation of human placental trophoblast-like cell lines (BeWo, JEG-3) through activation of the HTR2A receptor and subsequent activation of a downstream signaling cascade involving both the ERK1/2 and STAT3 signaling pathways (79, 103, 104). Based on the known roles of the ERK1/2 and STAT3 signaling pathways, it has been proposed that serotonin regulates placental development and structure by controlling cell viability, differentiation, migration and invasion (103, 104). In vivo, the role of serotonin in regulating placental development is supported by a study in a knock-out mouse model showing that altered serotonin levels in placental intervillous space impair trophoblast survival and disrupt normal placental structure (105).
One more role assigned to the HTR2A receptor in the human placenta relates to the regulation of placental estrogen production. Specifically, studies in human primary trophoblasts (106) as well as trophoblast-like cell lines (BeWo, JEG-3) (107) have shown that serotonin induces the expression and activity of aromatase CYP19, a key enzyme in placental estrogen synthesis, via activation of the HTR2A receptor. The observed increase in aromatase activity was induced by HTR2A-mediated stimulation of the protein kinase C pathway (107) and possibly the JAK2/STAT3 pathway (104).
The expression of various serotonin receptor subtypes (cf. 3.1.4), coupled to different intracellular signaling pathways, suggests diverse and as yet unknown roles for serotonin in the human placenta. For example, some of the serotonin receptor subtypes expressed in the human term placenta (i.e., HTR1F, HTR3, and HTR4) have been shown to regulate mitochondrial function and homeostasis in different mouse organs (108, 109). It would be interesting to investigate whether serotonin plays a similar role in the human placenta during late pregnancy.
3.3. Regulation of serotonin system components in the human placenta
The mechanisms that regulate components of the serotonin system in the human placenta are largely unexplored, with studies to date focusing on SERT and MAOA.
Expression of the SERT is regulated by two distinct variable number tandem repeat polymorphisms in the promoter and intron 2 region (5HTTLPR and STin2, respectively), as well as by several single nucleotide polymorphisms (SNPs) in the promoter region, including rs25531 and rs25532 (110). In addition to genetic variants, epigenetic mechanisms play an important role in the regulation of SERT expression. SERT promoter contains a region enriched in CpG dinucleotides (CpG island), a sequence context in which cytosine is frequently methylated. Increased methylation of this region correlates with decreased SERT mRNA expression (111). We have found that SERT mRNA levels in the human term placenta are predominantly determined by SERT methylation in this region and not by SERT genetic variants (45). Studies in the trophoblast-like cell line JAR suggest that small noncoding RNAs, namely miR-15a and miR-16, also play an important role in the epigenetic regulation of SERT expression in the human placenta (46).
Expression and activity of the SERT in JAR cells is modulated by cytokines. Interleukin-1 upregulated SERT expression in JAR cells via cyclic adenosine monophosphate-independent signaling pathways (112), whereas interleukin-6 downregulated its expression and activity via a STAT3-dependent signaling pathway (113).
SERT activity in placental cells is regulated also by hormones such as insulin and estrogen. Insulin upregulates SERT activity in primary human trophoblast by enhancing dissociation of SERT protein from the endoplasmic reticulum chaperone ERp44, thereby enabling its maturation and translocation to the cell surface (55). Estrogen (17β-estradiol) decreased the activity of SERT in trophoblast-like BeWo cells, but increased the level of SERT protein and had no effect on the level of SERT mRNA (114). A discrepancy between the levels of SERT mRNA and SERT protein in human placenta was also observed in relation to the effects of gestational age (69) and trophoblast differentiation (95), indicating the presence of important regulatory mechanisms acting at the translational level.
The increasing use of SERT-targeting antidepressants (115–117) and psychostimulants (118) during pregnancy has led to investigations into the potential of these drugs to alter SERT activity in the placenta. We have shown that many common antidepressants at therapeutic plasma concentrations effectively inhibit the activity of SERT in primary trophoblasts isolated from human term placentas (89). This finding and studies in other placental models (82, 94, 119–121) highlight that antidepressant therapy in pregnancy may affect serotonin homeostasis in the placenta.
Expression of the MAOA gene is regulated by a variable number tandem repeat polymorphism located upstream of the MAOA coding region (MAOA-uVNTR), consisting of a 30-bp sequence present in 2, 3, 3.5, 4 or 5 copies (122). In trophoblast-like JAR cells, alleles with 3.5 and 4 repeats were transcribed more efficiently than those with 3 or 5 repeats (122), suggesting that alleles with 3.5 and 4 repeats correspond to the optimal length of this regulatory region. Consistent with this, the 4-repeat allele was associated with higher levels of MAOA mRNA in human term placenta than the 3-repeat allele (123). The MAOA promoter also contains two CpG islands (124), but their role in regulating MAOA transcription in the placenta has not yet been studied.
3.4. Changes of placental serotonin system components in pregnancy pathologies
It has long been known that serotonin signaling plays a role in the pathogenesis of pre-eclampsia (PE), a hypertensive pregnancy disorder characterized by multiple organ dysfunction. As suggested recently (125), dysregulation of serotonin signaling may underlie several features of PE, including excessive platelet aggregation, vascular hyporeactivity, and pro-inflammation. The placentas of pregnancies complicated with PE show decreased MAOA activity (71, 126), which may lead to decreased serotonin catabolism and contribute to the elevated circulating serotonin levels observed in women with PE. On the other hand, placental SERT activity is unchanged in PE (126). Decreased vascular reactivity of placental and umbilical vessels to serotonin has also been noted in PE (96, 98), possibly contributing to decreased umbilico-placental blood flow (127).
Changes in placental serotonin system have been found also in gestational diabetes mellitus (GDM), with most studies focusing on SERT. An initial study in a small cohort found decreased SERT mRNA and protein levels in placentas from GDM pregnancies (80). However, later studies with larger and better defined samples reported increased SERT mRNA levels in GDM placentas (45, 128). In contrast, primary trophoblasts isolated from GDM placentas showed decreased activity of SERT, which was attributed to decreased localization of the SERT protein to the plasma membrane (55). Interestingly, trophoblasts isolated from GDM placentas also showed an attenuated response to in vitro insulin treatment in terms of dissociation of SERT from the endoplasmic reticulum chaperone ERp44, suggesting that defects in insulin signaling are responsible for the impaired functional expression of SERT on the cell surface in GDM trophoblasts (55). In addition to increased expression of SERT, GDM placentas exhibited decreased methylation of the SERT promoter region (45). It may be speculated that epigenetic mechanisms increase SERT transcription to counteract the impact of defective insulin signaling on the activity of SERT in GDM placentas. It has also been reported that expression of serotonin receptor HTR2A is decreased in GDM placentas (80). Interestingly, DNA methylation of the placental HTR2A gene was associated with maternal overweight/obesity and GDM only in female, but not in male placentas (129).
Maternal mental health in pregnancy was also associated with alterations in placental serotonin homeostasis. As with PE, downregulation of MAOA expression in the placenta has been associated with increased symptoms of maternal depression during pregnancy (130). Maternal alcohol consumption during pregnancy was associated with decreased SERT mRNA levels and increased TPH1 mRNA levels in the placenta (131).
Recent evidence links alterations in the placental serotonin system to fetal growth restriction (FGR) (68) and preterm birth (132), serious pregnancy conditions accounting for significant perinatal morbidity and mortality. Thus, increased TPH activity and altered expression of SERT, TPH2, HTR1D, and HTR5A genes were found in third-trimester placentas from FGR pregnancies compared with gestational age-matched control pregnancies (68). In placentas from spontaneous preterm births, expression of both SERT and OCT3 transporters was upregulated compared with placentas from term births (132).
