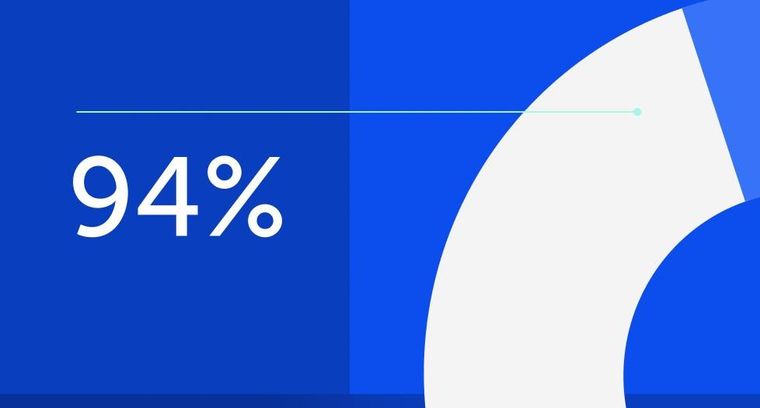
94% of researchers rate our articles as excellent or good
Learn more about the work of our research integrity team to safeguard the quality of each article we publish.
Find out more
CURRICULUM, INSTRUCTION, AND PEDAGOGY article
Front. Educ., 17 March 2023
Sec. STEM Education
Volume 8 - 2023 | https://doi.org/10.3389/feduc.2023.1110464
This article is part of the Research TopicInnovations and Technologies in Science/STEM Education: Opportunities, Challenges and Sustainable PracticesView all 13 articles
Synthetic biology is a new area of science that operates at the intersection of engineering and biology and aims to design and synthesize living organisms and systems to perform new or improved functions. Despite the important role it plays in resolving global issues, instructing synthetic biology can be challenged by a limited availability of specific educational materials and techniques for explaining complex molecular mechanisms. On the other hand, digital fabrication tools, which allow the creation of 3D objects, are increasingly used for educational purposes, and several computational structures of molecular components commonly used in synthetic biology processes are deposited in open databases. Therefore, we hypothesized that the use of computer-assisted design (CAD) and 3D printing to create biomolecular structural models through hands-on interaction, followed by reflective observation, critical and analytical thinking, could enhance students’ learning in synthetic biology. In this sense, the present work describes the design, 3D printing process, and evaluation in classrooms of the molecular models of the first synthetic biological circuit, the genetic toggle switch. The 3D printed molecular structures can be freely downloaded and used by teachers to facilitate the training of STEM students in synthetic biology. Most importantly, the results demonstrated that our resource showed a significant positive impact (p < 0.05) on students’ learning process, indicating that the proposed method helped them better understand the genetic toggle switch.
Synthetic biology comprises a series of disruptive technologies capable of providing new solutions to global challenges in health, agriculture, industry, and the environment (Cameron et al., 2014; Flores Bueso and Tangney, 2017; French, 2019). In its top-down approaches, such an area uses molecular biology tools and techniques coupled with standardized engineering principles to design and characterize biological parts, synthetic biological circuits, and systems. Thus, synthetic biology can assign new functions to organisms and redesign pre-existing biological systems to improve features of interest (Fu, 2006; Huang et al., 2010; Lv et al., 2022).
Jacob and Monod described, in the 1960s, the first genetic circuit, as they observed that inducible systems, such as the lactose operon from Escherichia coli, and repressible systems, like the tryptophan operon or lysogenic systems, respond to similar controlling elements, organized in different genetic circuits (Jacob and Monod, 1961). For example, in the lactose operon, the authors observed that the lactose catabolism was triggered only by a specific inducer molecule, structurally similar to lactose, which stimulated the coordinated synthesis of enzymes that allow lactose processing. On the other hand, when lactose is absent and glucose is present in the medium, the last sugar is preferred, due to easier processing, and the lac operon is silenced by the association of a repressor protein with the operator region of the lac promoter. The description of inducer and repressor components that could genetically control cellular machineries subsequently enabled, decades later, the development of synthetic biological circuits.
The progress made in the field of genetic engineering was also instrumental for the development of synthetic biology. In the 1970s, the discovery of restriction enzymes and the development of recombinant DNA technology allowed for the controlled manipulation and expression of DNA fragments in living organisms (Luria, 1970; Smith and Wilcox, 1970). This equipped researchers with the means to better regulate gene expression, leading to a deeper understanding of gene function. The foundations of synthetic biology are therefore closely intertwined with genetic engineering, as the latter has provided the fundamental molecular principles and DNA manipulation techniques needed to implement the former. The capability of synthetic biology to modify numerous nucleotides or gene loci across the genome sets it apart from conventional genetic engineering, which is limited to altering a limited number of nucleotides or genes typically using recombinant DNA technology (König et al., 2013). In the early 2000s, initial attempts were made to expand the frontiers of genetic engineering and create synthetic genetic circuits.
The first synthetic genetic switch assembled was based on two genes that repressed each other (Gardner et al., 2000). The application of a specific stimulus induced the expression of one of the genes, and its genetic product inhibited the expression of the other, and vice versa. One of the genes was placed in tandem with a reporter sequence encoding the green fluorescent protein (GFP), and thus the two possible states of the system were the presence or absence of green fluorescence. Due to its similarity to an electronic toggle switch, which also has two possible states—“ON” (light) or “OFF” (no light)—their system is recognized as the genetic toggle switch. Shortly after, Elowitz and Leibler developed the genetic oscillator, a circuit characterized by the interactions among three different genetic repressors, one of which also regulated GFP expression (Elowitz and Leibler, 2000). At a cellular level, the circuit caused periodic oscillations in the detection of green fluorescence, similar to what happens in electronic oscillators. Both studies strengthened the foundations of synthetic biology and demonstrated that it is possible to apply engineering principles to create synthetic biological circuits in living organisms.
