- 1Institut de Physique du Globe de Paris, Sorbonne Paris-Cité, UMR 7154 CNRS, Paris, France
- 2Institut des sciences de la mer de Rimouski (ISMER), Canada Research Chair in Marine Geology and GEOTOP, Université du Québec à Rimouski, Rimouski, QC, Canada
- 3Central Institute for Meteorology and Geodynamics, Vienna, Austria
Rapidly deposited layers (RDL) such as turbidites or hyperpycnites are mostly studied for their sedimentological properties, but are carefully avoided in paleomagnetic studies due to the disturbances caused by such sudden and rapid sediment accumulation. Therefore, these layers can also be seen as potential indicators of sediment parameters susceptible of affecting the alignment of magnetic grains and ultimately the acquisition of the natural remanent magnetization (NRM). We have compiled 13 Holocene rapidly deposited layers from core MD99-2222 in the Saguenay Fjord, eastern Canada (St-Onge and al., 2004) with varying thicknesses (from 7.1 cm to 1,510 cm) and 4 Quaternary turbidites of different origins, to document the influence of sedimentary and magnetic parameters on natural remanent magnetization acquisition. We found a logarithmic relationship between rapidly deposited layers thickness on the one hand, and the amplitude of inclination changes and magnetic grain sizes on the other. Inclination and magnetic grain sizes are themselves correlated to each other by a logarithmic law. As there is no relationship between inclination deviation and stratigraphic depth, compaction alone cannot account for such large effects on inclination. Flocculation is grain size sensitive, but it is expected to affect mainly the natural remanent magnetization intensity, rather than its direction. Turbulence that prevails during the rapid deposition of sediments during such events is most likely the dominant factor.
Introduction
Measurements of sediment natural remanent magnetization (NRM) are useful to chronostratigraphic, paleomagnetic and paleoenvironmental studies (e.g., Meynadier et al., 1992; Valet and Meynadier, 1993; Roberts et al., 1997; Kissel et al., 1998; Channell et al., 2000; Channell & Kleiven, 2000; Stoner et al., 2000; Valet, 2003; Stoner and St-Onge, 2007; Lisé-Pronovost et al., 2009; Barletta et al., 2010; Macrì et al., 2010; Mazaud et al., 2012; Caron et al., 2018; Deschamps et al., 2018; Bieber et al., 2021; Velle et al., 2022). Despite significant progress in the understanding of magnetization acquisition in sediments, the mechanisms that govern the detrital and post-detrital remanences remain relatively unconstrained (Tauxe et al., 2006). This uncertainty negatively affects the interpretation of paleomagnetic records. Various processes controlling NRM acquisition have been proposed through redeposition experiments and modelling (Nagata, 1961; Collinson, 1965; Stacey, 1972; Denham and Chave, 1982; Tauxe, 1993; Katari and Bloxham, 2001; Tauxe et al., 2006; Shcherbakov and Sycheva, 2010; Roberts et al., 2013). The first model of deposition proposed by Nagata (1961) focused on the rotation of magnetic grains within a fluid immersed in a magnetic field and predicted that all magnetic grains would be rapidly (<1 s) aligned by the field leading to saturation of the remanent magnetization. However, typical values of the natural remanence in sediments and in sediment redeposition experiments are two or three order of magnitudes below saturation (Tauxe, 1993; Tauxe et al., 2006; Spassov and Valet, 2012; Roberts et al., 2013). Collinson (1965) proposed that the absence of saturation could be linked to the Brownian motion, but this process affects only very fine particles (Stacey, 1972). Flocculation appears to be a more realistic controlling factor, as it agglomerates sedimentary particles and therefore affects the alignment of the magnetic grains by the field (Shcherbakov and Shcherbakova, 1983; Tauxe, 1993; Katari and Bloxham, 2001; Tauxe et al., 2006). In fact, the timing of magnetization acquisition depends on various sedimentary and magnetic parameters that can also introduce a delay between sediment deposition and lock-in of the remanent magnetization (Verosub, 1977; Sagnotti et al., 2005; Shcherbakov and Sycheva, 2010; Roberts et al., 2013).
Several experimental studies (Quidelleur et al., 1995; Katari et al., 2000; Carter-Stiglitz et al., 2006; Heslop et al., 2006; Tauxe et al., 2006; Spassov and Valet, 2012) attempted to evaluate the role played by specific parameters (e.g., water content, magnetic concentration, salinity, carbonate and clay content, flocculation, compaction) on the timing and alignment of magnetic grains within the sediment. However, the use of laboratory redeposition experiment as analogue to natural deposition is limited by lateral size limitations and short duration of the experiments compared to those in nature. Rapidly deposited layers (RDL) like turbidites can be seen as a natural analogue to laboratory redeposition experiment. So far, RDLs have been mostly studied for their sedimentological properties (e.g., Mulder and Alexander, 2001; Mulder et al., 2001; Alexander and Mulder, 2002; Zavala and Arcuri, 2016; Feng et al., 2021; Talling, 2021; Mérindol et al., 2022; Rodríguez-Tovar, 2022) and only a few rock magnetic parameters have been investigated (e.g., St-Onge et al., 2004; Lisé-Pronovost et al., 2014; Duboc et al., 2017; Kanamatsu et al., 2022).
A magnetic study of four distinct turbidites from the Bay of Bengal, Gulf of Corinth and Eastern China Sea was recently published by Tanty et al. (2016) (Figure 1). The sedimentary and magnetic grain sizes revealed a significant coarsening of both sediment particles and magnetic grains within the bottom layers. The most striking observation was the existence of progressive shallowing of the magnetic inclinations between the upper and bottom layers that increases with the size of the event and obeys a simple linear scaling law. These results have been however obtained from a low number of events. It is thus necessary to establish a more complete database that would incorporate RDLs of different sizes and nature and to assess whether common properties emerge that could pave the way to a better understanding of detrital remanent magnetization. In this paper, we will thus investigate 17 turbidites from four different regions ranging from 7.1 cm to 15.1 m, and use them to investigate possible NRM acquisition mechanisms in turbidites.
