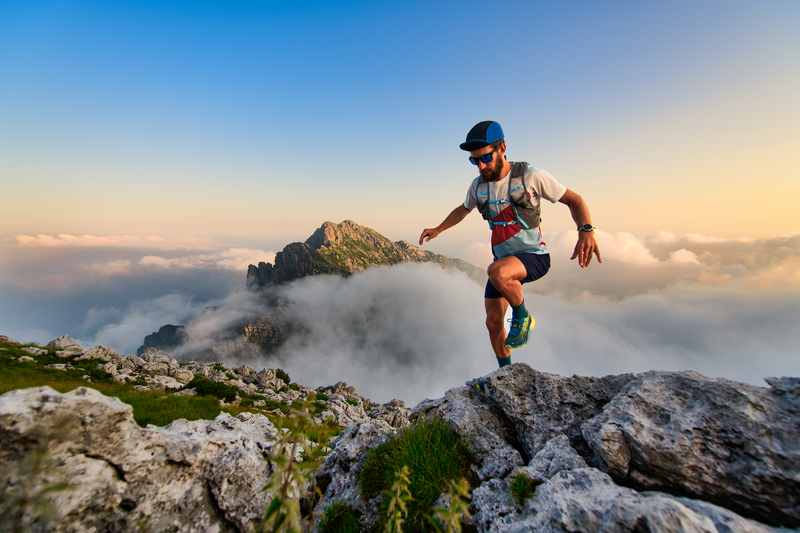
95% of researchers rate our articles as excellent or good
Learn more about the work of our research integrity team to safeguard the quality of each article we publish.
Find out more
ORIGINAL RESEARCH article
Front. Educ. , 29 December 2022
Sec. STEM Education
Volume 7 - 2022 | https://doi.org/10.3389/feduc.2022.1034140
This article is part of the Research Topic Visual Images in Science Education View all 10 articles
The aim of the study was to explore university students’ interpretations of chemical content in the form of physical constructions of atomic nuclei. Playdough was chosen as the means for expression, since it provided the students with the task of choosing the number, form, size, shape, and distance of particles. Data was collected in the form of photographs, written explanations as well as ad hoc notes. Data from 64 students was analyzed using the three levels of analysis as presented by Hedegaard and framed within the theories of models. Results show that students’ choices gave rise to 34 variations of the atomic nuclei. The analysis provided two different categories: models with close resemblance to the teaching model and models with less resemblance to the teaching model. Results show the limitations of verbal and written communication and add to the discussion concerning students’ interpretations of the multitude of atomic models used in teaching. The method was indeed a beneficial tool both for students, who could explore the composition of atomic nuclei and isotopes, and for teachers, who could connect their teaching to students’ interpretations of scientific content since the method brings a new level of detail to discussions.
The challenges facing chemistry teachers and learners are many and vary in their nature. The first challenge that a chemistry teacher may encounter is that the material world may seem self-evident to learners, so self-evident that it may in fact not even require an explanation (Tytler, 2000). The second challenge lies in what Vygotsky (2004) may have correctly stated when he theorized that “imagination is based on our experiences and creativity is a combination of experiences.” This conclusion is supported by decades of research showing the difficulty that learners face when trying to imagine the sub-microscopic world (Garnett and Hackling, 1995). Vygotsky’s conclusion suggests that for us to imagine something, we already need to have some experience of the phenomenon at hand. This is indeed a challenge for chemistry, as chemical phenomena are explained on the non-visual sub-microscopic level, a level that is difficult to provide experiences of. Our traditional way of introducing chemistry becomes the third challenge; that is, in chemistry, abstract concepts are defined using other, equally abstract, concepts in our explanations (Taber, 2019). Furthermore, the atom has been suggested to be a precursor model, i.e., fundamental for further concept formation in chemistry (Koerber and Osterhaus, 2019). Something that highlights the importance of understanding concept formation and learners’ interpretation of this specific concept. Most studies concerning the atom have focused on electrons, electron shells or orbitals (Taber, 2005; Stefani and Tsaparlis, 2009) but not atomic nuclei, their composition, repulsions, forces, weight and shape. This study was designed to fill this gap in the literature. It is also here assumed that learner’s interpretation of atomic nuclei may influence their perception of the entire atom. The present project was designed to explore a method for problem-solving that makes use of students’ expressions of the sub-microscopic level, whereby the learners are provided with as much creative self-expression as possible.