Teaching synthetic biology presents unique challenges compared to other engineering fields. Electrical engineering students, for example, can easily obtain parts of electrical circuits, such as conductor wires, electronic switches, and LEDs, to learn in practice the concepts studied during theoretical classes—such as building a simple electronic toggle switch to turn on a light bulb. In contrast, synthetic biology focuses on designing and building biological circuits, which operate at the molecular scale. Consequently, it is harder for students to connect the concepts learned in class with both the actual dimension of subcellular processes and the relations between biological genetic processes and engineering. One of the main reasons for that is the scarcity of specific pedagogical methods for teaching synthetic biology (Diep et al., 2021).
To date, efforts have been made to incorporate novel resources into synthetic biology education, such as software for molecular structure simulation and visualization, virtual labs, and virtual gaming environments (Muth et al., 2021). For example, the Pymol software helps in understanding protein shapes and their relation to specific functions, and is used by molecular science instructors for visualization and computation of structures (Lineback and Jansma, 2019). Virtual labs, such as Serial Cloner, provide a whole DNA assembly virtual environment, while GelBox offers an interactive simulation tool for gel electrophoresis, both of which are essential techniques in genetic engineering and synthetic biology (Basics, 2009; Gingold and Douglas, 2018). Virtual gaming environments, such as Hero.coli, which reproduces a bacteria incorporating genetic elements to gain new properties, and Nanocrafter, which simulates the assembly of DNA fragments to create new genetic devices, help students learn basic concepts of genetic transformations in a fun manner (Barone et al., 2015; Goujet, 2018). Interactive experimental methods, like BioBits, which allows students hands-on exposure to synthetic biology experiments involving fluorescence, fragrances, and hydrogels, have also been proposed for synthetic biology education (Huang et al., 2018, 2022).
Despite these efforts, the teaching of synthetic biology still faces challenges similar to those faced in the teaching of genetic engineering and molecular sciences. One of the main difficulties lies in transforming static, two-dimensional illustrations present in scientific articles and textbooks into dynamic, three-dimensional models that truly bring the subject to life (Wu et al., 2001). Unfortunately, the fact that many students have difficulty with three-dimensional mental visualizations is often overlooked and can result in a disadvantage in their careers in STEM fields (Pittalis and Christou, 2010). In this sense, the previously mentioned educational resources still have limitations in fully demonstrating molecular processes in three dimensions, which is crucial for students’ understanding of synthetic biology.
In the early 1950s, Linus Pauling, Robert Corey, and Herman Branson, pioneers in studies of protein structures, developed the first representation of macromolecules in terms of complex space-filling models (Pauling et al., 1951; Pauling and Corey, 1951). Their system represented atoms of different chemical elements as spheres of different colors, whose diameter was proportional to their atomic radius (Koltun, 1965; Olson, 2018). In 1958, researchers reported the first experimental structure of a macromolecule, and for 20 years physical models were the principal tool for representing the structures of biological macromolecules (Kendrew et al., 1958; Olson, 2018).
Physical models fell into disuse in the 1980s, when visualization of biomolecular structures by molecular computer graphics softwares became popular. Fortunately, in recent years, several independent efforts have created physical models of proteins using new rapid prototyping technologies based on 3D printing. 3D printers enable the manufacture of macromolecule structures using digital atomic coordinates, encoded in .pdb files and available for download from the Protein Data Bank (PDB), with low waste and high accuracy and efficiency, simplifying the process of making molecular models.
Recently, about 47 peer-reviewed articles were systematically reviewed to identify different justifications for incorporating 3D printing into higher education chemistry (Pernaa and Wiedmer, 2019). These justifications include addressing challenges in chemistry learning and teaching, overcoming the high cost and lack of suitable molecular models, and the limitations of current molecular models. The majority of the articles (about 37) focused on specific chemistry concepts or laboratory instruments that could benefit from 3D printing, as well as the development of printing methodologies, safety considerations, and pedagogical models to evaluate the impact of physical models on student learning and perception.
In addition, tactile feedback has already been found to be more valuable than 3D representations alone for students struggling to understand molecular biological concepts (Salzman et al., 1999). 3D printing has been explored in recent reviews for its potential in science education, with studies reporting increased biological and chemical conceptual gains for students using 3D printed models (Pinger et al., 2019; Hansen et al., 2020). The use of three-dimensional educational materials is particularly important for visually impaired students who face substantial barriers in the classroom due to a lack of tactile methods, which are fundamental for better understanding biological concepts (Stone et al., 2020).
We hypothesize that the use of 3D printed biological structures that comprise the genetic toggle switch can enhance students’ learning of synthetic biology. By allowing them to manipulate and understand theoretical concepts in a practical way, the 3D printed parts can provide a comprehensive educational experience that captures student interest and clarifies complex molecular concepts. By using this open resource, professors would be able to show biological parts on an adequate scale, helping students better understand macromolecule spatial relationships and genetic mechanisms and their relations to electrical engineering in a way that illustrations and computational models alone cannot.
To construct our educational resource, we selected the first genetic toggle switch, which was designed, synthesized, and successfully tested by Gardner et al. (2000). First, we identified the molecular elements that compose the switch: two molecular inducers, anhydrotetracycline (aTc) and isopropyl β-D-1-thiogalactopyranoside (IPTG); two repressor gene products, the lactose operon repressor (LacI) and the Tet repressor protein (TetR); and a reporter gene product, the green fluorescent protein (GFP). As the system depends on gene expression, we also included an RNA polymerase, to allow the understanding of the control of transcriptional activity, and two generic DNA strands, each to represent the two mutually repressive genetic cassettes.
Figure 1 shows a schematic representation of the steps to follow to obtain the 3D printed molecules of the genetic toggle switch. The .stl files encoding the 3D digital atomic coordinates of the proteins were downloaded from the Protein Data Bank (PDB; http://www.pdb.org/pdb/). For the smaller molecules, the. Mol files were downloaded from the Molview database. Structures were prepared in the PyMol and ChimeraX v1.3 (https://pymol.org/2/; https://www.rbvi.ucsf.edu/chimerax) software by extracting the asymmetric units from the unitary cells, removing waters and ligands, and calculating the molecular surfaces. Afterwards, the molecular surface structures were exported as .stl files using ChimeraX, or as VRML 2 files from PyMol for repair, and hollowed out with Autodesk Meshmixer v.3.5.474 or Blender software. In Blender, each element was imported individually as X3D Extensible 3D files. The skeleton of each piece was deleted, preserving only the empty surface shells. In Meshmixer, the .stl files of the molecular surfaces were hollowed out and repaired to generate the final .stl files, for use in the UltimakerCura slicer.