In summary, changes of serotonin system in the human placenta have been associated with several pregnancy-related medical conditions, including PE, GDM, maternal overweight/obesity, maternal mental health in pregnancy, FGR, and preterm birth. Whether the observed changes are causally involved in the pathogenesis of the above pathologies or are their consequence, or both, requires further investigation. Further studies are also needed to validate these findings in independent samples and to investigate the mechanisms underlying the observed changes.
4. Outlook - future perspectives
4.1. Does the human placenta provide serotonin to the fetus?
An important role attributed to the placental serotonin system in animal studies is to provide the embryo/fetus with an exogenous source of serotonin needed for proper brain and other organ development (93, 133, 134). However, studies disagree on the exact source of serotonin delivered to the fetus via the placenta, supporting either its maternal origin (133) or its synthesis in the placenta (93). In humans, it is generally believed that the placenta supplies maternal and/or placental serotonin to the developing fetal brain until the late first/early second trimester of pregnancy, when serotonergic neurons organize in the raphe nuclei and synthesis begins in situ (19, 135). This is supported by a recent discovery of a (male-specific) correlation between serotonin levels in the human fetal brain and the placenta (65).
As mentioned earlier (cf. 3.1.2), most studies to date support the ability of the human placenta to synthesize serotonin during both early and late pregnancy (68, 69, 83, 93). However, it should be noted that none of these studies examined the time and substrate concentration dependence of 5-HTP or serotonin production, or applied pharmacological approaches to demonstrate the involvement of TPH1/2 enzyme(s) (68, 69, 83, 93). In addition, the TPH activity assays used relatively high concentrations of L-trp (200 or 250 µM) compared with the Michaelis-Menten constant of human TPH for L-trp (7.5 µM) (136). Thus, convincing evidence for serotonin synthesis in the human placenta is still lacking. This is also supported by contrasting results obtained with a combination of immunohistochemical and pharmacological experiments on human placental tissue sections and placental explants (82). These argue that serotonin synthesis does not occur in the human placenta at any stage of pregnancy. Rather, the results suggest that serotonin released from maternal platelets into the intervillous space may be taken up into the syncytiotrophoblast via SERT and subsequently transferred to the fetal blood via a putative pathway involving several other proteins such as gap junction connexin-43 and OCT3 (82).
Placental perfusion is a method allowing studies into potential transfer of substances from the maternal to the fetal side of the placenta or in the opposite direction. The possible release of substances synthesized in the placenta into the maternal or fetal circulation can also be studied by this method. The only perfusion study performed to date on the human placenta suggests that serotonin is not transferred in appreciable amounts from the maternal to the fetal side at term pregnancy (137). This is consistent with results showing that serotonin taken up in the human term syncytiotrophoblast is rapidly catabolized by MAOA (70). Perfusion studies investigating the transfer of maternal/placental serotonin into fetal blood during early human pregnancy have not yet been performed.
In conclusion, current evidence does not support transplacental transfer of maternal serotonin into the fetal circulation at the end of human pregnancy. Further studies are needed to determine whether this might be different at earlier stages of pregnancy and to clarify whether the human placenta is capable of synthesizing serotonin under physiological conditions.
4.2. Epigenetic regulation of serotonin system components and epigenetic effects of serotonin
Epigenetic mechanisms are central to gene regulation and gene-environment interactions and are therefore of particular importance to the biology of the placenta as an organ that responds to changes in the intrauterine environment (138). The regulation of placental serotonin homeostasis by epigenetic mechanisms remains largely unexplored – so far, only two studies have addressed the role of DNA methylation (45) and noncoding RNAs (46) in the regulation of serotonin-related genes in the human placenta. Further studies on the role of DNA methylation, histone modifications, and noncoding RNAs and how they are modulated by various factors are warranted. In addition, further important studies should encompass the interaction of epigenetic mechanisms with functionally relevant genetic polymorphisms and post-translational regulatory mechanisms.
In addition to investigating the epigenetic regulation of genes involved in serotonin signaling, also the possible role of serotonin as a regulator of placental gene expression warrants studies. Recently, serotonylation of glutamine 5 on histone H3 (H3Q5ser) was identified as an epigenetic mechanism involved in the regulation of genes important for neuronal cell differentiation (23). The H3Q5ser modification was found in both neuronal and non-neuronal brain cells as well as in heart, colon, and blood samples. Its presence in the placenta has not been examined. However, findings on the presence of serotonin in the nuclei of various human placental cells (82) encourage future research on its presence and role in the human placenta.
4.3. Role of placental serotonin system in the developmental origins of health and disease
Animal studies show that changes in maternal and placental serotonin homeostasis not only impact the developing fetal brain but also have lasting neurochemical and neurobehavioral consequences (113, 114, 116, 117). In humans, changes in serotonin levels in maternal blood have been linked to an increased risk of neurodevelopmental disorders in the offspring, such as attention deficit/hyperactivity disorder (139) and autism (140). As for the human placenta, methylation of the placental HTR2A gene has been linked to key behavioral measures of neurodevelopment (quality of movement and attention) in human newborns (141), and placental expression of the SERT gene has been linked to regulatory behaviors in infants at two weeks of age (142). Furthermore, placental expression of the SERT and HTR2B genes has been associated with the incidence of febrile seizure in children (81), while placental expression of the MAOA gene has been identified as a biological mediator of the association between prenatal stress and child temperament at 12 months of age (143). Further longitudinal studies in human cohorts are needed to better understand the consequences of altered placental serotonin homeostasis in the developmental origins of health and disease. As mentioned earlier, changes in placental serotonin homeostasis have been associated with various pregnancy disorders (cf. 3.4). It is, therefore, of utmost importance to clarify whether they are innocent bystanders or play a role in the development of the disorder or in mediating the consequences for fetal health outcomes. This could easily open up new therapeutic opportunities, as there are already many approved drugs available that modulate the activity of various serotonin system components (144–146).
4.4. The importance of fetal sex
A well-established feature of the serotonin system in the brain of both humans and rodents is sexual dimorphism (cf. 2). Studies in rodents showing that serotonin uptake into placental membrane vesicles differs between female and male fetuses (70) suggest a sexual dimorphism also in placental handling of serotonin. The sexually dimorphic nature of the serotonin system in the human placenta is supported by the male-specific correlation of serotonin levels in placenta and fetal brain (65). In addition, methylation of the HTR2A gene in human placenta has been shown to be associated with maternal overweight/obesity only in female placentas (129), suggesting that fetal sex modulates the sensitivity of the placental serotonin system to the intrauterine environment. Furthermore, studies in animals and humans suggest an interaction between sex hormones and the serotonin system. For example, estrogens modulate the synthesis, uptake and catabolism of serotonin (147), while changes in serotonin signaling have been reported to affect estrogen production (106, 148). Overall, the influence of fetal sex needs to be considered in future studies of the function, regulation, and pathology-related changes of the serotonin system in the human placenta.
5. Final comments
Phylogenetically, serotonin is an ancient molecule. However, it has taken a long while until the components of the complex system regulating activity of serotonin have been identified in general. Only in the past decade, the serotonin system has received attention for its potential role in reproduction and development. Studies into serotonin’s involvement in the maternal-fetal interplay to ultimately contribute to fetal development through its actions on and within the placenta have been hampered for a variety of reasons. These include, but are not limited to, availability of proper experimental systems that fully capture the complexity of cellular interplay in the placenta, the difficulty in separating potential effects of maternal from those of fetal serotonin and by the temporal changes of placental cellular composition and function throughout pregnancy.