The definition of the word model used here is the one derived from the work of Acevedo-Díaz et al. (2017): A model is a simplified representation of an object, phenomena, process, idea, or system of a physical reality that is created to explain, predict, or communicate natural science. Models can then be further differentiated into scientific and educational models, where scientific models have been categorized as being composed of historic models and scientific models (Taber, 2019). Historic models are no longer used within the scientific community but are seen as important for education since they are part of our cultural history. Scientific models are the ones currently used by the scientific community, and they are also transformed into educational models, models that are adapted to be suitable for learners at different educational levels. Educational models can in turn be categorized into curricula models, expressed models, and individual models. Educational models can be defined as consensus models, meaning that scientists, textbook-writers, and other members of an educational community have agreed on the level of the simplifications made. These consensus models then become expressed models when used by the teacher in teaching (Taber, 2019). The individual then interprets the expressed models, and these become individual models (Gilbert et al., 2001). In this process of interpretation, it becomes important for teachers, who work with educational models, to be explicit concerning the nature of a model. A framework designed to help teachers work with models was described by Gilbert (2004), who suggested that the different aspects of modeling should include an understanding that a model is not a replica of the world; it is an interpretation, meaning that there can be many different models in use for the same phenomena. Models are used as tools for supporting conceptual understanding, solving problems or predicting outcomes of processes. Even if the model is perceived as an object, the model can be an interpretation of an “idea, a system, an event or a process” (Gilbert, 2004). As research progresses, new models are created leading to models becoming superseded and eventually seen as historic models. This multitude of historic models introduced to learners often as a way to introduce the cultural history of science may in fact not always be helpful, as research shows that teachers and textbooks in some cases present inaccurate hybrid models that are combinations of different historical models (Justi and Gilbert, 2000).
The actual process of how to support students’ own modeling has also been described by Justi and van Driel (2006) as beginning with initial observations of a phenomenon, where a few specific parts of the phenomenon at hand are chosen, and a model is initially produced, then later manipulated, and finally the limitations of the model discussed.
Another suggestion, more focused on analyses of the teaching models themselves, was made by Glynn and Duit (1995) who described six different steps for introducing models to students, beginning with the introduction of a target model and followed by an overview of the teaching model. These six steps are relatively similar to the steps suggested by Justi and van Driel (2006) with identification of the relevant aspects of the models, “finding similarities, mapping similarities, identification of where the analogy breaks down and drawing conclusions of the target model.” Indeed, types of systematic use of models can be done with three different kinds of intentions (van Joolingen, 2004), which are communication, analysis, or explanation: a model as a way of communication is where the intention is for students to create their own models to express their ideas; for analysis, the intention is to analyze the properties of the model itself or its usefulness in explanation, and models used for explanation involve the intention for students to recreate models to explain results or make predictions.
Research into the development of individual models is abundant and researchers have performed meta-analysis to categories the different types of individual models that have been found. One of these meta-analysis was performed by Talanquer (2006) here learner’s interpretations was categorized into the empirical assumptions; continuity, substantialism, essentialism, mechanical causality, and teleology. Assumptions that in combination with the following heuristics: association, reduction, fixation, and linear sequencing were found to be the basis of many of the individual interpretations. Another approach is to find and explore precursor models, i.e., individual models that are seen as fundamental for building further understanding of a subject area (Pantidos et al., 2022). The atom has been suggested to be one of the precursor models (Koerber and Osterhaus, 2019) something that makes research into students understanding of the atom an important field. Traditional methods using educational models for visualizing different aspects of the atom such as different types of 2 and 3-d models are commonly used where a sense of scale is provided using verbal or written communication. Today research points toward the importance of multimodal teaching approaches including body language (Pantidos et al., 2022) and better support for student using inscriptional stories as a new way to move from the everyday world to abstractions (Pantidos et al., 2022).
The type of modeling that is the focus of the present study is a model for communication or students’ own models used as a way of expression. Here, students construct a model in order to express their own ideas, which is a type of modeling used as means to gain insight into student ideas (Nersessian, 2002; Halloun, 2006) and to promote metacognition (Gilbert and Justi, 2016). This type of modeling provides an opportunity to work with a content on different levels (Gilbert, 2004): tangibly and concretely using 3-D material; verbally involving speech and writing where model discussions can use metaphors and analogies; visually with the help of drawings, diagrams, animations, illustrated stories and so on, as well as with gestures or bodily representations; and symbolically using formula equations.
Expressed models are composed of concepts and relationships that can be temporal, spatial, and causal relationships. The analysis of student-generated models can be made using appearance, literal similarity, tightness (closeness of source and the target), and with what has been described as true analogy (where the composition of the target cannot be transferred but the relationships can) (Duit, 1991).
