- 1Department of Science Education and Communication, Delft University of Technology, Delft, Netherlands
- 2Institute of Education and Child Studies, Leiden University, Leiden, Netherlands
- 3Department of Mathematics, Paris Lodron University of Salzburg, Salzburg, Austria
- 4School of Electrical and Electronic Engineering, Technological University Dublin, Dublin, Ireland
Empirical interdisciplinary research has explored the role of spatial ability in STEM learning and achievement. While most of this research indicates that fostering spatial thinking in educational contexts has the potential to positively impact students’ enrollment and performance in STEM subjects, there is less agreement on the best approach to do so. This article provides an overview of various types of effective spatial interventions and practices in formal or informal educational contexts, including targeted training of STEM-relevant spatial skills, spatialized curricula embedded in schools, integrated STEM practices addressing students’ use of spatial skills, and spatial activities in informal STEM education. Gender and socio-economic status of students – two variables that have been found to moderate the relationship between students’ spatial ability and their STEM performance – are also discussed in this article. Drawing on a wide spectrum of perspectives on situating spatial ability research in STEM education contexts, this article underscores the need for further inquiry into opportunities for developing K-12 students’ spatial ability through integrated and informal STEM practices. This article proposes a conjecture that the relationship between developing students’ spatial ability and enhancing their abilities to solve spatially complex STEM problems is bidirectional. Recommendations for future research are made on lingering questions about the effect of interventions, untapped resources for spatial ability training in formal and informal STEM education, and educational strategies for developing students’ spatial ability in authentic learning environments.
1. Introduction
Much of society values scientific knowledge and technological development as vital contributors to economic growth, innovation, and welfare (Davies and Horst, 2016; Freeman et al., 2019). Attracting more students to study Science, Technology, Engineering, and Mathematics (STEM) from primary, secondary, and university-levels of education has been on national agendas across the world (Rocard et al., 2007; Joyce and Dzoga, 2011; National Research Council, 2011; Gough, 2015; Fatourou et al., 2019). Variables such as interest in STEM (Caprile et al., 2015), a sense of belonging in STEM fields (Dortch and Patel, 2017; Murphy et al., 2020), and self-efficacy in learning STEM (Lent et al., 2010; Tracey, 2010) all influence students’ decisions to follow STEM studies and careers, and have been the focus of efforts to encourage students to enter the STEM field (Kearney, 2011; Caprile et al., 2015). Surprisingly, initiatives promoting STEM education have paid relatively little attention to cognitive factors such as the spatial ability levels of students, which appears to be a key predictor of future academic and professional involvement in the STEM field (Shea et al., 2001; Wai et al., 2009; Kell et al., 2013).
Spatial ability refers to the competence in representing and processing the location of objects, their shape, their relation to each other, and the orbits they take as they move (Newcombe, 2010); and can be developed through training and education (Uttal et al., 2013a). In a meta-analysis of over 200 studies, Uttal et al. (2013a) found that training students to think spatially and apply spatial skills led to significant increases in their spatial ability, with an average effect size of 0.47. Furthermore, spatial training interventions can potentially lead to transfer of gains to other domains, such as mathematics (Gilligan et al., 2020). Although most research on spatial ability and STEM performance has been situated in college education (e.g., Lord, 1987; Sorby, 2009; Hegarty, 2014), it is critical to make use of the formal and informal resources from early childhood on (Newcombe, 2010; Newcombe and Frick, 2010; Hawes et al., 2017). In fact, an increasing amount of research resources is being devoted to developing spatial interventions or spatialized curricula for K-12 education (e.g., Casey et al., 2008; Burte et al., 2017; Hawes et al., 2017; Lowrie et al., 2017; Sorby and Veurink, 2019).
Through this paper, we aim to characterize a general state of knowledge on the various types of practices that can foster spatial ability development in K-12 educational contexts, drawing insights from different disciplines, including cognitive psychology (e.g., Uttal et al., 2013a; Hawes et al., 2017; Mix, 2019; Gilligan et al., 2020), educational science (e.g., Sorby, 2009; Newcombe and Frick, 2010; Casey et al., 2011; Lowrie et al., 2019), and STEM education (e.g., Burte et al., 2017; Ramey and Uttal, 2017; Julià and Antolì, 2018; Atit et al., 2020). We specifically examine how educational spatial interventions and practices have been situated in authentic, formal and informal STEM environments. By authentic learning environments we mean “situating learning tasks in the context of future use” and in “real-world situations” (Herrington et al., 2014, p. 401). We begin with a section outlining spatial ability and its relation to STEM learning from a cognitive psychology perspective. In this section, we draw heavily on the literature about space-math associations, as math is the first STEM subject officially taught in school and fundamental to most other STEM subjects, and is also the subject that has been researched most extensively in the field of spatial cognition. We then inspect the different approaches used to develop students’ spatial ability, covering a wide range of interventions and educational practices, including targeted training of STEM-relevant spatial skills, spatialized curricula, integrated STEM practices that address students’ use of spatial skills, and spatial activities in informal STEM education. We focus on if and how integrated STEM practices and informal STEM learning can be harnessed and further developed to stimulate spatial skills development, as they present opportunities for spatial training in authentic learning environments. Lastly, as the goals of spatial interventions or spatialized educational practices always point to developing individual students’ spatial ability, we find it important to also discuss how individual variables, such as gender and socio-economic status, may moderate the relationship between students’ spatial ability development and STEM learning. We discuss how future interventions and educational practices aiming to develop students’ spatial abilities in STEM education contexts may benefit from taking these two variables into account.
2. The role of spatial ability in STEM learning
2.1. Understanding spatial ability: Typology of spatial skills
A clear classification and precise description of spatial skills are important for the development and evaluation of interventions (Buckley et al., 2018), as it provides a basis for systematic exploration of whether, how, and why some interventions may or may not be effective for training specific spatial skills. To create classifications, several researchers have followed a psychometric approach. Linn and Petersen (1985) distinguished between three categories of spatial skills, i.e., mental rotation, spatial perception, and spatial visualization. Mental rotation refers to the ability to mentally manipulate and rotate two- or three-dimensional objects; spatial perception requires individuals to ignore distracting information and determine the spatial relationship regarding their own orientation, e.g., perceiving the water level when the container is tilted; spatial visualization refers to the ability to carry out multistep manipulations of spatial information, e.g., visualizing a piece of paper being folded. Others have argued that this typology is too ambiguous and too broad to categorize tasks that may not be conceptually related (Voyer et al., 1995). The extended Cattell-Horn-Carroll framework (McGrew, 2009) suggested 11 spatial factors based on empirical support, including visualization, spatial relation, closure speed, flexibility of closure visual memory, spatial scanning, serial perceptual integration, length estimation, perceptual illusions, perceptual alternation, and imagery. Despite the fact that more spatial factors have been identified, there are discrepancies between their conceptualizations. A lack of theory-driven classification may be one of the reasons for these discrepancies (Uttal et al., 2013a).
In response to the lack of consensus regarding the definition and classification of spatial ability, Uttal et al. (2013a) adopted a classification system that grew out of linguistics, cognitive science, and neuroscience to distinguish different spatial abilities along two dimensions: intrinsic-extrinsic and static-dynamic (Palmer, 1978; Talmy, 2000; Chatterjee, 2008). The intrinsic-extrinsic dimension is classified according to whether the spatial information is within a single object or between multiple objects, while the static-dynamic dimension is classified according to whether transformation or movement is involved (Uttal et al., 2013a; Newcombe and Shipley, 2014). A two-by-two classification of spatial skills by combining these two fundamental dimensions renders four distinct sub-domains: intrinsic-static, intrinsic-dynamic, extrinsic-static, and extrinsic-dynamic, as shown in Figure 1. This classification cleverly reflects different components of spatial skills that have been described in previous research, such as the above-mentioned categories by Linn and Petersen (1985), and the additional categories, visuo-spatial perceptual speed and spatial relations, mentioned by Carroll (1993).
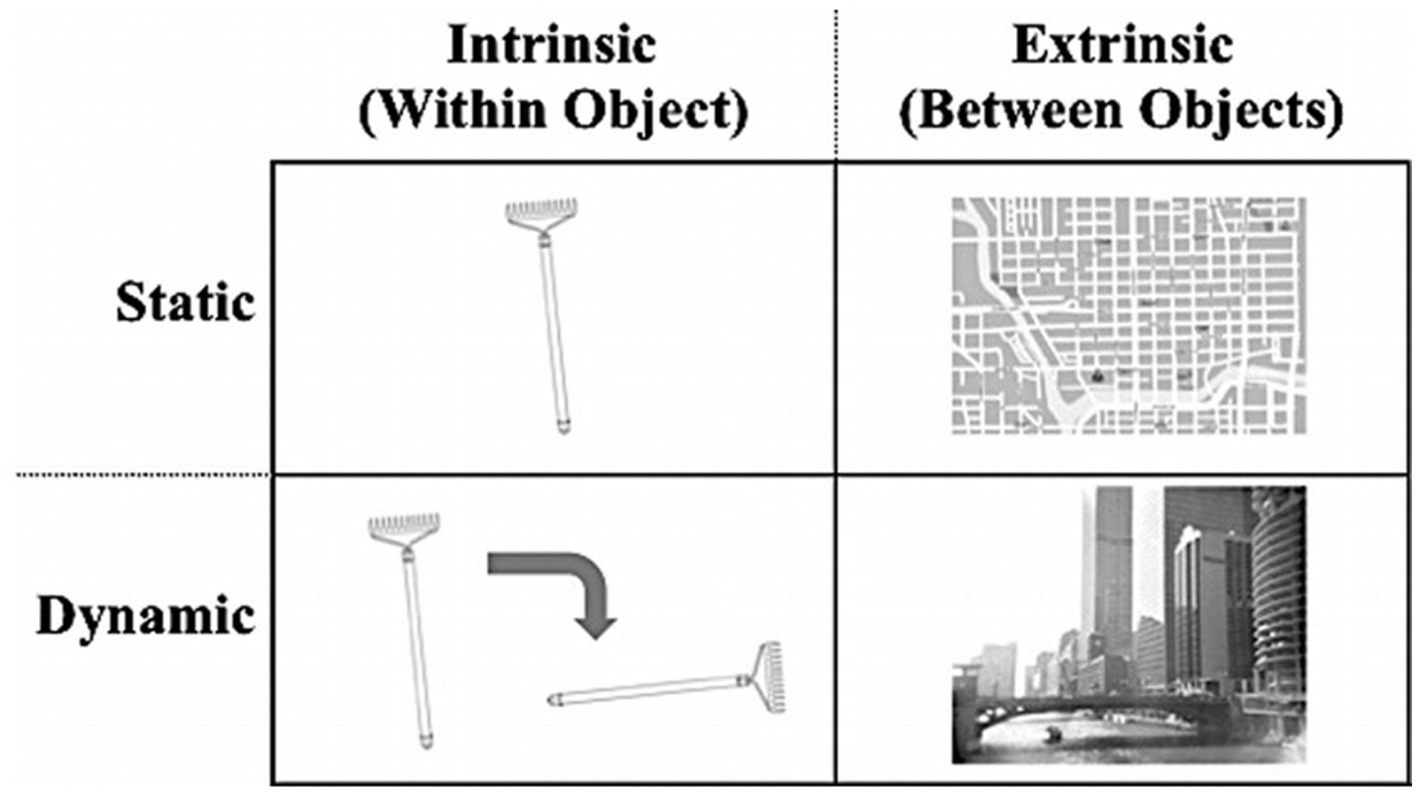
Figure 1. A two-by-two classification of spatial skills proposed by Uttal et al. (2013a). Reprinted with permission.
The two-by-two classification has been adopted and supported by multiple studies (e.g., Taylor and Hutton, 2013; Gilligan et al., 2018; Hodgkiss et al., 2018). Nevertheless, there was also a debate on whether spatial skill is unitary or multidimensional (Mix et al., 2016; Carroll, (1993)). Based on the two-by-two classification, Mix et al. (2018) conducted factor analyses on kindergarteners, third and sixth graders. The result indicated that the two-factor (intrinsic-extrinsic) model was the best fit for both kindergarteners and third graders, while the one-factor model fits better for sixth graders. This result suggested that the two-by-two framework, especially the dynamic-static dimension, may not accurately classify the latent structure underlying elementary school children’s spatial performance.
2.2. Relationship between spatial ability and STEM performance
Several studies have described a relationship between spatial ability and STEM learning. For example, in the domain of math, positive correlations between spatial ability and math performance have been found among preschoolers (Kyttälä et al., 2003; Rasmussen and Bisanz, 2005), middle childhood (Casey et al., 2001; Geary et al., 2007), preadolescence and young adulthood (Shea et al., 2001; Webb et al., 2007), suggesting that people who perform better in spatial tasks also achieve better overall mathematics performance. The correlation between spatial ability and math involves different subdomains of math, including subdomains that, superficially, do not appear to be spatial (Mix et al., 2016, 2018). For example, positive correlations have been found with geometry and word problem solving (Delgado and Prieto, 2004; Kyttälä and Lehto, 2008), as well as with arithmetic (Reuhkala, 2001) and early numeracy (Kyttälä et al., 2003; Lefevre et al., 2010). Besides math, spatial ability also correlates with performance in other STEM domains, including engineering (Sorby, 2009), geoscience (Atit et al., 2015), chemistry (Stieff et al., 2012), computer programming (Jones and Burnett, 2008), design (Lin, 2016), and medical studies (Hegarty et al., 2007).
Although many studies support the relationship between spatial ability and STEM performance, it is still unclear why they are associated, making it difficult to predict to what extent improvement of spatial ability would directly benefit STEM performance. Drawing from the literature on space-math associations (e.g., Mix, 2019; Hawes and Ansari, 2020; Hawes et al., 2022), it is likely that there are multiple ways in which spatial cognition and STEM learning are related. For example, in the domain of arithmetic, Mix (2019) theorized that spatial ability may allow individuals to decode the spatial arrangement of symbols in mathematical equations (e.g., differentiate 15 from 51). It further allows individuals to form mental representations of math problems (e.g., imagine the scene of a math problem), which is a critical step to successful problem solving (Duffy et al., 2020). Lastly, the mental number line may serve as the spatial representation that helps children solve arithmetic problems (e.g., locate the first addend and count up the number of spaces for the second addend). Thus, children with strong spatial skills may find it easier to understand and implement these skills.