In this review we have not only summarized the current knowledge in this emerging field, but also provided suggestions for further studies. We hope that new molecular and cellular data can be integrated into a larger framework to better understand the role of serotonin system for placental and fetal development and its potential dysregulation in pathological pregnancy conditions.
Author contributions
JŠ and GD conceived and designed the article. MP, IB and JŠ wrote the first draft. MP and IB prepared the figures and tables. LČ-Š, GD, and JŠ critically revised the manuscript. All authors read and approved the final version.
Funding
This work was funded by Croatian Science Foundation grant IP-2018-01-6547 to JŠ. MP is supported by Croatian Science Foundation grant DOK-2018-09-7794.
Conflict of interest
The authors declare that the research was conducted in the absence of any commercial or financial relationships that could be construed as a potential conflict of interest.
Publisher’s note
All claims expressed in this article are solely those of the authors and do not necessarily represent those of their affiliated organizations, or those of the publisher, the editors and the reviewers. Any product that may be evaluated in this article, or claim that may be made by its manufacturer, is not guaranteed or endorsed by the publisher.
Abbreviations
5-HIAA, 5-hydroxyindoleacetic acid; 5-HT, 5-hydroxytryptamine; 5-HTP, 5-hydroxytryptophan; 5-HTR, 5-hydroxytryptamine receptor; BH4, tetrahydrobiopterin; FGR, fetal growth restriction; GDM, gestational diabetes mellitus; IHC, immunohistochemistry; L-trp, L-tryptophan; MAO A/B, monoamine oxidase A/B; PE, pre-eclampsia; OCT 1/2/3, organic cation transporter 1/2/3; PMAT, plasma membrane monoamine transporter; VMAT 1/2, vesicular monoamine transporter 1/2; SERT, serotonin transporter; TPH1/2, tryptophan hydroxylase 1/2.
References
1. Burton GJ, Fowden AL. The placenta: A multifaceted, transient organ. Philos Trans R Soc Lond B Biol Sci (2015) 370:20140066. doi: 10.1098/rstb.2014.0066
2. Jaremek A, Jeyarajah MJ, Jaju Bhattad G, Renaud SJ. Omics approaches to study formation and function of human placental syncytiotrophoblast. Front Cell Dev Biol (2021) 9:674162. doi: 10.3389/fcell.2021.674162
3. Bulmer JN, Innes BA, Robson SC, Lash GE. Transient loss of endothelial cells in human spiral artery remodelling during early pregnancy: Challenging the dogma. Placenta (2020) 101:230–3. doi: 10.1016/j.placenta.2020.10.003
4. Jones CJ, Fox H. Ultrastructure of the normal human placenta. Electron Microsc Rev (1991) 4:129–78. doi: 10.1016/0892-0354(91)90019-9
5. Vialli M, Erspamer V. Ricerche sul secreto delle cellule enterocromaffini. Z fur Zellforschung und Mikrosk Anat (1937) 27:81–99. doi: 10.1007/bf00391792
6. Rapport MM, ArdaAlden G, Page IH. Serum vasoconstrictor (Serotonin). J Biol Chem (1948) 176:1243–51. doi: 10.1016/s0021-9258(18)57137-4
7. Berger M, Gray JA, Roth BL. The expanded biology of serotonin. Annu Rev Med (2009) 60:355–66. doi: 10.1146/annurev.med.60.042307.110802
8. Azmitia EC. Evolution of serotonin: Sunlight to suicide. Amsterdam, Netherlands: Elsevier B.V (2010). doi: 10.1016/s1569-7339(10)70069-2
9. Jacobs BL, Azmitia EC. Structure and function of the brain serotonin system. Physiol Rev (1992) 72:165–229. doi: 10.1152/physrev.1992.72.1.165
10. Yabut JM, Crane JD, Green AE, Keating DJ, Khan WI, Steinberg GR. Emerging roles for serotonin in regulating metabolism: New implications for an ancient molecule. Endocr Rev (2019) 40:1092–107. doi: 10.1210/er.2018-00283
11. Lv J, Liu F. The role of serotonin beyond the central nervous system during embryogenesis. frontiers in cellular neuroscience (2017) (Accessed September 12, 2022).
12. Whitaker-Azmitia PM, Druse M, Walker P, Lauder JM. Serotonin as a developmental signal. Behav Brain Res (1996) 73:19–29. doi: 10.1016/0166-4328(96)00071-x
13. Papadimas GK, Tzirogiannis KN, Mykoniatis MG, Grypioti AD, Manta GA, Panoutsopoulos GI. The emerging role of serotonin in liver regeneration. Swiss Med Weekly (2012) 142:1–6. doi: 10.4414/smw.2012.13548
14. Gaspar P, Cases O, Maroteaux L. The developmental role of serotonin: News from mouse molecular genetics. Nat Rev Neurosci (2003) 4:1002–12. doi: 10.1038/nrn1256
15. Sodhi MSK, Sanders-Bush E. Serotonin and brain development. Int Rev Neurobiol (2004) 59:111–74. doi: 10.1016/S0074-7742(04)59006-2
16. Azouzi S, Santuz H, Morandat S, Pereira C, Côté F, Hermine O, et al. Antioxidant and membrane binding properties of serotonin protect lipids from oxidation. Biophys J (2016) 112:1863–73. doi: 10.1016/j.bpj.2017.03.037
17. Sharp T, Barnes NM. Central 5-HT receptors and their function; present and future. Neuropharmacology (2020) 177:108155. doi: 10.1016/j.neuropharm.2020.108155
18. Celli J, Rappold G, Niesler B. The human serotonin type 3 receptor gene (HTR3A-e) allelic variant database. Hum Mutat (2017) 38:137–47. doi: 10.1002/humu.23136
19. Bonnin A, Levitt P. Fetal, maternal and placental sources of serotonin and new implications for developmental programming of the brain. Neuroscience (2011) 197:1–7. doi: 10.1016/j.neuroscience.2011.10.005
20. Dale GL. Coated-platelets: An emerging component of the procoagulant response. J Thromb Haemost (2005) 3:2185–92. doi: 10.1111/j.1538-7836.2005.01274.x
21. Walther DJ, Peter J-U, Winter S, Höltje M, Paulmann N, Grohmann M, et al. Serotonylation of small GTPases is a signal transduction pathway that triggers platelet alpha-granule release. Cell (2003) 115:851–62. doi: 10.1016/s0092-8674(03)01014-6
22. Paulmann N, Grohmann M, Voigt JP, Bert B, Vowinckel J, Bader M, et al. Intracellular serotonin modulates insulin secretion from pancreatic β-cells by protein serotonylation. PloS Biol (2009) 7:1–10. doi: 10.1371/journal.pbio.1000229
23. Farrelly LA, Thompson RE, Zhao S, Lepack AE, Lyu Y, Bhanu NV, et al. Histone serotonylation is a permissive modification that enhances TFIID binding to H3K4me3. Nature (2019) 567:535–9. doi: 10.1038/s41586-019-1024-7
24. Lechin F, van der Dijs B, Orozco B, Lechin M, Lechin AE. Increased levels of free serotonin in plasma of symptomatic asthmatic patients. Ann Allergy Asthma Immunol (1996) 77:245–53. doi: 10.1016/S1081-1206(10)63263-2