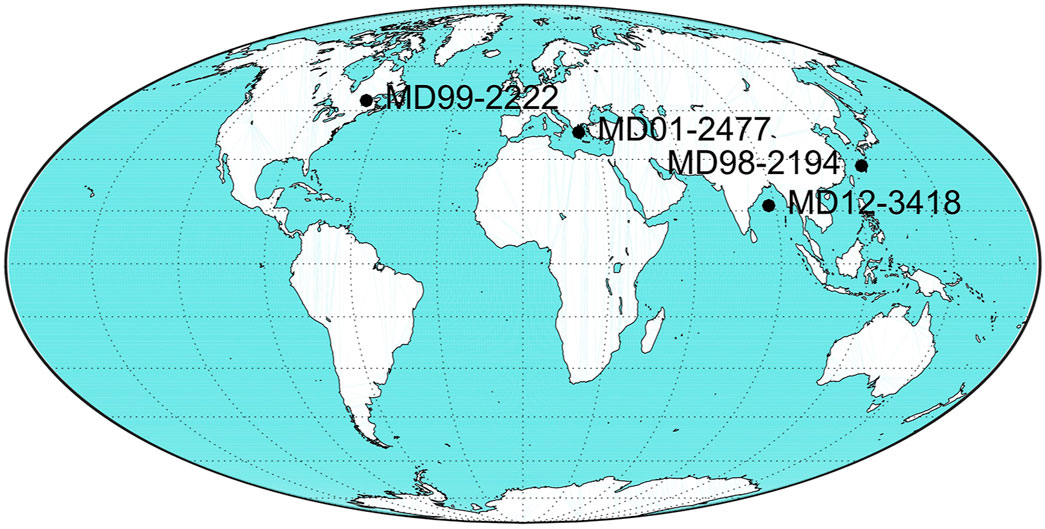
FIGURE 1. Location of the sampling sites of cores MD01-2477 (Gulf of Corinth), MD12-3418 (Bay of Bengal), MD98-2194 (China Sea) and MD99-2222 (Saguenay Fjord, Canada).
Methodology
Core MD99-2222
All properties of core MD99-2222 have been studied by St-Onge et al. (2004). Core MD99-2222 was sampled in the Saguenay Fjord, eastern Canada (48°18.28′ N, 70°15.44’ W, water depth 271 m, Figure 1). Low-field magnetic susceptibility (k) was measured every 2 cm with a GEOTEK Multi Sensor Core Logger on board of the R/V Marion Dufresne II. Grain size analyses were conducted at the Université de Bordeaux with a Malvern Supersizer “S” laser grain size analyzer. The grain size data were analyzed using the Gradistat software (Blott and Pye, 2001). The core was sampled using U-channels and sediment magnetization was measured every 1 cm at the University of California in Davis using a 2G Enterprises cryogenic magnetometer Model 755. The Natural Remanent Magnetization (NRM) was measured on U-channel samples and then demagnetized with an alternating field (AF) in 5 mT steps from 10 mT to 40 mT and then every 10 mT up to 80 mT. Inclination was calculated by principal component analysis (Kirschvink, 1980). An anhysteretic remanent magnetization (ARM) was produced using a 100 mT peak AF with a 50 μT direct current (DC) biasing field. This ARM was subsequently demagnetized with an alternating field (AF) at 10 mT, steps from 10 mT to 20 mT every 5 mT–40 mT and then every 10 mT up to 60 mT.
The kARM/k ratio was obtained by calculating the susceptibility of the ARM (kARM) by normalizing the ARM by the strength of the biasing field. Because k is sensitive to the coarser fraction of magnetite, it is frequently associated with ARM that responds dominantly to small magnetic grains and is therefore used as a magnetic grain size proxy (e.g., King et al., 1983; Stoner et al., 1996; Stoner and St-Onge, 2007). The ratio of the parameters depicts the evolution of magnetic grain size.
Cores from Tanty et al. (2016)
The study of Tanty et al. (2016) focused on the detrital remanent magnetization of four turbidites found in cores MD12-3418, MD01-2477 and MD98-2194. Two turbidites were sampled and studied in core MD12-3418 from the Bay of Bengal (16°30.27 N, 87°47.92 E, water depth 2,547 m), one in core MD01-2477 from the Gulf of Corinth (38°.133 N, 22°.333 E, water depth 867 m) and one in core MD98-2,194 from Eastern China Sea (28°06′ N; 127°22’ E, water depth 989 m, Figure 1). The turbidites of the first two cores were deposited during the Holocene, while the event identified in core MD98-2194 was dated to the Pleistocene (Tanty et al., 2016). The NRM of the discrete samples taken within each turbidite was measured at IPGP using a 2G Enterprises cryogenic magnetometer Model 755-R. All samples were demagnetized with an AF using an AGICO LDA-3 demagnetizer at 5 mT steps up to 30 mT and then by steps of 10 mT up to 80 mT.
Comparison of rapidly deposited layer from core MD99-2222
Magnetic characteristics
Core MD99-2222 from the Saguenay Fjord (Figure 1) was previously studied by St-Onge et al. (2004). It includes 13 Holocene RDL with varying thicknesses from 7.1 cm to 1,510 cm among which six turbidites, six hyperpycnites and one undefined event were identified (Figure 2). A hyperpycnite is different from a classic turbidite as it can produce inverse and then normal grading within the same event (e.g., Mulder et al., 1998; Mulder et al., 2003; Mulder and Chapron, 2011).