Hawes and Ansari (2020) proposed four explanatory accounts to explain why spatial ability and math learning are related. The spatial representation of number account, spatial modeling account, shared neural processing account, and working memory account. The first two accounts are similar to what Mix (2019) proposed, emphasizing the role of the number line and spatial representation. The shared neural processing account argues that spatial and math are related because they rely on the same brain regions. The working memory account assumes that individual differences in visual–spatial working memory are responsible for processing the short-term storage of visual and spatial information (Baddeley, 1993), therefore explaining the relation between spatial and math.
The above theoretical accounts highlighted potential mechanisms underlying the space-math associations. Besides, Gunderson et al. (2012) demonstrated from two longitudinal studies that children’s mental transformation ability at age 5 significantly predicted their approximate calculation performance at age 8 [β = 0.34, t (151) = 2.27, p < 0.05], with their numerical knowledge measured by the number line estimation task at age 6 mediating this relation [β = 0.51, t (151) = 3.26, p = 0.001]. These empirical findings suggested that mental transformation ability may help children create a meaningful numerical representation, which allows them to better comprehend the linear number line, similar to what the above-mentioned spatial representation of number account suggested.
However, many questions remain, such as why and under what conditions would these mechanisms work and no causal relation could be drawn without an experimental study. Future studies are needed to examine the above-mentioned mechanisms empirically in the area of math as well as in STEM. For example, the spatial representation of number account and spatial modeling account could be tested by providing children with spatial training to see if they achieve a better estimation of the number line or are more likely to form accurate and complete schematic representations in math problems.
3. Developing students’ spatial ability through STEM education
3.1. Spatial interventions and transfer to STEM performance
Empirical studies have shown that spatial skills are malleable (Uttal et al., 2013a). This malleability on the one hand and the well-established connection between spatial and STEM skills (Wai et al., 2009; Hodgkiss et al., 2018; Young et al., 2018) on the other, raise the question of whether training spatial skills will improve STEM performance. A recent meta-analysis on studies examining transfer of spatial training to mathematical skills (Hawes et al., 2022) showed from 29 studies that spatial training can have a positive effect on both spatial skills and mathematics performance. Specifically, the meta-analysis showed that spatial training improved spatial skills with a moderate average effect size [Hedges’s g = 0.49] and enhanced mathematics performance with a small to moderate average effect size [g = 0.28]. However, the effect varied depending on age, type of material, and type of transfer. Given the divergence of the outcomes regarding the effectiveness of spatial training on mathematics, the present section attempts to provide an overview of studies that can suggest optimal results in the educational settings.
The section below presents spatial training programs that show transfer to STEM performance in formal K-12 education, specifically in mathematics, from various countries using various methods and factors. We organized the section based on two training approaches: “spatial interventions” and “spatializing the curriculum interventions.” Spatial interventions target specific spatial skills, such as spatial visualization and mental rotation, using physical, digital, or hybrid materials and then examine the extent to which mathematics performance is improved. Whereas, spatializing the curriculum interventions seek to enrich the instructions in a classroom with spatial elements. We conclude this section by discussing how these interventions make use of formats or materials that aim to mimic authentic learning experiences.
3.2. Spatial interventions that show transfer to mathematics
3.2.1. Physical format
A study by Burte et al. (2017) is an example of using hands-on materials to improve third to sixth-grade students’ spatial thinking in the U.S. with the objective to support their mathematical performance. More specifically, the aim of the program is for students to engage in practices that are relevant to mathematical concepts (e.g., dividing a paper into fractional parts) and scientific reasoning (making sense of a diagram). The hands-on materials they used were origami and pop-up paper, part of an engineering-based program called “Think3D!.” The training included paper-folding and -cutting tasks for students to construct three-dimensional objects, interpret diagrams and solve real-life problems. The intermediate steps of this activity are similar to the Mental Paper Folding task and as such, allow students to train their mental rotation skills in an embodied manner. The construction activities nudged students to use spatial language, which is commonly used in math learning, such as angle, corner, direction, line, position, shape, side, and symmetry. These practices may also be used to spatialize the mathematics instruction, a spatial training approach we will be discussing in section 3.1.2. Results from this study revealed an interaction between grade and improved accuracy in visual representation problems [F(3, 77) = 3.38, p < 0.05, η2 = 0.04] and abstract math problem-solving [F(3, 79) = 5.11, p < 0.01, η2 = 0.06], with fifth and sixth graders improving but not third and fourth graders. An interaction was also found between grade and math problems that required spatial thinking [F(3, 79) = 10.35, p < 0.001, η2 = 0.11], with only students at higher grades showing an improvement. These findings suggested that spatial and mathematical gains from this embodied spatial intervention may be moderated by the age and the developmental stage of students. We argue that although this study indicated a potential association between the effectiveness of embodied training and students’ developmental stage (Burte et al., 2017), this conclusion is tentative and requires further research, including studies with a control group, or an examination of whether the activities used in the training were appropriate for younger students.
3.2.2. Digital format
In a different type of study, Gilligan et al. (2020) delivered computer-based spatial training on mental rotation or spatial scaling to 8-year-old students in the UK, following a randomized, controlled, pre-post training design. Students in the control group were trained in word reading. Students in the training groups (mental rotation; spatial scaling; word reading) were trained either by implicit or explicit video instruction within the school environment. Explicit-instruction-based training consisted of videos that instructed students on how to solve either mental rotation, spatial scaling, or word reading tasks. In the implicit-instruction-based training, students chose the answer they believed to be correct without receiving any guidance. Instead, these students received feedback on whether their answer was correct or not. Findings from this study are insightful as the brief, 3- to 6-min spatial training resulted in gains in the particular spatial skills that were trained (near transfer), the untrained spatial skills (intermediate transfer), and in mathematical tasks as well (far transfer). As far as the mathematical gains are concerned, students who received spatial scaling training significantly improved their performance on the number line estimation task [t(79) = 2.12, p = 0.037, d = 0.236]. Gilligan and colleagues suggested that this transfer is potentially due to the proportional reasoning demanded in both tasks. Students who received mental rotation training improved significantly on missing term problems [t(69) = 2.73, p = 0.008, d = 0.241], regardless of whether the instruction was explicit or implicit. This finding was in line with the results from Cheng and Mix (2014), who found that mental rotation training improved 6- to-8-year-old students’ performance at missing term tasks [t(30) = 2.79, p = 0.005]. However, in the study by Hawes et al. (2015), implicit mental rotation training failed to replicate Cheng and Mix’s (2014) results. In regard to why outcomes have not been consistent in these three studies, Gilligan and colleagues hypothesized that the common feature of a part-whole type of mental rotation training1 between the two studies may have been the catalytic factor for success. Another insight from this study is the “direct causal effect of spatial skills on mathematical performance,” without the authors ruling out the possibility of a “bidirectional relationship” between the two (Gilligan et al., 2020, p. 16), which requires further investigation. Meanwhile, computer-based spatial training like this provides a promising practice of spatial training both in terms of its cognitive outcomes and its feasibility, as it can be integrated into the math classroom by having students work on simple spatial activities on electronic devices.
3.2.3. Hybrid format
The classroom-based spatial training by Lowrie et al. (2019) combined the use of hands-on manipulatives with the extensive use of digital instruments. This three-week intervention was aimed at training spatial visualization of 10- to 12-year-old students in Australia. The intervention followed the Experience-Language-Pictorial-Symbolic-Application (ELPSA) pedagogical framework (see Lowrie and Patahuddin, 2015), emphasizing the Pictorial component. “Pictorial” stands for problem visualization, making sense of visual representations, and checking predictions after physically manipulating stimuli. In addition to using hands-on resources, this intervention included tasks in which students used digital applications to create symmetrical objects, predict how nets can form a cube, and explore which two-dimensional shapes can be formed from cutting three-dimensional objects. Compared to the control group, students who received the spatial training showed a moderate improvement in their mathematics performance [t(17) = 6.95, p = 0.016, d = 0.39], especially on geometry tasks [t(17) = 5.92, p = 0.025], and word problems [t(17) = 6.11, p = 0.023]. Importantly, this intervention can be conveniently implemented by teachers in their daily instruction after 10 hours of professional development training.
To conclude, studies have now shown that spatial skills can be trained, and that training may lead to enhanced performance in STEM subjects (e.g., Uttal et al., 2013a; Hawes et al., 2022). As it is currently unclear what the underlying mechanisms responsible for training and transfer effects are, it is still difficult to evaluate the effectiveness of spatial training. There are multiple ways in which spatial cognition and STEM performance might be related, such as the spatial representation of numbers account discussed in section 2.2, and the format and materials used in STEM tasks discussed in this section. Further research is needed to study which of those (or other) factors should be the primary target of intervention studies. Moreover, the occurrence of transfer irrespective of the length of training and the amount of spatial gains (Hawes et al., 2022) may suggest that transfer is not necessarily achieved through enhanced spatial skills or refined representations. Instead, spatial training may exert its effects by nudging students to use a spatial problem solving approach during STEM tasks. This hypothesis needs to be tested in future research. Research is also needed to compare the effectiveness of different types of spatial training and examine contextual and individual factors that facilitate training effects. In addition, the meta-analysis by Hawes et al. (2022) suggested that training gain and transfer of spatial gains to mathematics seem to be enhanced when hands-on materials are used, as opposed to computerized materials. Together, these findings suggest that efforts to reinforce the connection between spatial and STEM tasks may help to further stimulate transfer, particularly if hands-on materials are used.
3.2.4. Spatialized curriculum interventions
One way to enhance the connection between spatial cognition and STEM learning is to “spatialize the curriculum.” This approach has the potential to not only develop students’ spatial skills but also allow students to directly apply such skills while learning subject knowledge like mathematics. An additional advantage of spatializing the curriculum is to enhance the already existing instructions with spatial components, such as the use of spatial gestures and spatial language, without adding extra hours to the school schedule (Newcombe, 2017). Meanwhile, in order to spatialize the curriculum, new curriculum materials have to be designed and teachers need to be trained in order to spatially enhance their instruction (Newcombe, 2017).
An example where this approach was taken is the intervention by Hawes et al. (2017). This intervention focused on spatial visualization with training integrated into the routine for kindergarten to K-2 (4- to 7-year-old students in Canada) mathematics teaching during a thirty-two-week period. The researchers, using a design research paradigm, collaborated with teachers in order to develop and field test teaching plans that would provide opportunities for students to learn early geometry in a dynamic spatial manner. Moreover, these teaching plans incorporated activities for the students to gradually develop their spatial visualization skills. The goal of this intervention was to not teach geometry in a static way but provide the opportunity for students to realize geometric shapes through their spatial transformations. Through playful inquiry, students explored the possible two or three-dimensional configurations of five square tiles and cubes respectively, compared and studied the produced objects, and dynamically practiced axial reflection symmetry and area measurement all using hands-on material (see Figure 2. for an example). Compared to the control class, students in the intervention classes showed a greater improvement in spatial language, visual–spatial geometry, two-dimensional mental rotation, and in symbolic number comparison by the end of the academic school year. Another positive aspect of this particular effort to spatialize early geometry teaching is the high level of engagement demonstrated by both students and teachers.
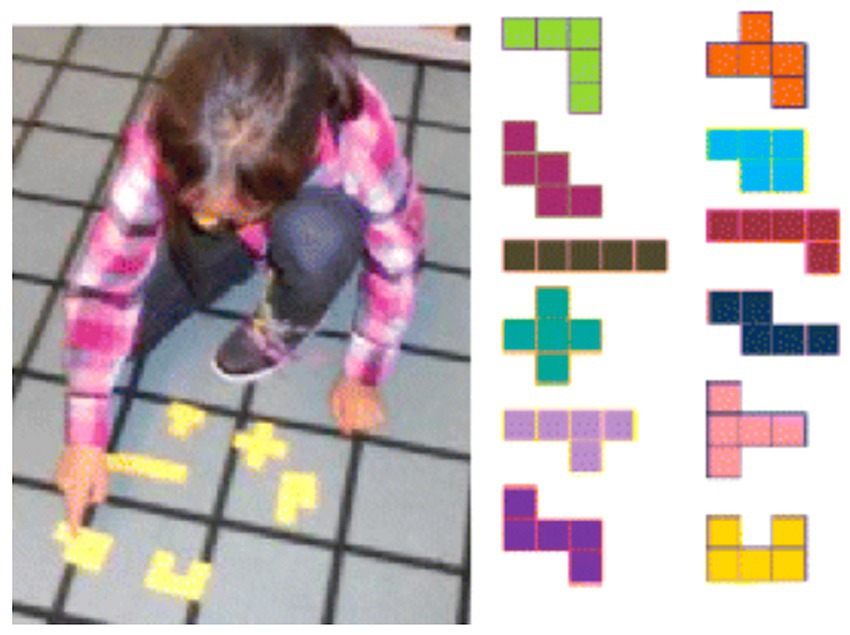
Figure 2. Example of a hands-on activity requiring visualization and mental rotation skills within a spatialized math curriculum (Hawes et al., 2017). Reprinted with permission.
3.2.5. Promising results: Where do the next steps lie?
In this section, we presented various modes of spatial training delivery that not only helped students improve their spatial abilities but also showed transfer to STEM learning, such as mathematics. However, research examining transfer of spatial interventions to performance in STEM subjects is still relatively new (Hawes et al., 2022). As a majority of the research on transfer has been centered around the transfer to mathematics, transfer from spatial interventions to performance in other STEM subjects, such as science, engineering, and technology, warrants further investigation (Uttal and Cohen, 2012; Stieff and Uttal, 2015; Margulieux, 2020).
Spatial interventions employing hands-on materials, computer-based instruction with feedback, or a mix of both, as well as the efforts to spatialize STEM curricula, all indicate a shift of focus from developing students’ spatial ability through paper and pencil task training to training that closely resembles how students learn together in classroom environments. Whether it is the incorporation of physical and virtual manipulatives, instructional feedback, or playful inquiry, we can see that spatial interventions developed in recent decades are becoming increasingly intentional in mimicking authentic learning experiences. In the following sections, we continue to explore opportunities to develop spatial ability in authentic learning environments.