25. Slominski AT, Zmijewski MA, Cezary S, Zbytek B, Slominski RM, Steketee JD. Sensing the environment: Regulation of local and globalhomeostasis by the skin neuroendocrine system. Adv Anat Embryol Cell Biol (2012) 212:1–98. doi: 10.1007/978-3-642-19683-6_1
26. Spohn SN, Mawe GM. Non-conventional features of peripheral serotonin signalling-the gut and beyond. Nat Rev Gastroenterol Hepatol (2017) 14:412–20. doi: 10.1038/nrgastro.2017.51
27. Yabut JM, Desjardins EM, Chan EJ, Day EA, Leroux JM, Wang B, et al. Genetic deletion of mast cell serotonin synthesis prevents the development of obesity and insulin resistance. Nat Commun (2020) 11:463. doi: 10.1038/s41467-019-14080-7
28. Walther DJ, Peter J-U, Bashammakh S, Hörtnagl H, Voits M, Fink H, et al. Synthesis of serotonin by a second tryptophan hydroxylase isoform. Science (2003) 299:76. doi: 10.1126/science.1078197
29. Sivasubramaniam SD, Finch CC, Rodriguez MJ, Mahy N, Billett EE. A comparative study of the expression of monoamine oxidase-a and -b mRNA and protein in non-CNS human tissues. Cell Tissue Res (2003) 313:291–300. doi: 10.1007/s00441-003-0765-6
30. Ramsay RR, Albreht A. Kinetics, mechanism, and inhibition of monoamine oxidase. J Neural Transm (2018) 125:1659–83. doi: 10.1007/s00702-018-1861-9
31. Ramamoorthy S, Giovanetti E, Qian Y, Blakely RD. Phosphorylation and regulation of antidepressant-sensitive serotonin transporters. J Biol Chem (1998) 273:2458–66. doi: 10.1074/jbc.273.4.2458
32. Duan H, Wang J. Selective transport of monoamine neurotransmitters by human plasma membrane monoamine transporter and organic cation transporter 3. J Pharmacol Exp Ther (2010) 335:743–53. doi: 10.1124/jpet.110.170142
33. Ahmad MF, Ferland D, Ayala-Lopez N, Contreras GA, Darios E, Thompson J, et al. Perivascular adipocytes store norepinephrine by vesicular transport. Arterioscler Thromb Vasc Biol (2019) 39:188–99. doi: 10.1161/ATVBAHA.118.311720
34. Almaça J, Molina J, Menegaz D, Pronin AN, Tamayo A, Slepak V, et al. Human beta cells produce and release serotonin to inhibit glucagon secretion from alpha cells. Cell Rep (2016) 17:3281–91. doi: 10.1016/j.celrep.2016.11.072
35. Takeuchi T, Harada Y, Moriyama S, Furuta K, Tanaka S, Miyaji T, et al. Vesicular polyamine transporter mediates vesicular storage and release of polyamine from mast cells. J Biol Chem (2017) 292:3909–18. doi: 10.1074/jbc.M116.756197
36. Wimalasena K. Vesicular monoamine transporters: Structure-function, pharmacology and meidcinal chemistry. Med Res Rev (2011) 31:483–519. doi: 10.1002/med.20187
37. De-Miguel FF, Trueta C. Synaptic and extrasynaptic secretion of serotonin. Cell Mol Neurobiol (2005) 25:297–312. doi: 10.1007/s10571-005-3061-z
38. Leon-Pinzon C, Cercós MG, Noguez P, Trueta C, De-Miguel FF. Exocytosis of serotonin from the neuronal soma is sustained by a serotonin and calcium-dependent feedback loop. Front Cell Neurosci (2014) 8:169. doi: 10.3389/fncel.2014.00169
39. Reed GL, Fitzgerald ML, Polgár J. Molecular mechanisms of platelet exocytosis: insights into the “secrete” life of thrombocytes. Blood (2000) 96:3334–42. doi: 10.1182/blood.V96.10.3334
40. Busch AE, Karbach U, Miska D, Gorboulev V, Akhoundova A, Volk C, et al. Human neurons express the polyspecific cation transporter hOCT2, which translocates monoamine neurotransmitters, amantadine, and memantine. Mol Pharmacol (1998) 54:342–52. doi: 10.1124/mol.54.2.342
41. Kekuda R, Prasad PD, Wu X, Wang H, Fei YJ, Leibach FH, et al. Cloning and functional characterization of a potential-sensitive, polyspecific organic cation transporter (OCT3) most abundantly expressed in placenta. J Biol Chem (1998) 273:15971–9. doi: 10.1074/jbc.273.26.15971
42. Hilber B, Scholze P, Dorostkar MM, Sandtner W, Holy M, Boehm S, et al. Serotonin-transporter mediated efflux: A pharmacological analysis of amphetamines and non-amphetamines. Neuropharmacology (2005) 49:811–9. doi: 10.1016/j.neuropharm.2005.08.008
43. Peters GH, Wang C, Cruys-Bagger N, Velardez GF, Madsen JJ, Westh P. Binding of serotonin to lipid membranes. J Am Chem Soc (2013) 135:2164–71. doi: 10.1021/ja306681d
44. Manca M, Pessoa V, Lopez AI, Harrison PT, Miyajima F, Sharp H, et al. The regulation of monoamine oxidase a gene expression by distinct variable number tandem repeats. J Mol Neurosci (2018) 64:459–70. doi: 10.1007/s12031-018-1044-z
45. Blazevic S, Horvaticek M, Kesic M, Zill P, Hranilovic D, Ivanisevic M, et al. Epigenetic adaptation of the placental serotonin transporter gene (SLC6A4) to gestational diabetes mellitus. PloS One (2017) 12:e0179934. doi: 10.1371/journal.pone.0179934
46. Moya PR, Wendland JR, Salemme J, Fried RL, Murphy DL. miR-15a and miR-16 regulate serotonin transporter expression in human placental and rat brain raphe cells. Int J Neuropsychopharmacol (2013) 16:621–9. doi: 10.1017/S1461145712000454
47. Ruble CL, Smith RM, Calley J, Munsie L, Airey DC, Gao Y, et al. Genomic structure and expression of the human serotonin 2A receptor gene (HTR2A) locus: Identification of novel HTR2A and antisense (HTR2A-AS1) exons. BMC Genet (2016) 17:1–15. doi: 10.1186/s12863-015-0325-6
48. Grohmann M, Hammer P, Walther M, Paulmann N, Büttner A, Eisenmenger W, et al. Alternative splicing and extensive RNA editing of human TPH2 transcripts. PloS One (2010) 5:e8956. doi: 10.1371/journal.pone.0008956
49. Ozsarac N, Santha E, Hoffman BJ. Alternative non-coding exons support serotonin transporter mRNA expression in the brain and gut. J Neurochem (2002) 82:336–44. doi: 10.1046/j.1471-4159.2002.00964.x
50. Woehler A, Wlodarczyk J, Ponimaskin EG. Specific oligomerization of the 5-HT1A receptor in the plasma membrane. Glycoconj J (2009) 26:749–56. doi: 10.1007/s10719-008-9187-8
51. Tate CG, Blakely RD. The effect of n-linked glycosylation on activity of the na+- and cl– dependent serotonin transporter expressed using recombinant baculovirus in insect cells. J Biol Chem (1994) 269:26303–10. doi: 10.1016/s0021-9258(18)47194-3