Figure 1. Schematic representation of the pipeline used for production of the genetic toggle switch as a system of 3D printed pieces.
For slicing in the UltimakerCura software, we scaled the .stl molecular files into two size scales. The inducers were printed at 100% of their initial size, and the other components at 70% of scale. These scales were chosen to facilitate manipulation of the biological structures by students while maintaining approximate natural proportions. The 3D prints were made on a fused deposition (FDM) 3D printer with a 0.4 mm diameter extrusion nozzle using 1.75 mm polylactic acid plastic (PLA) filaments. In the slicing software, we set the following parameters: (1) the extrusion width was set according to the extrusion nozzle diameter; (2) the printing temperatures ranged from 200 to 215°C, (3) the printing speed ranged from 20 to 60 mm/s, and the temperature and speed parameters varied according to the individual size of each printed part. 200 g of PLA filaments were used in yellow (LacI), white (IPTG), blue (TetR), light blue (aTc), gray (DNA), green (GFP), and pink (RNA polymerase). After printing, printing supports were removed from the models by hand, and small 5 × 5 mm neodymium magnets were added approximately at the real sites of the biological interactions to allow reversible binding between the pieces representing the genetic toggle switch molecules and better represent the activation and repression behaviors of transcription.
The pedagogical concept of this study is based on Kolb’s Experiential Learning Theory (ELT), which has been discussed as being built upon the works of Dewey, Lewin, and Piaget (Kolb, 1984). The ELT combines four learning styles into a four-stage learning cycle comprising (1) concrete learning, where students encounter a new experience; (2) reflective observation, where students reflect on the new experience while considering their existing knowledge; (3) abstract conceptualization, where students give rise to new ideas based on the previous considerations; and (4) active experimentation, where students experiment and apply the newly created knowledge in real situations. It is argued that students can achieve a better understanding of concepts through the challenge present in problem solving, reinforcing and enhancing learning and critical thinking; therefore, it underpins the chosen teaching method described here (Kolb, 1984; Kolb et al., 2001).
The approach proposed here has already been tested by other authors in the broader field of STEM education, in which synthetic biology is included. A number of significant findings highlight that active engagement learning strategies have already been shown to reduce the percentage of failure rates compared to regular lectures in undergraduate courses in STEM subjects, and are also associated with a statistically significant improvement in individual learning performance and an increase in average assessment scores in molecular science (Newman et al., 2018). Specifically in the context of molecular biology, the use of tactile models has been shown to increase learning gains related to the central dogma of molecular biology, DNA replication and transcription, and protein folding, for example (Beltramini et al., 2006; Davenport et al., 2017; Gordy et al., 2020).
Our approach focused on assessing whether 3D printed molecules could enhance students’ understanding of synthetic biology, specifically around the first synthetic biological circuit, the genetic toggle switch. Thus, the learning environment was the classrooms of the University of Brasília, and our participants were undergraduate students majoring in Biotechnology and Biological Sciences. More specifically, the participants were students who were already at least halfway through their respective courses and who were enrolled in one of the Genetic Engineering, Genetics or one of the two Molecular Biology classes (A and B) offered that semester. Besides that, the only prerequisite for participation was that the students had to have attended a class in which they studied the lactose operon, since the genetic regulation of this operon was the foundation for the development of the first synthetic genetic circuit. The number of students in each of the four classes evaluated was 21, 23, 8, and 33 respectively, resulting in a total of 85 students.
Figure 2 describes a pipeline of the learning environment, the steps involving the application of the Pretests and Posttests, and the relations of the educational resource proposed in this study to the ELT, which underlies the chosen teaching method.
Figure 2. Pipeline describing the learning environment. First, the responsible professors made clear that the study was conducted according to the ethical standards set by the University of Brasilia, Brazil. Then, students were randomly numbered and this first part of the class lasted 45 min (pretest). Using the traditional lecture method, the molecular components that compose the toggle switch were introduced, followed by an explanation of how these components control gene expression of two repressors proteins and the fluorescent reporter protein setting the ON and OFF cellular state. The students responded to the Pretest-Posttest questionnaire at the last 15 min of the first part of the class. In the second part of the class (posttest), which also lasted 45 min, the participants were divided into two groups. One group (control group) received the same traditional explanation and responded to the Pretest-Posttest Questionnaire in 15 min. The other group (experimental group) received the 3D printed biological structures that compose the toggle switch and were asked to manipulate the models to understand and explain to each other how the toggle switch functions to set the ON and OFF cellular state according to Kolb’s Experiential Learning Theory. The control group and experimental group students are represented by red and blue colors, respectively, in the figure. They responded to the Pretest-Posttest Questionnaire in 15 min. The data were analyzed, right answers scored 1 and wrong answers 0, and submitted to a non-parametric Mann–Whitney test.
Our main objective was to develop a new educational resource for synthetic biology that would improve student learning. To accomplish this goal, the coordinating professors of the study, with the assistance of undergraduate and graduate students serving as teaching assistants, consistently implemented the same teaching strategy and procedures across all tested classes. All classes lasted for 2 h. During the first 45 min, the professors in charge explained that the study was being conducted according to the ethical standards set by the University of Brasília, and they were assured that their participation would be private, confidential, and voluntary. They were also informed that their identity would remain anonymous and that the data collected would be used exclusively for academic purposes, and treated with the utmost confidentiality. The participants signed an agreement form acknowledging their participation and acceptance of the conditions outlined in the research methodological process. Next, the professors in charge, using the traditional lecture method based on the whiteboard and slide decks, introduced all the molecules and genetic parts involved in the genetic toggle switch, and explained how they operated. Participants were randomly numbered and asked to answer a Pretest questionnaire using the Pretest-Posttest questionnaire (Supplementary material). They were given 15 min to perform this task, synchronously. The questionnaire was composed of multiple choice and true and false questions.
