- 1Department of Earth Sciences, University of Cambridge, Cambridge, United Kingdom
- 2Division of Hydrologic Science, Desert Research Institute, Reno, NV, United States
Volatile metals are emitted at significant rates as gases and particulates from volcanoes, although their speciation, bioreactivity and longevity during atmospheric transport are essentially unknown. Ice cores provide detailed yet largely unexplored long-term records of volcanogenic volatile metals in air and precipitation. Here we evaluate the source and speciation of volatile metals (cadmium, lead, bismuth, and thallium) in Antarctic ice cores from the massive, halogen-rich and sulfur-poor ∼17.7 ka eruptions of Mt. Takahe, West Antarctic Rift. We show that these volatile, chalcophile metals were transported to the ice core as soluble aerosol, derived from magma degassing, in contrast to lithophile elements in the ice core that were transported as silicate ash. We use correlation analysis and chemical speciation modelling of the chlorine-rich volcanic plume to show that the volcanic metals cadmium, lead and bismuth were likely transported as water-soluble chloride aerosols in the atmosphere before they were scavenged from the plume by ice, water or ash and deposited onto the ice within 400 km of the vent. Our findings show that as well as recording trace metals sourced from much more distal regions, ice cores from Antarctica also record clear signatures of regional continental volcanism in the form of chloride aerosol.
Introduction
Volatile metals are emitted at significant rates as gases and particulates from volcanoes, although their speciation, bioreactivity and longevity during atmospheric transport are essentially unknown. Ice cores provide detailed yet largely unexplored long-term records of volcanogenic volatile metals in air and precipitation. Here we evaluate the source and speciation of volatile metals (cadmium, lead, bismuth, and thallium) in Antarctic ice cores from the massive, halogen-rich and sulfur-poor ∼17.7 ka eruptions of Mt. Takahe, West Antarctic Rift. We show that these volatile, chalcophile metals were transported to the ice core as soluble aerosol, derived from magma degassing, in contrast to lithophile elements in the ice core that were transported as silicate ash. We use correlation analysis and chemical speciation modelling of the chlorine-rich volcanic plume to show that the volcanic metals cadmium, lead and bismuth were likely transported as water-soluble chloride aerosols in the atmosphere before they were scavenged from the plume by ice, water or ash and deposited onto the ice within 400 km of the vent. Our findings show that as well as recording trace metals sourced from much more distal regions, ice cores from Antarctica also record clear signatures of regional continental volcanism in the form of chloride aerosol.
Volatile metals such as cadmium (Cd), bismuth (Bi), lead (Pb), and thallium (Tl), among other chalcophile metals e.g. mercury (Hg), gold (Au) and copper (Cu) are emitted into the atmosphere in gas and particulate form during volcanic eruptions at rates comparable to the industrial emissions of entire countries (Pennisi et al., 1988; Nriagu, 1989; Hinkley et al., 1999; Bagnato et al., 2014; Edmonds et al., 2018; Ilyinskaya et al., 2021). Emanation coefficients (Lambert et al., 1985; Pennisi et al., 1988) (the proportion of the metal in the volcanic gas phase over the silicate melt or magma) may be ∼70%–90% for Tl and Cd and ∼1% for Pb (Rubin, 1997; Gauthier and Le Cloarec, 1998; Aiuppa et al., 2000). Volcanoes in subduction zones, which tend to produce large explosive eruptions with the potential to impact global climate, produce gases and aerosols rich in elements carried by fluids devolatilized from subducting slabs (e.g., Tl, Bi, Pb, and As) (Cox et al., 2019), as well as metals whose solubility in silicate melts is optimised under oxidising conditions, e.g., Au (Botcharnikov et al., 2011), and those that tend to speciate with chlorine, a ligand inherently rich in “arc” melts and exsolved fluids (e.g., Pb, Bi, and Cd) (Edmonds et al., 2018; Zelenski et al., 2021).
After emission, volatile metal-bearing gases condense rapidly to form primary aerosol particulates (sulfates, chlorides, and oxides) (Hinkley, 1991; Mandon et al., 2019). These non-silicate volcanogenic particulates are comparable in size to desert dust (∼0.2 μm modal diameter (Kok et al., 2017; Mason et al., 2021)) and may adsorb to dust and other large particles. Secondary particles may form in the plume due to oxidation of gaseous SO2 to sulfate, which may form around existing particles in the plume, or may nucleate new particles, as observed for the Eyjafjallajokull (Iceland) volcanic plume (Boulon et al., 2011). Particulates may be lofted into the atmosphere and transported thousands of kilometres downwind, although this process is poorly understood or quantified. Volatile metals are then deposited at the surface, where they may accumulate in biological e.g., plants (Conti et al., 2020) and hydrological systems, including into groundwater systems and ultimately, drinking water supplies (Werner et al., 1997; Cuoco et al., 2013). Recent studies have shown that effusive basaltic eruptions at Kīlauea, Hawaii (Ilyinskaya et al., 2021), and Mt Etna, Italy (Brugnone et al., 2020) produce tropospheric volcanic plumes that deposit much of their aerosol-bound chalcophile metal load within a few hundred kilometres downwind of the vent (Brugnone et al., 2020; Ilyinskaya et al., 2021). The fate of volcanic volatile metals in the atmosphere from larger, explosive, stratospheric eruptions however, is unknown.
Volatile metals, if they are transported substantially in the atmosphere, may be deposited to geological archives such as glacier ice (Kellerhals et al., 2010) and sediments (Grasby et al., 2019), both as aerosol and in the silicate phase. Volatile metals in ice and sediments may be a useful tool to identify the sources of volcanic signatures, including those associated with mass extinctions in the geological record (Keller et al., 2020; Rakociński et al., 2021), although these archives have not yet been widely exploited. The chemical signatures of volcanism recorded in ice cores in terms of trace metals may be modified substantially from their “source” composition due to atmospheric processes such as scavenging by ice, water and ash phases during atmospheric transport and deposition (Textor et al., 2003; Moune et al., 2006; Ilyinskaya et al., 2021). Glacier ice archives have been exploited for their sulfate aerosol records of global explosive volcanism (Zielinski et al., 2002; Sigl et al., 2013) but are relatively unexplored for volcanogenic volatile metals. Cores drilled from glacier ice contain chemical signatures of volatile metals from both natural and anthropogenic sources e.g., Pb (McConnell et al., 2014). Natural sources of metals include windborne rock and soil dust, sea spray, forest fires, biogenic particulates, and volcanic activity (Nriagu, 1989). Emission sources may be local, for example volcanoes close to or under ice sheets, or thousands of kilometres distant from the deposition site in the case of most anthropogenic emissions and large tropical eruptions.
Previous studies investigating chemical species delivered to ice sheets as a result of anthropogenic activities have focused on metals such as Pb (McConnell et al., 2014; McConnell et al., 2019), Cd (Boutron et al., 1993; McConnell and Edwards, 2008), Tl (McConnell and Edwards, 2008; Kellerhals et al., 2010), mercury (Hg) (Schuster et al., 2002), Bi (Ferrari et al., 2000; Chellman et al., 2017), and copper (Cu) [e.g., (Boutron et al., 1995)]. Ice core Tl, Bi, Pb, and Cd concentrations may also be tracers of pre-industrial volcanic activity (Ferrari et al., 2000; Gabrielli et al., 2005; Kellerhals et al., 2010). Thallium peaks have been found in ice cores associated with large tropical eruptions with plume injection into the stratosphere such as the 1815 C.E. Tambora and 1883 C.E. Krakatoa (Indonesia) eruptions (Kellerhals et al., 2010). It has also been shown that during the last 26 ka (pre-industrial), between 50%–95% of Bi measured in Central Greenland ice cores was supplied by volcanism, although it is unknown how much of this may have come from local Icelandic volcanism (Ferrari et al., 2000).