52. Xia Z, Gray JA, Compton-Toth BA, Roth BL. A direct interaction of PSD-95 with 5-HT2A serotonin receptors regulates receptor trafficking and signal transduction. J Biol Chem (2003) 278:21901–8. doi: 10.1074/jbc.M301905200
53. Gorinski N, Bijata M, Prasad S, Wirth A, Abdel Galil D, Zeug A, et al. Attenuated palmitoylation of serotonin receptor 5-HT1A affects receptor function and contributes to depression-like behaviors. Nat Commun (2019) 10:3924. doi: 10.1038/s41467-019-11876-5
54. Cooper A, Woulfe D, Kilic F. Post-translational modifications of serotonin transporter. Pharmacol Res (2019) 140:7–13. doi: 10.1016/j.phrs.2018.10.018
55. Li Y, Hadden C, Singh P, Mercado CP, Murphy P, Dajani NK, et al. GDM-associated insulin deficiency hinders the dissociation of SERT from ERp44 and down-regulates placental 5-HT uptake. Proc Natl Acad Sci United States America (2014) 111:E5697–705. doi: 10.1073/pnas.1416675112
56. Gottfries CG, Roos BE, Winblad B. Determination of 5-hydroxytryptamine, 5-hydroxyindoleacetic acid and homovanillic acid in brain tissue from an autopsy material. Acta Psychiatr Scand (1974) 50:496–507. doi: 10.1111/j.1600-0447.1974.tb09711.x
57. Young SN, Gauthier S, Anderson GM, Purdy WC. Tryptophan, 5-hydroxyindoleacetic acid and indoleacetic acid in human cerebrospinal fluid: Interrelationships and the influence of age, sex, epilepsy and anticonvulsant drugs. J Neurol Neurosurg Psychiatry (1980) 43:438–45. doi: 10.1136/jnnp.43.5.438
58. Nishizawa S, Benkelfat C, Young SN, Leyton M, Mzengeza S, De Montigny C, et al. Differences between males and females in rates of serotonin synthesis in human brain. Proc Natl Acad Sci United States America (1997) 94:5308–13. doi: 10.1073/pnas.94.10.5308
59. Biver F, Lotstra F, Monclus M, Wiklera D, Damhaut P, Mendlewicz J, et al. Sex difference in 5HT 2 receptor in the living human brain. Neurosci Lett (1996) 204:25–8. doi: 10.1016/0304-3940(96)12307-7
60. Jovanovic H, Lundberg J, Karlsson P, Cerin Å, Saijo T, Varrone A, et al. Sex differences in the serotonin 1A receptor and serotonin transporter binding in the human brain measured by PET. NeuroImage (2008) 39:1408–19. doi: 10.1016/j.neuroimage.2007.10.016
61. Carlsson M, Svensson K, Eriksson E, Carlsson A. Rat brain serotonin: Biochemical and functional evidence for a sex difference. J Neural Transm (1985) 63:297–313. doi: 10.1007/BF01252033
62. Dickinson SL, Curzon G. 5-hydroxytryptamine-mediated behaviour in male and female rats. Neuropharmacology (1986) 25:771–6. doi: 10.1016/0028-3908(86)90094-8
63. Jones MD, Lucki I. Sex differences in the regulation of serotonergic transmission and behavior in 5-HT receptor knockout mice. Neuropsychopharmacology (2005) 30:1039–47. doi: 10.1038/sj.npp.1300664
64. Clarke G, Grenham S, Scully P, Fitzgerald P, Moloney RD, Shanahan F, et al. The microbiome-gut-brain axis during early life regulates the hippocampal serotonergic system in a sex-dependent manner. Mol Psychiatry (2013) 18:666–73. doi: 10.1038/mp.2012.77
65. Ceasrine AM, Devlin BA, Bolton JL, Jo YC, Patrick B, Washington K, et al. Maternal diet disrupts the placenta-brain axis in a sex-specific manner. bioRxiv (2022) doi: 10.1101/2021.11.12.468408
66. Llorente R, O’Shea E, Gutierrez-Lopez MD, Llorente-Berzal A, Colado MI, Viveros M-P. Sex-dependent maternal deprivation effects on brain monoamine content in adolescent rats. Neurosci Lett (2010) 479:112–7. doi: 10.1016/j.neulet.2010.05.039
67. Campbell KSJ, Williams LJ, Bjornson BH, Weik E, Brain U, Grunau RE, et al. Prenatal antidepressant exposure and sex differences in neonatal corpus callosum microstructure. Dev Psychobiol (2021) 63:1–15. doi: 10.1002/dev.22125
68. Ranzil S, Ellery S, Walker DW, Vaillancourt C, Alfaidy N, Bonnin A, et al. Disrupted placental serotonin synthetic pathway and increased placental serotonin: Potential implications in the pathogenesis of human fetal growth restriction. Placenta (2019) 84:74–83. doi: 10.1016/j.placenta.2019.05.012
69. Karahoda R, Abad C, Horackova H, Kastner P, Zaugg J, Cerveny L, et al. Dynamics of tryptophan metabolic pathways in human placenta and placental-derived cells: Effect of gestation age and trophoblast differentiation. Front Cell Dev Biol (2020) 8:574034. doi: 10.3389/fcell.2020.574034
70. Karahoda R, Horackova H, Kastner P, Matthios A, Cerveny L, Kucera R, et al. Serotonin homeostasis in the materno-foetal interface at term: Role of transporters (SERT/SLC6A4 and OCT3/SLC22A3) and monoamine oxidase a (MAO-a) in uptake and degradation of serotonin by human and rat term placenta. Acta Physiol (2020) 229:e13478. doi: 10.1111/apha.13478
71. Sivasubramaniam SD, Finch CC, Billett MA, Baker PN, Billett EE. Monoamine oxidase expression and activity in human placentae from pre-eclamptic and normotensive pregnancies. Placenta (2002) 23:163–71. doi: 10.1053/plac.2001.0770
72. Auda GR, Kirk SH, Billett MA, Billett EE. Localization of monoamine oxidase mRNA in human placenta. J Histochem Cytochem (1998) 46:1393–400. doi: 10.1177/002215549804601208
73. O’Carroll AM, Anderson MC, Tobbia I, Phillips JP, Tipton KF. Determination of the absolute concentrations of monoamine oxidase a and b in human tissues. Biochem Pharmacol (1989) 38:901–5. doi: 10.1016/0006-2952(89)90278-5
74. Ahmadimoghaddam D, Zemankova L, Nachtigal P, Dolezelova E, Neumanova Z, Cerveny L, et al. Organic cation transporter 3 (OCT3/SLC22A3) and multidrug and toxin extrusion 1 (MATE1/SLC47A1) transporter in the placenta and fetal tissues: expression profile and fetus protective role at different stages of gestation. Biol Reprod (2013) 88:55. doi: 10.1095/biolreprod.112.105064
75. Lee N, Hebert MF, Prasad B, Easterling TR, Kelly EJ, Unadkat JD, et al. Effect of gestational age on mRNA and protein expression of polyspecific organic cation transporters during pregnancy. Drug Metab Dispos (2013) 41:2225–32. doi: 10.1124/dmd.113.054072
76. Hasegawa N, Furugen A, Ono K, Koishikawa M, Miyazawa Y, Nishimura A, et al. Cellular uptake properties of lamotrigine in human placental cell lines: Investigation of involvement of organic cation transporters (SLC22A1–5). Drug Metab Pharmacokinet (2020) 35:266–73. doi: 10.1016/j.dmpk.2020.01.005