Afterward, the participants were divided into two groups (control and experimental). The second part of the class lasted 45 min for both groups (Posttest). The students who were randomly assigned as even numbers composed the control group, and were asked to move to a different classroom and were given the same traditional explanation. They were then asked to respond to the Pretest-Posttest questionnaire, also in 15 min.
For the remaining students, who were assigned as odd numbers and who comprised the experimental group, the professors in charge reproduced the following steps in front of the class with the 3D printed molecules:
1. Introduce again all the molecules and genetic parts involved in the synthetic genetic circuit, describing that IPTG and aTc are the inducers and LacI and TetR are the repressor proteins for pTrc-2 and PLtetO-1, respectively. As promoters, PLtetO-1 and pTrc-2 control the expression of LacI and TetR together with GFP, respectively, in the two different expression cassettes.
2. Simulate how RNA polymerase transcribes both of the cassettes. In the first case, the magnetic connection of the RNA polymerase with the pTrc-2 promoter region of a DNA strand causes the expression of TetR and GFP. In the second case, the transcription leads to the expression of LacI. These final products were not be visible to the class until transcription has been simulated. However, the translation process omitted in our system was highlighted as the step responsible for converting the information contained in the expressed mRNAs into individual proteins.
3. Show how the addition of the two repressors, TetR and LacI, blocks RNA polymerase activity at each of the two promoters in the two expression cassettes. Repeat the last step, but explain that a physical attachment of LacI or TetR on the promoter region of pTrc-2 or PLtetO-1, respectively, impedes the transcription of each cassette by RNA polymerase. No transcription and consequently no translation takes place, so the class did not get to see the final product. At his point, the responsible professors stated that it is important to note that in the system, the binding of only one molecular repressor per promoter is a simplified representation of the cell’s reality; many more molecules operate to block promoter regions.
4. Explain how the two cassettes interact with each other.
a. Draw a parallel with a standard light switch that can set a simple electrical system into two possible states: the presence (ON) or absence (OFF) of light. Thus, the genetic switch can set the biological system into two possible states: the presence (ON) or absence (OFF) of green fluorescence. In this case, the responsible professors stated that it is worth noting that GFP is the product that confers this characteristic.
b. Draw a parallel between the two cassettes and show that IPTG, once added to the medium, is present inside the cell, and that the repressor product of the second cassette, LacI, has the property of binding to this molecule, using the magnets embedded in both parts. Then, the responsible professores asked the students: if LacI is not bonded to pTrc-2 due to the interaction with IPTG, what happens to the expression of the pTrc-2 cassette? As a consequence, the transcription process was shown again, which results in the synthesis of TetR and GFP.
c. Highlight the TetR produced by the first cassette. Explain that this second molecular repressor attaches to the PLtetO-1 promoter of the second cassette and blocks its expression. Consequently, LacI is no longer produced. Finally, the teacher asked: “What state does the system assume when IPTG is added to the medium?” The final answer was: “The system is in its ON state and shows a green fluorescence.”
d. Explain how the second state is reached by showing the property of the molecular repressor aTc to bind to TetR by a magnetic connection. Then the teacher asked the students: “If, after its addition to the medium, aTc interacts with TetR, what happens to the expression of the PLtetO-1 cassette?” In this case, the transcription process is restarted, leading to the synthesis of LacI, a repressor of the pTrc-2 promoter. When the pTrc-2 is blocked, there is no expression of GFP and TetR. Finally, the teacher asked: “What state does the system assume when aTc is present in the medium?” The final answer was: “The system is OFF and colorless.”
Then, the students in the experimental group were divided into small groups of two, given the 3D models, and asked to individually reproduce how biological structures interact to regulate gene expression, leading to ON and OFF states. Following this step, students were asked to reflect and reproduce to each other what they had learned, proceeding with reflective observation, analytical and critical thinking, and verifying if the concepts were indeed fully understood. Students were also encouraged to correlate what they had learned with electrical circuits, encouraging them to expand their knowledge to more complex circuits. Immediately after, they were asked to respond to the Pretest-Posttest questionnaire, also in 15 min.
The questionnaires were scored as follows: correct answers were graded with one point, and wrong answers with zero points. After scoring the Pretest and Posttest questionnaires for the four classes in both control and experimental conditions, we conducted a difficulty analysis. The difficulty scores for the Pretest and Posttest were calculated by dividing the number of correct answers by the total number of questionnaires answered in each class and condition.
The learning objective of the class was to enhance the students’ comprehension of the control of gene expression underlying the two different states of the toggle switch, ON and OFF, through manipulation of the 3D printed biological structures that compose the genetic circuit, and reflective observation, analytical and critical thinking. At the end, it is expected that the participants can apply the new ideas learned from the proposed method to understand more complex biological circuits, and in the future use this knowledge to develop projects in the realm of synthetic biology.
The proposed educational activity aimed to foster the STEM competencies expected for the 21st century, including problem-solving, critical thinking, creativity, innovation, communication, and collaboration, as identified by the UNESCO report on STEM competencies for the 21st century (UNESCO International Bureau of Education, 2019). The SynBio in 3D activity relies on the manipulation of 3D printed biological structures by students, mimicking the inductors and protein functions inside the cell. Through explaining the structures to each other in a reflective and analytical way, it is hypothesized that the students will better understand the genetic control underlying the toggle switch, and generate abstract principles that can be applied in more complex circuits. Therefore, the competencies underlying this learning activity encompass both hard and soft skills. Specifically, the core competencies would be the ability and willingness to learn, conceptual/critical thinking, teamwork and cooperation, analytical thinking, digital literacy, cultural awareness, and social responsibility, all of which are essential for preparing undergraduate students for the complex and rapidly changing STEM landscape of the future. By drawing upon the UNESCO report on STEM competencies for the 21st century, the educational activity was designed to effectively promote these competencies, equipping students with the skills and knowledge they need to succeed in their studies.