High concentrations of volcanogenic chemical species in ice cores are typically transient in that they are associated with local or distal eruptions that produce emissions for a limited time (e.g., months to a few years). However, sustained delivery of trace elements at lower levels due to quiescent degassing of volcanoes around the world may also make an important contribution to concentrations of volatile metals measured in ice cores, e.g., zinc (Zn), Cu, Cd, indium (In), Pb, antimony (Sb), and selenium (Se) (Weiss et al., 1978; Matsumoto and Hinkley, 2001). A more complete understanding of volcanic metals recorded in polar ice relies on knowledge of volatile metal contributions from local Antarctic or Arctic volcanism, as well as the processes that may alter chemical signatures during atmospheric transport from the emission source to the deposition site. Significant knowledge gaps remain as to how volatile metals are speciated during atmospheric transport from volcanic sources, the particle size distribution and residence time of metals in the atmosphere (Dusek et al., 2006), and how transport at different altitudes (e.g., stratospheric versus tropospheric) may affect these processes and ultimately, their preservation potential in ice cores (Hong et al., 2003).
Here, the transport and deposition of volatile metals is examined using detailed geochemical data from ice cores recording a ∼192-year series of halogen-rich eruptions from Mt. Takahe, an alkaline volcano on the West Antarctic Rift System (WARS). Through modeling, we establish the speciation of the volatile metals at- and near-source, and assess the potential for long-distance global transport of volcanic volatile metals and the implications for the interpretation of ice records of volcanism.
Volcanism in the West Antarctic Rift System
The WARS is one of the most extensive regions of stretched continental crust on Earth, with at least 138 volcanoes located along its length, and is thought to have been intermittently active since the Cenozoic and into the Holocene (LeMasurier, 1990) (Figure 1A). Lava compositions from subaerial outcrops along the WARS are typically of intermediate alkaline composition, with less common instances of alkali basaltic or more evolved eruptions (e.g., trachytic, rhyolitic) (de Vries et al., 2018) (Supplementary Figure S1). Compared to subalkalic basaltic volcanic gas plumes, only a small number of alkaline volcanic gas plumes have been sampled directly for their source metal emissions: at Mt. Erebus, Antarctica (Wardell et al., 2008), and Nyiragongo, Democratic Republic of the Congo (Calabrese et al., 2014). Alkaline intraplate magmas are typically chlorine-rich, which may produce higher concentrations of gaseous volatile metals than subalkalic basaltic intraplate volcanoes due to the higher availability of chloride ligands in the exsolved fluids (Mandon et al., 2019), although this has not yet been demonstrated.
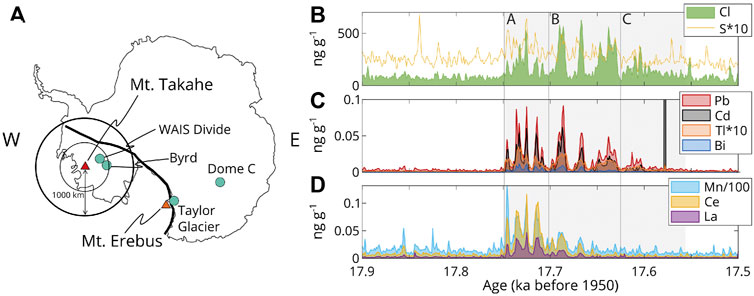
FIGURE 1. Mt. Takahe and the 17.7 ka eruption sequence, lasting 192 years. (A) Location of Mt. Takahe and Mt. Erebus on the West Antarctic Rift System (WARS; approximate location shown by solid black line). Ice core drilling locations are shown as blue circles. (B–D): Concentrations (in ng g−1, uncorrected for sea-salt or background) of elements measured in the WAIS divide ice core (McConnell et al., 2017). The eruption sequence is divided into stages A–C, as described in the text.
Mount Takahe (76.28°S, 112.08°W, and 3460 m a.s.l.) is a volcano on an incipient rift in Marie Byrd Land, Antarctica surrounded by the West Antarctic Ice Sheet (Figure 1A). Tephra studies have identified its magma composition as peralkaline trachyte, and suggest alternating subaerial magmatic and hydrovolcanic eruptions from 30 to 20 thousand years before present (ka B.P.), followed by a sustained period of hydrovolcanic eruptions from 20 to 14 ka B.P. (Palais et al., 1988; McConnell et al., 2017). During the latter period, eruptive activity from Mt. Takahe was most intense between 17.748 and 17.556 ka B.P., with a 192-year series of massive, halogen-rich volcanic eruptions that have been documented in the West Antarctic Ice Sheet Divide (WAIS Divide, or WD, ∼360 km distant), Byrd (∼450 km distant), Taylor Glacier (∼1300 km distant) and Dome C (∼2800 km distant) ice cores (Hammer et al., 1997; Gabrielli et al., 2005; McConnell et al., 2017). The 17.7 ka Mt. Takahe eruption sequence is unique in the ∼68 ka WD ice core record in terms of both halogen concentrations [chlorine (Cl), fluorine (F)], and volatile metals such as Cd, Pb, Tl, and Bi (Gabrielli et al., 2005; McConnell et al., 2017). Sulfur isotope anomalies (i.e., mass independent fractionation) indicate that eruption plumes reached the stratosphere, and marked decreases in ice core bromine concentrations, consistent with increased surface UV radiation, suggest that they led to stratospheric ozone depletion (McConnell et al., 2017).
Methods
Unless otherwise indicated, all ice core concentrations used here for the Mt. Takahe 17.7 ka event are from McConnell et al. (2017) (all data may be found in Supplementary Data Sheet S1), which also includes measurements from the Byrd ice core, 450 km distant from Mt. Takahe, and the Taylor Glacier and Dome C ice cores, which all show chemical signatures associated with the 17.7 ka event. Analyses were conducted on longitudinal samples (∼100 mm by ∼33 mm by ∼33 mm) using the Desert Research Institute’s continuous-flow analysis with trace elements analytical system, including two Element2 (Thermo Scientific) high-resolution inductively coupled plasma mass spectrometers (HR-ICP-MS) operating in parallel for measurement of a broad range of ∼35 elements. Uncertainties in the ice core measurements were discussed in detail by McConnell et al. (2017). Comparisons of replicate measurements from within the WD core and between the WD and Byrd ice cores (McConnell et al., 2017) indicate that results were highly repeatable for nearly all elements, despite the extremely low concentration levels (as low as 20 fg g−1) for elements such as the low-boiling-point heavy metals (bismuth, cadmium, and thallium) and REE (lanthanum, cerium, and europium), and despite the distance between the WD and Byrd coring sites. Under-recovery of more recalcitrant elements such as Al and Fe contained in larger insoluble dust particles is implicit in continuous methods (McConnell et al., 2007; Rhodes et al., 2011) but does not impact measurements of heavy metals or other elements associated with gaseous volcanic emissions that generally coat the outside of insoluble particles because of the addition of ultra-pure nitric acid to the sample flow stream immediately after the melter head. The enrichments in trace metals during the 17.7 ka Mt Takahe event were very large (e.g., ∼5 to ∼25 for Tl, ∼40 to ∼1500 for Cd) for all four heavy metals so small differences in recovery during the continuous measurements would have little impact on our overall findings (Arienzo et al., 2019).