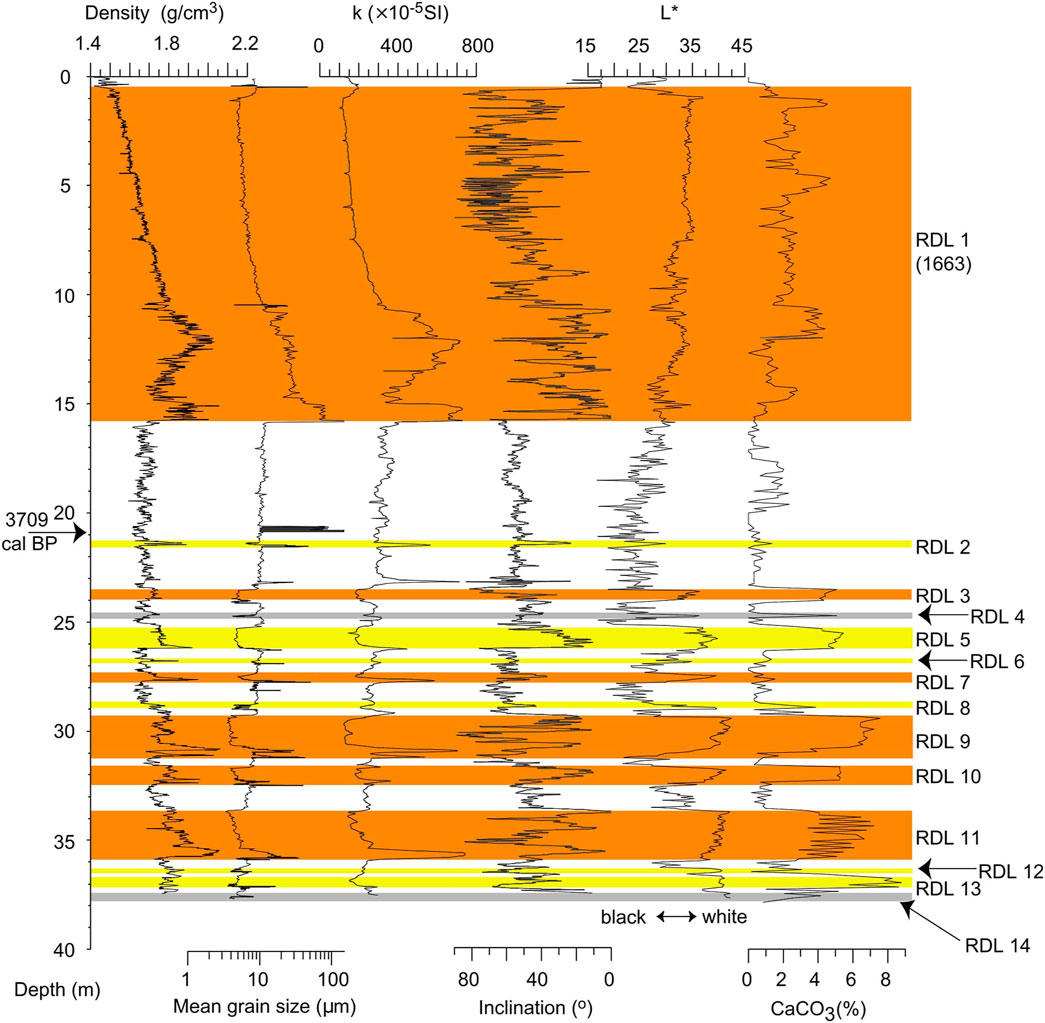
FIGURE 2. Sedimentological and physical properties of core MD99-2222 (St-Onge et al., 2004).
The sedimentological and physical properties of the material from core MD99-2222 have been previously reported by St-Onge et al. (2004) (Figure 2). Magnetic mineralogy was studied at 1.5 m intervals (St-Onge et al., 2004) and showed no change within the RDL. Magnetic granulometry indicates the presence of pseudo-single domain (PSD) and multidomain (MD) grains. These characteristics remain similar for each RDL. We infer that since the Earth’s magnetic field remained constant during the very short period of a turbiditic process, any evolution in direction and/or intensity of the remanent magnetization can only result from changes in magnetization acquisition processes.
Variations of sediment grain size, k, kARM/k and NRM inclination within the 1,510-cm thick hyperpycnite from core MD99-2222 are illustrated in Figure 3. The evolution of sediment grain size with depth is the most sensitive parameter to define the RDL boundaries. All four indicators follow a parallel evolution which confirms the characteristics reported by Tanty et al. (2016) from other turbidites. Coarsest sediment and magnetic particles occur at the base of the event, while the NRM inclination deviates by up to ∼60° from the inclination of the geocentric axial dipole (GAD) at the site.
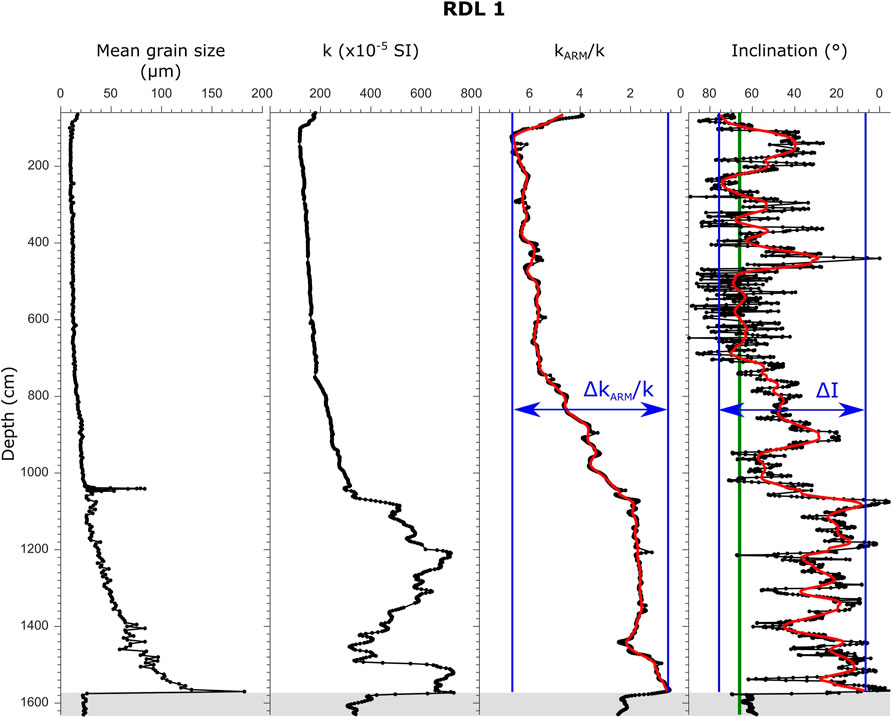
FIGURE 3. Sedimentological and physical properties of the RDL1 event from core MD99-2222 (St-Onge et al., 2004). Red curves were obtained by singular spectrum analysis (SPA). The maximum amplitude changes in inclinations and grain sizes were calculated from the SPA results and are shown by blue arrows. Green line in the inclination represents the geocentric axial dipole (GAD) value. The grey zone represents a normal period of sedimentation before the RDL1 deposition.