The rationale for this proposal relies on the hypothesis that students would benefit from 3D models to better understand concepts of genetic circuits. We selected the toggle switch as a starting point, not only because it was the first synthetic circuit assembled, which paved the way for further designs, but also due to its simplicity: it involves only a negative control that relies on two repressors to regulate the expression of the reporter GFP gene. Figure 3 describes all genetic parts that comprise this switch, comparing the computational models experimentally obtained and submitted online and the 3D printed pieces. By preserving the dimensions of each molecule, 3D printed molecular inducers (IPTG and aTc) were significantly smaller than 3D printed protein repressors (TetR and LacI). Also, we used filaments colored in similar tones to the products derived from each of the cassettes, so students could easily associate which system is which.
Figure 3. Visual comparison of 3D printed models that compose the genetic toggle switch in relation to their respective computational structures.
After preparation, the final genetic circuit parts were 3D printed and finalized with magnets. All files are available in the Supplementary material, on our laboratory’s website, synbiolabunb.com, and under the CC BY 4.0 license. This authorizes the work to be shared and adapted under conditions of giving credit to the original authors, not using the materials for commercial purposes, and not applying legal terms or technological measures that legally restrict others from doing anything the license permits.
Although Jacob and Monod had pointed out in the ‘60s the existence of biological regulatory circuits, it was not until the beginning of the new millennium that Gardner and collaborators reported the first design and test of a synthetic genetic circuit, the toggle switch (Jacob and Monod, 1961; Gardner et al., 2000).
Figure 4 describes the different cellular states of the genetic toggle switch dependent on its intracellular inducer content. It shows how the genetic control expression is linked to Boolean algebra function as binary variables, considering 1 when the gene is expressed and 0 when it is not. First, it explains the design of the synthetic genetic circuit to control the expression and accumulation of the reporter protein, GFP. With the IPTG addition to the medium, it diffuses within the cells and binds to the LacI protein. This way, the operator of the pTrc-2 promoter is free, allowing the flow of the RNA polymerase—a process called a transcription “current,” analogous to an electric current as a transistor-like device, named transcriptor. The result is the expression of the cassette composed of TetR and GFP. TetR binds to the promoter of the other cassette, PLtetO-1, blocking the expression of LacI, and GFP sets the “ON” state of the system, making the cells turn fluorescent green. Then, the figure illustrates how the cells can change state and turn colorless. When aTc is added to the medium, it enters the cell and binds to the TetR protein, leaving the operator of the PLtetO-1 promoter available for the RNA polymerase to transcribe. Hence, LacI is produced, and it blocks the promoter of the other cassette, pTrc-2, setting the “OFF” state of the system: that is, cells are unable to express GFP and turn colorless. In summary: IPTG causes the cells to turn fluorescent green, whereas aTc has the opposite effect, maintaining them colorless.
Figure 4. Different cellular states that compose the toggle genetic circuit in 3D printed molecules. The molecules and the genetic parts needed in the synthetic genetic circuit to turn it on its ON state are: IPTG (the inducer), LacI (repressor protein), and pTrc-2 (promoter). In its initial state, the LacI repressor is bound to the pTrc-2 promoter, blocking the RNA polymerase flow. After adding IPTG to the medium, it diffuses into the cellular environment, binds the LacI protein, releasing it from the pTrc-2 promoter, and allowing the function of the transcriptor. The cell is now in its ON state, expressing GFP and TetR. The TetR repressor protein binds to the PLtetO-1 promoter and blocks the RNA polymerase flow along the cassette. Then, now, to switch to the OFF state the molecules and the genetic parts needed will be aTc (inducer), TetR (repressor protein), and PLtetO-1 (promoter). Once the aTc is added to the medium, it diffuses into the cellular environment, binds the TetR protein, releasing it from the PLtetO-1 promoter, and allowing the function of the transcriptor. The cell is now in its OFF state, expressing LacI and thus repressing the first cassette from transcribing and traducing TetR and GFP.
The biological molecules that compose the genetic toggle switch were 3D printed and presented to undergraduate students enrolled in Genetics, Genetic Engineering, or one of the two Molecular Biology classes offered at the University of Brasilia that semester. As explained in the previous section, to determine whether the use of 3D printed molecules improved students’ comprehension of the genetic toggle switch, they were first given the Pretest-Posttest questionnaire (Supplementary material) after receiving the traditional theoretical explanation (using only a whiteboard and slide decks). This phase was referred to as the Pretest. Then, the students were separated into two groups (Posttest): one group was given the traditional explanation again (control group), while the other group reviewed the concepts with the aid of 3D printed molecules (experimental group).
After scoring the Pretest and Posttest questionnaires (Table 1), a difficulty analysis (Table 2) was conducted. As all groups had a difficulty score above 50%, the test was found to have an intermediate or moderate difficulty, allowing us to proceed with data analysis. It is important to note that in most classes, the experimental conditions had higher difficulty scores than the control conditions. Since difficulty score values are inversely related to test difficulty, these findings suggested that the use of 3D printed models was a valuable educational tool that improved students’ comprehension of the genetic toggle switch.
Table 1. Mean and standard deviation of the students’ Pretest and Posttest scores (average number of correct questions) on the four different classes evaluated in this study.
Nonetheless, given the Pretest-Posttest study design employed in this investigation, we sought to examine whether differences in learning existed between the control and experimental groups. To achieve this, we calculated the difference between the Pretest and Posttest scores for each person, and then analyzed these differences (i.e., gain score approach, sensu Gliner et al., 2003). To compare these differences, we employed a non-parametric test (Mann–Whitney test) since the data distribution was non-normal (Shapiro–Wilk test W = 0.97; p < 0.05). The gain score analysis is commonly considered a good approach for Pretest-Posttest comparisons, considering that there are no Pretest differences between groups (Gliner et al., 2003; Zientek et al., 2016).
The evaluation of the Pretest scores revealed no significant differences between students in the control and experimental groups (Mann–Whitney U = 769, d.f. = 82, p = 0.33). As a result, we were able to use gain scores to assess the efficacy of the treatment by evaluating between-groups differences. The test indicated significant differences between the two groups, with students in the experimental group achieving a median gain score that was 40% higher than that of students in the control group (Mann–Whitney U = 643, d.f. = , p = 0.03; Figure 5).