Where volatile metals are shown with the prefix “enr”, they represent enrichments relative to Ce (a crustal dust tracer) (Supplementary Figure S2) using “mean sediment” ratios (Bowen, 1979)
Where the prefix “ex” is used, non-crustal or excess metal concentrations have been determined by subtracting estimated crustal contributions (McConnell et al., 2019). Instead of ash, the proportion of an element’s concentration derived from crustal sources is calculated using the measured Ce concentration and “mean sediment” ratios from Bowen (1979). This approach assumes that volatile metals in ice are from crustal and volcanic sources only, which is reasonable as these are the major natural sources of these elements globally (Nriagu, 1989) and anthropogenic pollution was negligible in ice cores in pre-industrial times (i.e., during the 17.7 ka Mt. Takake eruptions).
An additional adjustment is applied here to the Cl data to remove “background” or non-volcanic sources of Cl. An average background Cl concentration is approximated using part of the time series outside of the 192-year eruption period, specifically from 17.97 to 17.76 ka. This yields a background Cl concentration of 94 ± 27 ng g−1. Where this background concentration has been removed from the measured Cl concentrations, data are denoted as nbgCl (non-background Cl). Note that there were no significant changes in sea spray sources of Cl as shown by relatively constant levels of sodium (Na) during this period, with Na in WAIS Divide ice derived largely from sea spray.
Chemical speciation modelling
The Gibbs free energy minimisation (GEM) module of HSC Chemistry (version 9.9.2, Outotec Research Oy, Finland) was used to model gas-phase speciation in volcanic plumes. Equilibrium speciation models solve a series of mass balance and mass action equations to determine the species present at a given temperature and pressure. Detailed descriptions of the model are provided in previous works (Symonds et al., 1994). Model inputs are molar gas concentrations of major and trace species (kmol or mol%), pressure (bar), temperature (°C), and the output phases expected in the plume.
The equilibrium speciation results calculated in HSC chemistry depend strongly on the input major and trace H-, C-, S-, O- and halide-bearing gases. Ideally, the inputs of these gases would be as close to the real composition in the plume as possible. However, measurements of these gases other than H2O, CO2, SO2, H2S, and hydrogen halides are rarely made at exactly the same time as trace metal and metalloid measurements. Major gas compositions are not available for Mt. Takahe; therefore the model assumes the composition of the passive degassing from Mt. Erebus (Oppenheimer et al., 2011) (H2O, CO2, CO, H2, SO2, H2S, CO, OCS, and S2; Supplementary Table S1); and S/Cl (Supplementary Figure S3a) and Cl/F (Supplementary Figure S4) ratios from ice core measurements for the 17.7 ka Mt. Takahe event are used to calculate HCl gas and HF gas input concentrations, respectively. All ratios are corrected based on the assumption that ∼25% of Cl versus ∼80% of S reaches the stratosphere (Textor et al., 2003). Comparable amounts of F and Cl are assumed to reach the stratosphere, i.e., the Cl/F ratio is not altered. Metal-to-Cl ratios for the 17.7 ka event (Figure 2) are then used to calculate input concentrations of Bi, Cd, Pb, and Tl (Supplementary Figure S3b–e). Metal input concentrations were not corrected for contributions from ash as these already have been taken into account by a correction relative to Ce, and ash contributions for the volatile metals considered here are typically low. All ratios are taken from stage A of the eruption sequence, as labelled in Figure 1.
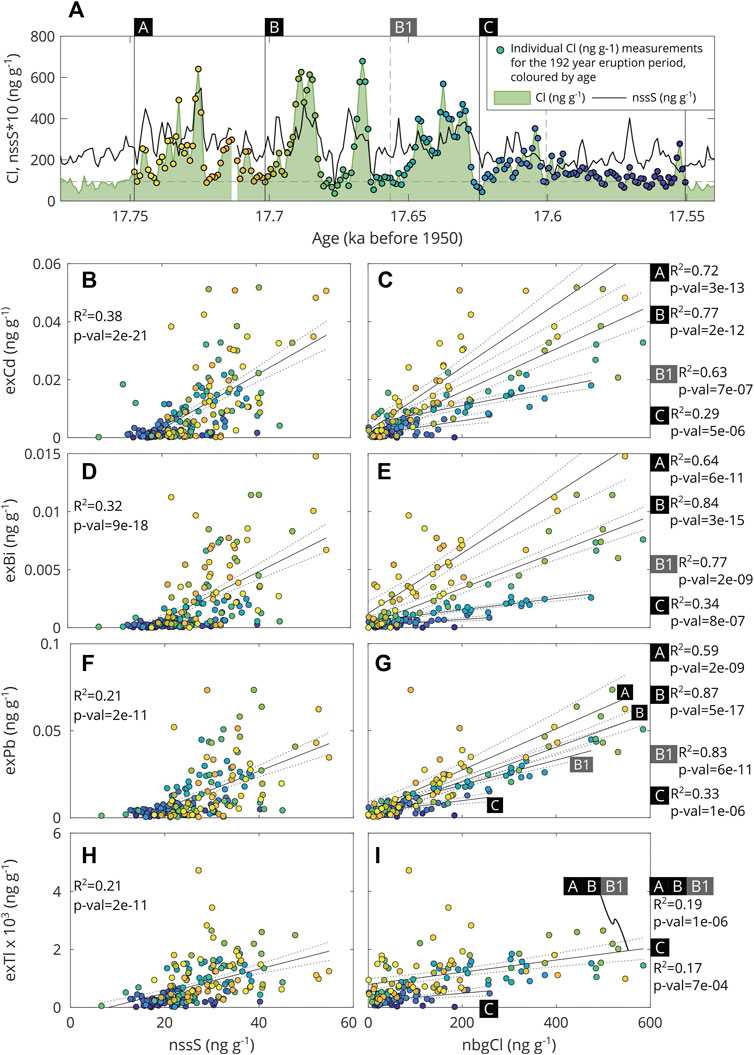
FIGURE 2. Correlations between volcanogenic metals and ligand-forming elements (S, Cl) in the ice core record linked to the 17.7 ka Mt. Takahe event. All data are from McConnell et al. (2017). (A): Reference timeseries showing the concentrations of non-sea-salt S (nssS, black line) and Cl (green area) over time. The horizontal grey dashed line represents the background Cl value (94 ppm) used to calculate non-background Cl (nbgCl; as described in text). Data points that fall within the 192-year eruption period are shown as circular data points, coloured by their age. These coloured data points are the same as those used in (B–I). (B–I) compare the concentrations of metals Cd, Bi, Pb, and Tl to S and Cl concentrations. The “ex” prefix denotes concentrations in excess of crustal sources, i.e., the crustal component as been estimated using the crustal tracer Ce and subtracted from the total. The solid black line represents a linear regression model to which the values given for each Fig (R2, p-value) are derived. Dashed black lines represent the 95% confidence bounds of the fit. Separate regressions are only given for time periods A, B, and C where 95% confidence bounds do not fully overlap for at least two of the time periods. Due to variability within stage B, two different ratios have been calculated. For exPb, one value at ∼0.2 ng g−1 is omitted from the Figure to make the other data points easier to distinguish, and also is excluded from the regression.