3.3. Developing students’ spatial ability through integrated STEM education
Looking at STEM education holistically, there is a lack of consensus on what STEM education actually entails, as the definition of STEM varies greatly across contexts and among various stakeholders such as researchers, teachers, and school administrators (Brown et al., 2011). Given this variability, it is important that we clarify the kind of STEM education we are focusing on in this section.
Instead of describing STEM as merely a cluster of science, technology, engineering, and mathematics subjects, the term, integrated STEM education, has gained increasing recognition from educational researchers in North America, Europe, and Asia (e.g., Stohlmann et al., 2012; Bryan et al., 2015; Mustafa et al., 2016; Thibaut et al., 2018). Kelley and Knowles (2016) defined integrated STEM education as “the approach to teaching the STEM content of two or more STEM domains, bound by STEM practices within an authentic context for the purpose of connecting these subjects to enhance student learning” (p. 3). Carefully designed integrated STEM education can lead to a range of desirable learning outcomes, such as increased knowledge in STEM (Kelley et al., 2022), improved test performance in subjects like math and science (Tillman et al., 2014), enhanced problem-solving skills (Netwong, 2018), a more positive attitude toward STEM (Sisman et al., 2021), and higher levels of engagement with STEM courses (Taylor and Hutton, 2013; Peng and Sollervall, 2014). Given that STEM education is, at its core, an intersection of different disciplines, we believe it is valuable to discuss where and how researchers and practitioners of integrated STEM may support students’ spatial ability development.
To enhance students’ spatial ability in authentic learning contexts, we need to prepare students with spatial knowledge and spatial thinking skills that address the challenges they face in different disciplines (e.g., Hinze et al., 2014; Stieff et al., 2014; Atit et al., 2020). Instead of taking the learning of spatial skills as a separate domain, the National Research Council in the U.S. recommended viewing it as a “missing link” across different subject knowledge that “permeates” different disciplines (2006, p. 7). Thus, understanding how to develop students’ spatial thinking skills in integrated STEM education not only aligns with the goal of developing spatial skills with content knowledge but also renders a possible answer to how spatial thinking may tie the learning in different STEM subjects integratively.
In the conceptual framework for integrated STEM education, Kelley and Knowles (2016) highlighted four learning approaches that well accommodate integrated STEM learning, including engineering design, technological literacy, scientific inquiry, and mathematical thinking. What ground the four approaches are the shared practices across disciplines and the situated context for learning, so that “learning is authentic and relevant, therefore representative of an experience found in actual STEM practice” (Kelley and Knowles, 2016, p. 4). In the following subsections, we present an overview of how existing spatial interventions or spatialized educational practices leverage each of the four integrated STEM education approaches, and how integrated STEM problems that originate from real-world problems can be used to challenge students’ spatial skills development.
3.3.1. Engaging students in spatial thinking through engineering design
Engineering design projects offer a promising platform to weave knowledge and skills needed for different disciplines together while challenging students’ inquiry, analytical, and problem-solving skills (Kelley and Knowles, 2016). Several interventions that target students’ spatial ability development have been delivered in the form of engineering design projects (e.g., Taylor and Hutton, 2013; Ramey and Uttal, 2017). For example, a programming robot intervention for grade 4th to 6th students led to an increase in a range of spatial reasoning skills such as building 3D objects from pictures and shape rotation (Francis et al., 2021). Francis and colleagues reported significant improvement in the spatial task performance from both the week-long short-term group (e.g., in building 3D objects from pictures, [t(36) = −2.9, p < 0.05]) and the one-year, long-term group (e.g., in shape rotation, [t(47) = −3.0, p < 0.05]).
Julià and Antolì (2018) developed a multidisciplinary STEM course on robotics to develop students’ spatial skills and mechanical reasoning skills. This year-long course for sixth and seventh-grade students used educational robotics kits to promote hands-on, collaborative learning. Students were given the chance to solve mechanical modeling and building problems in groups, which activated their knowledge and skills related to engineering and technology. The classroom teacher observed that the course also helped students to learn concepts related to science, engineering, and technology in addition to mathematics. To gain a comprehensive view of students’ spatial ability, this study tested students with a variety of spatial tasks before and after the course. Students’ pre- and post-test scores showed that the robotics course led to significant increases in perspective-taking spatial orientation task performance of sixth-grade students [t(24) = −2.16, p = 0.0356], and seventh-grade students [t(23) = −2.04, p = 0.0471]. Other spatial tests, such as the card rotation test and the paper folding test, as well as the mechanical reasoning skills test, yielded promising, close to significant results in terms of improvement from pre- to post-test. Despite the small sample size, this study shows how a STEM course that is integrative in its nature has the potential to develop both spatial skills and STEM-related reasoning skills among students.
3.3.2. Engaging students in spatial thinking through technological literacy
Technology, as Kelley and Knowles phrased, is not just an important tool or vehicle through which students learn about science, engineering, and mathematics, but is itself a discipline consisting of knowledge and practices involved in “designing, making, and using” of technology (p. 6). As the use of technology is ubiquitous across various disciplines, opportunities exist to spatialize technology-focused or technology-enhanced learning experiences. For example, Bhaduri et al. (2021) conducted a pilot study to teach seventh and eighth-grade students 3D modeling and 3D printing with the goal of understanding and supporting students’ spatial thinking during computer-aided designs. As students solved an authentic engineering design problem that required the making of a 3D model prosthetic for animals with disabilities, they developed technological literacy in computer-aided modeling tools as well as 3D printing technology. Meanwhile, students exercised their spatial skills such as mental rotation, perspective-changing, and forming mental models of their digital designs.
In another example, Peng and Sollervall (2014) developed a technology-supported, outdoor learning activity that engaged students in learning mathematics concepts, solving a real-world mathematical problem, and exercising their spatial orientation skills. Using a mobile application that informed students of the relative distance between students themselves and the physical markers on the field, the sixth-grade students oriented themselves in the outdoor environment, continuously estimated and calculated distances, and tested out different spatial orientation and coordination strategies in order to solve the math problem.
3.3.3. Engaging students in spatial thinking through scientific inquiry
Learning science through inquiry is essential to prepare students for scientific investigations. Existing inquiry-based educational practices have shown an effort to address students’ spatial ability development. As an illustration, during 6 months of geometry instruction that aimed to engage students in mathematical inquiry, secondary students were able to formulate questions that reflected thoughtful mathematical reasoning and spatial reasoning (Lehrer et al., 2013).
Understanding and creating scientific diagrams also demand spatial thinking (Newcombe, 2010). In secondary chemistry classrooms, Stieff (2011) investigated the use of computer-based visualization in a guided-inquiry curriculum to develop students’ competence in working with scientific representations. By exploring and visualizing the properties of different chemical substances and the dynamic chemical processes, students in the visualization-focused curriculum developed a better understanding of the content knowledge than those who received traditional lecture instruction, and also developed more competency in creating scientific representations like chemistry professionals do.
For another example, Oberle (2020) recorded sixth, seventh, and eighth-grade students’ learning through the National Geographic Geo-Inquiry Process, a curriculum that presents rich opportunities for spatial thinking such as comprehending geographic representations and creating geographic representations using data or maps. While the researcher did not explicitly focus on the gains of spatial ability among the students, the learning of geographic skills often demands multiple types of spatial thinking, such as spatial orientation, spatial visualization, and complex spatial reasoning (National Research Council, 2006). Compared to the control group who received a traditional, non-inquiry-focused curriculum, those who participated in the Geo-Inquiry class showed modest improvement in tasks such as discussing spatial patterns at different scales and elaborating on spatial patterns using maps.
3.3.4. Engaging students in spatial thinking through mathematical thinking
Cohrssen et al. (2017) and Cohrssen and Pearn (2021) incorporated project-based learning, which is another important way to practice integrated STEM (Ritz and Fan, 2015), to teach soon-to-be elementary children about spatial thinking and consequently support their mathematical thinking. Children who were facing a transition from kindergarten to elementary school were challenged to learn the route to their new school and visually represent their route through map-making. The core and complementary activities provided ample opportunities for children to actively reason about spatial orientation and spatial visualization. Moreover, children were encouraged to use directional and locational language and other symbolic representations such as gesturing and sketching to represent their thinking. Taking a qualitative lens, this study allowed researchers to dive deep into how children’s spatial thinking has been developed during these activities. For example, providing children with the vocabulary to describe positions, directions, and 2D and 3D shapes, together with prompting children’s use of such words through questions, led to fruitful spatial and mathematical conversations between children and teachers. In addition to exercising navigation skills, using spatial memory, and making spatial representations, these children were also actively using their mathematics knowledge during the project-based learning experience.
3.3.5. Designing spatial interventions and spatial practices in integrated STEM education
The studies mentioned above situated students’ development of spatial skills in the learning of multiple subject areas, resonating with the suggestion that the use of spatial skills to solve STEM problems is often context-dependent (e.g., Hegarty et al., 2007; Ormand et al., 2014; Atit et al., 2020). Whether it is using integrated STEM curricula as channels to develop students’ spatial ability or training students’ spatial skills alongside other academic and cognitive skills, integrated STEM education holds promise for developing both spatial and subject knowledge in different disciplines. Meanwhile, these studies presented students with real-world STEM problems to ensure an authentic learning experience. Such organic educational settings accommodate the pursuit of improving students’ abilities to solve spatially-complex STEM problems, which potentially leads to transfer of desirable learning outcomes in STEM disciplines (e.g., Hawes et al., 2017). It is worth mentioning that some studies fall into more than one category of the integrated STEM approach, for example, addressing both engineering design and technology literacy. This in turn reveals the nature of integrated STEM, where ideally the four approaches (engineering design, technological literacy, scientific inquiry, and mathematical thinking) will leverage and support each other.
Many of the interventions, case studies, or design research discussed above adopted a mixed-method research approach in which researchers gathered both quantitative data, such as changes in students’ spatial scores, and qualitative data, such as classroom observations and in-depth interviews. This approach allows researchers to comprehensively portray how students approached various spatial problems or the difficulties they faced when thinking spatially. The valuable pieces of information from qualitative data are often unattainable through purely quantitative data, and they might offer evidence on why and how the educational approaches lead to spatial learning outcomes.
While spatial ability studies often rely on the results of students’ spatial tests, existing spatial ability tests might not fully reflect the spatial skills required in STEM courses. For example, Julià and Antolì (2018) noted that the spatial skills practiced in their educational robotics STEM course did not align well with the tasks in the spatial ability test they employed. In addition, many widely used psychometric measures of spatial ability “do not capture all of the spatial skills required to solve STEM-specific spatial problems” (Atit et al., 2020, p. 2). An example is the penetrative thinking skill, which means “visualizing spatial relations inside an object” (p. 147) and is critical in geology, where students need to understand, for example, the microstructures in minerals (Ormand et al., 2014). However, this skill is fundamentally different from some of the commonly assessed spatial skills, such as visualization or mental rotation (Ormand et al., 2014). Employing a suite of multiple psychometric measures may be one solution (Ormand et al., 2014; Schneider and McGrew, 2018). On the other hand, developing more comprehensive spatial ability measures is urgently needed (Schneider and McGrew, 2018). Future research implementing spatial intervention and spatialized educational practices through integrated STEM education needs to carefully consider how to evaluate students’ spatial skills development in classrooms with the context of educational needs in mind.
3.4. Spatial ability development in informal STEM education settings
3.4.1. Informal STEM education
Much STEM learning takes place outside of school (Falk and Dierking, 2010). When out-of-school-time science activities are voluntary and intentionally designed not to be a part of a school’s curriculum, they are referred to as informal STEM education. This includes “the act of delivering STEM content outside of the traditional student/teacher relationship to STEM stakeholders (students, parents, teachers, among others) in order to support and increase the understanding, awareness, and interest in STEM disciplines” (Tillinghast et al., 2020, p. 10). These informal settings provide a unique platform to reach students of all grade levels and ages, using different delivery methods (e.g., lectures, active learning, problem-based learning, workshops, camps, events), communicating different scientific areas (e.g., biology, chemistry, engineering, physics), within different settings (e.g., social media, schools, museums, cafés).
While not as often discussed as formal education, informal learning environments have been nudging students from kindergarten to 12th grade to delve deeper into STEM concepts or ideas they may or may not have experienced in their traditional school setting (Mohr-Schroeder et al., 2014). By using hands-on activities that emphasize embodied learning and creativity, informal STEM learning programs have been shown to increase students’ confidence and interest in STEM topics, especially for students from underrepresented backgrounds or with limited access to STEM resources (Boone et al., 2020). Extra-curricular STEM involvement has been shown to predict sixth to 12th grade girls’ interest and confidence in mathematics (Heaverlo, 2011). Moreover, informal STEM learning experience was one of the predictors of secondary students’ interest in STEM careers (Stocklmayer et al., 2010; Halim et al., 2021).
Overall, informal STEM learning is an important way to provide students with authentic experiences that can increase their interest in STEM (Stocklmayer et al., 2010; Mohr-Schroeder et al., 2014; Roberts et al., 2018) and in STEM careers (Dabney et al., 2012; Kitchen et al., 2018). Considering a fair amount of research has been focusing on the role of informal STEM education in attracting students into STEM careers by enhancing their self-efficacy (Tracey, 2010; Lent et al., 2018), STEM motivation (Suter, 2016; Vennix et al., 2018), and STEM interest (Heaverlo, 2011; Dabney et al., 2013), it is worth investigating if and how informal STEM education settings can also be a tool to address another important factor that predicts STEM success: spatial ability (Shea et al., 2001; Sorby, 2009).