77. Sata R, Ohtani H, Tsujimoto M, Murakami H, Koyabu N, Nakamura T, et al. Functional analysis of organic cation transporter 3 expressed in human placenta. J Pharmacol Exp Ther (2005) 315:888–95. doi: 10.1124/jpet.105.086827
78. Bottalico B, Larsson I, Brodszki J, Hernandez-Andrade E, Casslén B, Marsál K, et al. Norepinephrine transporter (NET), serotonin transporter (SERT), vesicular monoamine transporter (VMAT2) and organic cation transporters (OCT1, 2 and EMT) in human placenta from pre-eclamptic and normotensive pregnancies. Placenta (2004) 25:518–29. doi: 10.1016/j.placenta.2003.10.017
79. Sonier B, Lavigne C, Arseneault M, Ouellette R, Vaillancourt C. Expression of the 5-HT2A serotoninergic receptor in human placenta and choriocarcinoma cells: mitogenic implications of serotonin. Placenta (2005) 26:484–90. doi: 10.1016/j.placenta.2004.08.003
80. Viau M, Lafond J, Vaillancourt C. Expression of placental serotonin transporter and 5-HT 2A receptor in normal and gestational diabetes mellitus pregnancies. Reprod BioMed Online (2009) 19:207–15. doi: 10.1016/s1472-6483(10)60074-0
81. Thébault-Dagher F, Robles M, Herba CM, St-Pierre J, Brochen C, Muckle G, et al. Febrile seizure incidence and age at first occurrence are associated with changes in placental normalized gene expression: the “3D” pregnancy cohort study. J Neuroendocrinol (2021) 33:e13046. doi: 10.1111/jne.13046
82. Kliman HJ, Quaratella SB, Setaro AC, Siegman EC, Subha ZT, Tal R, et al. Pathway of maternal serotonin to the human embryo and fetus. Endocrinology (2018) 159:1609–29. doi: 10.1210/en.2017-03025
83. Laurent L, Deroy K, St-Pierre J, Côté F, Sanderson JT, Vaillancourt C. Human placenta expresses both peripheral and neuronal isoform of tryptophan hydroxylase. Biochimie (2017) 140:159–65. doi: 10.1016/j.biochi.2017.07.008
84. Gujrati VR, Shanker K, Vrat S, Chandravati, Parmar SS. Novel appearance of placental nuclear monoamine oxidase: Biochemical and histochemical evidence for hyperserotonomic state in preeclampsia-eclampsia. Am J Obstet Gynecol (1996) 175:1543–50. doi: 10.1016/S0002-9378(96)70104-7
85. Lee N, Hebert MF, Wagner DJ, Easterling TR, Liang CJ, Rice K, et al. Organic cation transporter 3 facilitates fetal exposure to metformin during pregnancy. Mol Pharmacol (2018) 94:1125–31. doi: 10.1124/mol.118.112482
86. Huang WQ, Zhang CL, Di XY, Zhang RQ. Studies on the localization of 5-hydroxytryptamine and its receptors in human placenta. Placenta (1998) 19:655–61. doi: 10.1016/s0143-4004(98)90027-3
87. Pavličev M, Wagner GP, Chavan AR, Owens K, Maziarz J, Dunn-Fletcher C, et al. Single-cell transcriptomics of the human placenta: inferring the cell communication network of the maternal-fetal interface. Genome Res (2017) 27:349–61. doi: 10.1101/gr.207597.116
88. Suryawanshi H, Morozov P, Straus A, Sahasrabudhe N, Max KEA, Garzia A, et al. A single-cell survey of the human first-trimester placenta and decidua. Sci Adv (2018) 4:eaau4788. doi: 10.1126/sciadv.aau4788
89. Baković P, Kesić M, Perić M, Bečeheli I, Horvatiček M, George M, et al. Differential serotonin uptake mechanisms at the human maternal–fetal interface. Int J Mol Sci (2021) 22:7807. doi: 10.3390/ijms22157807
90. Prasad PD, Hoffmans BJ, Moe AJ, Smith CH, Leibach FH, Ganapathy V. Functional expression of the plasma membrane serotonin transporter but not the vesicular monoamine transporter in human placental trophoblasts and choriocarcinoma cells. Placenta (1996) 17:201–7. doi: 10.1016/s0143-4004(96)90039-9
91. Vaillancourt C, Petit A, Gallo-Payet N, Bellabarba D, Lehoux JG, Bélisle S. Labelling of D2-dopaminergic and 5-HT2-serotonergic binding sites in human trophoblastic cells using [3H]-spiperone. J Recept Res (1994) 14:11–22. doi: 10.3109/10799899409066993
92. Yavarone MS, Shuey DL, Sadler TW, Lauder JM. Serotonin uptake in the ectoplacental cone and placenta of the mouse. Placenta (1993) 14:149–61. doi: 10.1016/s0143-4004(05)80257-7
93. Bonnin A, Goeden N, Chen K, Wilson ML, King J, Shih JC, et al. A transient placental source of serotonin for the fetal forebrain. Nature (2011) 472:347–50. doi: 10.1038/nature09972
94. Balkovetz DF, Tiruppathi C, Leibach FH, Mahesh VB, Ganapathy V. Evidence for an imipramine-sensitive serotonin transporter in human placental brush-border membranes. J Biol Chem (1989) 264:2195–8. doi: 10.1016/S0021-9258(18)94161-X
95. Vachalova V, Karahoda R, Ottaviani M, Anandam KY, Abad C, Albrecht C, et al. Functional reorganization of monoamine transport systems during villous trophoblast differentiation: evidence of distinct differences between primary human trophoblasts and BeWo cells. Reprod Biol Endocrinol (2022) 20:112. doi: 10.1186/s12958-022-00981-8
96. Bertrand C, St-Louis J. Reactivities to serotonin and histamine in umbilical and placental vessels during the third trimester after normotensive pregnancies and pregnancies complicated by preeclampsia. Am J Obstet Gynecol (1999) 180:650–9. doi: 10.1016/s0002-9378(99)70268-1
97. Reviriego J, Fernandez-Alfonso MS, Marín J. Actions of vasoactive drugs on human placental vascular smooth muscle. Gen Pharmacol (1990) 21:719–27. doi: 10.1016/0306-3623(90)91024-l
98. Ugun-Klusek A, Tamang A, Loughna P, Billett E, Buckley G, Sivasubramaniam S. Reduced placental vascular reactivity to 5-hydroxytryptamine in pre-eclampsia and the status of 5HT(2A) receptors. Vascul Pharmacol (2011) 55:157–62. doi: 10.1016/j.vph.2011.07.006
99. Rothbauer M, Patel N, Gondola H, Siwetz M, Huppertz B, Ertl P. A comparative study of five physiological key parameters between four different human trophoblast-derived cell lines. Sci Rep (2017) 7:5892. doi: 10.1038/s41598-017-06364-z
100. Sheridan MA, Fernando RC, Gardner L, Hollinshead MS, Burton GJ, Moffett A, et al. Establishment and differentiation of long-term trophoblast organoid cultures from the human placenta. Nat Protoc (2020) 15:3441–63. doi: 10.1038/s41596-020-0381-x
101. Ciuclan L, Hussey MJ, Burton V, Good R, Duggan N, Beach S, et al. Imatinib attenuates hypoxia-induced pulmonary arterial hypertension pathology via reduction in 5-hydroxytryptamine through inhibition of tryptophan hydroxylase 1 expression. Am J Respir Crit Care Med (2013) 187:78–89. doi: 10.1164/rccm.201206-1028OC