In the models presented here, all gas phases available in HSC chemistry for the input elements in the model are included as expected phases, except organic phases, which are unstable at high temperatures. Only gaseous species with thermodynamic properties known at more than ∼600°C are used; i.e., this is the limit to which extrapolation beyond known thermodynamic properties is allowed in the model. For comparison, a model for a basaltic continental rift volcano, Erta ‘Ale (Ethiopia) is also presented, over the same range of temperature and atmospheric mixing, based on data from Symonds et al. (1994). All inputs to this model are given in Supplementary Table S1 (Symonds et al., 1994).
The equilibrium speciation is modelled during the addition of air immediately after emission at the lava-air interface. In this case the magmatic plume is simplified to a 52:47 mixture of H2O and CO2 (to match that used in the Mt. Takahe model), and air is a 78:21:1 mixture of N2, O2, and Ar. Air addition is modelled between atmospheric (VA) to magmatic (VM) gas ratios between 0 and 0.33 (at increments of 0.01). The temperature of the gas mixture at the range of mixing ratios considered (0<(VA/VM)<0.33) is determined using a simple fluid mixing model (Mason et al., 2021):
where
In order to be able to directly relate the literature major gas species compositions to those measured in the studies presented here, element to Cl (X/Cl) are scaled to the HCl concentration used in the model input compositions, as follows:
Where
Chemical features of the 17.7 ka Mt. Takahe eruption sequence
The WD ice core records of volatiles (S and chlorine Cl), volatile metals (Cd, Bi, Tl and Pb) and refractory elements [manganese (Mn), cerium (Ce), lanthanum (La)] are shown in Figures 1B–D. To aid interpretation, the eruption sequence, which spans 192 years, has been divided into three stages (A–C; Figure 1). Stage A contains high concentrations of Cl, refractory (non-volatile) elements (Mn, La, and Ce) and volatile metals (McConnell et al., 2017; Zelenski et al., 2021), which we interpret to reflect that this stage of the eruption was characterised by plumes rich in both silicate material (ash), which hosted the refractory elements, as well as soluble aerosol (which hosted the Cl and S and a fraction of the volatile metals). In stage B, Cl and volatile metal concentrations remain high, but concentrations of refractory elements decrease, consistent with ash being less abundant but plumes remaining rich in aerosol. In stage C, concentrations of Cl, refractory elements and volatile metals all decrease relative to stages A and B, while S concentrations remain at a relatively low, broadly similar level.
The average S/Cl mass ratio for the full eruption sequence is 0.13 ± 0.05 (mean ±1 SD), and is relatively consistent throughout the eruption sequence for non-background Cl (nbgCl) values greater than 150 ng g−1 (Supplementary Figure S3a). This ratio is at the low end of measured values when compared to a global compilation of volcanic gas S/Cl ratios, including Mt. Erebus, for which S/Cl ratios range from 0.2 to 3.2 (Wardell et al., 2008; Aiuppa, 2009), although these ratios are comparable to other alkaline volcanic systems (Zelenski et al., 2013). We would, however, not expect the S/Cl ratio preserved in the ice core to faithfully record the same ratio in volcanic gases. Volcanic SO2 in gaseous form is slowly oxidised to sulfate in the stratosphere (Bekki, 1995; Robock, 2000). Sulfate has a much longer lifetime than gaseous SO2 and will be dispersed in the stratosphere/atmosphere before being deposited onto the ice, whereas HCl is scavenged without any chemical transformation. During hydrovolcanic activity, external ice-water may modulate pressure at the vent as well as interact directly with magmatic gases. The production of fine hydrovolcanic ash may also promote chemical scavenging processes on ash surfaces by adsorption (Ayris et al., 2013; Rowell et al., 2021). Large explosive eruptions, such as the early ash-producing eruptions associated with the 17.7 ka event, may generate rapidly ascending plumes that can reach heights in excess of 30–40 km, although the eruption column heights for this series of eruptions are unconstrained. It has been shown from other studies that during ascent through the lower atmosphere acidic species may be removed from the plume through scavenging by water or ice (Textor et al., 2003). This process preferentially removes HCl (up to 75% of the total) over SO2 (∼20%) (Halmer et al., 2002). Further, it has been shown that the amount of HCl gas reaching the atmosphere is limited by adsorption to ash to a greater extent than for S-bearing gases (Pinto et al., 1989). As a result of these scavenging processes, Cl may be associated with larger particles, which fall out of the plume more rapidly under gravity, thereby reducing the residence time of Cl in the atmosphere.
Correlations between the volcanic volatile metals with potential ligands of S and Cl provide clues about metal speciation in the volcanic plume (Moune et al., 2010). During the early stages of the eruption sequence, Cd, Bi, and Pb display stronger correlations with Cl than with S, and the strength of the correlation between these metals and Cl decreases over time (R2∼0.6–0.8 in stages A and B versus R2∼0.3 in stage C; Figure 2). For Tl the correlations are weaker, and similar for S and Cl (R2∼0.2). Ratios of Cd/Cl, Bi/Cl and Pb/Cl also decrease through the eruption sequence, while there is no discernible change in Tl/Cl ratios (Supplementary Figure S3). Ratios of Cd, Bi, Pb, and Tl to Cl in the Mt. Takahe eruption sequence are comparable, to within an order of magnitude, to emissions from other basaltic volcanoes (Supplementary Figure S5). Cd/Cl and Pb/Cl are at the lower end of values measured in the gases and particulates emitted from Mt. Erebus (Wardell et al., 2008) (Tl and Bi measurements of volcanic gases are not available for Mt. Erebus). It is therefore plausible that the ratios observed in the ice core are comparable to volcanic gas emissions; however without direct source measurements it is impossible to state this conclusively. In contrast to metal-to-Cl ratios, metal-to-S ratios do not appear to change significantly through the early stages of the eruption (A and B); however, there is an indication that they decrease in the later stages of the eruption sequence in stage C (and perhaps also towards the end of stage B; Supplementary Figure S3a). The data therefore suggest that Cd, Bi, and Pb are speciated dominantly as chloride, whereas Tl does not appear to be dominantly speciated as either chloride or sulfate.