3.4.2. Opportunities to develop students’ spatial ability through informal STEM education
Many focus areas inside STEM domains, including teaching methods, assessment tools, curriculum developments, and informal learning programs, can be identified as promoting STEM understanding. Informal STEM learning, in particular, remains one of the primary methods to promote STEM disciplines. By using various delivery methods to reach different age groups in diverse settings, informal STEM learning leads students to integrate knowledge, concepts, and methodologies from different fields in order to achieve specific goals. This integration is often difficult to achieve within traditional pedagogy (Tillinghast et al., 2020). Implicit, or indirect, spatial training occurs when spatial training becomes a part of the normal learning activities such as math-related tasks within a math lesson (Uttal et al., 2013b; Maquet et al., 2022). Such implicit training can also be adopted in informal STEM education settings. Moreover, activities in informal STEM education programs often involve hands-on, project-based learning, and have the potential to give a spatial dimension to STEM content (Newcombe et al., 2013).
A middle school summer camp for engineering education explored the role of spatial thinking in engineering learning (Ramey and Uttal, 2017). This camp consisted of a mixture of lectures and hands-on engineering activities. The researchers found that both construction kit activities – a kit containing written/diagrammatic instructions and building materials, asking the participants to follow the instructions to build a specific device – and engineering design activities – a design challenge with specific guidelines and material constraints that allowed them to take multiple creative pathways to a solution – played important and complementary roles in eliciting engineering-relevant spatial skills. The researchers also identified a series of students’ actions during the engineering, design, or making activities that reflected their cognitive spatial processes, which they defined as spatial sensemaking activities. These include, for example, gesturing to represent “a dynamic spatial arrangement/process,” “drawing out ideas for the purpose of design,” and “discussing shape, orientation, position, or movement of objects, groups of objects, or representation” (p. 289–290). Importantly, the summer camp environment allowed the researchers to unpack how different kinds of cognitive spatial processes – typically identified in the lab or through psychometric assessments – might look like in everyday learning contexts.
In a set of technology-enhanced, STEAM-making (STEM + Arts) activities, Ramey et al. (2020) examined how spatial reasoning contributed to fifth and sixth-graders’ learning. These activities integrated concepts from STEAM disciplines, such as math or science, into making and tinkering activities, rather than traditional lectures or reading materials. Moreover, they were suitable for both in-school and out-of-school learning (Stevens et al., 2016). Ramey and colleagues found that hands-on, collaborative problem-solving with spatial tools and representations improved students’ spatial reasoning, and that different types of spatial reasoning were used frequently. While previous studies to improve students’ spatial ability have typically taken place in psychology laboratories or instructional courses (Uttal et al., 2013c), these interventions often used spatial representations that do not reflect the ones students encounter when solving STEM problems in their daily lives (Ramey et al., 2020). Therefore, incorporating spatial training in informal STEM education settings can provide students with a more authentic experience of the spatial representations they will encounter in real-world scenarios.
3.4.3. Using knowledge from existing spatial interventions to develop informal STEM education activities
Past research joined spatial ability dimensions and informal STEM education programs by challenging students with spatially complex STEM activities (e.g., Samaroo et al., 2018) or by understanding students’ cognitive spatial processes involved in these activities (e.g., Ramey and Uttal, 2017; Ramey et al., 2020). A window of opportunity is open to use the knowledge gained from past spatial ability research alongside informal STEM education to advance this field of study. By joining spatial ability studies and informal STEM education, we may come closer to filling some gaps in research. For instance, we could investigate whether students’ spatial ability levels affect their enjoyment or engagement in informal STEM education programs, or whether their spatial ability levels mediate the positive effect of informal STEM education programs on their self-efficacy.
To the best of our knowledge, existing studies that involve spatial activities within informal STEM education activities, have not collected spatial reasoning data or looked specifically into how spatial reasoning occurs during these activities. Instead, researchers have utilized the spatial dimension by engaging students in spatially complex activities and examining the impact of these activities on raising awareness of STEM careers. For example, in one informal STEM learning activity (Samaroo et al., 2018), the students from sixth to eighth grades were required to (1) model the engineering design process by making blueprints of their cities; and (2) create replica models showing a block of their cities using all recyclable materials. Through visualizing and sketching their designs, as well as transferring the design from paper to three-dimensional representation, the students made a connection from 2D to 3D which promotes spatial thinking. It is worth discussing how future research might integrate spatial ability research and informal STEM education research using established frameworks, such as the two-by-two spatial skills framework (Uttal et al., 2013a), or the lists of spatial sensemaking activities (Ramey and Uttal, 2017), to understand the cognitive spatial processes experienced by students.
Future informal STEM education programs might also make use of a wide range of activities that have been shown to support individuals’ spatial ability development. Toys that provide construction play (such as Legos and blocks) have been shown to engage children in thinking spatially (Casey and Bobb, 2003; Sorby, 2009; Verdine et al., 2014). Origami-based instruction helped students understand concepts that had previously been difficult for them, such as angle, geometric shapes, area, and fractions (Cakmak et al., 2014). Technology play can also promote students’ spatial skills development (Newcombe, 2010; Uttal et al., 2013c). For example, playing spatially challenging video games led to increased visual–spatial attention (Newcombe et al., 2013). Furthermore, Minecraft, which provides an open digital world that allows players freedom in the way of playing and building (Canossa et al., 2013), can be used as an instructional tool for supporting spatial skill development, with gains similar to the use of other 3D applications such as Google SketchUp and augmented reality (AR) (Carbonell-Carrera et al., 2021). The wide range of learning activities and experiences offered by informal STEM education allows it to engage a diverse range of audiences with spatial activities and training, helping them to develop familiarity and proficiency with spatial skills that are critical in STEM learning.
4. The moderating effects of gender and socioeconomic status
Aside from the various interventional or educational approaches to developing students’ spatial abilities we have discussed, it is crucial to recognize that the development of spatial abilities occurs at the individual level. Individual variables, including gender and socioeconomic status, may moderate the impact of spatial interventions or spatialized educational practices on individual students. To situate students’ learning of spatial skills in authentic educational contexts and to ensure that students from all backgrounds are equipped with the spatial skills vital for STEM learning, it is important to investigate whether certain student populations may be in stronger need of resources. In the following two sections, we first discuss the relationship between spatial ability and gender and how learning can be structured to reduce gender differences in spatial ability. We then discuss how socioeconomic status can impact students’ acquisition and application of spatial skills, and how the development of future spatial interventions and spatial practices can benefit from taking these factors into consideration.
4.1. Gender differences in spatial tests, interventions, and practices
In many countries, participation in STEM fields is still facing the elephant in the room: a significant gender gap between males and females. Data collected by PISA (Programme for International Student Assessment) in 2015 shed some light on this topic, providing two compelling facts: while male students scored higher in mathematics and science on average, female students were stronger in reading; moreover, male students displayed more confidence and interest in learning science (Stoet and Geary, 2018), while female students showed less intention to choose a STEM career than male students. The underrepresentation of women in the STEM field implies that women have less promising career prospects in this field (Ruthsatz et al., 2012). When it comes to scientific and technological development, the industry is suffering from a dearth of female talent, viewpoints, and experiences.
Given the significance of science and technology in our society, it is necessary to address the possible causes of this gender gap. As we discussed earlier, spatial ability is one of the key factors to success in STEM areas (Shea et al., 2001; Wai et al., 2009; Ruthsatz et al., 2012), and having high spatial ability is a predictor for choosing a STEM career (Wai et al., 2009). Nevertheless, this ability is not absent of gender differences. Several studies have pointed out sex-related differences in spatial skills (e.g., Gorska et al., 1998; Sorby and Veurink, 2010; Neuburger et al., 2015; Moon et al., 2016; Newcombe, 2020), such as mental rotation and spatial perception tasks (Newcombe, 2020).
4.1.1. Gender differences in spatial ability: Where do they come from?
Gender differences in spatial task performance have been widely studied. They usually appear from the age of puberty (Quaiser-Pohl et al., 2016), although some studies suggested that they can emerge at around the age of 10 (Neuburger et al., 2012). The origin of these differences is not yet clear. A recent meta-analysis on gender differences in spatial ability showed that although decades of research have tried to find biological causes, none could sufficiently explain gender differences in spatial ability (Bartlett and Camba, 2023). Instead, Bartlett and Camba suggested that gender roles and social norms may play a more decisive role in shaping these disparities. Traditionally speaking, many non-formal spatial activities, such as football (Voyer et al., 2000) and construction play (Sorby, 2009), are regarded as better suited for boys. This may be why boys have been more encouraged than girls to play with toys that promote spatial ability development, engage in sports, and STEM-related courses (Lippa et al., 2010; Moè, 2012). Research indicated that parents even tend to use more spatial language with boys than with girls (Newcombe, 2020). Having more exposure to spatially complex activities from a young age potentially gives male students an advantage over female students in spatial tasks. Moreover, it is a common societal belief that boys are more suited for and capable of spatially complex activities than girls. Such beliefs can negatively impact girls’ self-concept and potentially constrain their spatial skills development (Moè, 2012; Neuburger et al., 2015).
In spatial ability task performance, gender differences may be explained by the use of holistic or analytic strategies when solving spatial tasks (e.g., Kail et al., 1979; Hsi et al., 1997; Hegarty, 2018). Using holistic strategies means visualizing and manipulating the visual information as a whole, while using analytic strategies implies focusing on one part of the visual information at a time or employing verbal descriptions, which is less effective and more time-consuming (Maresch, 2014a). Although multiple strategies can be applied when solving a task (Maresch, 2014b), women tend to apply more analytic strategies, while men are more likely to apply more holistic strategies, potentially making their performance faster and more accurate (Glück et al., 2005).
Women also tend to answer more carefully than men do and hence, more slowly (Quaiser-Pohl et al., 2016). Therefore, when there is a time limit for a test, men tend to outperform women. Research indicated that if the time variable is eliminated or enough time is given, males and females performed more evenly (Moè and Pazzaglia, 2006; Voyer, 2011; Maeda and Yoon, 2015; Wang and Degol, 2017). On the other hand, some have suggested that removing the time factor or giving abundant time to solve the tests, could lead to a less accurate measurement of spatial skills, as they might be using more analytic strategies (Shepard and Metzler, 1971 Linn and Petersen, 1985).
All in all, while it is difficult to pinpoint the origin of gender differences in spatial ability, it seems that nurture, as well as the environments in which children develop, play essential roles (Newcombe, 2020). As discussed in previous sections, individuals’ spatial abilities can be improved by training (Uttal et al., 2013a). In the following section, we explore how spatial interventions have the promise to reduce gender differences in spatial task performance (Newcombe, 2010).
4.1.2. Addressing gender differences through spatial interventions
As one of the possible causes for gender differences in spatial performance is male students having more exposure to spatial activities than female students, spatial interventions can potentially support female students’ spatial ability development and confidence (Sorby et al., 2005). However, certain intervention designs may be more beneficial than others. Neuburger et al. (2012) explored how social expectations and self-concept influence fifth-grade students’ spatial task performance. In their research, they created three conditions for an intervention, instructing students that: (a) girls outperform boys in mental rotation tasks; (b) boys outperform girls in such tasks; and (c) both girls and boys are equally skilled. In the “girls better” and “no gender difference” conditions, girls’ performance on spatial tasks improved while boys’ performance worsened. Moè (2012) found that assuming that performance depends on effort instead of innate abilities improved the spatial task performance of both women and men. These results revealed that it is important for spatial intervention to address psychological factors, such as their beliefs, self-concept, and self-efficacy (Lent et al., 2010; Tracey, 2010), that may heavily affect students’ performance.
Practicing with feedback has also been found to enhance female students’ performance in spatial thinking. Feedback, defined as information provided by an agent about one’s performance or understanding (Hattie, 2008), is a powerful tool through which the learning process can be enhanced (Hattie and Timperley, 2007). However, its efficacy depends on various factors, with some related to the characteristics of the feedback, such as the person who gives the feedback, the timing, and the content, and some concerning students’ individualities, such as their motivation levels, learning goals, prior knowledge, or gender (Maier et al., 2016). In fact, previous research showed that, in general, female students benefited more than male students from practicing with feedback, resulting in more gains in spatial ability development (Narciss et al., 2014). Moreover, the reaction time of females improved after receiving feedback, as it seemed to make them feel more confident about their skills and answers (Rahe et al., 2019). Therefore, practicing with feedback might translate into a motivational strategy that reduces the gender gap by improving women’s performance in spatial tasks (Kass et al., 1998). In order to enhance female students’ spatial ability and consequently, their presence in STEM activities, it is fundamental to provide them with interventions that attend to their needs.
Lastly, creating a collaborative learning environment also shows promise in reducing the gender gap in spatial task performance (Phelps and Damon, 1989). Hoskyns-Staples and Blackmore (2020) noticed that when girls were constructing brick buildings in mixed-age groups, they were able to share their expertise and support each other with the spatial skills and mathematical knowledge needed to build and arrange the brick buildings. In addition, these girls made use of their social knowledge to ensure that the brick town reflected the facilities equipped in a real-life town. Therefore, it is worth considering adding the element of collaboration as well as using real-life problem-solving scenarios in spatial interventions, so that both girls and boys can have the opportunity to capitalize on their collaborative skills and social knowledge.
4.1.3. Developing interventions that support spatial ability development of both genders
After decades of research in spatial ability, there are still many unanswered questions, such as how gender differences impact individuals’ spatial ability at different developmental stages, and how gender may moderate the relationship between spatial ability and STEM learning. Even though the gender gap in spatial ability cannot be completely erased yet, there are several steps that can be taken in order to minimize them.
First, both girls and boys should be equally encouraged to play with spatial toys (e.g., construction bricks, tangrams, puzzles, etc.) and to engage in spatial activities (e.g., playing sports, attending science courses, etc.), as these set the path for developing spatial skills (Newcombe and Frick, 2010). Special attention should also be paid to the language used with children. This not only refers to using more spatial language but also to reassuring children of their spatial abilities. It is also worth investigating if playing materials that have been traditionally gendered can be utilized to promote girls’ spatial skills development. Crafting, sewing, and textile design, while typically regarded as feminine, actually demand a range of spatial skills (Newcombe et al., 1983; Workman and Ling Zhang, 1999). For example, E-textiles, which are fabric artifacts that require knowledge of computing and electronics (Berzowska, 2005), have been found to be especially engaging for girls (Buechley et al., 2013) and stimulated their leadership in making (Buchholz et al., 2014). Innovative technology like this may be especially valuable to challenge girls’ spatial thinking while developing their interdisciplinary knowledge and skills.