Coherent features between rapidly deposited layers
Using this extended database, we can investigate further the relationship between magnetic parameters and RDL that was reported by Tanty et al. (2016). Typical parallel evolutions of the NRM inclination and kARM/k within an RDL event are shown in Figure 3. We selected RDL 1 due to its thickness; similar features describe all events as seen in supplementary material. Singular spectrum analysis (Vautard and Ghil, 1989) was used to obtain inclinations and kARM/k trends for all RDL events from core MD99-2222 (red lines in Figure 3). Inclination variations, from 20° to 80°, correlate with the decreasing grain size trend, from coarsest at the bottom to finest at the top. Superimposed to this trend, inclination shows high-frequency large-amplitude fluctuations that do not occur in grain size. These fluctuations likely result from turbulent conditions that affected the alignment of the magnetic grains in the Earth’s magnetic field. Turbulence is expected to strongly affect the orientation of suspended particles, especially larger ones (Heslop, 2007), but not the grain size dependence of the mean settling velocity, which is responsible for graded bedding.
We first scrutinized the inclination changes within each event and defined the amplitude of inclination changes from the results of singular spectrum analysis as ΔI = Imax − Imin (Figure 3). Inclination changes within each RDL sequence, including those studied by Tanty et al. (2016), depends logarithmically on the sequence thickness (Figure 4). The 1,510 cm thick turbidite, which is much larger than all other events, has been excluded from this comparison. The best-fit logarithmic curve (Figure 4, black curve with shaded confidence interval) is the best fit obtained using a simulated annealing and bootstrap estimator (Efron and Tibshirani, 1986). The observed relation between ΔI and thickness differs from the linear curve that was previously reported by Tanty et al. (2016), probably because of the larger number of data. Except for the smallest turbidite from Tanty et al. (2016) and one turbidite from MD9-2222, all other RDL lie within the confidence interval. We note that the turbiditic or hyperpycnite nature of the RDL does not seem to influence the logarithmic trend shown in Figure 4.
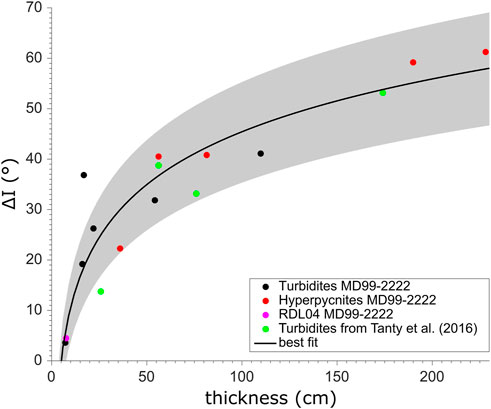
FIGURE 4. Amplitude of inclination changes within 16 RDLs as a function of their thickness. Black, red and pink closed circles are for turbidites, hyperpycnites and other RDLs from core MD99-2222, respectively. Green closed circles show the values of the four turbidites studied by Tanty et al. (2016).
Similarly, we investigated the relationship between the event thickness and the maximum amplitude ∆(kARM/k) of changes in the magnetic grain size proxy resulting from singular spectrum analysis (Figure 5). In this case, we were constrained to restrain the study to the 12 RDL events from core MD99-2222 because other field values were used by Tanty et al. (2016) to induce the ARMs. Also in this case, magnetic grain size changes depend logarithmically on the event thickness, as seen by the trend line obtained with the same annealing and bootstrap estimator used in Figure 4. All data points lie within the confidence interval except for a single hyperpycnite.
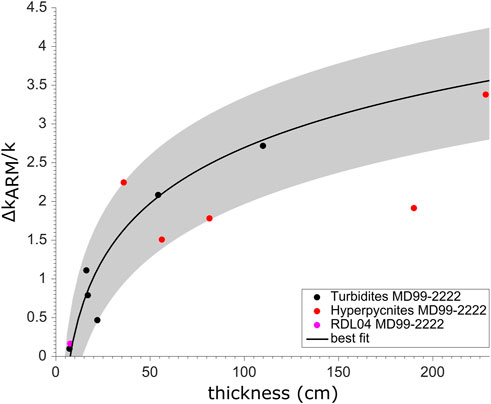
FIGURE 5. Amplitude of grain size changes within 12 RDLs as a function of their thickness. Black, red and pink closed circles are for turbidites, hyperpycnites and other RDLs from core MD99-2222, respectively.
For layers thinner than 0.5 m, the amplitude of inclination and magnetic grain size fluctuations are almost linearly correlated with the thickness of the event. For layers thicker than 0.5 m, the correlation becomes more evidently a logarithmic one. When plotted with respect to each other, the amplitudes of inclination and magnetic grain size changes are not linearly correlated, but follow again a logarithmic dependence (Figure 6). One turbidite and two hyperpycnite do not lie within the confidence interval.
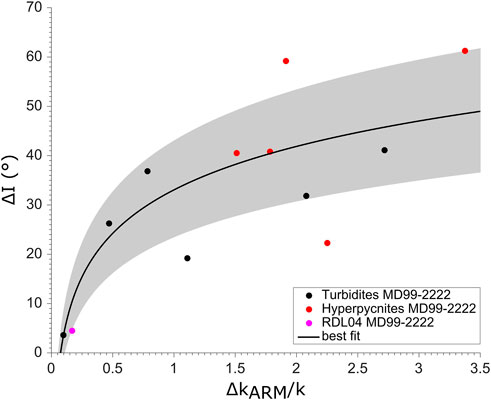
FIGURE 6. Amplitude of inclination changes as a function of grain size changes within 12 RDLs. Same symbols as in Figure 5.
Discussion
Magnetic and/or sedimentary material that feed the turbidites and the hyperpycnites in the Saguenay Fjord come from the same source (Mulder et al., 1998; St-Onge et al., 2004). The evolution of the magnetic and sediment grain sizes follows a similar pattern in all RDLs with significant decreasing grain size from the bottom to the top. The amplitude of the changes increases with the magnitude of the event as shown by the data from core MD99-2222. Turbidites and hyperpycnites from the four different sites (Figure 1) were produced in different water column thicknesses, and therefore the water column depth would have no significant impact on the grain sizes pattern within the sediment and also no influence on the magnetic alignment near or at the sea floor. The high concentration of particles during a turbidity or an hyperpycnal current favored the formation of aggregates after segregation of coarse magnetic and sedimentary grains, that is, not during the primary stage of the discharge, but also not long after deposition, because rapid accumulation of sediment reinforces particle cohesion and impedes post-depositional reorientation (Tanty et al., 2016).
