- 1DeWitt Daughtry Family Department of Surgery, University of Miami Miller School of Medicine, Miami, FL, United States
- 2Vascular Biology Institute, University of Miami Miller School of Medicine, Miami, FL, United States
- 3Department of Biochemistry & Molecular Biology, University of Miami Miller School of Medicine, Miami, FL, United States
Cardiovascular diseases (CVD) continue to be the leading cause of morbidity and mortality globally and claim the lives of over 17 million people annually. Current management of CVD includes risk factor modification and preventative strategies including dietary and lifestyle changes, smoking cessation, medical management of hypertension and cholesterol lipid levels, and even surgical revascularization procedures if needed. Although these strategies have shown therapeutic efficacy in reducing major adverse cardiovascular events such as heart attack, stroke, symptoms of chronic limb-threatening ischemia (CLTI), and major limb amputation significant compliance by patients and caregivers is required and off-target effects from systemic medications can still result in organ dysfunction. Stem cell therapy holds major potential for CVD applications but is limited by the low quantities of cells that are able to traffic to and engraft at diseased tissue sites. New preclinical investigations have been undertaken to modify mesenchymal stem cells (MSCs) to achieve targeted cell delivery after systemic administration. Although previous reviews have focused broadly on the modification of MSCs for numerous local or intracoronary administration strategies, here we review recent preclinical advances related to overcoming challenges imposed by the high velocity and dynamic flow of the circulatory system to specifically deliver MSCs to ischemic cardiac and peripheral tissue sites. Many of these technologies can also be applied for the targeted delivery of other types of therapeutic cells for treating various diseases.
1. Introduction
The efficacy of cell-based therapeutics, such as those utilizing mesenchymal stem/stromal cells (MSCs), is contingent upon effective cellular engraftment and homing to disease sites in order to re-establish homeostasis and enact their therapeutic benefits. Local injection to the site of disease or intravascular delivery are the most commonly utilized methods of administration of stem cells—including intraarterial injection that quickly delivers therapeutic cells to the tissue/organs fed by a given artery, and intravenous routes, by which therapeutic cells are infused into the bloodstream (systemic delivery) (1, 2). Unfortunately, these delivery routes are associated with substantial limitations. Direct injection is a popular method of administration particularly in sites with poor systemic perfusion; however, cell-based therapy is significantly limited by cell viability and retention even when locally dispensed to sites of disease (1, 3). Moreover, cells delivered to local disease sites may not function appropriately given environmental factors at the tissue interface including poor blood flow, hyperglycemia, hypoxia, and wide-spread local inflammation associated with disease (4–7). Limited space and even physical pressure at sites of inoculation may further diminish cellular characteristics or hamper cell viability (5, 8, 9). However, many disease sites are not amenable to access by local inoculation given their intra-cavitary anatomic location (cardiothoracic organs, brain, aorta, etc.), which would necessitate highly invasive injection procedures. Systemic administration via intravenous routes can partially overcome this limitation in delivery to locations that are traditionally hard to access. Unfortunately, this can lead to indiscriminate trafficking of cells and often results in a low number of cells delivered to anatomic locations afflicted by disease (1, 10). As a result, there is a critical, unmet need for novel methods to augment delivery of a sufficient quantity of therapeutic cells to diseased tissue sites.
A novel area of investigation within the field of cell-based therapeutics is the advent of targeted cell delivery to limit the downsides of systemic cell administration as well as enhance cell therapeutic efficacy. The purpose of this review is to summarize the most recent preclinical advances in the arena of targeted cell delivery for applications in cardiovascular and peripheral vascular disease applications achieved through physical, chemical, and genetic modifications to enhance the delivery of MSCs (Figure 1).