Figure 5. Comparison of gain scores obtained from the students who participated in the study (n = total number of students = 85). The gain scores were calculated as the differences between the pretest and posttest scores obtained by students within each group. The “Control” group consisted of students that were submitted to the theoretical explanation, and the “Experimental” group consisted of students that were submitted to the presentation of the 3D printed molecules. Horizontal lines indicate median values and boxes indicate 1st and 3rd quartiles. We used the non-parametric Mann–Whitney test for comparison because data distribution was not normal (see text for details).
In addition, the qualitative perception of students’ comprehension of the toggle switch was evaluated after they attended the lecture (Supplementary Figure 1). The results showed that in the experimental group, after manipulating the 3D printed molecules (phase 2), a higher number of students perceived an improvement in their understanding of the genetic toggle switch compared to the control group in both phases 1 and 2. Moreover, the subjective response to question 8 (optional) of the Pretest-Posttest questionnaire also corroborates the latter results, as most students in the experimental group reported that their comprehension of the genetic toggle switch and the gene regulation control required for its function improved by using 3D printed molecules as an educational resource in the classroom (Supplementary Figure 2).
Genetic circuits are a combinatorial network of switches that can perceive different inputs, process the information, and generate outputs. Since the beginning of the 2000s, several simple synthetic circuits have been designed, built, and tested (Elowitz and Leibler, 2000; Gardner et al., 2000). These were the cornerstone for assembling more complex ones, which led to further implications in biotechnology, medicine, agriculture, and food sectors, bringing the synthetic biology area to a pivotal importance in the 21st century (Khalil and Collins, 2010; El Karoui et al., 2019; Brooks and Alper, 2021). Therefore, it is of great interest that future researchers in the different areas of science have a thorough understanding of genetic circuits.
The traditional educational system still fosters a passive learning process in which students are stimulated to hear explanations, take notes and then take exams to prove their knowledge. However, we hypothesized that complex and emerging areas, such as synthetic biology, cannot be fully understood without a modern and practical educational approach. The process of elaborating a synthetic biology project is deeply amalgamated into digital technologies, such as apps made to design plasmids from scratch and machines that automate strain engineering. Hence, classes dedicated to synthetic biology education also need to adapt and integrate the use of technology, following up with the tendency and allowing students to usufruct from active learning methodologies.
Indeed, progress in teaching synthetic biology has resulted in the incorporation of new resources, such as virtual laboratories, software for simulating molecular structures, and interactive experimental methods (Huang et al., 2018; Lineback and Jansma, 2019; Muth et al., 2021). Additionally, 3D printed molecular structures are now shown to be also a valuable tool in improving synthetic biology education. The novel SynBio in 3D method was successfully implemented in classrooms, resulting in a positive impact on students’ learning processes (Figure 5). It helped students understand the genetic toggle switch and the relationship between gene expression regulation control and electrical circuits, turning cells “ON” and “OFF.” These results are consistent with the students’ perception that manipulating the 3D printed molecules helped improve their understanding of the subject (Supplementary Figures 1, 2). Thus, our work demonstrates the success of integrating a new type of technology into synthetic biology education by linking digital fabrication tools and molecular computational structures to create engaging content. This innovative approach has the potential to transform traditional educational practices, offering students a modern and interactive learning experience that enhances understanding of emerging areas such as synthetic biology.
In conclusion, the method showcased in this article has as a foundation the combination of digital manufacturing processes, which can be used to make (almost) anything, anywhere, with readily-available molecular computational elements (Gershenfeld, 2012). Digital fabrication is a design and production process focused on turning bits into atoms, increasingly present in academic and school settings. On the other hand, databases like PDB are free to use and reunite thousands of digital molecular components, which allows the exportation in formats compatible with 3D printers. Bridging the gap on how simple the process of connecting those two resources is a crucial step to incentivizing teachers to explore it, and also develop systems for their classes in order to help students to better understand the complex concepts underlying the synthetic biology area.
We had some technical issues during the 3D printing testing phase due to (1) .stl files were too large and did not load in the Ultimaker Cura; (2) the settings of the printer were not ideal, and the resulting pieces were low in quality. We solved these issues by reducing the size of the files in Blender and exporting it again as .stl files, optimizing the printing supports, and improving the definition of the molecule surfaces.
The original contributions presented in the study are included in the article/Supplementary material, further inquiries can be directed to the corresponding author.
The studies involving human participants were reviewed and approved by CEP/FS-UnB - Comitê de Ética em Pesquisa com Seres Humanos Faculdade de Ciências da Saúde Universidade de Brasília. The patients/participants provided their written informed consent to participate in this study.
CC conceived the study. HO performed the data collection and adapted the data to 3D printer. MC and AG set up the conditions and printed all the biological structures. CC, MC, AG, AB, SR, LS, and VR elaborated the questionnaire and applied the proposed method in the different classes. HO and EV performed the statistical analysis. HO and CC wrote the manuscript. All authors contributed to the article and approved the submitted version.
We thank our supporting agencies, DPI/DPG (Decanato de Pesquisa e Inovação e Decanato de Pós-Graduação, from the University of Brasilia, Brazil), DEX (Decanato de Extensão, from the University of Brasilia, Brazil), FAPDF (Research Support Foundation of the Federal District, Brazil), and CNPq (National Council for Scientific and Technological), Brazil.
We thank the professors of the Genetic Engineering class, Lídia Maria Pepe de Moraes and Fernando Araripe Gonçalves Torres; the professors of the Molecular Biology classes, Ildinete Silva-Pereira, Marcelo Brígido, and Juliana Franco; and the professor of the Genetics class, Márcio José Poças, for letting us present the 3D printed toggle switch in their classes.
The authors declare that the research was conducted in the absence of any commercial or financial relationships that could be construed as a potential conflict of interest.
All claims expressed in this article are solely those of the authors and do not necessarily represent those of their affiliated organizations, or those of the publisher, the editors and the reviewers. Any product that may be evaluated in this article, or claim that may be made by its manufacturer, is not guaranteed or endorsed by the publisher.