A chemical speciation model for Mt. Takahe volcanic gas emissions
The speciation of volatile trace elements during degassing provides key initial conditions for the subsequent atmospheric transport, solubility, deposition and resulting hazard from these elements (Ilyinskaya et al., 2021). Major elements—such as S, oxygen (O), hydrogen (H) and Cl (and other halogens)—are the main ligand-forming elements for volatile trace metals and metalloids in volcanic gas emissions, e.g., as sulfates (
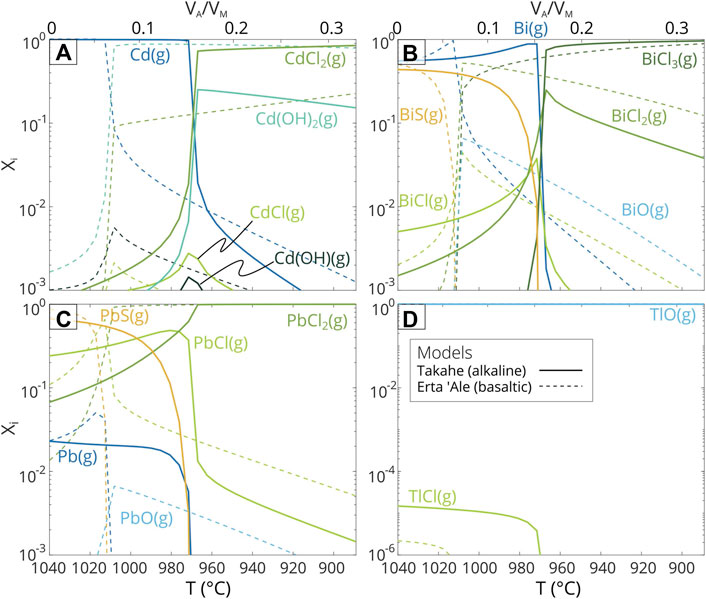
FIGURE 3. Chemical speciation results for Mt. Takahe and Erta ‘Ale. Figs (A–D) show changes in speciation for concurrent mixing between VA/VM=0 and 0.33 and cooling between 1040 and 889°C (as calculated by the mixing model outlined in Methods). Species are shown as mixing ratios (Xi) representing the contribution each species makes to the total concentration of each element. Note the different y-axis scale for Tl (D). Input and output data are in Supplementary Data Sheet S1.
In the model simulations, Cd, Bi, and Pb are dominantly present as gaseous chloride species (Figure 3), in agreement with correlation analysis of our ice core observations (Figure 2). Tl is again an exception, with the vast majority of it present as TlO gas in the model (Figure 3D). The increase in concentrations of metal-chloride complexes after mixing between volcanic gases and atmosphere has also been observed in speciation models for basaltic ocean island volcanoes e.g., Kīlauea Volcano (Mason et al., 2021) and is thought to be caused by an increase in reactive halide species during atmospheric mixing (Gerlach, 2004). Here, a comparison model using compositions from Erta ‘Ale, a basaltic volcano located on the East African Rift, Ethiopia (Zelenski et al., 2013), is also shown (Figure 3; Supplementary Data Sheet S1). Due to the higher oxygen fugacity (SupplementaryTable S1) of the Erta ‘Ale volcanic gases, the compositional discontinuity occurs after less atmospheric mixing than for Mt. Takahe. Concentrations of metal-chloride complexes also increase with atmospheric mixing for Erta’ Ale, with chloride species dominant for Bi and Pb. For Cd; however, the dominant chemical species is Cd(OH)2 gas, and PbO and BiO gases are more abundant than for Mt. Takahe. Comparatively higher concentrations of metal-chloride gas species at Mt. Takahe are likely to be driven by higher Cl concentrations in alkaline magmas (Mandon et al., 2019).
Implications for volcanogenic volatile metals in ice core archives
The 17.7 ka sequence of halogen-rich eruptions at Mt Takahe in West Antarctica preserved in the ice core allows quantification of the deposition of metal-bearing chloride aerosols within 400 km of the volcanic source (Pinto et al., 1989; Textor et al., 2003). We show here, by analysis and correlation statistics of Antarctic ice core geochemistry and by gas phase speciation modelling, that volatile metals such as Cd, Bi, and Pb are likely transported in the atmosphere as chloride aerosols in volcanic plumes and clouds. Our interpretations are consistent with previous work: chloride is reported as the most efficient ligand at transporting metals Cu, Bi, Zn, Pb at White Island (New Zealand) and other subduction zone volcanoes (Mandon et al., 2019; Zelenski et al., 2021); although at Masaya (Nicaragua), correlation analysis may suggest that Tl, Bi, Cu, and Te appear to have more affinity with S than with Cl (Moune et al., 2010). Proximal deposition of metal-chloride complexes might be a ubiquitous feature of volcanic eruption plumes. Prolonged stratospheric transport of volcanic clouds over larger spatial and temporal scales from explosive tropical eruptions may lead to the majority of volcanic chloride aerosol being lost between the source and Antarctic or Greenland ice sheets, giving rise to the typical ice core sulfate signals we observe associated with these eruptions, which are not accompanied by large chloride signals (Delmas et al., 1992).
In contrast however, speciation and correlation analysis results indicate that Tl may have less affinity for Cl, which may extend its atmospheric residence time, perhaps suggesting that it is a more promising tracer of distal (global) volcanism, although further study of Tl behaviour in volcanic plumes is warranted. Further, metals known to complex with sulfate [possibly tellurium (Te), copper (Cu), cesium (Cs), rubidium (Rb) sulfates] based on correlation analysis (Aiuppa et al., 2000; Moune et al., 2010); and Zn sulfate based on SEM analysis (Mandon et al., 2019) may also have longer residence times in the atmosphere as they are not expected to be scavenged as extensively as chloride complexes. Previous work may support this hypothesis. A study of volcanogenic metals in the Illimani ice core, Bolivia, associated with massive explosive eruptions such as Tambora, 1815 A.D. and Krakatoa, 1883 A.D. in Indonesia observed enrichments in Tl (as well as sulfate and fluoride) but no enrichment in Cl, Cd, Pb, and Bi relative to crustal sources (dust) (Kellerhals et al., 2010). We note however that some studies have postulated that the ratio of Pb, Cd, In, and Tl in pre-industrial Antarctic ice directly reflects input from tropospheric, passively degassing volcanoes (Matsumoto and Hinkley, 2001). Studies of Himalayan ice cores (Xu et al., 2009) and the Mount Logan ice core (Yukon Territory, Canada) (Osterberg et al., 2014) have linked sulfate spikes relating to known eruptions with spikes in Bi concentrations, although it is not clear whether the Bi was transported to the ice as soluble aerosol, or as fine ash.
We note that the dry, cold conditions in the Antarctic atmosphere may have extended the atmospheric residence times of volcanogenic halogens and metals, particularly during the dark winter months where reactions relying on UV radiation would have been limited (McConnell et al., 2017). This may have been particularly relevant for the 17.7 ka eruption of Mt. Takahe which occurred during the extremely dry and cold conditions of the last glacial maximum. These extreme conditions, along with the metal- and halogen-rich nature of alkaline volcanism, may act together to deliver high concentrations of metal-chlorides to the Antarctic ice. Local continental volcanism may be an important contributor to Antarctic ice and the metal assemblage associated with local Antarctic volcanism may be quite different than that associated with sulfate-rich large tropical eruptions. Indeed, this is clear in Antarctic ice cores where no Pb, Tl, Bi, or Cd anomalies are associated with well-known tropical eruptions such as Tambora (Vallelonga et al., 2003). As many ice core drilling locations are located close to the WARS (Figure 1A), the geochemical contribution from local volcanoes should always be considered carefully.
Data availability statement
The original contributions presented in the study are included in the article/Supplementary Material, further inquiries can be directed to the corresponding author.
Author contributions
EM analysed data provided by JM and conducted model simulations. EM, ME, and JM wrote the manuscript.
Funding
EM was funded by an UKRI EPSRC studentship during this work. The WD ice core was analysed at the Desert Research Institute with funding from United States National Science Foundation to JRM (grants 0538427, 0839093, and 1142166). Clare Hall, Cambridge, provided additional support to JRM through the Sir Nicholas Shackleton Fellowship.