102. Eddahibi S, Fabre V, Boni C, Martres MP, Raffestin B, Hamon M, et al. Induction of serotonin transporter by hypoxia in pulmonary vascular smooth muscle cells. Relatsh Mitogenic Action Serotonin Circ Res (1999) 84:329–36. doi: 10.1161/01.res.84.3.329
103. Oufkir T, Arseneault M, Sanderson JT, Vaillancourt C. The 5-HT2A serotonin receptor enhances cell viability, affects cell cycle progression and activates MEK–ERK1/2 and JAK2–STAT3 signalling pathways in human choriocarcinoma cell lines. Placenta (2010) 31:439–47. doi: 10.1016/j.placenta.2010.02.019
104. Oufkir T, Vaillancourt C. Phosphorylation of JAK2 by serotonin 5-HT (2A) receptor activates both STAT3 and ERK1/2 pathways and increases growth of JEG-3 human placental choriocarcinoma cell. Placenta (2011) 32:1033–40. doi: 10.1016/j.placenta.2011.09.005
105. Hadden C, Fahmi T, Cooper A, Savenka AV, Lupashin VV, Roberts DJ, et al. Serotonin transporter protects the placental cells against apoptosis in caspase 3-independent pathway. J Cell Physiol (2017) 232:3520–9. doi: 10.1002/jcp.25812
106. Hudon Thibeault A-A, de Los Santos YL, Doucet N, Sanderson JT, Vaillancourt C. Serotonin and serotonin reuptake inhibitors alter placental aromatase. J Steroid Biochem Mol Biol (2019) 195:105470. doi: 10.1016/j.jsbmb.2019.105470
107. Klempan T, Hudon-Thibeault A-A, Oufkir T, Vaillancourt C, Sanderson JT. Stimulation of serotonergic 5-HT2A receptor signaling increases placental aromatase (CYP19) activity and expression in BeWo and JEG-3 human choriocarcinoma cells. Placenta (2011) 32:651–6. doi: 10.1016/j.placenta.2011.06.003
108. Gibbs WS, Collier JB, Morris M, Beeson CC, Megyesi J, Schnellmann RG. 5-HT1F receptor regulates mitochondrial homeostasis and its loss potentiates acute kidney injury and impairs renal recovery. Am J Physiol Renal Physiol (2018) 315:F1119–28. doi: 10.1152/ajprenal.00077.2018
109. Wang Q, Zhang H, Xu H, Guo D, Shi H, Li Y, et al. 5-HTR3 and 5-HTR4 located on the mitochondrial membrane and functionally regulated mitochondrial functions. Sci Rep (2016) 6:37336. doi: 10.1038/srep37336
110. Murphy DL, Moya PR. Human serotonin transporter gene (SLC6A4) variants: their contributions to understanding pharmacogenomic and other functional G×G and G×E differences in health and disease. Curr Opin Pharmacol (2011) 11:3–10. doi: 10.1016/j.coph.2011.02.008
111. Philibert R, Madan A, Andersen A, Cadoret R, Packer H, Sandhu H. Serotonin transporter mRNA levels are associated with the methylation of an upstream CpG island. Am J Med Genet Part B: Neuropsychiatr Genet (2007) 144B:101–5. doi: 10.1002/ajmg.b.30414
112. Ramamoorthy S, Ramamoorthy JD, Prasad PD, Bhat GK, Mahesh VB, Leibach FH, et al. Regulation of the human serotonin transporter by interleukin-1 beta. Biochem Biophys Res Commun (1995) 216:560–7. doi: 10.1006/bbrc.1995.2659
113. Kong E, Sucic S, Monje FJ, Savalli G, Diao W, Khan D, et al. STAT3 controls IL6-dependent regulation of serotonin transporter function and depression-like behavior. Sci Rep (2015) 5:9009. doi: 10.1038/srep09009
114. Hudon Thibeault A-A, Laurent L, Vo Duy S, Sauvé S, Caron P, Guillemette C, et al. Fluoxetine and its active metabolite norfluoxetine disrupt estrogen synthesis in a co-culture model of the feto-placental unit. Mol Cell Endocrinol (2017) 442:32–9. doi: 10.1016/j.mce.2016.11.021
115. Andrade SE, Raebel MA, Brown J, Lane K, Livingston J, Boudreau D, et al. Use of antidepressant medications during pregnancy: a multisite study. Am J Obstet Gynecol (2008) 198:e1–5:194. doi: 10.1016/j.ajog.2007.07.036
116. Bakker MK, Kölling P, van den Berg PB, de Walle HEK, de Jong vdBLTW. Increase in use of selective serotonin reuptake inhibitors in pregnancy during the last decade, a population-based cohort study from the Netherlands. Br J Clin Pharmacol (2008) 65:600–6. doi: 10.1111/j.1365-2125.2007.03048.x
117. Cooper WO, Willy ME, Pont SJ, Ray WA. Increasing use of antidepressants in pregnancy. Am J Obstet Gynecol (2007) 196:544.e1–5. doi: 10.1016/j.ajog.2007.01.033
118. Smid MC, Metz TD, Gordon AJ. Stimulant use in pregnancy: An under-recognized epidemic among pregnant women. Clin Obstet Gynecol (2019) 62:168–84. doi: 10.1097/GRF.0000000000000418
119. Cool DR, Leibach FH, Bhalla VK, Mahesh VB, Ganapathy V. Expression and cyclic AMP-dependent regulation of a high affinity serotonin transporter in the human placental choriocarcinoma cell line (JAR). J Biol Chem (1991) 266:15750–7. doi: 10.1016/S0021-9258(18)98473-5
120. Cool DR, Leibach FH, Ganapathy V. High-affinity paroxetine binding to the human placental serotonin transporter. Am J Physiol (1990) 259:C196–204. doi: 10.1152/ajpcell.1990.259.2.C196
121. Cool DR, Liebach FH, Ganapathy V. Interaction of fluoxetine with the human placental serotonin transporter. Biochem Pharmacol (1990) 40:2161–7. doi: 10.1016/0006-2952(90)90249-k
122. Sabol SZ, Hu S, Hamer D. A functional polymorphism in the monoamine oxidase a gene promoter. Hum Genet (1998) 103:273–9. doi: 10.1007/s004390050816
123. Zhang H, Smith GN, Liu X, Holden JJA. Association of MAOA, 5-HTT, and NET promoter polymorphisms with gene expression and protein activity in human placentas. Physiol Genomics (2010) 42:85–92. doi: 10.1152/physiolgenomics.00220.2009
124. Shumay E, Fowler JS. Identification and characterization of putative methylation targets in the MAOA locus using bioinformatic approaches. Epigenetics (2010) 5:325–42. doi: 10.4161/epi.5.4.11719
125. Gumusoglu S, Scroggins S, Vignato J, Santillan D, Santillan M. The serotonin-immune axis in preeclampsia. Curr Hypertens Rep (2021) 23:37. doi: 10.1007/s11906-021-01155-4
126. Carrasco G, Cruz MA, Dominguez A, Gallardo V, Miguel P, González C. The expression and activity of monoamine oxidase a, but not of the serotonin transporter, is decreased in human placenta from pre-eclamptic pregnancies. Life Sci (2000) 67:2961–9. doi: 10.1016/S0024-3205(00)00883-3
127. Pinter SZ, Kripfgans OD, Treadwell MC, Kneitel AW, Fowlkes JB, Rubin JM. Evaluation of umbilical vein blood volume flow in preeclampsia by angle-independent 3D sonography. J Ultrasound Med (2018) 37:1633–40. doi: 10.1002/jum.14507