As expected, the results in Figure 6 reveal that the large changes in magnetic grain size within the thick events correlate with large inclination changes. Inclination shallowing decreases within the event. The inclinations are near zero with some negative values at the base of the thickest turbidites like the 1,510 cm thick hyperpycnite from core MD99-2222 (Figure 3). In this case, inclination shows a regular trend with a zone of chaotic fluctuations below 1,100 cm and a mean inclination of ∼25°, followed by a transition interval that ends with a mean inclination of ∼55° above 700 cm. For comparison, the modern field inclination at the site is 71°. The observed inclination trend correlates with the magnetic grain size coarsening recorded by the kARM/k variations (Figure 3).
Compaction is frequently considered to be responsible for inclination shallowing in sediments (Anson and Kodama, 1987; Arason and Levi, 1990). The logarithmic relationship between inclination and magnetic grain size may also support the role of compaction (Maier et al., 2013). However, compaction does not generate inclination deviations as large as 60° (Anson and Kodama, 1987; Arason and Levi, 1990; Sun and Kodama, 1992) and becomes significant only at much larger depths (below 100 m, Sun and Kodama, 1992). The absence of any relationship between the mean inclination of each RDL event and the core depth of the event (Figure 7) further confirms that the inclination deviations are not controlled by compaction.
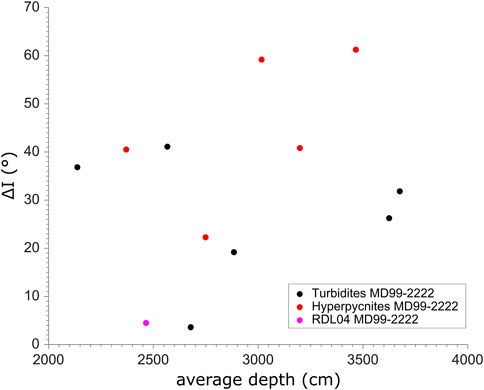
FIGURE 7. Amplitude of inclination changes within 12 RDLs as a function of their average depth in core MD99-2222. Black, red and pink closed circles are for turbidites, hyperpycnites and other RDLs from core MD99-2222, respectively.
Turbulence and flocculation (Tauxe, 1993; Tanty et al., 2016) can both affect the orientation of the magnetic grains. Flocculation has been discussed in several models (Katari and Bloxham, 2001; Tauxe et al., 2006; Shcherbakov and Sycheva, 2010; Roberts et al., 2013). The flocculation process depends to some extent on sediment grain size, as finer sediment particles are more cohesive than larger ones (van Leussen, 1988). In turbidites, turbulence is expected to play a major role, as it promotes flocculation through increased collision frequencies but also breaks large aggregates, thus determining the maximum size and minimum density of flocs (Clark and Flora, 1991; Winterwerp, 1998). Because of the lack of correlation between ΔI and sediment grain size (Figure 8), the changes in inclination across each event must be controlled by turbulence. Largest inclination shallowing occurs systematically at the base of an event, where sediment material was deposited under maximum shear flow. Shear flows tends to align the long axis of individual particles and flocs parallel to the flow axis (Harada et al., 2006). Repeated turbulence-induced floc breakup and aggregation will thus progressively align individual constituents, including magnetic particles, along the horizontal flow direction. Because the magnetic moment of a ferrimagnetic grain tend to align with the grain’s longest axis, newly formed flocs tend to contain magnetic particles with their moments aligned along the flow direction, rather than parallel to the magnetic field, explaining the progressive transition from sub-horizontal to GAD-like inclinations.
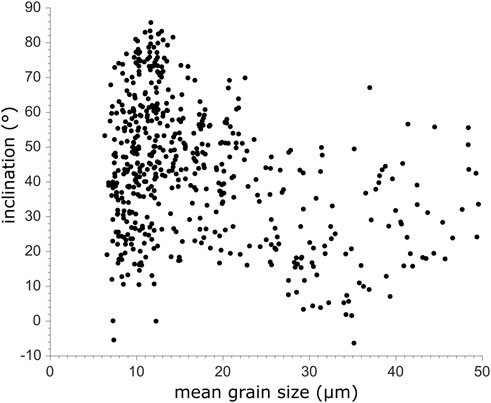
FIGURE 8. Inclination as a function of sediment mean grain size in 13 RDLs from core MD99-2222 (St-Onge et al., 2004). The linear correlation coefficient is R2 = 0.14.
Conclusion
We have compared the magnetic characteristics of 17 RDLs of different sizes and origins. The results confirmed several observations concerning the alignment of magnetic grains in turbulent conditions that were derived from a previous study of four turbidites. A major characteristic is that the degree of inclination shallowing within the sequence increases with the magnitude of events and that this relationship can be well described by a logarithmic dependence on the event thickness. We could pinpoint the origin of inclination shallowing within RDLs to the turbulence associated with shear flow. Sediment grain size and compaction, on the other hand, have insignificant effects in these settings. We explain inclination shallowing by the preferential alignment of magnetic particles with the horizontal flow direction during floc breakup and reaggregation.
The much smaller degree of inclination shallowing observed in quiet depositional environments emphasizes the importance of post-depositional reorientation of magnetic grains in the surface mixed layer of regularly deposited sediments. This reorientation suppresses or drastically reduces the inclination shallowing inherited by the original remanent magnetization acquired during deposition (Zhao et al., 2016). Post-depositional reorientation does not occur at the bottom of the turbidites due to the fast accumulation of the upper sediment layers which prevents grain mobility within the lower layers and/or long exposure to bioturbation.
Data availability statement
Publicly available datasets were analyzed in this study. This data can be found here: https://doi.org/10.1016/j.quascirev.2003.03.001 and https://doi.org/10.1002/2016GC006378 the data are in published article.
Author contributions
All authors listed have made a substantial, direct, and intellectual contribution to the work and approved it for publication.
Funding
This research was funded by the Natural Sciences and Engineering Research Council of Canada (NSERC) Discovery grant RGPIN-2017-05294 to GSO and by the ERC advanced Grant agreement ID: 339899 “EDIFICE” funded under the ERC’s 7th framework program FO7-IDEAS-ERC (European Union).
Acknowledgments
We also thank the captains, the crew and the scientific participants of the IMAGES IV, IMAGES V, MD124 Geosciences 2 and MD191 MONOPOL campaigns on board the Marion Dufresne II in 1998, 1999, 2001 and 2012, respectively. Many thanks to Cyrielle Tanty and Fernando Lopes at IPGP for various advices and Amel Philippe for proofreading this article. This is xxxx IPGP contribution number.