The Supplementary material for this article can be found online at: https://www.frontiersin.org/articles/10.3389/feduc.2023.1110464/full#supplementary-material
Barone, J., Bayer, C., Copley, R., Barlow, N., Burns, M., Rao, S., et al. (2015). “Nanocrafter: design and evaluation of a DNA nanotechnology game.” in Proceedings of the 10th International Conference on the Foundations of Digital Games (FDG 2015).
Basics, S. (2009). Serial Cloner. Available at: http://serialbasics.free.fr.Serial_Cloner.html.
Beltramini, L. M., Araújo, A. P., de Oliveira, T. H., Dos Santos Abel, L. D., da Silva, A. R., and Dos Santos, N. F. (2006). A new three-dimensional educational model kit for building DNA and RNA molecules: development and evaluation. Biochem. Mol. Biol. Educ. 34, 187–193. doi: 10.1002/bmb.2006.49403403187
Brooks, S. M., and Alper, H. S. (2021). Applications, challenges, and needs for employing synthetic biology beyond the lab. Nat. Commun. 12:1390. doi: 10.1038/s41467-021-21740-0
Cameron, D., Bashor, C., and Collins, J. (2014). A brief history of synthetic biology. Nat. Rev. Microbiol. 12, 381–390. doi: 10.1038/nrmicro3239
Davenport, J., Pique, M., Getzoff, E., Huntoon, J., Gardner, A., and Olson, A. (2017). A self-assisting protein folding model for teaching structural molecular biology. Structure 25, 671–678. doi: 10.1016/j.str.2017.03.001
Diep, P., Boucinha, A., Kell, B., Yeung, B. A., Chen, X., Tsyplenkov, D., et al. (2021). Advancing undergraduate synthetic biology education: insights from a Canadian iGEM student perspective. Can. J. Microbiol. 67, 749–770. doi: 10.1139/cjm-2020-0549
El Karoui, M., Hoyos-Flight, M., and Fletcher, L. (2019). Future trends in synthetic biology—a report. Front. Bioeng. Biotechnol. 7:175. doi: 10.3389/fbioe.2019.00175
Elowitz, M., and Leibler, S. (2000). A synthetic oscillatory network of transcriptional regulators. Nature 403, 335–338. doi: 10.1038/35002125
Flores Bueso, Y., and Tangney, M. (2017). Synthetic biology in the driving seat of the bioeconomy. Trends Biotechnol. 35, 373–378. doi: 10.1016/j.tibtech.2017.02.002
French, K. E. (2019). Harnessing synthetic biology for sustainable development. Nat. Sustain. 2, 250–252. doi: 10.1038/s41893-019-0270-x
Fu, P. (2006). A perspective of synthetic biology: assembling building blocks for novel functions. Biotechnol. J. 1, 690–699. doi: 10.1002/biot.200600019
Gardner, T. S., Cantor, C. R., and Collins, J. J. (2000). Construction of a genetic toggle switch in Escherichia coli. Nature 403, 339–342. doi: 10.1038/35002131
Gershenfeld, N. (2012). How to make almost anything: the digital fabrication revolution. Foreign Aff. 91, 43–57.
Gingold, C., and Douglas, S. M. (2018). Gelbox—an interactive simulation tool for gel electrophoresis. BioRxiv [Preprint]. doi: 10.1101/406132
Gliner, J. A., Morgan, G. A., and Harmon, R. J. (2003). Pretest-posttest comparison group designs: analysis and interpretation. J. Am. Acad. Child Adolesc. Psychiatry 42, 500–503. doi: 10.1097/01.CHI.0000046809.95464.BE
Gordy, C. L., Sandefur, C. I., Lacara, T., Harris, F. R., and Ramirez, M. V. (2020). Building the lac operon: a guided-inquiry activity using 3D-printed models. J. Microbiol. Biol. Educ. 21:21.1.28. doi: 10.1128/jmbe.v21i1.2091
Goujet, R. (2018). Hero. Coli: A video game empowering stealth learning of synthetic biology: A continuous analytics-driven game design approach. Doctoral dissertation. Sorbonne Paris Cité).
Hansen, A. K., Langdon, T. R., Mendrin, L. W., Peters, K., Ramos, J., and Lent, D. D. (2020). Exploring the potential of 3D-printing in biological education: a review of the literature. Integr. Comp. Biol. 60, 896–905. doi: 10.1093/icb/icaa100
Huang, A., Bryan, B., Kraves, S., Alvarez-Saavedra, E., and Stark, J. C. (2022). Implementing hands-on molecular and synthetic biology education using cell-free technology. Methods Mol. Biol. 2433, 413–432. doi: 10.1007/978-1-0716-1998-8_25
Huang, H. H., Camsund, D., Lindblad, P., and Heidorn, T. (2010). Design and characterization of molecular tools for a synthetic biology approach towards developing cyanobacterial biotechnology. Nucleic Acids Res. 38, 2577–2593. doi: 10.1093/nar/gkq164
Huang, A., Nguyen, P. Q., Stark, J. C., Takahashi, M. K., Donghia, N., Ferrante, T., et al. (2018). BioBits™ explorer: a modular synthetic biology education kit. Sci. Adv. 4:eaat5105. doi: 10.1126/sciadv.aat5105
Jacob, F., and Monod, J. (1961). Genetic regulatory mechanisms in the synthesis of proteins. J. Mol. Biol. 3, 318–356. doi: 10.1016/s0022-2836(61)80072-7
Kendrew, J. C., Bodo, G., Dintzis, H. M., Parrish, R. G., Wyckoff, H., and Phillips, D. C. (1958). A three-dimensional model of the myoglobin molecule obtained by x-ray analysis. Nature 181, 662–666. doi: 10.1038/181662a0
Khalil, A. S., and Collins, J. J. (2010). Synthetic biology: applications come of age. Nat. Rev. Genet. 11, 367–379. doi: 10.1038/nrg2775
Kolb, D. A. (1984). Experiential Learning: Experience as the Source of Learning and Development. Englewood Cliffs, NJ: Prentice-Hall, 20–38.