Feedback, which has been shown to positively impact female students’ performance in spatial tasks, should also be considered in future spatial ability assessments, as well as in teaching and learning in authentic environments. Further research is needed to determine when and how feedback can be provided during spatial interventions to best support both female and male students. Lastly, as Bartlett and Camba (2023) concluded, many of the traditionally-used spatial tests were built upon the criteria of “maximizing gender differences in favor of males” (p. 17). Thus, future research must be cautious in selecting approaches to measure students’ spatial ability in ways that do not reinforce the gender gap.
4.2. Socioeconomic status and its effect on students’ spatial ability
Aside from the gender gap in STEM, the underrepresentation of students from low socioeconomic status (SES), ethnic minorities, and other marginalized groups in STEM fields (National Research Council, 2011; MacPhee et al., 2013; Saw et al., 2018; Rosenthal, 2021) remains a particular concern that requires attention. Underrepresentation can restrict the pool from which skillful individuals can be selected to follow careers in STEM and negatively impact the self-efficacy in STEM learning of marginalized groups, making them particularly vulnerable to dropping out of STEM programs (Marginson et al., 2013). Rather alarmingly, students who suffer from more than one social disadvantage, such as being from an ethnic minority group and having a low SES background, face even greater challenges in developing an interest and building self-efficacy in learning STEM, as well as in entering and persisting in the STEM fields compared to those who face only one or none social disadvantage (MacPhee et al., 2013; Saw et al., 2018).
Tracking more than 500 primary school children in the United States for 2 years and assessing their spatial ability at four time points, Levine et al. (2005) found that students from the high- and middle-SES groups consistently performed better than those from the low-SES group on both the mental rotation task and the aerial-maps task. Casey et al. (2011) derived similar findings from their fourth-grade sample that children from low-income communities performed worse on spatial reasoning than children from affluent communities. Such discrepancy between the high- & middle-SES groups’ and the low-SES groups’ performance on spatial tasks has been seen as early as in preschool (Jirout and Newcombe, 2015; Bower et al., 2020). Multiple factors, such as the lack of access to spatially challenging toys or games (Levine et al., 2005; Jirout and Newcombe, 2015), lower quality of spatial play (Bower et al., 2020), and limited learning opportunities and environmental stimuli that promote spatial practices from their immediate environment (Casey et al., 2011), may have led to disparities in spatial abilities.
4.2.1. Spatial interventions and their indications for students from underprivileged backgrounds
Bower et al. (2020) carried out a five-week, high-quality, playful spatial task training that incorporated feedback, gestures, and spatial language from trainers to help children correct errors during their play. Contrary to children without training, children from low-SES backgrounds who received spatial training showed enhanced performance in 2D spatial tasks [ß = 0.28, p < 0.001]. A moderation effect was found between SES and the transfer of training effect from trained 2D spatial task to untrained 3D spatial task. Through the playful training with 2D tasks, only children from low-SES backgrounds improved in their performance of solving part of the 3D spatial tasks [ß = 0.19, p = 0.027] but not those from high-SES backgrounds. Overall, children from low-SES backgrounds benefited substantially from spatial training compared to their high-SES counterparts. As Bower and colleagues explained, spatial training might have given children from low-SES backgrounds the spatial skills and tools that are essential to solving spatial and math problems. Without spatial training, the experiences of practicing and applying these skills may not have been easily accessible to children from low-SES backgrounds compared to their high-SES counterparts.
To understand how SES can moderate spatial skills’ relationship to STEM performance, Casey et al. (2011) analyzed how students’ performance on spatial tasks relates to their performance on math tasks. They found that the association between spatial reasoning scores and math problem-solving performance among students from affluent communities was strong with a large effect size (from 0.69 to 0.83), whereas such an association was not statistically significant for students from low-income communities. This study highlighted the need for future research on educational spatial interventions to consider not only the spatial ability levels of students from low-SES backgrounds but also their abilities to utilize acquired spatial skills to solve academic or real-world problems.
Another way to address the needs of underprivileged students is to unpack the challenges they face when confronted with spatially demanding tasks. Bhaduri et al. (2021) conducted a study with 397 secondary students from rural schools, who were mostly from low-SES and ethnic-minority backgrounds, to introduce them to 3D design and 3D printing, as well as to understand the challenges in spatial thinking faced by these students when using online 3D interfaces. Using a combination of quantitative and qualitative data, such as interviews and screen recordings from the design interface, the research team was able to investigate several difficulties students experienced when building spatially complex models on the virtual 3D platform. The insights gained from this research are helpful for educators and future researchers to understand the practical and technical barriers that may hinder students’ spatial skills development. Meanwhile, the in-depth qualitative data shed light on different types of scaffolds that can be provided to underprivileged students during future 3D design processes.
4.2.2. Making spatial interventions inclusive for students from underprivileged backgrounds
While it can be difficult to conduct studies solely focused on a minority group, due to reasons such as a limited number of participants and other demographic concerns, it is necessary for future researchers to consider the potential moderating effect of socioeconomic status aside from, and along with, gender when intervening to develop students’ spatial ability.
Some researchers have been making more efforts to involve students from diverse backgrounds in their sample, such as recruiting participants from a wide range of SES backgrounds (e.g., Casey et al., 2011; Ramful et al., 2017; Lowrie and Jorgensen, 2018). This approach not only increases the representativeness of the studies but also ensures that research findings are relevant to previously understudied groups who could potentially benefit the most from these interventions. Yet, despite these efforts, there is still a dearth of studies that address the relationship between SES and spatial ability (Carr et al., 2018) and the problem of underrepresentation of socially disadvantaged students in STEM persists. Innovative practices in interventions need to be geared toward all SES groups, with a focus on developing spatial interventions to equip students with the necessary spatial skills they may not have been able to gain from their day-to-day environment (Bower et al., 2020). Meanwhile, special attention needs to be paid to the issue of intersectionality through the recruitment of groups who experience multiple social disadvantages, such as female students from low-SES backgrounds and/or ethnic minorities, as they could be the ones who benefit the most from spatial interventions. In addition, when analyzing and interpreting data from educational spatial interventions, future research needs to factor in the moderating effect of variables such as SES, ethnicity, and gender.
Various social disadvantages might interfere with students’ performance in spatial tasks as well as in STEM. From a research point of view, a mixed-method research design may help researchers gain a richer understanding of the needs of and the barriers faced by students from underprivileged groups. For example, semi-structured interviews allowed researchers to identify difficulties faced by students when working in the 3D space (Bhaduri et al., 2021). Qualitatively analyzing the robotics design work of underprivileged students from rural areas, Leonard and colleagues found that these students incorporated “elements of culture and place into game design” (2016, p. 873). The in-depth information obtained from qualitative data in addition to quantitative data allowed researchers to design future authentic learning experiences that foster an interest in STEM among underprivileged students (Leonard et al., 2016).
As we mentioned earlier, informal STEM education makes authentic and engaging learning experiences available to a wide range of audiences. With the increasing number of summer camps and afterschool programs that target minority students or students from underprivileged backgrounds (Repenning et al., 2010), informal STEM learning may also be one of the solutions to supply these students with learning and playing resources that are spatially stimulating.
5. Conclusion
One theme that stood out in our paper was the progress made, and steps to be taken, in situating spatial interventions, spatial activities, and spatialized educational practices, in authentic learning contexts.
Revisiting the relationship between spatial ability and STEM learning from a cognitive science perspective, we highlighted the need to understand the cognitive mechanisms that explain how different spatial skills are related to different aspects of STEM learning. A further question we can ask from this, similar to a point of deliberation raised by existing studies (Stull et al., 2012; Stieff et al., 2014; Atit et al., 2020), is whether excelling at spatial task performance necessarily leads to effective applications of spatial skills to solve real-world STEM problems.
Among spatial interventions that have been delivered in physical, digital, or hybrid format, as well as the increasing efforts to spatialize STEM curricula, we noticed that one tendency in the design of recent spatial interventions is to align training materials and training modes more closely with students’ daily learning experiences. Whether it is using hands-on manipulatives, providing digital feedback when students solve spatial tasks, or adapting existing student-centered pedagogies to support students’ spatial development needs, they represent the intentional recruitment of authentic, contextually-relevant teaching and learning methods.
To spotlight additional approaches to developing K-12 students’ spatial ability through authentic learning experiences, we turned to integrated STEM education and informal STEM education, which both play important roles in general STEM education but have rarely been explored by researchers in the field of spatial ability. Integrated STEM education encompasses the interdisciplinary essence of STEM and the interplay among the four elements, engineering design, technological literacy, scientific inquiry, and mathematical thinking. We suggested that spatializing more aspects of integrated STEM education would be desirable and feasible given how existing studies have made use of one or more of these elements to develop students’ spatial abilities. Despite that most of the preceding discussions have focused on formal education, informal STEM education presents a promising avenue that offers untapped resources to engage and immerse students in spatial thinking. While some of the spatial representations in existing spatial training do not necessarily reflect the types of spatial representations encountered by students when solving real-world problems (e.g., Julià and Antolì, 2018; Atit et al., 2020; Ramey et al., 2020), both integrated STEM education and informal STEM education have the potential to familiarize students with spatial representations they will see and use in authentic learning environments.
Weighing various approaches to developing students’ spatial ability, while emphasizing the importance of situating learning in authentic contexts, we propose a conjecture that the overall relationship between developing students’ spatial thinking and enhancing their abilities to solve real-world STEM problems is bidirectional and can be conveniently categorized as shown in Figure 3. It is clear that spatial ability plays a vital role in STEM learning (e.g., Sorby, 2009; Mix et al., 2021; Hawes et al., 2022), and that one of the key objectives of developing students’ spatial abilities is to increase the enrollment and performance in STEM (e.g., Newcombe, 2010; Uttal et al., 2013a; Stieff and Uttal, 2015). Meanwhile, researchers have underscored the need for spatial training that authentically addresses the spatial skills students use to solve real-world STEM problems (e.g., Ormand et al., 2014; Atit et al., 2020; Ramey et al., 2020). Therefore, we anticipate that directly using spatially complex STEM problems to target students’ spatial skills development may be a desirable option and is readily applicable in conventional classroom teaching, integrated STEM practices, as well as in informal STEM activities. Additionally, spatially complex STEM problems may have the benefit of developing students’ spatial ability along with their content knowledge and skills in multiple disciplines (e.g., Peng and Sollervall, 2014; Burte et al., 2017; Julià and Antolì, 2018). Explicitly encouraging students to use spatial skills when solving spatially demanding problems is expected to support their understanding of scientific and technological concepts and practices. For example, having secondary school students create visualizations of mechanical or chemical systems not only solidified their understanding of these systems but also developed their spatial thinking skills (Bobek and Tversky, 2016). More research is needed to further examine this interplay.
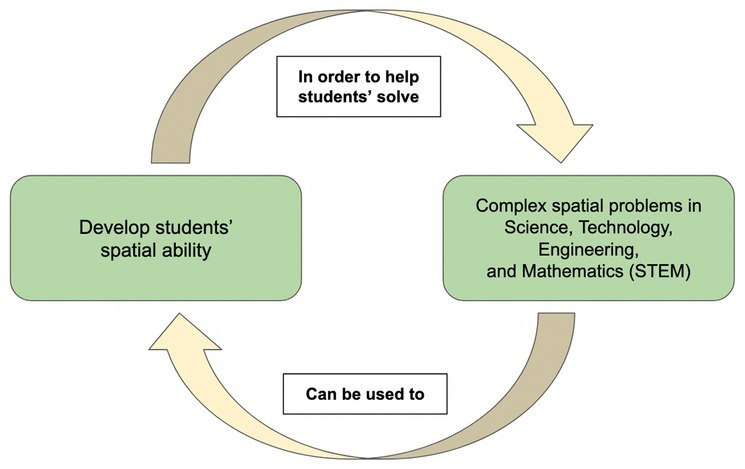
Figure 3. The conjectured relationship between developing students’ spatial ability and enhancing their abilities to solve spatially complex STEM problems.
Finally, we discussed how gender and SES may moderate the effect of spatial interventions on students’ spatial ability development. While the reasons contributing to such moderation effects are often complicated, we believe it is worthwhile for future research to take factors such as gender, SES, and the interaction between these two factors into careful consideration when planning research, drawing samples, and interpreting data. In addition, while a large number of spatial intervention studies relied on quantitative measures, mixed-method research designs and qualitative data collection methods may yield more nuanced insights into educational contexts and differential individual experiences. By obtaining a comprehensive understanding of the effect of spatial interventions, researchers can then design scaffolds that support the spatial ability development of students from underprivileged social groups or those who have been underrepresented in the STEM field. Overall, extrapolating spatial intervention findings with gender, socioeconomic status, and other potential moderating factors in mind can help translate interventions into sustainable educational practices (Casey et al., 2011; Bower et al., 2020) and make research findings more informative for both educational practitioners and policymakers.
Author contributions
CZ contributed to drafting and final editing. CL, EL, MV, and NS-C contributed equally to drafting and editing. DJ, GD, GM, MP, and RK contributed to editing, providing feedback, and proofreading. All authors contributed to the manuscript and approved it for submission.
Funding
This research is part of the SellSTEM - Spatially Enhanced Learning Linked to STEM - Marie Skłodowska-Curie Innovative Training Network to investigate the role of spatial ability in and for STEM learning. It has received funding from the European Union’s Horizon 2020 research and innovation programme under the Marie Skłodowska-Curie grant agreement No. 956124.
Conflict of interest
The authors declare that the research was conducted in the absence of any commercial or financial relationships that could be construed as a potential conflict of interest.
Publisher’s note
All claims expressed in this article are solely those of the authors and do not necessarily represent those of their affiliated organizations, or those of the publisher, the editors and the reviewers. Any product that may be evaluated in this article, or claim that may be made by its manufacturer, is not guaranteed or endorsed by the publisher.
Footnotes
1. ^Part-whole type of mental rotation means rotating an object and combining it with another element in order to produce a whole.