Conflict of interest
The authors declare that the research was conducted in the absence of any commercial or financial relationships that could be construed as a potential conflict of interest.
The reviewer LS declared a past co-authorship with the author JPMV to the handling editor.
Publisher’s note
All claims expressed in this article are solely those of the authors and do not necessarily represent those of their affiliated organizations, or those of the publisher, the editors and the reviewers. Any product that may be evaluated in this article, or claim that may be made by its manufacturer, is not guaranteed or endorsed by the publisher.
Supplementary material
The Supplementary Material for this article can be found online at: https://www.frontiersin.org/articles/10.3389/feart.2022.1079229/full#supplementary-material
References
Alexander, J., and Mulder, T. (2002). Experimental quasi-steady density currents. Mar. Geol. 186 (3), 195–210. doi:10.1016/S0025-3227(02)00313-4
Anson, G. L., and Kodama, K. P. (1987). Compaction-induced inclination shallowing of the post-depositional remanent magnetization in a synthetic sediment. Geophys. J. Int. 88 (3), 673–692. doi:10.1111/j.1365-246X.1987.tb01651.x
Arason, P., and Levi, S. (1990). Compaction and inclination shallowing in deep-sea sediments from the Pacific Ocean. J. Geophys. Res. 95 (B4), 4501–4510. doi:10.1029/JB095iB04p04501
Barletta, F., St-Onge, G., Stoner, J. S., Lajeunesse, P., and Locat, J. (2010). A high-resolution Holocene paleomagnetic secular variation and relative paleointensity stack from eastern Canada. Earth Planet. Sci. Lett. 298 (1–2), 162–174. doi:10.1016/j.epsl.2010.07.038
Bieber, A., St-Onge, G., Feuillet, N., Carlut, J., Moreno, E., and Michel, E. (2021). Regional chronostratigraphy in the eastern Lesser Antilles quaternary fore-arc and accretionary wedge sediments: Relative paleointensity, oxygen isotopes and reversals. Quat. Geochronol. 65, 101179. doi:10.1016/j.quageo.2021.101179
Blott, S. J., and Pye, K. (2001). Gradistat: A grain size distribution and statistics package for the analysis of unconsolidated sediments. Earth Surf. Process. Landforms 26 (11), 1237–1248. doi:10.1002/esp.261
Caron, M., St-Onge, G., Montero-Serrano, J.-C., Rochon, A., Georgiadis, E., Giraudeau, J., et al. (2018). Holocene chronostratigraphy of northeastern Baffin Bay based on radiocarbon and palaeomagnetic data. Boreas 48, 147–165. doi:10.1111/bor.12346
Carter-Stiglitz, B., Valet, J.-P., and LeGoff, M. (2006). Constraints on the acquisition of remanent magnetization in fine-grained sediments imposed by redeposition experiments. Earth Planet. Sci. Lett. 245 (1), 427–437. doi:10.1016/j.epsl.2006.03.002
Channell, J. E. T., and Kleiven, H. F. (2000). Geomagnetic palaeointensities and astrochronological ages for the matuyama–brunhes boundary and the boundaries of the jaramillo subchron: Palaeomagnetic and oxygen isotope records from ODP site 983. Philosophical Trans. R. Soc. Lond. Ser. A Math. Phys. Eng. Sci. 358 (1768), 1027–1047. doi:10.1098/rsta.2000.0572
Channell, J. E. T., Stoner, J. S., Hodell, D. A., and Charles, C. D. (2000). Geomagnetic paleointensity for the last 100 kyr from the sub-antarctic south atlantic: A tool for inter-hemispheric correlation. Earth Planet. Sci. Lett. 175 (1–2), 145–160. doi:10.1016/S0012-821X(99)00285-X
Clark, M. M., and Flora, J. R. V. (1991). Floc restructuring in varied turbulent mixing. J. Colloid Interface Sci. 147, 407–421. doi:10.1016/0021-9797(91)90174-7
Collinson, D. W. (1965). Depositional remanent magnetization in sediments. J. Geophys. Res. 70 (18), 4663–4668. doi:10.1029/jz070i018p04663
Denham, C. R., and Chave, A. D. (1982). Detrital remanent magnetization: Viscosity theory of the lock-in zone. J. Geophys. Res. 87 (B8), 7126–7130. doi:10.1029/jb087ib08p07126
Deschamps, C.-E., St-Onge, G., Montero-Serrano, J.-C., and Polyak, L. (2018). Chronostratigraphy and spatial distribution of magnetic sediments in the Chukchi and Beaufort seas since the last deglaciation. Boreas 47 (2), 544–564. doi:10.1111/bor.12296
Duboc, Q., St-Onge, G., and Lajeunesse, P. (2017). Sediment records of the influence of river damming on the dynamics of the Nelson and Churchill Rivers, Western Hudson Bay, Canada, during the last centuries. Holocene 27 (5), 712–725. doi:10.1177/0959683616670465
Efron, B., and Tibshirani, R. (1986). Bootstrap methods for standard errors, confidence intervals, and other measures of statistical accuracy. Stat. Sci. 1 (1), 54–75. doi:10.1214/ss/1177013815
Feng, Y., Zou, C., Li, J., Lin, C., Wang, H., Jiang, S., et al. (2021). Sediment gravity-flow deposits in Late Cretaceous Songliao postrift downwarped lacustrine basin, northeastern China. Mar. Petroleum Geol. 134, 105378. doi:10.1016/j.marpetgeo.2021.105378
Harada, S., Tanaka, R., Nogami, H., and Sawada, M. (2006). Dependence of fragmentation behavior of colloidal aggregates on their fractal structure. J. Colloid Interface Sci. 301, 123–129. doi:10.1016/j.jcis.2006.04.051
Heslop, D. (2007). Are hydrodynamic shape effects important when modelling the formation of depositional remanent magnetization. Geophys. J. Int. 171, 1029–1035. doi:10.1111/j.1365-246X.2007.03588.x
Heslop, D., Witt, A., Kleiner, T., and Fabian, K. (2006). The role of magnetostatic interactions in sediment suspensions. Geophys. J. Int. 165 (3), 775–785. doi:10.1111/j.1365-246X.2006.02951.x