Today many schools are culturally diverse and multilingual (Wilmes et al., 2018). A situation that has placed focus on the need to provide learners with multiple ways of accessing and expressing knowledge, especially using non-lingual modes (Roth and Lawless, 2002). Researchers have argued for an expansion of the concept of literacy to include visual (graphs and images), spatial (episodic movement), gestural (hand and body movement), auditory (sound effects and music), tactile (sensory hands on), oral (speech), and written modes (Williams and Tang, 2020). In this context science is viewed as a specialized language, something that may become an additional challenge for learners at all ages. Research shows that gestures and body language support learners both in learning (Cervetti et al., 2015; Pantidos and Givry, 2021) as it can promote moving between spoken language and thought (Bracey and Zoë, 2017), fill gaps in language (Korkmaz and Unsal, 2017), be used as pedagogical scaffolds (Santau et al., 2010), as well as communicating understanding (Siry and Martin, 2014). In the present study, student-generated models was designed to be expressed in the form of playdough creations. Combining creative expression with science is as old as science itself and can be seen in stone carvings dating back to 3,500 before christ (BC) that display lunar cycles and calculations (Steele, 2000). Research shows that creative expressions in science education have many advantages such as providing connections between different subject content (Herro and Quigley, 2016) and placing science in a real-world context (Gilbert et al., 2011). Creative expressions are also important for visual thinking, structures, interpretation of 3-dimensional aspects of phenomena, combining both processes and concepts in expression (Begoray and Stinner, 2005). They can also be a way to integrate learners’ concepts and canonical science (Scott et al., 2011) and become foundations for new pedagogies (Braund and Reiss, 2019), and a ways to express knowledge beyond written or spoken language for second language learners (Aubusson et al., 2006).
The use of teaching models cannot be avoided when teaching and learning chemistry, especially since many of the explanations in chemistry are based on a sub-microscopic level. The tools that teachers can use for providing their students with experiences on this non-visual level are the use of analogy and models; all of which by necessity are macroscopic in their nature. The words used for describing the sub-macroscopic level were not all newly invented as our understanding of this level developed. Instead, some of the words used are also macroscopic in nature and may lead to unwanted analogy formation. In the Swedish language, for example, the word shell (skal) is the word also used for different fruit peels such as an orange peel and apple peel or an eggshell. The word nuclei (kärna) is the same as the word used for kernels, which can lead many students toward fruit analogs in connection to the atom. Other analogs found in connection with the atom are that the atom is seen like a ball, or a solar system (Harrison and Treagust, 1996). This particular problem, using a macroscopic view of the sub-microscopic level, can derive from many different sources, such as, macroscopic word use, the learning process itself where children connect new experiences to previous macroscopic ones, or a lack of ways to provide experiences of the sub-microscopic world. Indeed, research shows that it is not uncommon to find students who believe that atoms can be viewed in a normal microscope or that the illustration that they see in textbooks and other media are real images of the atom (Papageorgiou et al., 2016).
When turning toward research concerning the learning process itself, it shows that young children’s learning of the concept of small (atoms and molecules) begins with the use of analogy (Adbo and Vidal Carulla, 2020), where for example pieces of crushed sugar seen through a magnifying glass are described as ice blocks. The development of a sense of scale and an understanding of the sub-microscopic world can be enhanced by zooming-in videos that visualize the transition between the macroscopic and the sub-microscopic level. A sense of scale can be provided if the zooming-in video is perceived as occurring at constant speed; the difference in perceived time between the different levels of organization then gives an understanding of scale to the extent that 5-year old children describe atoms initially as meatballs, and then recognizing that the analogy is not enough, the children conclude that these are not meatballs, they just look like meatballs; the word atom was then introduced. The sense of scale is seen through comments, such as, “They are everywhere in everything.” In summary, the concept of atom at that point in time did include shape, 3-dimensionality, and a sense of scale (Adbo and Vidal Carulla, 2020).
The aim of the present study was to move beyond language and provide students with as much creative self-expression as possible to express their own models of selected atomic nuclei. The study aimed in particular at exploring:
- What do the students’ expressed models look like when they are given the opportunity to move beyond spoken language?
- In what way can creative visualizations contribute to contemporary science education?