Acknowledgments
ME acknowledges UKRI funding through the NERC grant V-Plus NE/S00436X/1.
Conflict of interest
The authors declare that the research was conducted in the absence of any commercial or financial relationships that could be construed as a potential conflict of interest.
Publisher’s note
All claims expressed in this article are solely those of the authors and do not necessarily represent those of their affiliated organizations, or those of the publisher, the editors and the reviewers. Any product that may be evaluated in this article, or claim that may be made by its manufacturer, is not guaranteed or endorsed by the publisher.
Supplementary material
The Supplementary Material for this article can be found online at: https://www.frontiersin.org/articles/10.3389/feart.2022.1002366/full#supplementary-material
References
Aiuppa, A., Allard, P., D’Alessandro, W., Michel, A., Parello, F., Treuil, M., et al. (2000). Mobility and fluxes of major, minor and trace metals during basalt weathering and groundwater transport at Mt. Etna volcano (Sicily). Geochimica Cosmochimica Acta 64, 1827–1841. doi:10.1016/s0016-7037(00)00345-8
Aiuppa, A. (2009). Degassing of halogens from basaltic volcanism: Insights from volcanic gas observations. Chem. Geol. 263, 99–109. doi:10.1016/j.chemgeo.2008.08.022
Arienzo, M. M., McConnell, J. R., Chellman, N., and Kipfstuhl, S. (2019). Method for correcting continuous ice-core elemental measurements for under-recovery. Environ. Sci. Technol. 53, 5887–5894. doi:10.1021/acs.est.9b00199
Ayris, P., Lee, A., Wilson, K., Kueppers, U., Dingwell, D., and Delmelle, P. (2013). SO2 sequestration in large volcanic eruptions: High-temperature scavenging by tephra. Geochimica Cosmochimica Acta 110, 58–69. doi:10.1016/j.gca.2013.02.018
Bagnato, E., Barra, M., Cardellini, C., Chiodini, G., Parello, F., and Sprovieri, M. (2014). First combined flux chamber survey of mercury and CO2 emissions from soil diffuse degassing at Solfatara of Pozzuoli crater, Campi Flegrei (Italy): Mapping and quantification of gas release. J. Volcanol. Geotherm. Res. 289, 26–40. doi:10.1016/j.jvolgeores.2014.10.017
Bekki, S. (1995). Oxidation of volcanic SO2: A sink for stratospheric OH and H2O. Geophys. Res. Lett. 22, 913–916. doi:10.1029/95gl00534
Botcharnikov, R. E., Linnen, R. L., Wilke, M., Holtz, F., Jugo, P. J., and Berndt, J. (2011). High gold concentrations in sulphide-bearing magma under oxidizing conditions. Nat. Geosci. 4, 112–115. doi:10.1038/ngeo1042
Boulon, J., Sellegri, K., Hervo, M., and Laj, P. (2011). Observations of nucleation of new particles in a volcanic plume. Proc. Natl. Acad. Sci. U. S. A. 108, 12223–12226. doi:10.1073/pnas.1104923108
Boutron, C. F., Candelone, J.-P., and Hong, S. J. S. O. T. T. E. (1995). Greenland snow and ice cores: Unique archives of large-scale pollution of the troposphere of the northern hemisphere by lead and other heavy metals. Sci. Total Environ. 160, 233–241. doi:10.1016/0048-9697(95)04359-9
Boutron, C. F., Rudniev, S. N., Bolshov, M. A., Koloshnikov, V. G., Patterson, C. C., Barkov, N. J. E., et al. (1993). Changes in cadmium concentrations in Antarctic ice and snow during the past 155, 000 years. Earth Planet. Sci. Lett. 117, 431–441. doi:10.1016/0012-821x(93)90095-q
Brugnone, F., D’Alessandro, W., Calabrese, S., Li Vigni, L., Bellomo, S., Brusca, L., et al. 2020. A Christmas gift: Signature of the 24th December 2018 eruption of Mt. Etna on the chemical composition of bulk deposition in eastern Sicily. 139, 341–358, doi:10.3301/ijg.2020.08
Calabrese, S., Scaglione, S., Milazzo, S., D'Alessandro, W., Bobrowski, N., Giuffrida, G. B., et al. (2014). Passive degassing at nyiragongo (DR Congo) and Etna (Italy) volcanoes. Gill 57.
Chellman, N., McConnell, J. R., Arienzo, M., Pederson, G. T., Aarons, S. M., and Csank, A. (2017). Reassessment of the upper fremont glacier ice-core chronologies by synchronizing of ice-core-water isotopes to a nearby tree-ring chronology. Environ. Sci. Technol. 51, 4230–4238. doi:10.1021/acs.est.6b06574
Conti, M. E., Plà, R., Simone, C., Jasan, R., Finoia, M. G. J. E. S., and Research, P. (2020). Implementing the monitoring breakdown structure: Native lichens as biomonitors of element deposition in the southern patagonian forest connected with the puyehue volcano event in 2011—a 6-year survey (2006–2012). Biomonitors, 1–16.
Cox, D., Watt, S. F., Jenner, F. E., Hastie, A. R., and Hammond, S. J. (2019). Chalcophile element processing beneath a continental arc stratovolcano. Earth Planet. Sci. Lett. 522, 1–11. doi:10.1016/j.epsl.2019.06.017
Cuoco, E., Spagnuolo, A., Balagizi, C., De Francesco, S., Tassi, F., Vaselli, O., et al. (2013). Impact of volcanic emissions on rainwater chemistry: The case of Mt. Nyiragongo in the virunga volcanic region (DRC). J. Geochem. Explor. 125, 69–79. doi:10.1016/j.gexplo.2012.11.008
De Vries, M. V. W., Bingham, R. G., and Hein, A. S. J. G. S. (2018). A new volcanic province: An inventory of subglacial volcanoes in West Antarctica. Lond. Spec. Publ. 461, 231–248. doi:10.1144/sp461.7
Delmas, R. J., Kirchner, S., Palais, J. M., and Petit, J. R. J. T. B. (1992). 1000 years of explosive volcanism recorded at the South Pole. Tellus B 44, 335–350. doi:10.1034/j.1600-0889.1992.00011.x
Dusek, U., Frank, G. P., Hildebrandt, L., Curtius, J., Schneider, J., Walter, S., et al. (2006). Size matters more than chemistry for cloud-nucleating ability of aerosol particles. Science 312, 1375–1378. doi:10.1126/science.1125261
Edmonds, M., Mather, T. A., and Liu, E. J. (2018). A distinct metal fingerprint in arc volcanic emissions. Nat. Geosci. 11, 790–794. doi:10.1038/s41561-018-0214-5
Ferrari, C., Hong, S., van De Velde, K., Boutron, C., Rudniev, S., Bolshov, M., et al. (2000). Natural and anthropogenic bismuth in Central Greenland. Atmos. Environ. X. 34, 941–948. doi:10.1016/s1352-2310(99)00257-5
Gabrielli, P., Barbante, C., Boutron, C., Cozzi, G., Gaspari, V., Planchon, F., et al. (2005). Variations in atmospheric trace elements in Dome C (East Antarctica) ice over the last two climatic cycles. Atmos. Environ. X. 39, 6420–6429. doi:10.1016/j.atmosenv.2005.07.025
Gauthier, P.-J., and le Cloarec, M.-F. (1998). Variability of alkali and heavy metal fluxes released by Mt. Etna volcano, Sicily, between 1991 and 1995. J. Volcanol. Geotherm. Res. 81, 311–326. doi:10.1016/s0377-0273(98)00002-x
Gerlach, T. (2004). Volcanic sources of tropospheric ozone‐depleting trace gases. Geochem. Geophys. Geosyst. 5. doi:10.1029/2004gc000747
Giggenbach, W. F. (1980). Geothermal gas equilibria. Geochimica cosmochimica acta 44, 2021–2032. doi:10.1016/0016-7037(80)90200-8
Grasby, S. E., Them, T. R., Chen, Z., Yin, R., and Ardakani, O. H. J. E.-S. R. (2019). Mercury as a proxy for volcanic emissions in the geologic record, 196, 102880.