128. Murthi P, Vaillancourt C. Placental serotonin systems in pregnancy metabolic complications associated with maternal obesity and gestational diabetes mellitus. Biochim Biophys Acta - Mol Basis Dis (2020) 1866:165391. doi: 10.1016/j.bbadis.2019.01.017
129. Horvatiček M, Perić M, Bečeheli I, Klasić M, Žutić M, Kesić M, et al. Maternal metabolic state and fetal sex and genotype modulate methylation of the serotonin receptor type 2A gene (HTR2A) in the human placenta. Biomedicines (2022) 10:467. doi: 10.3390/biomedicines10020467
130. Blakeley PM, Capron LE, Jensen AB, O’Donnell KJ, Glover V. Maternal prenatal symptoms of depression and down regulation of placental monoamine oxidase a expression. J Psychosom Res (2013) 75:341–5. doi: 10.1016/j.jpsychores.2013.07.002
131. Ruyak SL, Noor S, DiDomenico J, Sun MS, Fernandez Oropeza AK, Rodriguez DE, et al. Effects of prenatal opioid and alcohol exposures on immune and serotonin factors in human placenta. Exp Neurol (2022) 353:114057. doi: 10.1016/j.expneurol.2022.114057
132. Karahoda R, Robles M, Marushka J, Stranik J, Abad C, Horackova H, et al. Prenatal inflammation as a link between placental expression signature of tryptophan metabolism and preterm birth. Hum Mol Genet (2021) 30:2053–67. doi: 10.1093/hmg/ddab169
133. Côté F, Fligny C, Bayard E, Launay J-M, Gershon MD, Mallet J, et al. Maternal serotonin is crucial for murine embryonic development. Proc Natl Acad Sci U.S.A. (2007) 104:329–34. doi: 10.1073/pnas.0606722104
134. Fligny C, Fromes Y, Bonnin P, Darmon M, Bayard E, Launay J-M, et al. Maternal serotonin influences cardiac function in adult offspring. FASEB J (2008) 22:2340–9. doi: 10.1096/fj.07-100743
135. Takahashi H, Nakashima S, Ohama E, Takeda S, Ikuta F. Distribution of serotonin-containing cell bodies in the brainstem of the human fetus determined with immunohistochemistry using antiserotonin serum. Brain Dev (1986) 8:355–65. doi: 10.1016/s0387-7604(86)80055-9
136. McKinney J, Knappskog PM, Pereira J, Ekern T, Toska K, Kuitert BB, et al. Expression and purification of human tryptophan hydroxylase from escherichia coli and pichia pastoris. Protein Expression Purification (2004) 33:185–94. doi: 10.1016/j.pep.2003.09.014
137. Mathiesen L, Bay-Richter C, Wegener G, Liebenberg N, Knudsen LE. Maternal stress and placental function; ex vivo placental perfusion studying cortisol, cortisone, tryptophan and serotonin. PloS One (2020) 15:e0233979. doi: 10.1371/journal.pone.0233979
138. Hjort L, Novakovic B, Cvitic S, Saffery R, Damm P, Desoye G. Placental DNA methylation in pregnancies complicated by maternal diabetes and/or obesity: State of the art and research gaps. Epigenetics (2022) 17:1–21. doi: 10.1080/15592294.2022.2111755
139. Halmøy A, Johansson S, Winge I, McKinney JA, Knappskog PM, Haavik J. Attention-deficit/hyperactivity disorder symptoms in offspring of mothers with impaired serotonin production. Arch Gen Psychiatry (2010) 67:1033–43. doi: 10.1001/archgenpsychiatry.2010.124
140. Leboyer M, Philippe A, Bouvard M, Guilloud-Bataille M, Bondoux D, Tabuteau F, et al. Whole blood serotonin and plasma beta-endorphin in autistic probands and their first-degree relatives. Biol Psychiatry (1999) 45:158–63. doi: 10.1016/s0006-3223(97)00532-5
141. Paquette AG, Lesseur C, Armstrong DA, Koestler DC, Appleton AA, Lester BM, et al. Placental HTR2A methylation is associated with infant neurobehavioral outcomes. Epigenetics (2013) 8:796–801. doi: 10.4161/epi.25358
142. Räikkönen K, Pesonen A-K, O’Reilly JR, Tuovinen S, Lahti M, Kajantie E, et al. Maternal depressive symptoms during pregnancy, placental expression of genes regulating glucocorticoid and serotonin function and infant regulatory behaviors. Psychol Med (2015) 45:3217–26. doi: 10.1017/S003329171500121X
143. Pehme PM, Zhang W, Finik J, Pritchett A, Buthmann J, Dana K, et al. Placental MAOA expression mediates prenatal stress effects on temperament in 12-month-olds. Infant Child Dev (2018) 27:e2094. doi: 10.1002/icd.2094
144. Köhler S, Cierpinsky K, Kronenberg G, Adli M. The serotonergic system in the neurobiology of depression: Relevance for novel antidepressants. J Psychopharmacol (2016) 30:13–22. doi: 10.1177/0269881115609072
145. Garfield AS, Heisler LK. Pharmacological targeting of the serotonergic system for the treatment of obesity. J Physiol (2009) 587:49–60. doi: 10.1113/jphysiol.2008.164152
146. Beattie D, Smith J. Serotonin pharmacology in the gastrointestianl tract: a review. Naunyn-Schmiedeberg’s Arch Pharmacol (2008) 377:181–203. doi: 10.1007/s00210-008-0276-9
147. Hernández-Hernández OT, Martínez-Mota L, Herrera-Pérez JJ, Jiménez-Rubio G. Role of estradiol in the expression of genes involved in serotonin neurotransmission: Implications for female depression. Curr Neuropharmacol (2019) 17:459–71. doi: 10.2174/1570159X16666180628165107
Keywords: human placenta, pregnancy, serotonin, serotonin receptors, serotonin transporters, MAO, TPH, fetal sex
Citation: Perić M, Bečeheli I, Čičin-Šain L, Desoye G and Štefulj J (2022) Serotonin system in the human placenta – the knowns and unknowns. Front. Endocrinol. 13:1061317. doi: 10.3389/fendo.2022.1061317
Received: 04 October 2022; Accepted: 10 November 2022;
Published: 01 December 2022.
Edited by:
Alicia E. Damiano, Faculty of Pharmacy and Biochemistry, University of Buenos Aires, ArgentinaReviewed by:
Gendie Lash, Guangzhou Medical University, ChinaFernanda Regina Giachini, Federal University of Mato Grosso, Brazil
Copyright © 2022 Perić, Bečeheli, Čičin-Šain, Desoye and Štefulj. This is an open-access article distributed under the terms of the Creative Commons Attribution License (CC BY). The use, distribution or reproduction in other forums is permitted, provided the original author(s) and the copyright owner(s) are credited and that the original publication in this journal is cited, in accordance with accepted academic practice. No use, distribution or reproduction is permitted which does not comply with these terms.
*Correspondence: Jasminka Štefulj, amFzbWlua2Euc3RlZnVsakBpcmIuaHI=
†These authors have contributed equally to this work and share first authorship