The participants in this study were 64 students taking the first of two chemistry courses in a university course during a so-called foundation year, which provides preparatory education of science content that is equivalent to the upper secondary level. The requirements for admission to the foundation year are a completed upper secondary level program that is preparatory for higher studies. The foundation year provides the basic natural science courses required for entrance to university level in natural science for those students who wish to obtain higher grades or add courses that were not completed in previous studies. Several of the students included in this study, therefore, had previous experience in chemistry at this level. All students included had formal chemistry at lower secondary school, students age 13–16, something that contain the structure of matter, atoms, electrons, and nuclear particles. At the beginning of the course the atom was reintroduced to the students. The teaching model for the general atom in use within this course was the one in Figure 1. This model includes protons and neutrons depicted in a way that attempts to show the three-dimensional structure of the nucleus. Electron movements are communicated as probability clouds (marked with gray color). The mass of all particles in the form of atomic mass units (u) and the charge of all particles are included in the model. There is also a small difference in size between electrons and protons, a difference that by no means is close to the perceived reality but a difference, nonetheless.
The students were asked to build a selection of atomic nuclei using play dough and choose different colors for the different sub-atomic particles included. Additional instructions were:
1. Build the following atomic nuclei: The most common form of Hydrogen, Helium, Carbon and Oxygen and take a photo of it.
2. Draw the nucleus.
3. Provide a written explanation to the built nuclei and calculate their masses in u.
The students were also provided with a periodic table that included atomic number and atomic mass.
The choice of using playdough was made since it provides ample creative freedom for working in three dimensions, without restrictions on size and shape. The students were also asked to provide a written explanation to their different nuclei, calculate the mass of each nucleus using units (u), draw their nuclei, and take a photo of each nucleus. Information that was used in the interpretation of students’ constructions. The students worked in groups of 2–4 students, but each student made his or her own nuclei. Notes of ad hoc discussions were collected while the teacher circulated in the room. The only other instruction the students were provided was to use one color for each of the particles. The fact that the playdough was colored was misleading, but an issue that could not be resolved. Each student had at least three different colors of playdough to choose from.
To meet ethical considerations, consent forms were collected from all students. All data collection and data storage are in compliance with general data protection regulation (GDPR) according to the european union (EU) standards (2016).
Data in the form of construction from playdough, written explanations and ad hoc field notes was analyzed independently by two researchers using Hedegaard’s three levels of qualitative analysis (Hedegaard, 1995) where the first level is called the commonsense interpretation. At this level of interpretation data from the 64 students were merged into, individual, consecutive series of atomic nuclei. The second level of analysis, called situated interpretation, was then applied to the data set. In this level of analysis, students written explanations and common structural and functional patterns of the models were in focus. Especially, the differences between the teaching model and the student expressed model were analyzed for literal appearance and tightness and the images was then sorted into two different categories; models with close resemblance to the teaching model and models with less resemblance to the teaching model. Representational series of images for each set of deviations were selected. The third level of analysis, called thematic and conceptual interpretation, was performed here with analytical focus on identifying general connections between the findings and the existent research literature. The analysis did not include body language or social interactions.
Due to the diversity of the student group and the number of atomic nuclei models available to students today, it is only the teaching model in use within this course that can be addressed and the conclusions regarding teaching models can only be drawn from students’ models. When comparing the teaching model to students expressed model, no difference between the different student groups could be detected, i.e., meaning that there was no difference between the students that had previously studied chemistry at upper secondary school level and those who had not.
Results are presented in the form of photos of the students’ expressed models and categorized into two major categories, one with close resemblance (Figure 2) to the teaching model and one with less resemblance to the teaching model (Figure 3). The construct of individual students’ series was maintained within the categories since it provided information of student reasoning when creating nuclei of different sizes with different types of interactions between the subatomic particles. The models were built on a single occasion, proving a cameo-shot of the diversity of student’s interpretations and considerations. The series of categorized images that were found in the dataset here referred to as: close resemblance and less resemblance, represent the different variations of playdough models, deriving from the 64 students participating in the project. Models with close resemblance are presented in Figure 2 and models with less resemblance in Figure 3. The category with close resemblance to the teaching model included models that were correct regarding written explanations, number of protons and neutrons in the playdough model, and mass was provided in a correct manner. Even though the answers were in close resemblance with the teaching model, 16 different series of nuclei were found in the assembly of the constructed model. Of the 64 students included in the study 70% of the students’ models was found in category: close resemblance. In the category: less resemblance (Figure 3) the students included an incorrect number of sub-atomic particles, ignored the size differences of the particles or added particles not relevant to atomic nuclei. A total of 30% of student models were found in this category. Results deriving from the series of atomic nuclei (H, He, C, and O) show in total 32 different variations.
Figure 2. Models with close resemblance to the teaching model regarding written explanations, a correct number of protons and neutrons in the playdough model, and mass was provided in a correct manner. Series of atomic nuclei in the order: Hydrogen (H), Helium (He), Carbon (C), and Oxygen (O). One student in each row (C1–C16) and the different versions of the nuclei are found in the columns.