Halmer, M., Schmincke, H.-U., and Graf, H.-F. (2002). The annual volcanic gas input into the atmosphere, in particular into the stratosphere: A global data set for the past 100 years. J. Volcanol. Geotherm. Res. 115, 511–528. doi:10.1016/s0377-0273(01)00318-3
Hammer, C., Clausen, H., and Langway, C. J. C. C. 1997. 50, 000 years of recorded global volcanism. 35, 1–15.
Hinkley, T. K. (1991). Distribution of metals between particulate and gaseous forms in a volcanic plume. Bull. Volcanol. 53, 395–400. doi:10.1007/bf00280229
Hinkley, T. K., Lamothe, P. J., Wilson, S. A., Finnegan, D. L., and Gerlach, T. M. (1999). Metal emissions from Kilauea, and a suggested revision of the estimated worldwide metal output by quiescent degassing of volcanoes. Earth Planet. Sci. Lett. 170, 315–325. doi:10.1016/s0012-821x(99)00103-x
Hong, S., Kim, Y., Boutron, C. F., Ferrari, C. P., Petit, J. R., Barbante, C., et al. (2003). Climate‐related variations in lead concentrations and sources in Vostok Antarctic ice from 65, 000 to 240, 000 years BP. Vostok, 30.
Ilyinskaya, E., Mason, E., Wieser, P. E., Holland, L., Liu, E. J., Mather, T. A., et al. (2021). Rapid metal pollutant deposition from the volcanic plume of Kīlauea, Hawai’i. Commun. Earth Environ. 2, 78–15. doi:10.1038/s43247-021-00146-2
Keller, G., Mateo, P., Monkenbusch, J., Thibault, N., Punekar, J., Spangenberg, J. E., et al. (2020). Mercury linked to Deccan Traps volcanism, climate change and the end-Cretaceous mass extinction. Glob. Planet. Change 194, 103312. doi:10.1016/j.gloplacha.2020.103312
Kellerhals, T., Tobler, L., BrüTsch, S., Sigl, M., Wacker, L., GäGgeler, H. W., et al. (2010). Thallium as a tracer for preindustrial volcanic eruptions in an ice core record from Illimani. Bolivia 44, 888–893.
Kok, J. F., Ridley, D. A., Zhou, Q., Miller, R. L., Zhao, C., Heald, C. L., et al. (2017). Smaller desert dust cooling effect estimated from analysis of dust size and abundance. Nat. Geosci. 10, 274–278. doi:10.1038/ngeo2912
Lambert, G., le Cloarec, M., Ardouin, B., and le Roulley, J. (1985). Volcanic emission of radionuclides and magma dynamics. Earth Planet. Sci. Lett. 76, 185–192. doi:10.1016/0012-821x(85)90158-x
Lemasurier, W. (1990). Late cenozoic volcanism on the antarctic plate: An overview. Volcanoes Antarct. plate South. oceans 48, 1–17.
Mandon, C. L., Christenson, B. W., Schipper, C. I., Seward, T. M., Garaebiti, E. J. J. O. V., and Research, G. (2019). Metal transport in volcanic plumes: A case study at white island and yasur volcanoes. J. Volcanol. Geotherm. Res. 369, 155–171. doi:10.1016/j.jvolgeores.2018.11.024
Martin, R., Mather, T., Pyle, D. J. G., and Geophysics, G. E. O. S. Y. S. T. E. M. S. (2006). High‐temperature mixtures of magmatic and atmospheric gases, 7.
Mason, E., Wieser, P. E., Liu, E. J., Edmonds, M., Ilyinskaya, E., Whitty, R. C., et al. (2021). Volatile metal emissions from volcanic degassing and lava–seawater interactions at Kīlauea Volcano, Hawai’i. Commun. Earth Environ. 2, 79–16. doi:10.1038/s43247-021-00145-3
Matsumoto, A., and Hinkley, T. K. (2001). Trace metal suites in Antarctic pre-industrial ice are consistent with emissions from quiescent degassing of volcanoes worldwide. Earth Planet. Sci. Lett. 186, 33–43. doi:10.1016/s0012-821x(01)00228-x
McConnell, J. R., Aristarain, A. J., Banta, J. R., Edwards, P. R., and Simões, J. C. (2007). 20th-Century doubling in dust archived in an Antarctic Peninsula ice core parallels climate change and desertification in South America. Proc. Natl. Acad. Sci. U. S. A. 104, 5743–5748. doi:10.1073/pnas.0607657104
McConnell, J. R., Burke, A., Dunbar, N. W., Köhler, P., Thomas, J. L., Arienzo, M. M., et al. (2017). Synchronous volcanic eruptions and abrupt climate change∼ 17.7 ka plausibly linked by stratospheric ozone depletion. Proc. Natl. Acad. Sci. U. S. A. 114, 10035–10040. doi:10.1073/pnas.1705595114
McConnell, J. R., Chellman, N. J., Wilson, A. I., Stohl, A., Arienzo, M. M., Eckhardt, S., et al. (2019). Pervasive Arctic lead pollution suggests substantial growth in medieval silver production modulated by plague, climate, and conflict. Proc. Natl. Acad. Sci. U. S. A. 116, 14910–14915. doi:10.1073/pnas.1904515116
McConnell, J. R., and Edwards, R. (2008). Coal burning leaves toxic heavy metal legacy in the Arctic. Proc. Natl. Acad. Sci. U. S. A. 105, 12140–12144. doi:10.1073/pnas.0803564105
McConnell, J. R., Maselli, O. J., Sigl, M., Vallelonga, P., Neumann, T., Anschütz, H., et al. (2014). Antarctic-wide array of high-resolution ice core records reveals pervasive lead pollution began in 1889 and persists today. Sci. Rep. 4 (1), 1–5. doi:10.1038/srep05848
Moune, S., Gauthier, P.-J., and Delmelle, P. (2010). Trace elements in the particulate phase of the plume of Masaya Volcano, Nicaragua. J. Volcanol. Geotherm. Res. 193, 232–244. doi:10.1016/j.jvolgeores.2010.04.004
Moune, S., Gauthier, P.-J., Gislason, S. R., and Sigmarsson, O. (2006). Trace element degassing and enrichment in the eruptive plume of the 2000 eruption of Hekla volcano, Iceland. Geochimica Cosmochimica Acta 70, 461–479. doi:10.1016/j.gca.2005.09.011
Nriagu, J. O. (1989). A global assessment of natural sources of atmospheric trace metals. Nature 338, 47–49. doi:10.1038/338047a0
Oppenheimer, C., Moretti, R., Kyle, P. R., Eschenbacher, A., Lowenstern, J. B., Hervig, R. L., et al. (2011). Mantle to surface degassing of alkalic magmas at Erebus volcano, Antarctica. Earth Planet. Sci. Lett. 306, 261–271. doi:10.1016/j.epsl.2011.04.005
Osterberg, E. C., Mayewski, P. A., Fisher, D. A., Kreutz, K. J., Maasch, K. A., Sneed, S. B., et al. (2014). Mount Logan ice core record of tropical and solar influences on aleutian low variability: 500–1998 ad. J. Geophys. Res. Atmos. 119 (19), 11–189. doi:10.1002/2014jd021847
Palais, J. M., Kyle, P. R., Mcintosh, W. C., Seward, D. J. J. O. V., and Research, G. (1988). Magmatic and phreatomagmatic volcanic activity at Mt. Takahe, West Antarctica, based on tephra layers in the Byrd ice core and field observations at Mt. Takahe. J. Volcanol. Geotherm. Res. 35, 295–317. doi:10.1016/0377-0273(88)90025-x
Pennisi, M., le Cloarec, M., Lambert, G., and le Roulley, J. (1988). Fractionation of metals in volcanic emissions. Earth Planet. Sci. Lett. 88, 284–288. doi:10.1016/0012-821x(88)90085-4
Pinto, J. P., Turco, R. P., and Toon, O. B. J. J. O. G. R. A. (1989). Self‐limiting physical and chemical effects in volcanic eruption clouds, 94, 11165–11174.