Kanamatsu, T., Ikehara, K., and Hsiung, K.-H. (2022). Stratigraphy of deep-sea marine sediment using paleomagnetic secular variation: Refined dating of turbidite relating to giant earthquake in Japan Trench. Mar. Geol. 443, 106669. doi:10.1016/j.margeo.2021.106669
Katari, K, and Bloxham, J. (2001). Effects of sediment aggregate size on DRM intensity: A new theory. Earth Planet. Sci. Lett. 186 (1), 113–122. doi:10.1016/s0012-821x(00)00386-1
Katari, K., Tauxe, L., and King, J. (2000). A reassessment of post-depositional remanent magnetism: Preliminary experiments with natural sediments. Earth Planet. Sci. Lett. 183 (1), 147–160. doi:10.1016/s0012-821x(00)00255-7
King, J. W., Banerjee, S. K., and Marvin, J. (1983). A new rock-magnetic approach to selecting sediments for geomagnetic paleointensity studies: Application to paleointensity for the last 4000 years. J. Geophys. Res. 88 (B7), 5911–5921. doi:10.1029/jb088ib07p05911
Kirschvink, J. L. (1980). The least-squares line and plane and the analysis of palaeomagnetic data. Geophys. J. Int. 62 (3), 699–718. doi:10.1111/j.1365-246X.1980.tb02601.x
Kissel, C., Laj, C., Mazaud, A., and Dokken, T. (1998). Magnetic anisotropy and environmental changes in two sedimentary cores from the Norwegian Sea and the North Atlantic. Earth Planet. Sci. Lett. 164 (3), 617–626. doi:10.1016/S0040-1951(98)00223-6
Lisé-Pronovost, A., St-Onge, G., Brachfeld, S., Barletta, F., and Darby, D. (2009). Paleomagnetic constraints on the Holocene stratigraphy of the arctic alaskan margin. Glob. Planet. Change 68 (1), 85–99. doi:10.1016/j.gloplacha.2009.03.015
Lisé-Pronovost, A., St-Onge, G., Gogorza, C., Jouve, G., Francus, P., and Zolitschka, B. (2014). Rock-magnetic signature of precipitation and extreme runoff events in south-eastern Patagonia since 51, 200 cal BP from the sediments of Laguna Potrok Aike. Quat. Sci. Rev. 98, 110–125. doi:10.1016/j.quascirev.2014.05.029
Macrì, P., Sagnotti, L., Dinarès-Turell, J., and Caburlotto, A. (2010). Relative geomagnetic paleointensity of the brunhes chron and the matuyama–brunhes precursor as recorded in sediment core from wilkes land basin (Antarctica). Phys. Earth Planet. Interiors 179 (1), 72–86. doi:10.1016/j.pepi.2009.12.002
Maier, D. B., Rydberg, J., Bigler, C., and Renberg, I. (2013). Compaction of recent varved lake sediments. GFF 135 (3–4), 231–236. doi:10.1080/11035897.2013.788551
Mazaud, A., Channell, J. E. T., and Stoner, J. S. (2012). Relative paleointensity and environmental magnetism since 1.2 ma at IODP site U1305 (eirik drift, NW atlantic). Earth Planet. Sci. Lett. 357 (358), 137–144. doi:10.1016/j.epsl.2012.09.037
Mérindol, M., St-Onge, G., Sultan, N., Lajeunesse, P., and Garziglia, S. (2022). Earthquake-triggered submarine landslides in the St. Lawrence Estuary (Québec, Canada) during the last two millennia and the record of the major 1663 CE M≥ 7 event. Quat. Sci. Rev. 291, 107640. doi:10.1016/j.quascirev.2022.107640
Meynadier, L., Valet, J.-P., Weeks, R., Shackleton, N. J., and Hagee, V. L. (1992). Relative geomagnetic intensity of the field during the last 140 ka. Earth Planet. Sci. Lett. 114 (1), 39–57. doi:10.1016/0012-821X(92)90150-T
Mulder, T, and Alexander, J. (2001). The physical character of subaqueous sedimentary density flows and their deposits. Sedimentology 48 (2), 269–299. doi:10.1046/j.1365-3091.2001.00360.x
Mulder, T, and Chapron, E. (2011). Flood deposits in continental and marine environments: Character and significance. AAPG Stud. Geol. 61, 1–30.
Mulder, T, Syvitski, J. P. M., Migeon, S., Faugères, J.-C., and Savoye, B. (2003). Marine hyperpycnal flows: Initiation, behavior and related deposits. A review. Mar. Petroleum Geol. 20 (6–8), 861–882. doi:10.1016/j.marpetgeo.2003.01.003
Mulder, T, Syvitski, J. P. M., and Skene, K. I. (1998). Modeling of erosion and deposition by turbidity currents generated at river mouths. J. Sediment. Res. 68 (1). Retrieved from:http://archives.datapages.com/data/sepm/journals/v66-67/data/068/068001/0124.HTM. doi:10.1306/d4268725-2b26-11d7-8648000102c1865d
Mulder, T., Migeon, S., Savoye, B., and Jouanneau, J.-M. (2001). Twentieth century floods recorded in the deep Mediterranean sediments. Geol. 29 (11), 1011–1014. doi:10.1130/0091-7613(2001)029<1011:tcfrit>2.0.co;2
Quidelleur, X., Valet, J.-P., LeGoff, M., and Bouldoire, X. (1995). Field dependence on magnetization of laboratory-redeposited deep-sea sediments: First results. Earth Planet. Sci. Lett. 133 (3), 311–325. doi:10.1016/0012-821x(95)00088-t
Roberts, A. P., Lehman, B., Weeks, R. J., Verosub, K. L., and Laj, C. (1997). Relative paleointensity of the geomagnetic field over the last 200, 000 years from ODP sites 883 and 884, north pacific ocean. Earth Planet. Sci. Lett. 152 (1), 11–23. doi:10.1016/S0012-821X(97)00132-5
Roberts, A. P., Tauxe, L., and Heslop, D. (2013). Magnetic paleointensity stratigraphy and high-resolution quaternary geochronology: Successes and future challenges. Quat. Sci. Rev. 61, 1–16. doi:10.1016/j.quascirev.2012.10.036
Rodríguez-Tovar, F. J. (2022). Ichnological analysis: A tool to characterize deep-marine processes and sediments. Earth-Science Rev. 228, 104014. doi:10.1016/j.earscirev.2022.104014
Sagnotti, L., Budillon, F., Dinarès-Turell, J., Iorio, M., and Macrì, P. (2005). Evidence for a variable paleomagnetic lock-in depth in the Holocene sequence from the Salerno Gulf (Italy): Implications for “high-resolution” paleomagnetic dating. Geochem. Geophys. Geosyst. 6 (11). doi:10.1029/2005GC001043