References
Atit, K., Gagnier, K., and Shipley, T. F. (2015). Student gestures aid penetrative thinking. J. Geosci. Educ. 63, 66–72. doi: 10.5408/14-008.1
Atit, K., Uttal, D. H., and Stieff, M. (2020). Situating space: Using a discipline-focused lens to examine spatial thinking skills. Cogn. Res. 5, 1–16. doi: 10.1186/s41235-020-00210-z
Baddeley, A. (1993). “Working memory or working attention?” in Attention: Selection, awareness and control. A tribute to Donald Broadbent. eds. A. Baddeley and L. Weiskrantz (Oxford: Oxford University Press), 152–170.
Bartlett, K. A., and Camba, J. D. (2023). Gender differences in spatial ability: A critical review. Educ. Psychol. Rev. 35, 1–29. doi: 10.1007/s10648-023-09728-2
Berzowska, J. (2005). Electronic textiles: Wearable computers, reactive fashion, and soft computation. Text 3, 58–75. doi: 10.2752/147597505778052639
Bhaduri, S., Biddy, Q. L., Bush, J., Suresh, A., and Sumner, T. (2021). 3DnST: A framework towards understanding children’s interaction with Tinkercad and enhancing spatial thinking skills. Proceedings of the Interaction Design and Children (IDC’21), Association for Computing Machinery, Athens, Greece.
Bobek, E., and Tversky, B. (2016). Creating visual explanations improves learning. Cogn. Res. 1, 27–14. doi: 10.1186/s41235-016-0031-6
Boone, A., Vanderwall, J., Klitsner, M., and Spyridakis, I. (2020). STEM outreach in underrepresented communities through the lens of play, creativity, and movement. Proceedings of the 2020 IEEE Global Humanitarian Technology Conference (GHTC), Seattle, WA, USA.
Bower, C., Zimmermann, L., Verdine, B., Toub, T. S., Islam, S., Foster, L., et al. (2020). Piecing together the role of a spatial assembly intervention in preschoolers’ spatial and mathematics learning: Influences of gesture, spatial language, and socioeconomic status. Dev. Psychol. 56, 686–698. doi: 10.1037/dev0000899
Brown, R., Brown, J., Reardon, K., and Merrill, C. (2011). Understanding STEM: Current perceptions. Technol. Eng. Teach. 70, 5–9.
Bryan, L. A., Moore, T. J., Johnson, C. C., and Roehrig, G. H. (2015). “Integrated STEM education” in STEM road map: A framework for integrated STEM education. eds. C. C. Johnson, E. E. Peters-Burton, and T. J. Moore (New York, NY: Routledge Taylor & Francis Group)
Buchholz, B., Shively, K., Peppler, K., and Wohlwend, K. (2014). Hands on, hands off: Gendered access in crafting and electronics practices. Mind Cult. Act. 21, 278–297. doi: 10.1080/10749039.2014.939762
Buckley, J., Seery, N., and Canty, D. (2018). A heuristic framework of spatial ability: A review and Synthesis of spatial factor literature to support its translation into STEM education. Educational Psychology Review 30, 947–972. doi: 10.1007/s10648-018-9432-z
Buechley, L., Peppler, K. A., Eisenberg, M., and Kafai, Y. B. (Eds.). (2013). Textile messages: Dispatches from the world of e-textiles and education. New York, NY: Peter Lang.
Burte, H., Gardony, A. L., Hutton, A., and Taylor, H. A. (2017). Think3d!: Improving mathematics learning through embodied spatial training. Cogn. Res. 2, 13–18. doi: 10.1186/s41235-017-0052-9
Cakmak, S., Isiksal, M., and Koc, Y. (2014). Investigating effect of origami-based instruction on elementary students’ spatial skills and perceptions. J. Educ. Res. 107, 59–68. doi: 10.1080/00220671.2012.753861
Canossa, A., Martinez, J. B., and Togelius, J. (2013). Give me a reason to dig Minecraft and psychology of motivation. Proceedings of the 2013 IEEE Conference on Computational Inteligence in Games (CIG), Niagara Falls, ON, Canada.
Caprile, M., Palmén, R., Sanz, P., and Dente, G. (2015). Encouraging STEM studies labour market situation and comparison of practices targeted at young people in different member states. Publications Office. 1–38. doi: 10.2861/519030
Carbonell-Carrera, C., Jaeger, A. J., Saorín, J. L., Melián, D., and de la Torre-Cantero, J. (2021). Minecraft as a block building approach for developing spatial skills. Entertain. Comput. 38:100427. doi: 10.1016/j.entcom.2021.100427
Carroll, J. B. (1993). Human cognitive abilities: a survey off actor analytic studies (Cambridge: Cambridge University Press).
Carr, M., Alexeev, N., Wang, L., Barned, N., Horan, E., and Reed, A. (2018). The development of spatial skills in elementary school students. Child Dev. 89, 446–460. doi: 10.1111/cdev.12753
Casey, B. M., Andrews, N., Schindler, H., Kersh, J. E., Samper, A., and Copley, J. (2008). The development of spatial skills through interventions involving block building activities. Cogn. Instr. 26, 269–309. doi: 10.1080/07370000802177177
Casey, B. M., and Bobb, B. (2003). Early childhood corner: The power of block building. Teach. Child. Math. 10, 98–102. doi: 10.5951/TCM.10.2.0098
Casey, B. M., Dearing, E., Vasilyeva, M., Ganley, C. M., and Tine, M. (2011). Spatial and numerical predictors of measurement performance: The moderating effects of community income and gender. J. Educ. Psychol. 103, 296–311. doi: 10.1037/A0022516
Casey, M. B., Nuttall, R. L., and Pezaris, E. (2001). Spatial-mechanical reasoning skills versus mathematics self-confidence as mediators of gender differences on mathematics subtests using cross-national gender-based items. J. Res. Math. Educ. 32, 28–57. doi: 10.2307/749620
Chatterjee, A. (2008). The neural organization of spatial thought and language. Semin. Speech Lang. 29, 226–238. doi: 10.1055/s-0028-1082886
Cheng, Y. L., and Mix, K. S. (2014). Spatial training improves children’s mathematics ability. J. Cogn. Dev. 15, 2–11. doi: 10.1080/15248372.2012.725186
Cohrssen, C., de Quadros-Wander, B., Page, J., and Klarin, S. (2017). Between the big trees: A project-based approach to investigating shape and spatial thinking in a kindergarten program. Australas. J. Early Childhood 42, 94–104. doi: 10.23965/AJEC.42.1.11
Cohrssen, C., and Pearn, C. (2021). Assessing preschool children’s maps against the first four levels of the primary curriculum: Lessons to learn. Math. Educ. Res. J. 33, 43–60. doi: 10.1007/s13394-019-00298-7
Dabney, K. P., Chakraverty, D., and Tai, R. H. (2013). The association of family influence and initial interest in science. Sci. Educ. 97, 395–409. doi: 10.1002/sce.21060
Dabney, K. P., Tai, R. H., Almarode, J. T., Miller-Friedmann, J. L., Sonnert, G., Sadler, P. M., et al. (2012). Out-of-school time science activities and their association with career interest in STEM. Int. J. Sci. Educ. Part B 2, 63–79. doi: 10.1080/21548455.2011.629455
Davies, S. R., and Horst, M. (2016). Science communication: Culture, identity and citizenship, 1st Edn. Berlin: Springer.
Delgado, A. R., and Prieto, G. (2004). Cognitive mediators and sex-related differences in mathematics. Int. Intell. 32, 25–32. doi: 10.1016/S0160-2896(03)00061-8
Dortch, D., and Patel, C. (2017). Black undergraduate women and their sense of belonging in STEM at predominantly white institutions. NASPA J. Women Higher Educ. 10, 202–215. doi: 10.1080/19407882.2017.1331854
Duffy, G., Sorby, S. A., and Bowe, B. (2020). An investigation of the role of spatial ability in representing and solving word problems among engineering students. J. Eng. Educ. 109, 424–442. doi: 10.1002/jee.20349
Falk, J. H., and Dierking, L. D. (2010). The 95 percent solution. Am. Sci. 98, 486–493. doi: 10.1511/2010.87.486
Fatourou, P., Papageorgiou, Y., and Petousi, V. (2019). Women are needed in STEM: European policies and incentives. Commun. ACM 62, 52–57. doi: 10.1145/3312565
Francis, K., Rothschuh, S., Poscente, D., and Davis, B. (2021). Malleability of spatial reasoning with short-term and long-term robotics interventions. Technol. Knowl. Learn. 27, 927–956. doi: 10.1007/s10758-021-09520-7
Freeman, B., Marginson, S., and Tytler, R. (2019). “An international view of STEM education” in STEM Education, 2.0 (Leiden, Netherlands: Brill Sense)
Geary, D. C., Hoard, M. K., Byrd-Craven, J., Nugent, L., and Numtee, C. (2007). Cognitive mechanisms underlying achievement deficits in children with mathematical learning disability. Child Dev. 78, 1343–1359. doi: 10.1111/j.1467-8624.2007.01069.x
Gilligan, K. A., Hodgkiss, A., Thomas, M. S., and Farran, E. K. (2018). The use of discrimination scalingtasks: A novel perspective on the development of spatial scaling in children. Cognitive Development. 47, 133–145. doi: 10.1016/j.cogdev.2018.04.001
Gilligan, K. A., Thomas, M. S. C., and Farran, E. K. (2020). First demonstration of effective spatial training for near transfer to spatial performance and far transfer to a range of mathematics skills at 8 years. Dev. Sci. 23:e12909. doi: 10.1111/desc.12909
Glück, J., Kaufmann, H., Dünser, A., and Steinbügl, K. (2005). Geometrie und Raumvorstellung – Psychologische Perspektiven. Informationsblätter der Geometrie (IBDG) 24, 4–11.
Gorska, R., Sorby, S. A., and Leopold, C. (1998). Gender differences in visualization skills-an international perspective. Eng. Des. Graph. J. 62, 9–18.
Gough, A. (2015). STEM policy and science education: Scientistic curriculum and sociopolitical silences. Cult. Stud. Sci. Educ. 10, 445–458. doi: 10.1007/s11422-014-9590-3
Gunderson, E. A., Ramirez, G., Beilock, S. L., and Levine, S. C. (2012). The relation between spatial skill and early number knowledge: The role of the linear number line. Dev. Psychol. 48, 1229–1241. doi: 10.1037/a0027433
Halim, L., Mohd Shahali, E. H., and Iksan, H. Z. (2021). Effect of environmental factors on students’ interest in STEM careers: The mediating role of self-efficacy. Res. Sci. Technol. Educ. doi: 10.1080/02635143.2021.2008341, [Epub ahead of print]
Hattie, J. (2008). Visible learning: A synthesis of over 800 meta-analyses relating to achievement. 1st Edn. New York: Routledge.
Hattie, J., and Timperley, H. (2007). The power of feedback. Rev. Educ. Res. 77, 81–112. doi: 10.3102/003465430298487
Hawes, Z., and Ansari, D. (2020). What explains the relationship between spatial and mathematical skills? A review of evidence from brain and behavior. Psychon. Bull. Rev. 27, 465–482. doi: 10.3758/s13423-019-01694-7
Hawes, Z. C. K., Gilligan-Lee, K. A., and Mix, K. S. (2022). Effects of spatial training on mathematics performance: A meta-analysis. Dev. Psychol. 58, 112–137. doi: 10.1037/dev0001281
Hawes, Z., Moss, J., Caswell, B., Naqvi, S., and MacKinnon, S. (2017). Enhancing children’s spatial and numerical skills through a dynamic spatial approach to early geometry instruction: Effects of a 32-week intervention. Cogn. Instr. 35, 236–264. doi: 10.1080/07370008.2017.1323902
Hawes, Z., Moss, J., Caswell, B., and Poliszczuk, D. (2015). Effects of mental rotation training on children’s spatial and mathematics performance: A randomized controlled study. Trends Neurosci. Educ. 4, 60–68. doi: 10.1016/j.tine.2015.05.001
Heaverlo, C. (2011). STEM development: A study of 6th-12th grade girls’ interest and confidence in mathematics and science. [Doctoral Dissertation]. Ames, IW: Iowa State University.
Hegarty, M. (2014). Spatial thinking in undergraduate science education. Spat. Cogn. Comput. 14, 142–167. doi: 10.1080/13875868.2014.889696
Hegarty, M. (2018). Ability and sex differences in spatial thinking: What does the mental rotation test really measure? Psychon. Bull. Rev. 25, 1212–1219. doi: 10.3758/s13423-017-1347-z
Hegarty, M., Keehner, M., Cohen, C., Montello, D. R., and Lippa, Y. (2007). “The role of spatial cognition in medicine: Applications for selecting and training professionals” in Applied spatial cognition: From research to cognitive technology. ed. G. L. Allen (Mahwah, NJ: Lawrence Erlbaum Associates Publishers), 285–315.
Herrington, J., Reeves, T. C., and Oliver, R. (2014). “Authentic learning environments” in Handbook of research on educational communications and technology. eds. J. Spector, M. Merrill, J. Elen, and M. Bishop (New York, NY: Springer)
Hinze, S. R., Williamson, V. M., Shultz, M. J., Deslongchamps, G., Williamson, K. C., and Rapp, D. N. (2014). “Spatial ability and learning from visualizations in STEM disciplines” in Space in mind: Concepts for spatial learning and education. eds. D. R. Montello, K. Grossner, and D. G. Janelle, The MIT Press 99–118.