Rakociński, M., Pisarzowska, A., Corradini, C., Narkiewicz, K., Dubicka, Z., and Abdiyev, N. (2021). Mercury spikes as evidence of extended arc-volcanism around the Devonian–Carboniferous boundary in the South Tian Shan (southern Uzbekistan). Sci. Rep. 11, 5708–5715. doi:10.1038/s41598-021-85043-6
Rhodes, R. H., Baker, J. A., Millet, M.-A., and Bertler, N. A. (2011). Experimental investigation of the effects of mineral dust on the reproducibility and accuracy of ice core trace element analyses. Chem. Geol. 286, 207–221. doi:10.1016/j.chemgeo.2011.05.006
Robock, A. (2000). Volcanic eruptions and climate. Rev. Geophys. 38, 191–219. doi:10.1029/1998rg000054
Rowell, C., Jellinek, M., Hajimirza, S., and Aubry, T. J. (2021). External surface water influence on explosive eruption dynamics, with implications for stratospheric sulfur delivery and volcano-climate feedback. Surface 67, 753.
Rubin, K. (1997). Degassing of metals and metalloids from erupting seamount and mid-ocean ridge volcanoes: Observations and predictions. Geochimica Cosmochimica Acta 61, 3525–3542. doi:10.1016/s0016-7037(97)00179-8
Schuster, P. F., Krabbenhoft, D. P., Naftz, D. L., Cecil, L. D., Olson, M. L., Dewild, J. F., et al. (2002). Atmospheric mercury deposition during the last 270 years: A glacial ice core record of natural and anthropogenic sources. Environ. Sci. Technol. 36, 2303–2310. doi:10.1021/es0157503
Sigl, M., McConnell, J. R., Layman, L., Maselli, O., Mcgwire, K., Pasteris, D., et al. (2013). A new bipolar ice core record of volcanism from WAIS Divide and NEEM and implications for climate forcing of the last 2000 years. J. Geophys. Res. Atmos. 118, 1151–1169. doi:10.1029/2012jd018603
Symonds, R. B., Rose, W. I., Bluth, G. J., and Gerlach, T. M. (1994). Volcanic-gas studies; methods, results, and applications. Rev. Mineralogy Geochem. 30, 1–66.
Textor, C., Graf, H. F., Herzog, M., and Oberhuber, J. J. J. O. G. R. A. (2003). Injection of gases into the stratosphere by explosive volcanic eruptions, 108.
Vallelonga, P., Candelone, J.-P., van De Velde, K., Curran, M., Morgan, V., and Rosman, K. (2003). Lead, Ba and Bi in antarctic law Dome ice corresponding to the 1815 AD Tambora eruption: An assessment of emission sources using Pb isotopes. Earth Planet. Sci. Lett. 211, 329–341. doi:10.1016/s0012-821x(03)00208-5
Wardell, L., Kyle, P., Counce, D. J. J. O. V., and Research, G. (2008). Volcanic emissions of metals and halogens from White Island (New Zealand) and Erebus volcano (Antarctica) determined with chemical traps. J. Volcanol. Geotherm. Res. 177, 734–742. doi:10.1016/j.jvolgeores.2007.07.007
Weiss, H. V., Herron, M. M., and Langway, C. C. J. N. (1978). Natural enrichment of elements in snow. Nature 274, 352–353. doi:10.1038/274352a0
Werner, C., Goff, F., and Janik, C. (1997). Geochemistry of summit fumarole vapors and flanking thermal/mineral waters at Popocatépetl volcano, Mexico. Los Alamos, NM (United States): Los Alamos National Lab.
Xu, J., Kaspari, S., Hou, S., Kang, S., Qin, D., Ren, J., et al. (2009). Records of volcanic events since AD 1800 in the East Rongbuk ice core from Mt. Qomolangma. Sci. Bull. (Beijing). 54, 1411–1416. doi:10.1007/s11434-009-0020-y
Zelenski, M. E., Fischer, T. P., De Moor, J. M., Marty, B., Zimmermann, L., Ayalew, D., et al. (2013). Trace elements in the gas emissions from the Erta Ale volcano, Afar, Ethiopia. Chem. Geol. 357, 95–116. doi:10.1016/j.chemgeo.2013.08.022
Zelenski, M., Simakin, A., Taran, Y., Kamenetsky, V., and Malik, N. J. G. E. C. A. (2021). Partitioning of elements between high-temperature, low-density aqueous fluid and silicate melt as derived from volcanic gas geochemistry. Geochim. Cosmochim. Acta 295, 112–134. doi:10.1016/j.gca.2020.12.011
Keywords: degassing, volatile metals, ice core, stratosphere, volcanic
Citation: Mason E, Edmonds M and McConnell JR (2022) Volatile trace metals deposited in ice as soluble volcanic aerosols during the 17.7.ka eruptions of Mt Takahe, West Antarctic Rift. Front. Earth Sci. 10:1002366. doi: 10.3389/feart.2022.1002366
Received: 25 July 2022; Accepted: 01 November 2022;
Published: 16 November 2022.
Edited by:
Juliana Troch, Smithsonian National Museum of Natural History (SI), United StatesReviewed by:
Jacob B. Lowenstern, United States Geological Survey (USGS), United StatesSeverine Moune, UMR7154 Institut de Physique du Globe de Paris (IPGP), France
Copyright © 2022 Mason, Edmonds and McConnell. This is an open-access article distributed under the terms of the Creative Commons Attribution License (CC BY). The use, distribution or reproduction in other forums is permitted, provided the original author(s) and the copyright owner(s) are credited and that the original publication in this journal is cited, in accordance with accepted academic practice. No use, distribution or reproduction is permitted which does not comply with these terms.
*Correspondence: Marie Edmonds, marie.edmonds@esc.cam.ac.uk