Kolb, D. A., Boyatzis, R. E., and Mainemelis, C. (2001). “Experiential learning theory: previous research and new directions” in Perspectives on Thinking, Learning, and Cognitive Styles. eds. R. J. Sternberg and L.-F. Zhang (Mahwah, NJ: Lawrence Erlbaum Associates Publishers), 227–247.
Koltun, W. L. (1965). Precision space-filling atomic models. Biopolymers 3, 665–679. doi: 10.1002/bip.360030606
König, H., Frank, D., Heil, R., and Coenen, C. (2013). Synthetic genomics and synthetic biology applications between hopes and concerns. Curr. Genomics 14, 11–24. doi: 10.2174/1389202911314010003
Lineback, J. E., and Jansma, A. L. (2019). PyMOL as an instructional tool to represent and manipulate the myoglobin/hemoglobin protein system. J. Chem. Educ. 96, 2540–2544. doi: 10.1021/acs.jchemed.9b00143
Luria, S. E. (1970). The recognition of DNA in bacteria. Sci. Am. 222, 88–102. doi: 10.1038/scientificamerican017088
Lv, X., Hueso-Gil, A., Bi, X., Wu, Y., Liu, Y., Liu, L., et al. (2022). New synthetic biology tools for metabolic control. Curr. Opin. Biotechnol. 76:102724. doi: 10.1016/j.copbio.2022.102724
Muth, L. T., Jenkins Sánchez, L. R., Claus, S., Salvador Lopez, J. M., and Van Bogaert, I. (2021). A toolbox for digitally enhanced teaching in synthetic biology. FEMS Microbiol. Lett. 368:fnab115. doi: 10.1093/femsle/fnab115
Newman, D. L., Stefkovich, M., Clasen, C., Franzen, M. A., and Wright, L. K. (2018). Physical models can provide superior learning opportunities beyond the benefits of active engagements. Biochem. Mol. Biol. Educ. 46, 435–444. doi: 10.1002/bmb.21159
Olson, A. J. (2018). Perspectives on structural molecular biology visualization: from past to present. J. Mol. Biol. 430, 3997–4012. doi: 10.1016/j.jmb.2018.07.009
Pauling, L., and Corey, R. B. (1951). The pleated sheet, a new layer configuration of polypeptide chains. Proc. Natl. Acad. Sci. U. S. A. 37, 251–256. doi: 10.1073/pnas.37.5.251
Pauling, L., Corey, R. B., and Bransson, H. R. (1951). The structure of proteins; two hydrogen-bonded helical configurations of the polypeptide chain. Proc. Natl. Acad. Sci. U. S. A. 37, 205–211. doi: 10.1073/pnas.37.4.205
Pernaa, J., and Wiedmer, S. (2019). A systematic review of 3D printing in chemistry education – analysis of earlier research and educational use through technological pedagogical content knowledge framework. Chem. Teach. Int. 2:20190005. doi: 10.1515/cti-2019-0005
Pinger, C. W., Geiger, M. K., and Spence, D. M. (2019). Applications of 3D-printing for improving chemistry education. J. Chem. Educ. 97, 112–117. doi: 10.1021/acs.jchemed.9b00588
Pittalis, M., and Christou, C. (2010). Types of reasoning in 3D geometry thinking and their relation with spatial ability. Educ. Stud. Math. 75, 191–212. doi: 10.1007/s10649-010-9251-8
Salzman, M. C., Dede, C., Loftin, R. B., and Chen, J. (1999). A model for understanding how virtual reality aids complex conceptual learning. Presence Teleop. Virt. 8, 293–316. doi: 10.1162/105474699566242
Smith, H. O., and Wilcox, K. W. (1970). A restriction enzyme from Hemophilus influenzae. I. Purification and general properties. J. Mol. Biol. 51, 379–391. doi: 10.1016/0022-2836(70)90149-x
Stone, B., Kay, D., Reynolds, A., and Brown, D. (2020). 3D printing and service learning: accessible open educational resources for students with visual impairment. Int. J. Teach. Learn. High. Educ. 32, 336–346.
UNESCO International Bureau of Education (2019). Exploring STEM competences for the 21st century (current and critical issues in curriculum, learning and assessment, 30). IBE/2019/WP/CD/30 REV. Geneva: IBE.
Wu, H.-K., Krajcik, J. S., and Soloway, E. (2001). Promoting understanding of chemical representations: Students' use of a visualization tool in the classroom. J. Res. Sci. Teach. 38, 821–842. doi: 10.1002/tea.1033
Keywords: synthetic biology (synbio), genetic toggle switch, 3D printing, stem education, educational resource
Citation: Oss Boll H, Leitão MC, Valle Garay A, Batista ACC, Resende SG, Silva LF, Reis VCB, Vieira EM and Coelho CM (2023) SynBio in 3D: The first synthetic genetic circuit as a 3D printed STEM educational resource. Front. Educ. 8:1110464. doi: 10.3389/feduc.2023.1110464
Received: 28 November 2022; Accepted: 03 March 2023;
Published: 17 March 2023.
Edited by:
Wang-Kin Chiu, The Hong Kong Polytechnic University, ChinaReviewed by:
Monique Meier, Technical University Dresden, GermanyCopyright © 2023 Oss Boll, Leitão, Valle Garay, Batista, Resende, Silva, Reis, Vieira and Coelho. This is an open-access article distributed under the terms of the Creative Commons Attribution License (CC BY). The use, distribution or reproduction in other forums is permitted, provided the original author(s) and the copyright owner(s) are credited and that the original publication in this journal is cited, in accordance with accepted academic practice. No use, distribution or reproduction is permitted which does not comply with these terms.
*Correspondence: Cíntia Marques Coelho, Y2ludGlhY29lbGhvbUB1bmIuYnI=
Disclaimer: All claims expressed in this article are solely those of the authors and do not necessarily represent those of their affiliated organizations, or those of the publisher, the editors and the reviewers. Any product that may be evaluated in this article or claim that may be made by its manufacturer is not guaranteed or endorsed by the publisher.
Research integrity at Frontiers
Learn more about the work of our research integrity team to safeguard the quality of each article we publish.