Figure 3. Models with less resemblance to the teaching model. Series of atomic nuclei in the order: Hydrogen (H), Helium (He), Carbon (C), and Oxygen (O). One student in each row (L1–L16) and the different versions of the nuclei are found in the columns.
In this category the series with close resemblance toward the teaching model of the atomic nuclei concerning the number of protons, neutrons and their respective mass, show 16 different variations in the placement of protons and neutrons (Figure 2). In general, the differences between the students’ models and the teaching model depend on what specific feature (Lakoff and Johnson, 1987) the student focusing on at the time. This approach of working with one or a few features at a time shows how the students used the models as a form of visual thinking (Begoray and Stinner, 2005) in integrating different experiences into science (Scott et al., 2011).
The overall shape of protons and neutrons was also a feature that became important as it contributed to the overall shape of the of the nuclei. The shapes of protons, neutrons, and electrons were either circular leading to there being space in-between them (for example, C5 in Figure 2), or they were shaped as wedges leading to no space in-between them (for example, C8 and C15). The protons and neutrons could also be loosely assembled (for example, C7) or close together (for example, C2). Different students used different entailments when building their nuclei, which led to nuclei that were 2 or 3-dimensional, tetradic (for example C1), flat (for example, C5), round (C15), square (C10 and C14), circular (C13), linear (C12), linear-curved (C13), triangular (C6), donut-shaped (C8), and star-shaped (C7).
One group of students saw the construction of atomic nuclei as a continuous repetition of helium nuclei (C12), and the students explained that “the carbon and oxygen nuclei are made of three and four helium nuclei.” These models hold no or little visual resemblance to the teaching model, but they do make sense when focusing on one specific feature, such as number of protons and neutrons. If the focus was only on producing the correct number of protons and neutrons, then the student may not have considered other aspects such as structure and repulsion; one example is C11. If C12 placed focus only on repulsions but not shape, then linear nuclei could also be a reasonable result. These series point toward the students at least initially using one or a few features at the time when working with the teaching models for the atom. This result shows the importance of giving careful attention to teaching models that simultaneously include all relevant features surrounding atomic nuclei.
All these results point to the importance of moving beyond the use of language. Some examples stand out; a student wrote, “Helium has two protons and two neutrons in the nucleus” which is an answer that could have been deemed correct until the student built a nucleus (round ball) and then attached two protons to it (L7 in Figure 3). Several of the students provide correct answers regarding the number of protons and neutrons, but built models with a different number of particles. The opposite also occurs, where the model looks reasonable, but the written answer was incorrect. This finding shows the flexibility of models in use during the learning process.
Although the teacher pointed out to the students on several occasions that they should only build the nucleus and not the atom, totally 16% of the students added electrons to their play-dough models.
In this category student expressed model deviates from the teaching model in that it did not include the correct number of protons or neutrons, electrons were added, size differences were ignored or particles not relevant to atomic nuclei was added (Figure 3). The addition of electrons placed focus on size differences between the subatomic particles. Size and scale are inevitable problems when teaching chemistry, as the actual distance cannot be displayed using the normal variety of teaching models available to teachers. Despite this problem, teaching models do show some size difference, and even if not realistic, many of the students did not take this difference into account (L16) but instead placed the visual similarity on distance between the electron and the nuclei (see for example, L2). Most students that added electrons to their nuclei provided explanations that confirmed that this was their intention. Some also included an electron trajectory; one example is L2 with “one electron trajectory and one electron and one proton.” The addition of electrons did provide variations in the form of shells and the placement of electrons. Some placed electrons on one side of the nucleus (L6); it is difficult to draw a conclusion about the reasons behind this construction, but it is nonetheless an important feature for teachers to address. For some students, the electrons were evenly spread out in different shells or placed closely around the atom. Several of the students placed the electron directly onto the proton in the hydrogen nucleus (L5 and L16), but the distance between electrons and the nucleus increases when the students build larger nuclei, which is a placement that may stem from students trying to depict the small size of the first two atoms and possibly recognizing that space occupied by electrons. The atomic nuclei in Series L1–L6 in Figure 3 contained the same kinds of deviations as seen in the category: close resemblance.
Series L7–L16 in Figure 3 includes the models that deviates the most from the teaching model. This category includes student models were the number of particles either did not match the number of particles in their written explanations (L8–L11) or the number of neutrons in the nuclei was incorrect (L12 and L15). Some included additional particles not found in the teaching model (L7), added protons and electrons in same sizes (L16) or added protons and neutrons of different sizes to their models (L13). One student placed the neutrons in a circle around a core of protons (L14). Of the 64 series in total 17% were included into series L7–L16.