Shcherbakov, V. P., and Shcherbakova, V. V. (1983). On the theory of depositional remanent magnetization in sedimentary rocks. Geophys. Surv. 5 (4), 369–380. doi:10.1007/BF01453987
Shcherbakov, V., and Sycheva, N. (2010). On the mechanism of formation of depositional remanent magnetization. Geochem. Geophys. Geosyst. 11 (2). doi:10.1029/2009GC002830
Spassov, S., and Valet, J.-P. (2012). Detrital magnetizations from redeposition experiments of different natural sediments. Earth Planet. Sci. Lett. 351, 147–157. doi:10.1016/j.epsl.2012.07.016
St-Onge, G., Mulder, T., Piper, D. J. W., Hillaire-Marcel, C., and Stoner, J. S. (2004). Earthquake and flood-induced turbidites in the Saguenay Fjord (québec): A Holocene paleoseismicity record. Quat. Sci. Rev. 23 (3–4), 283–294. doi:10.1016/j.quascirev.2003.03.001
Stacey, F. D. (1972). On the role of Brownian motion in the control of detrital remanent magnetization of sediments. Pure Appl. Geophys. 98 (1), 139–145. doi:10.1007/bf00875588
Stoner, Joseph S., Channell, J. E. T., and Hillaire-Marcel, C. (1996). The magnetic signature of rapidly deposited detrital layers from the Deep Labrador Sea: Relationship to North Atlantic Heinrich layers. Paleoceanography 11 (3), 309–325. doi:10.1029/96PA00583
Stoner, J. S., Channell, J. E. T., Hillaire-Marcel, C., and Kissel, C. (2000). Geomagnetic paleointensity and environmental record from labrador sea core MD95-2024: Global marine sediment and ice core chronostratigraphy for the last 110 kyr. Earth Planet. Sci. Lett. 183 (1–2), 161–177. doi:10.1016/S0012-821X(00)00272-7
Stoner, J., and St-Onge, G. (2007). Chapter three magnetic stratigraphy in paleoceanography: Reversals, excursions, paleointensity, and secular variation. Dev. Mar. Geol. 1, 99–138. doi:10.1016/S1572-5480(07)01008-1
Sun, W. W., and Kodama, K. P. (1992). Magnetic anisotropy, scanning electron microscopy, and X ray pole figure goniometry study of inclination shallowing in a compacting clay-rich sediment. J. Geophys. Res. 97 (B13), 19599–19615. doi:10.1029/92JB01589
Talling, P. J. (2021). Fidelity of turbidites as earthquake records. Nat. Geosci. 14 (3), 113–116. doi:10.1038/s41561-021-00707-2
Tanty, C., Valet, J.-P., Carlut, J., Bassinot, F., and Zaragosi, S. (2016). Acquisition of detrital magnetization in four turbidites. Geochem. Geophys. Geosyst. 17 (8), 3207–3223. doi:10.1002/2016GC006378
Tauxe, L. (1993). Sedimentary records of relative paleointensity of the geomagnetic field: Theory and practice. Rev. Geophys. 31 (3), 319–354. doi:10.1029/93rg01771
Tauxe, L., Steindorf, J. L., and Harris, A. (2006). Depositional remanent magnetization: Toward an improved theoretical and experimental foundation. Earth Planet. Sci. Lett. 244 (3), 515–529. doi:10.1016/j.epsl.2006.02.003
Valet, J.-P., and Fournier, A. (2003). Deciphering records of geomagnetic reversals. Rev. Geophys. 41 (1), 410–446. doi:10.1002/2015RG000506
Valet, J.-P., and Meynadier, L. (1993). Geomagnetic field intensity and reversals during the past four million years. Nature 366 (6452), 234–238. doi:10.1038/366234a0
Vautard, R., and Ghil, M. (1989). Singular spectrum analysis in nonlinear dynamics, with applications to paleoclimatic time series. Phys. D. Nonlinear Phenom. 35 (3), 395–424. doi:10.1016/0167-2789(89)90077-8
Velle, J. H., Walczak, M. H., Reilly, B., St-Onge, G., Stoner, J. S., Fallon, S., et al. (2022). High resolution inclination records from the Gulf of Alaska, IODP expedition 341 sites U1418 and U1419. Geophys. J. Int. 229 (1), 345–358. doi:10.1093/gji/ggab479
Verosub, K. L. (1977). Depositional and postdepositional processes in the magnetization of sediments. Rev. Geophys. 15 (2), 129–143. doi:10.1029/RG015i002p00129
Winterwerp, J. C. (1998). A simple model for turbulence induced flocculation of cohesive sediment. J. Hydraulic Res. 36, 309–326. doi:10.1080/00221689809498621
Zavala, C., and Arcuri, M. (2016). Intrabasinal and extrabasinal turbidites: Origin and distinctive characteristics. Sediment. Geol. 337, 36–54. doi:10.1016/j.sedgeo.2016.03.008
Keywords: natural remanent magnetisation, turbidite, hyperpycnite, sediment, detrital remanent magnetization, depositional remanence
Citation: Philippe ÉGH, Valet J-P, St-Onge G and Egli R (2022) Impact of turbulence on magnetic alignment in sediments. Front. Earth Sci. 10:1079229. doi: 10.3389/feart.2022.1079229
Received: 25 October 2022; Accepted: 28 November 2022;
Published: 12 December 2022.
Edited by:
Eric C. Ferre, University of Louisiana at Lafayette, United StatesReviewed by:
Leonardo Sagnotti, Istituto Nazionale di Geofisica e Vulcanologia (INGV), ItalyCarl Richter, University of Louisiana at Lafayette, United States
Copyright © 2022 Philippe, Valet, St-Onge and Egli. This is an open-access article distributed under the terms of the Creative Commons Attribution License (CC BY). The use, distribution or reproduction in other forums is permitted, provided the original author(s) and the copyright owner(s) are credited and that the original publication in this journal is cited, in accordance with accepted academic practice. No use, distribution or reproduction is permitted which does not comply with these terms.
*Correspondence: Édouard G. H. Philippe, egh.philippe@gmail.com