Hodgkiss, A., Gilligan, K. A., Tolmie, A. K., Thomas, M. S., and Farran, E. K. (2018). Spatial cognition and science achievement: The contribution of intrinsic and extrinsic spatial skills from 7 to 11 years. Br. J. Educ. Psychol. 88, 675–697. doi: 10.1111/bjep.12211
Hoskyns-Staples, L., and Blackmore, K. (2020). “Collaborative problem-solving in primary mathematics: Developing shape and spatial awareness” in Social and learning relationships in primary schools. Bloomsbury monograph series eds. A. Kington and K. Blackmore (London: Bloomsbury Academic), 9–30. doi: 10.5040/9781350096097.ch-001
Hsi, S., Linn, M. C., and Bell, J. E. (1997). The role of spatial reasoning in engineering and the design of spatial instruction. J. Eng. Educ. 86, 151–158. doi: 10.1002/j.2168-9830.1997.tb00278.x
Jirout, J. J., and Newcombe, N. S. (2015). Building blocks for developing spatial skills: Evidence from alarge, representative US sample. Psychologicalscience 26, 302–310. doi: 10.1177/0956797614563338
Jones, S., and Burnett, G. (2008). Spatial ability and learning to program. Hum. Technol. 4, 47–61. doi: 10.17011/ht/urn.200804151352
Joyce, A., and Dzoga, M. (2011). Intel white paper: Science, technology, engineering and mathematics education–overcoming challenges in Europe. Brussels: European SchoolNet-Intel Educator Academy EMEA.
Julià, C., and Antolì, J. Ò. (2018). Enhancing spatial ability and mechanical reasoning through a STEM course. Int. J. Technol. Des. Educ. 28, 957–983. doi: 10.1007/S10798-017-9428-X
Kail, R., Carter, P., and Pellegrino, J. (1979). The locus of sex differences in spatial ability. Percept. Psychophys. 26, 182–186. doi: 10.3758/BF03199867
Kass, S. J., Ahlers, R. H., and Dugger, M. (1998). Eliminating gender differences through practice in an applied visual spatial task. Hum. Perform. 11, 337–349. doi: 10.1207/s15327043hup1104_3
Kearney, C. (2011). Efforts to increase students’ interest in pursuing science, technology, engineering and mathematics studies and careers. National Measures. Brussels, Belgium: European Schoolnet.
Kell, H. J., Lubinski, D., Benbow, C. P., and Steiger, J. H. (2013). Creativity and technical innovation: Spatial ability’s unique role. Psychol. Sci. 24, 1831–1836. doi: 10.1177/0956797613478615
Kelley, T. R., and Knowles, J. G. (2016). A conceptual framework for integrated STEM education. Int. J. STEM Educ. 3, 1–11. doi: 10.1186/s40594-016-0046-z
Kelley, T. R., Sung, E., Han, J., and Knowles, J. G. (2022). Impacting secondary students’ STEM knowledge through collaborative STEM teacher partnerships. Int. J. Technol. Des. Educ. 1–22. doi: 10.1007/s10798-022-09783-w
Kitchen, J. A., Sonnert, G., and Sadler, P. M. (2018). The impact of college- and university-run high school summer programs on students’ end of high school STEM career aspirations. Sci. Educ. 102, 529–547. doi: 10.1002/sce.21332
Kyttälä, M., Aunio, P., Lehto, J. E., Van Luit, J., and Hautamäki, J. (2003). Visuospatial working memory and early numeracy. Educ. Child Psychol. 20, 65–76. doi: 10.53841/bpsecp.2003.20.3.65
Kyttälä, M., and Lehto, J. E. (2008). Some factors underlying mathematical performance: The role of visuospatial working memory and non-verbal intelligence. Eur. J. Psychol. Educ. 23, 77–94. doi: 10.1007/BF03173141
Lefevre, J. A., Fast, L., Skwarchuk, S. L., Smith-Chant, B. L., Bisanz, J., Kamawar, D., et al. (2010). Pathways to mathematics: Longitudinal predictors of performance. Child Dev. 81, 1753–1767. doi: 10.1111/j.1467-8624.2010.01508.x
Lehrer, R., Kobiela, M., and Weinberg, P. J. (2013). Cultivating inquiry about space in a middle school mathematics classroom. ZDM 45, 365–376. doi: 10.1007/s11858-012-0479-x
Lent, R. W., Sheu, H.-B., Gloster, C. S., and Wilkins, G. (2010). Longitudinal test of the social cognitive model of choice in engineering students at historically black universities. J. Vocat. Behav. 76, 387–394. doi: 10.1016/j.jvb.2009.09.002
Lent, R. W., Sheu, H.-B., Miller, M. J., Cusick, M. E., Penn, L. T., and Truong, N. N. (2018). Predictors of science, technology, engineering, and mathematics choice options: A meta-analytic path analysis of the social–cognitive choice model by gender and race/ethnicity. J. Couns. Psychol. 65, 17–35. doi: 10.1037/cou0000243
Leonard, J., Buss, A., Gamboa, R., Mitchell, M., Fashola, O. S., Hubert, T., et al. (2016). Using robotics and game design to enhance children’s self-efficacy, STEM attitudes, and computational thinking skills. J. Sci. Educ. Technol. 25, 860–876. doi: 10.1007/s10956-016-9628-2
Levine, S. C., Vasilyeva, M., Lourenco, S. F., Newcombe, N. S., and Huttenlocher, J. (2005). Socioeconomic status modifies the sex difference in spatial skill. Psychol. Sci. 16, 841–845. doi: 10.1111/j.1467-9280.2005.01623.x
Lin, H. (2016). Influence of design training and spatial solution strategies on spatial ability performance. Int. J. Technol. Des. Educ. 26, 123–131. doi: 10.1007/s10798-015-9302-7
Linn, M. C., and Petersen, A. (1985). Emergence and characterization of sex differences in spatial ability: A meta-analysis. Child Dev. 56, 1479–1498. doi: 10.2307/1130467
Lippa, R. A., Collar, M. L., and Peters, M. (2010). Sex differences in mental rotation and line angle judgements are positively associated with gender equality and economic development across 53 nations. Arch. Sex. Behav. 39, 990–997. doi: 10.1007/d10508-008-9460-8
Lord, T. R. (1987). A look at spatial abilities in undergraduate women science majors. J. Res. Sci. Teach. 24, 757–767. doi: 10.1002/tea.3660240808
Lowrie, T., and Jorgensen, R. (2018). Equity and spatial reasoning: Reducing the mathematical achievement gap in gender and social disadvantage. Math. Educ. Res. J. 30, 65–75. doi: 10.1007/s13394-017-0213-7
Lowrie, T., Logan, T., and Hegarty, M. (2019). The influence of spatial visualization training on students’ spatial reasoning and mathematics performance. J. Exp. Educ. 20, 729–751. doi: 10.1080/15248372.2019.1653298
Lowrie, T., Logan, T., and Ramful, A. (2017). Visuospatial training improves elementary students’ mathematics performance. Br. J. Educ. Psychol. 87, 170–186. doi: 10.1111/bjep.12142
Lowrie, T., and Patahuddin, S. M. (2015). ELPSA as a lesson design framework. J. Math. Educ. 6, 77–92. doi: 10.22342/JME.6.2.2166.77-92
MacPhee, D., Farro, S., and Canetto, S. S. (2013). Academic self-efficacy and performance of underrepresented STEM majors: Gender, ethnic, and social class patterns. Anal. Soc. Issues Public Policy 13, 347–369. doi: 10.1111/asap.12033
Maeda, Y., and Yoon, Y. S. (2015). Are gender differences in spatial ability real or an artifact? Evaluation of measurement invariance on the revised PSVT:R. J. Psychoeduc. Assess. 34, 397–403. doi: 10.1177/0734282915609843
Maier, U., Wolf, N., and Randler, C. (2016). Effects of a computer-assisted formative assessment intervention based on multiple-tier diagnostic items and different feedback types. Comput. Educ. 95, 85–98. doi: 10.1016/j.compedu.2015.12.002
Maquet, L., Dunbar, R., Buckley, J., Seery, N., and Sorby, S. (2022). A review of the literature to inform the efficacy of a spatial skills intervention for secondary level STEM education. In the 39th pupils attitude towards technology (PATT) conference proceedings, Canada.
Maresch, G. (2014a). Spatial ability–the phases of spatial ability research. J. Geometry Graphics 17, 237–250.
Maresch, G. (2014b). Strategies for assessing spatial ability tasks. J. Geometry Graphics 18, 125–132.
Marginson, S., Tytler, R., Freeman, B., and Roberts, K., (2013). STEM: Country comparisons: International comparisons of science, technology, engineering and mathematics (STEM) education. Final Report. Australian Council of Learned Academies. Melbourne, VIC.
Margulieux, L. E. (2020). Spatial encoding strategy theory: The relationship between spatial skill and stem achievement. ACM Inroads 11, 65–75. doi: 10.1145/3381891
McGrew, K. S. (2009). CHC theory and the human cognitive abilities project: Standing on the shoulders of the giants of psychometric intelligence research. Intelligence 37, 1–10. doi: 10.1016/j.intell.2008.08.004
Mix, K. S. (2019). Why are spatial skill and mathematics related? Child Dev. Perspect. 13, 121–126. doi: 10.1111/cdep.12323
Mix, K. S., Hambrick, D. Z., Satyam, V. R., Burgoyne, A. P., and Levine, S. C. (2018). The latent structure of spatial skill: A test of the 2× 2 typology. Cognition 180, 268–278. doi: 10.1016/j.cognition.2018.07.012
Mix, K. S., Levine, S. C., Cheng, Y. L., Stockton, J. D. S., and Bower, C. (2021). Effects of spatial training on mathematics in first and sixth grade children. J. Educ. Psychol. 113, 304–314. doi: 10.1037/edu0000494
Mix, K. S., Levine, S. C., Cheng, Y. L., Young, C., Hambrick, D. Z., Ping, R., et al. (2016). Separate but correlated: The latent structure of space and mathematics across development. J. Exp. Psychol. Gen. 145, 1206–1227. doi: 10.1037/xge0000182
Moè, A. (2012). Gender difference does not mean genetic difference: Externalizing improves performance in mental rotation. Learn. Individ. Differ. 22, 20–24. doi: 10.1016/j.lindif.2011.11.001
Moè, A., and Pazzaglia, F. (2006). Following the instructions! Learn. Individ. Differ. 16, 369–377. doi: 10.1016/j.lindif.2007.01.002
Mohr-Schroeder, M. J., Jackson, C., Miller, M., Walcott, B., Little, D. L., Speler, L., et al. (2014). Developing middle school students’ interests in STEM via summer learning experiences: See blue STEM camp: STEM camp. Sch. Sci. Math. 114, 291–301. doi: 10.1111/ssm.12079
Moon, Y., Jo, H., Kim, J., and Ryu, J. (2016). Exploring gender differences in spatial orientation ability on representing cognitive map. Int. J. Psychol. Behav. Sci. 6, 91–98. doi: 10.5923/j.ijpbs.20160602.09
Murphy, M. C., Gopalan, M., Carter, E. R., Emerson, K. T., Bottoms, B. L., and Walton, G. M. (2020). A customized belonging intervention improves retention of socially disadvantaged students at a broad-access university. Sci. Adv. 6:eaba4677. doi: 10.1126/sciadv.aba4677
Mustafa, N., Ismail, Z., Tasir, Z., and Mohamad Said, M. N. H. (2016). A meta-analysis on effective strategies for integrated STEM education. Adv. Sci. Lett. 22, 4225–4228. doi: 10.1166/asl.2016.8111
Narciss, S., Sosnovsky, S., Schnaubert, L., Andrès, E., Eichelmann, A., Goguadze, G., et al. (2014). Exploring feedback and student characteristics relevant for personalizing feedback strategies. Comput. Educ. 71, 56–76. doi: 10.1016/j.compedu.2013.09.011
National Research Council (2006). Learning to think spatially. Washington, DC: The National Academies Press.
National Research Council. (2011). Successful K-12 STEM education: Identifying effective approaches in science, technology, engineering, and mathematics. Washington, DC: The National Academies Press.
Netwong, T. (2018). Development of problem solving skills by integration learning following stem education for higher education. Int. J. Inform. Educ. Technol. 8, 639–643. doi: 10.18178/IJIET.2018.8.9.1114
Neuburger, S., Jansen, P., Heil, M., and Quaiser-Pohl, C. (2012). A threat in the classroom: Gender stereotype activation and mental-rotation performance in elementary-school children. Z. Psychol. 220, 61–69. doi: 10.1027/2151-2604/a000097
Neuburger, S., Ruthsatz, V., Jansen, P., and Quaiser-Pohl, C. (2015). Can girls think spatially? Influence of implicit gender stereotype activation and rotational axis on fourth graders’ mental-rotation performance. Learn. Individ. Differ. 37, 169–175. doi: 10.1016/j.lindif.2014.09.003
Newcombe, N. S. (2010). Picture this: Increasing math and science learning by improving spatial thinking. Am. Educ. 34:29.
Newcombe, N. S. (2017). Harnessing spatial thinking to support stem learning. OECD Education Working Papers. OECD Publishing: Paris.
Newcombe, N. S. (2020). The puzzle of spatial sex differences: Current status and prerequisites to solutions. Child Dev. Perspect. 14, 251–257. doi: 10.1111/cdep.12389
Newcombe, N., Bandura, M. M., and Taylor, D. G. (1983). Sex differences in spatial ability and spatial activities. Sex Roles 9, 377–386. doi: 10.1007/BF00289672
Newcombe, N. S., and Frick, A. (2010). Early education for spatial intelligence: Why, what, and how. Mind Brain Educ. 4, 102–111. doi: 10.1111/j.1751-228X.2010.01089.x
Newcombe, N. S., and Shipley, T. F. (2014). “Thinking about spatial thinking: New typology, new assessments” in Studying visual and spatial reasoning for design creativity. ed. J. Gero (Dordrecht: Springer Netherlands)
Newcombe, N. S., Uttal, D. H., and Sauter, M. (2013). “Spatial development” in Oxford handbook of developmental psychology, Vol 1: Body and mind. ed. P. Zelazo (Oxford: Oxford University Press), 564–590.
Oberle, A. (2020). Advancing students’ abilities through the geo-inquiry process. J. Geogr. 119, 43–54. doi: 10.1080/00221341.2019.1698641
Ormand, C. J., Manduca, C., Shipley, T. F., Tikoff, B., Harwood, C. L., Atit, K., et al. (2014). Evaluating geoscience students’ spatial thinking skills in a multi-institutional classroom study. J. Geosci. Educ. 62, 146–154. doi: 10.5408/13-027.1
Palmer, S. (1978). “Fundamental aspects of cognitive representation” in Cognition and categorization. ed. E. Rosch (Hillsdale, NJ: Erlbaum), 259–303.