The detailed analysis of the results from the atomic nuclei in Figure 2 shows how uncertain the students were, even those who had built a model with close resemblance to the teaching model (here, the hydrogen nuclei). They expressed doubts concerning their construction; for example, “Is it only one ball? It seems too easy.” In the category of less resemblance some students used the word atom for their construction of the atomic nuclei or built an atom and then referred to it as an atomic nucleus. Analysis of the written and verbal explanations for the hydrogen atom (see Table 1) shows the difference in word use. Only eight variations in explanations were found amongst the 64 students, a result that shows how similar students’ explanations were, a result that may suggest that definitions/explanations were rote learned.
Table 1. A summary and categorization of all students written explanations regarding the hydrogen nucleus.
These results of the playdough constructions can depend on several different reasons, such as a mix-up of words or how the task was formulated; for example, a task that included weight might have given the impression that the electron should be included in the expressed model since the weight of the electron was included in the teaching model. Additionally, it is not uncommon in textbooks to represent both the atomic nuclei and the atom as one circular 3-dimensional ball depending on what phenomena the author is addressing. These types of mixing of teaching models were nonetheless an issue that became visible when the students were provided with the opportunity for creative expression in this form.
The result of this study shows the importance of the mode of expressions provided to students. Written explanations provided eight categories or variations while the playdough constructions opened for choices in assembly of nucleic particles and as a result the number of variations increased. The use of one feature at a time, such as only considering number of particles with no regard for 3-dimensionality, place focus on the importance of not only allowing for the exploration but also on the importance of the teacher to provide a holistic view where all relevant features are taken into consideration. This approach to problem-solving concepts, that is, giving the student a form of creative freedom to express the atom and atomic nuclei, provided teachers with student-generated models suitable for discussion using the framework for models (Gilbert and Justi, 2016).
Moving beyond verbal and written language did indeed provide a level of detail to students’ interpretations of concepts that would otherwise have been difficult to reach. Variations to the method, for example, by asking students to build an oxygen atom and then changing it to a nitrogen atom or building a nitrogen atom and changing it to a carbon-14 isotope, could be tasks that would place more focus on isotopes than the problems used within this study.
Data collection includes 64 students, a number that does not provide saturation of the data set. The results presented here is a cameo-shot of students’ interpretations and consideration expressed only in the form of playdough models and written explanations. The design of the study did not include data collection and analysis of body language such as gestures something that may have delimited the results. Also, a more in-depth analysis would have been possible if discussions and social interactions had been recorded.
The original contributions presented in this study are included in the article/supplementary material, further inquiries can be directed to the corresponding author/s.
Ethical review and approval was not required for the study involving human participants in accordance with the local legislation and institutional requirements. The participants provided written informed consent to participate in the study.
Both authors listed have made a substantial, direct, and intellectual contribution to the work, and approved it for publication.
The authors declare that the research was conducted in the absence of any commercial or financial relationships that could be construed as a potential conflict of interest.
All claims expressed in this article are solely those of the authors and do not necessarily represent those of their affiliated organizations, or those of the publisher, the editors and the reviewers. Any product that may be evaluated in this article, or claim that may be made by its manufacturer, is not guaranteed or endorsed by the publisher.
Acevedo-Díaz, J. A., García-Carmona, A., María del Mar, A. M., and Oliva-Martínez, J. M. (2017). Scientific models: Meaning and role in scientific practice. Rev. Cient. 3, 155–166. doi: 10.14483/23448350.12288
Adbo, K., and Vidal Carulla, C. (2020). Learning about science in preschool: Play-based activities to support children’s understanding of chemistry concepts. Int. J. Early Child. 52, 17–35. doi: 10.1007/s13158-020-00259-3
Aubusson, P. J., Ritchie, S. M., and Harrison, A. G. (2006). Metaphor and Analogy in Science Education. Dordrecht: Springer. doi: 10.1007/1-4020-3830-5
Begoray, D. L., and Stinner, A. (2005). Representing science through historical drama: Lord Kelvin and the age of the earth debate. Sci. Educ. 14, 457–471. doi: 10.1007/s11191-005-0780-y
Bracey, B., and Zoë, E. (2017). Students from non-dominant linguistic backgrounds making sense of cosmology visualizations. J. Res. Sci. Teach. 54, 29–57. doi: 10.1002/tea.21337
Braund, M., and Reiss, M. J. (2019). The ‘great divide’: How the arts contribute to science and science education. Can. J. Sci. Math. Technol. Educ. 19, 219–236. doi: 10.1007/s42330-019-00057-7
Cervetti, G. N., Hiebert, E. H., Pearson, P. D., and McClung, N. A. (2015). Factors that influence the difficulty of science words. J. Lit. Res. 47, 153–185. doi: 10.1177/1086296X15615363
Duit, R. (1991). On the role of analogies and metaphors in learning science. Sci. Educ. 75, 649–672. doi: 10.1002/sce.3730750606
Garnett, P. J., and Hackling, M. W. (1995). Refocusing the chemistry lab: A case for laboratory- based investigations. Aust. Sci. Teach. J. 41, 26–32.
Gilbert, J. K. (2004). Models and modelling: Routes to more authentic science education. Int. J. Sci. Math. Educ. 2, 115–130. doi: 10.1007/s10763-004-3186-4
Gilbert, J. K., and Justi, R. (2016). Modelling-Based Teaching in Science Education. Cham: Springer International Publishing AG.
Gilbert, J. K., Bulte, A. M. W., and Pilot, A. (2011). Concept development and transfer in context-based science education. Int. J. Sci. Educ. 33, 817–837. doi: 10.1080/09500693.2010.493185
Gilbert, J. K., Taber, K. S., and Watts, D. M. (2001). “Quality, level, and acceptability, of explanation in chemical education,” in Proceedings of the 6th European conference on research in chemical education/2nd European conference on chemical education (Aveiro).
Glynn, S. M., and Duit, R. (eds) (1995). “Teaching science with analogies: A strategy for constructing knowledge,” in Learning science in the schools, eds S. M. Glynn and R. Duit (Oxfordshire: Routledge), 259–286. doi: 10.4324/9780203053287-19
Harrison, A. G., and Treagust, D. F. (1996). Secondary students’ mental models of atoms and molecules: Implications for teaching chemistry. Sci. Educ. 80, 509–534. doi: 10.1002/(SICI)1098-237X(199609)80:5<509::AID-SCE2<3.0.CO;2-F
Hedegaard, M. (1995). “The qualitative analysis of the development of a child’s theoretical knowledge and thinking,” in Sociocultural psychology, eds L. M. W. Martin, K. Nelson, and E. Tobach (Cambridge: Cambridge University Press), 293–325. doi: 10.1017/CBO9780511896828.015
Herro, D., and Quigley, C. (2016). Innovating with STEAM in middle school classrooms: Remixing education. Horizon 24, 190–204. doi: 10.1108/OTH-03-2016-0008
Justi, R., and Gilbert, J. (2000). History and philosophy of science through models: Some challenges in the case of “the atom.”. Int. J. Sci. Educ. 22, 993–1009. doi: 10.1080/095006900416875
Justi, R., and van Driel, J. (2006). The use of the interconnected model of teacher professional growth for understanding the development of science teachers’ knowledge on models and modelling. Teach. Teach. Educ. 22, 437–450. doi: 10.1016/j.tate.2005.11.011
Koerber, S., and Osterhaus, C. (2019). Individual differences in early scientific thinking: Assessment, cognitive influences, and their relevance for science learning. J. Cogn. Dev. 20, 510–533. doi: 10.1080/15248372.2019.1620232
Korkmaz, F., and Unsal, S. (2017). Analysis of attainments and evaluation questions in sociology curriculum according to the SOLO taxonomy. Eurasian J. Educ. Res. 17, 75–92. doi: 10.14689/ejer.2017.69.5
Lakoff, G., and Johnson, M. (1987). Metaphors we live by. J. Jpn. Soc. Artif. Intell. 2, 127–128. doi: 10.11517/jjsai.2.1_127
Nersessian, N. J. (2002). Abstraction via generic modeling in concept formation in science. Mind Soc. 3, 129–154. doi: 10.1007/BF02511871
Pantidos, P., and Givry, D. (2021). A semiotic approach for the teaching of energy: Linking mechanical work and heat with the world of objects and events. Rev. Sci. Math. ICT Educ. 15, 5–30. doi: 10.26220/rev.3563
Pantidos, P., Fragkiadaki, G., Kaliampos, G., and Ravanis, K. (2022). Inscriptions in science teaching: From realism to abstraction. Front. Educ. 7:905272. doi: 10.3389/feduc.2022.905272
Papageorgiou, G., Markos, A., and Zarkadis, N. (2016). Understanding the atom and relevant misconceptions: Students’ profiles in relation to three cognitive variables. Sci. Educ. Int. 27, 464–488.