Peng, A., and Sollervall, H. (2014). Primary school students’ spatial orientation strategies in an outdoor learning activity supported by mobile technologies. Int. J. Educ. Math. Sci. Technol. 2, 246–256. doi: 10.18404/IJEMST.61603
Phelps, E., and Damon, W. (1989). Problem solving with equals: Peer collaboration as a context for learning mathematics and spatial concepts. J. Educ. Psychol. 81, 639–646. doi: 10.1037/0022-0663.81.4.639
Quaiser-Pohl, C., Jansen, P., Lehmann, J., and Kudielka, B. M. (2016). Is there a relationship between the performance in a chronometric mental-rotations test and salivary testosterone and estradiol levels in children aged 9-14 years?: Mental rotation and salivary hormones in (pre-)puberty. Dev. Psychobiol. 58, 120–128. doi: 10.1002/dev.21333
Rahe, M., Ruthsatz, V., Jansen, P., and Quaiser-Pohl, C. (2019). Different practice effects for males and females by psychometric and chronometric mental-rotation tests. J. Cogn. Psychol. 31, 92–103. doi: 10.1080/20445911.2018.1561702
Ramey, K. E., Stevens, R., and Uttal, D. H. (2020). In-FUSE-ing STEAM learning with spatial reasoning: Distributed spatial sensemaking in school-based making activities. J. Educ. Psychol. 112, 466–493. doi: 10.1037/edu0000422
Ramey, K. E., and Uttal, D. H. (2017). Making sense of space: Distributed spatial sensemaking in a middle school summer engineering camp. J. Learn. Sci. 26, 277–319. doi: 10.1080/10508406.2016.1277226
Ramful, A., Lowrie, T., and Logan, T. (2017). Measurement of spatial ability: Construction and validation of the spatial reasoning instrument for middle school students. J. Psychoeduc. Assess. 35, 709–727. doi: 10.1177/0734282916659207
Rasmussen, C., and Bisanz, J. (2005). Representation and working memory in early arithmetic. J. Exp. Child Psychol. 91, 137–157. doi: 10.1016/j.jecp.2005.01.004
Repenning, A., Webb, D., and Ioannidou, A. (2010). Scalable game design and the development of a checklist for getting computational thinking into public schools. In Proceedings of the 41st ACM technical symposium on Computer science education, New York, NY, United States
Reuhkala, M. (2001). Mathematical skills in ninth-graders: Relationship with visuo-spatial abilities and working memory. Educ. Psychol. 21, 387–399. doi: 10.1080/01443410120090786
Ritz, J. M., and Fan, S. C. (2015). STEM and technology education: International state-of-the-art. Int. J. Technol. Des. Educ. 25, 429–451. doi: 10.1007/s10798-014-9290-z
Roberts, T., Jackson, C., Mohr-Schroeder, M. J., Bush, S. B., Maiorca, C., Cavalcanti, M., et al. (2018). Students’ perceptions of STEM learning after participating in a summer informal learning experience. Int. J. STEM Educ. 5:35. doi: 10.1186/s40594-018-0133-4
Rocard, M., Csermely, P., Jorde, D., Lenzen, D., Walberg-Henriksson, H., and Hemmo, V. (2007). Science education NOW: A renewed education for the future of Europe. European Commission Directorate-General for Research. Publications Office.
Rosenthal, K. (2021). Is gender equality still an issue? Gender (Im) balances in STEM. Chemie Ingenieur Technik 93, 1207–1209. doi: 10.1002/cite.202000216
Ruthsatz, V., Neuburger, S., and Quaiser-Pohl, C. (2012). The social relevance and the socio-cultural origins of gender differences in spatial abilities. Acta Universitatis Lodziensis Folia Sociol 43, 17–32.
Samaroo, D., Villatoro, M., Narine, S., Iqbal, A., and Natal, K. (2018). “A multitier approach to integrating STEM education into a local elementary school” in Science Education and Civic Engagement: An International Journal. 10, 35–42.
Saw, G., Chang, C. N., and Chan, H. Y. (2018). Cross-sectional and longitudinal disparities in STEM career aspirations at the intersection of gender, race/ethnicity, and socioeconomic status. Educ. Res. 47, 525–531. doi: 10.3102/0013189X18787818
Schneider, W. J., and McGrew, K. S. (2018). “The Cattell–Horn–Carroll theory of cognitive abilities,” in Contemporary intellectual assessment: Theories, tests, andissues. eds. D. P. Flanagan and E. M. McDonough (The Guilford Press), 73–163.
Shepard, R. N., and Metzler, J. (1971). Mental rotation of three-dimensional objects. Science. Science. 171, 701–703. doi: 10.1126/science.171.3972.701
Shea, D. L., Lubinski, D., and Benbow, C. P. (2001). Importance of assessing spatial ability in intellectually talented young adolescents: A 20-year longitudinal study. J. Educ. Psychol. 93, 604–614. doi: 10.1037/0022-0663.93.3.604
Sisman, B., Kucuk, S., and Yaman, Y. (2021). The effects of robotics training on children’s spatial ability and attitude toward STEM. Int. J. Soc. Robot. 13, 379–389. doi: 10.1007/s12369-020-00646-9
Sorby, S. A. (2009). Educational research in developing 3-D spatial skills for engineering students. Int. J. Sci. Educ. 31, 459–480. doi: 10.1080/09500690802595839
Sorby, S., Drummer, T., Hungwe, K., and Charlesworth, P. (2005). “Developing 3-D spatial visualization skills for non-engineering students” in Proceedings of the 2005 American Society for Engineering Education Annual Conference & Exposition Portland, Oregon: American Society for Engineering Education. doi: 10.18260/1-2--15370
Sorby, S., and Veurink, N. (2010). Are the visualization skills of first year engineering students changing? Paper presented at 2010 Annual Conference & Exposition, Louisville, Kentucky
Sorby, S. A., and Veurink, N. (2019). Preparing for STEM: Impact of spatial visualization training on middle school math performance. J. Women Minorities Sci. Eng. 25, 1–23. doi: 10.1615/JWomenMinorScienEng.2018024516
Stevens, R., Jona, K., Penney, L., Champion, D., Ramey, K. E., Hilppö, J., et al. (2016). FUSE: An alternative infrastructure for empowering learners in schools. Proceedings of the 12th international conference of the learning sciences, Singapore.
Stieff, M. (2011). Improving representational competence using molecular simulations embedded in inquiry activities. J. Res. Sci. Teach. 48, 1137–1158. doi: 10.1002/tea.20438
Stieff, M., Dixon, B. L., Ryu, M., Kumi, B. C., and Hegarty, M. (2014). Strategy training eliminates sex differences in spatial problem solving in a stem domain. J. Educ. Psychol. 106, 390–402. doi: 10.1037/a0034823
Stieff, M., Ryu, M., Dixon, B., and Hegarty, M. (2012). The role of spatial ability and strategy preference for spatial problem solving in organic chemistry. J. Chem. Educ. 89, 854–859. doi: 10.1021/ed200071d
Stieff, M., and Uttal, D. (2015). How much can spatial training improve STEM achievement? Educ. Psychol. Rev. 27, 607–615. doi: 10.1007/s10648-015-9304-8
Stocklmayer, S. M., Rennie, L. J., and Gilbert, J. K. (2010). The roles of the formal and informal sectors in the provision of effective science education. Stud. Sci. Educ. 46, 1–44. doi: 10.1080/03057260903562284
Stoet, G., and Geary, D. C. (2018). The gender-equality paradox in science, technology, engineering, and mathematics education. Psychol. Sci. 29, 581–593. doi: 10.1177/0956797617741719
Stohlmann, M., Moore, T. J., and Roehrig, G. H. (2012). Considerations for teaching integrated STEM education. J. Pre-College Eng. Educ. Res. 2, 28–34. doi: 10.5703/1288284314653
Stull, A. T., Hegarty, M., Dixon, B., and Stieff, M. (2012). Representational translation with concrete models in organic chemistry. Cogn. Instr. 30, 404–434. doi: 10.1080/07370008.2012.719956
Suter, L. E. (2016). Outside school time: An examination of science achievement and non-cognitive characteristics of 15-year olds in several countries. Int. J. Sci. Educ. 38, 663–687. doi: 10.1080/09500693.2016.1147661
Talmy, L. (2000). Towards a cognitive semantics: Concept structuring system. Cambridge, MA: MIT Press.
Taylor, H. A., and Hutton, A. (2013). Think3d!: Training spatial thinking fundamental to STEM education. Cogn. Instr. 31, 434–455. doi: 10.1080/07370008.2013.828727
Thibaut, L., Ceuppens, S., De Loof, H., De Meester, J., Goovaerts, L., Struyf, A., et al. (2018). Integrated STEM education: A systematic review of instructional practices in secondary education. Eur. J. STEM Educ. 3:2. doi: 10.20897/ejsteme/85525
Tillinghast, R. C., Appel, D. C., Winsor, C., and Mansouri, M. (2020). STEM outreach: A literature review and definition. Proceedings of the 2020 IEEE integrated STEM education conference (ISEC), Princeton, NJ, USA.
Tillman, D. A., An, S. A., Cohen, J. D., Kjellstrom, W., and Boren, R. L. (2014). Exploring wind power: Improving mathematical thinking through digital fabrication. J. Educ. Multimedia Hypermedia. Waynesville, NCUSA: Association for the Advancement of Computing in Education (AACE). 23, 401–421.
Tracey, T. J. G. (2010). Relation of interest and self-efficacy occupational congruence and career choice certainty. J. Vocat. Behav. 76, 441–447. doi: 10.1016/j.jvb.2009.10.013
Uttal, D. H., Amaya, M., del Rosario Maita, M., Hand, L. L., Cohen, C. A., O’Doherty, K., et al. (2013b). It works both ways: Transfer difficulties between manipulatives and written subtraction solutions. Child Dev. Res. 2013, 1–13. doi: 10.1155/2013/216367
Uttal, D. H., and Cohen, C. A. (2012). Spatial thinking and STEM education. Psychol. Learn. Motiv. 57, 147–181. doi: 10.1016/B978-0-12-394293-7.00004-2
Uttal, D. H., Meadow, N. G., Tipton, E., Hand, L. L., Alden, A. R., Warren, C., et al. (2013a). The malleability of spatial skills: A meta-analysis of training studies. Psychol. Bull. 139, 352–402. doi: 10.1037/a0028446
Uttal, D. H., Miller, D. I., and Newcombe, N. S. (2013c). Exploring and enhancing spatial thinking: Links to achievement in science, technology, engineering, and mathematics? Curr. Dir. Psychol. Sci. 22, 367–373. doi: 10.1177/0963721413484756
Vennix, J., den Brok, P., and Taconis, R. (2018). Do outreach activities in secondary STEM education motivate students and improve their attitudes towards STEM? Int. J. Sci. Educ. 40, 1263–1283. doi: 10.1080/09500693.2018.1473659
Verdine, B. N., Golinkoff, R. M., Hirsh-Pasek, K., Newcombe, N. S., Filipowicz, A. T., and Chang, A. (2014). Deconstructing building blocks: Preschoolers’ spatial assembly performance relates to early mathematical skills. Child Dev. 85, 1062–1076. doi: 10.1111/cdev.12165
Voyer, D. (2011). Time limits and gender differences on paper-and-pencil tests of mental rotation: A meta-analysis. Psychon. Bull. Rev. 18, 267–277. doi: 10.3758/s13423-010-0042-0
Voyer, D., Nolan, C., and Voyer, S. (2000). The relation between experience and spatial performance in men and women. Sex Roles 43, 891–915. doi: 10.1023/a:1011041006679
Voyer, D., Voyer, S., and Bryden, M. P. (1995). Magnitude of sex differences in spatial abilities: A meta-analysis and consideration of critical variables. Psychol. Bull. 117, 250–270. doi: 10.1037/0033-2909.117.2.250
Wai, J., Lubinski, D., and Benbow, C. P. (2009). Spatial ability for STEM domains: Aligning over 50 years of cumulative psychological knowledge solidifies its importance. J. Educ. Psychol. 101, 817–835. doi: 10.1037/a0016127
Wang, M.-T., and Degol, J. L. (2017). Gender gap in science, technology, engineering and mathematics (STEM): Current knowledge, implications for practice, policy, and future directions. Educ. Psychol. Rev. 29, 119–140. doi: 10.1007/s10648-015-9355-x
Webb, R. M., Lubinski, D., and Benbow, C. P. (2007). Spatial ability: A neglected dimension in talent searches for intellectually precocious youth. J. Educ. Psychol. 99, 397–420. doi: 10.1037/0022-0663.99.2.397
Workman, J. E., and Zhang, L. (1999). Relationship of general and apparel spatial visualization ability. Cloth. Text. Res. J. 17, 169–175. doi: 10.1177/0887302X9901700401
Keywords: spatial ability, spatial intervention, mathematics education, integrated STEM education, informal STEM education, gender difference, socio-economic status
Citation: Zhu C, Leung C.O-Y., Lagoudaki E, Velho M, Segura-Caballero N, Jolles D, Duffy G, Maresch G, Pagkratidou M and Klapwijk R (2023) Fostering spatial ability development in and for authentic STEM learning. Front. Educ. 8:1138607. doi: 10.3389/feduc.2023.1138607
Edited by:
Lisbet Rønningsbakk, UiT The Arctic University of Norway, NorwayReviewed by:
Danielle Harris, University of Canberra, AustraliaClodagh Reid, Technological University of the Shannon: Midlands Midwest, Ireland
Copyright © 2023 Zhu, Leung, Lagoudaki, Velho, Segura-Caballero, Jolles, Duffy, Maresch, Pagkratidou and Klapwijk. This is an open-access article distributed under the terms of the Creative Commons Attribution License (CC BY). The use, distribution or reproduction in other forums is permitted, provided the original author(s) and the copyright owner(s) are credited and that the original publication in this journal is cited, in accordance with accepted academic practice. No use, distribution or reproduction is permitted which does not comply with these terms.
*Correspondence: Caiwei Zhu, Yy56aHUtMUB0dWRlbGZ0Lm5s