Roth, W.-M., and Lawless, D. (2002). Scientific investigations, metaphorical gestures, and the emergence of abstract scientific concepts. Learn. Instr. 12, 285–304. doi: 10.1016/S0959-4752(01)00023-8
Santau, A. O., Secada, W., Maerten-Rivera, J., Cone, N., and Lee, O. (2010). US Urban elementary teachers’ knowledge and practices in teaching science to English Language learners: Results from the first year of a professional development intervention. Int. J. Sci. Educ. 32, 2007–2032. doi: 10.1080/09500690903280588
Scott, P., Mortimer, E., and Ametller, J. (2011). Pedagogical link-making: A fundamental aspect of teaching and learning scientific conceptual knowledge. Stud. Sci. Educ. 47, 3–36. doi: 10.1080/03057267.2011.549619
Siry, C., and Martin, S. N. (2014). Facilitating reflexivity in preservice science teacher education using video analysis and cogenerative dialogue in field-based methods courses. Eurasia J. Math. Sci. Technol. Educ. 10, 481–508. doi: 10.12973/eurasia.2014.1201a
Steele, J. M. (2000). Eclipse prediction in Mesopotamia. Arch. Hist. Exact Sci. 54, 421–454. doi: 10.1007/s004070050007
Stefani, C., and Tsaparlis, G. (2009). Students’ levels of explanations, models, and misconceptions in basic quantum chemistry: A phenomenographic study. J. Res. Sci. Teach. 46, 520–536. doi: 10.1002/tea.20279
Taber, K. S. (2005). Learning quanta: Barriers to stimulating transitions in student understanding of orbital ideas. Sci. Educ. 89, 94–116. doi: 10.1002/sce.20038
Taber, K. S. (2019). Alternative conceptions and the learning of chemistry. Israel J. Chem. 59, 450–469. doi: 10.1002/ijch.201800046
Talanquer, V. (2006). Commonsense chemistry: A model for understanding students’ alternative conceptions. J. Chem. Educ. 83, 811–816. doi: 10.1021/ed083p811
Tytler, R. (2000). A comparison of year 1 and year 6 students’ conceptions of evaporation and condensation: Dimensions of conceptual progression. Int. J. Sci. Educ. 22, 447–467. doi: 10.1080/095006900289723
van Joolingen, W. (2004). “A tool for the support of qualitative inquiry modeling,” in Proceedings of the IEEE international conference on advanced learning technologies, 2004, (Piscataway, NJ: IEEE), 96–100. doi: 10.1109/ICALT.2004.1357382
Vygotsky, L. S. (2004). Imagination and creativity in childhood. J. Russ. East Eur. Psychol. 42, 7–97. doi: 10.1080/10610405.2004.11059210
Williams, M., and Tang, K. S. (2020). The implications of the non-linguistic modes of meaning for language learners in science: A review. Int. J. Sci. Educ. 42, 1041–1067. doi: 10.1080/09500693.2020.1748249
Keywords: chemistry education, atomic nuclei, sub-microscopic level, models of science, playdough, creative
Citation: Adbo K and AAkesson-Nilsson G (2022) Moving beyond the language–Visualizing chemical concepts through one’s own creative expression. Front. Educ. 7:1034140. doi: 10.3389/feduc.2022.1034140
Received: 01 September 2022; Accepted: 25 November 2022;
Published: 29 December 2022.
Edited by:
Vasilia Christidou, Aristotle University of Thessaloniki, GreeceReviewed by:
Panagiotis Pantidos, National and Kapodistrian University of Athens, GreeceCopyright © 2022 Adbo and AAkesson-Nilsson. This is an open-access article distributed under the terms of the Creative Commons Attribution License (CC BY). The use, distribution or reproduction in other forums is permitted, provided the original author(s) and the copyright owner(s) are credited and that the original publication in this journal is cited, in accordance with accepted academic practice. No use, distribution or reproduction is permitted which does not comply with these terms.
*Correspondence: Karina Adbo, a2FyaW5hLmFkYm9AbWF1LnNl; Gunilla AAkesson-Nilsson, Z3VuaWxsYS5ha2Vzc29uLm5pbHNzb25AYnRoLnNl
†These authors have contributed equally to this work
Disclaimer: All claims expressed in this article are solely those of the authors and do not necessarily represent those of their affiliated organizations, or those of the publisher, the editors and the reviewers. Any product that may be evaluated in this article or claim that may be made by its manufacturer is not guaranteed or endorsed by the publisher.
Research integrity at Frontiers
Learn more about the work of our research integrity team to safeguard the quality of each article we publish.