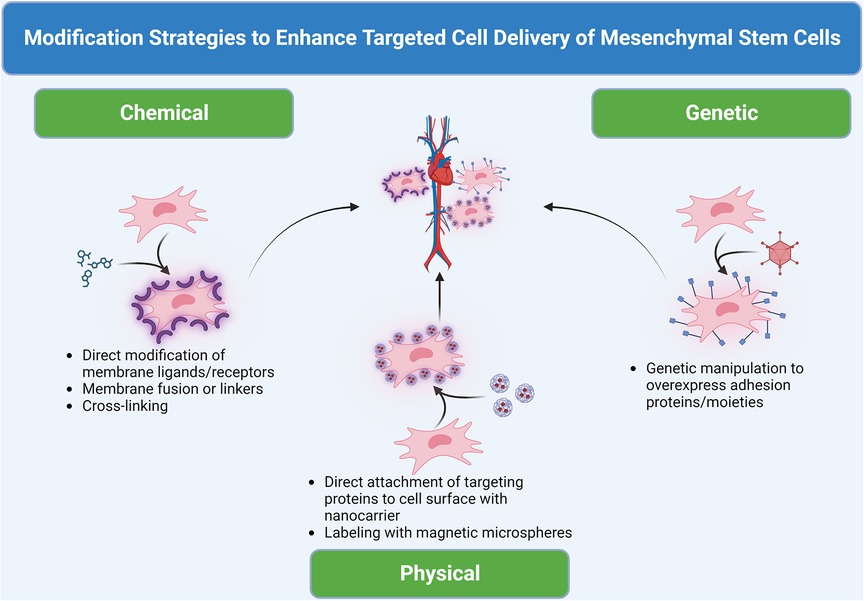
Figure 1. Domains of modification of therapeutic MSCs for targeted cell delivery to cardiovascular sites. Three methods, including chemical, physical and biological, can be used to modify the cell surface or label cells to create or install “GPS”/“sensor” and guide cell homing.
2. Basis for modifying MSCs for targeted cell delivery in cardiovascular applications
At the circulatory system interface, endothelial cells form a selectively permeable luminal barrier between blood and tissues under homeostatic conditions. As a result, unstimulated/unactivated healthy endothelium in normal tissue forms a smooth lining that contributes to the laminar flow of the circulatory system and facilitate the transport of cells and nutrients to peripheral sites. After physiologic insult from tissue injury, inflammation, and/or malignancy, numerous chemokines and cytokines such as stromal cell-derived factor 1 alpha (SDF-1α) and transforming growth factor beta (TGF-β) activate neighboring endothelial cells (11, 12). Resultant stimulation by a cytokine milieu specific to each pathologic state can cause a variety of cell adhesion molecules (CAMs) such as integrins and selectins to be highly expressed within the luminal endothelium in the diseased tissue; thereby, making the endothelial lining “sticky” and augmenting its ability to tether circulating cells, such as endothelial progenitor cells (EPCs), MSCs, and leukocytes, responding to tissue repair and inflammatory signals (12). As a result, methods to exploit cognate protein-receptor binding interactions between circulating MSCs and activated endothelial cells at sites of ischemic vasculature after myocardial infarction (MI) or peripheral ischemic insults can dramatically augment the ability of MSCs to traffic to diseased tissue sites.
Ultimately, the cytokine profile and subsequent endothelial cell surface molecules induced are unique to the underlying inflammatory, ischemic, or oncologic pathophysiology (Figure 2). To better characterize highly upregulated target CAMs on endothelial sites in diseased tissues, the utilization of newly available RNA sequencing, protein array technology, and single-cell multiomics has widely propagated (13–15). For example, Fournier et al. (16) utilized bulk RNA sequencing of meningeal endothelial cells to identify upregulation of coxsackie- and adenovirus receptor-like membrane protein (CLMP) as a trafficking molecule upregulated by inflammation in neurologic conditions such as multiple sclerosis. They further demonstrated that overexpressed CLMP is utilized by immune cells to traffic across endothelial cells into the central nervous system to modulate neuroinflammation, and this CLMP overexpression could represent a new therapeutic target for modified cell therapies to exploit and target the central nervous system (16). The continued applications of similar profiling methods will help to uncover unique adhesion molecules expressed on endothelial cells at specific disease sites in order to target their cognate ligands as molecular mechanisms and augment the targeted cell delivery of stem cells for cardiovascular applications in future work.
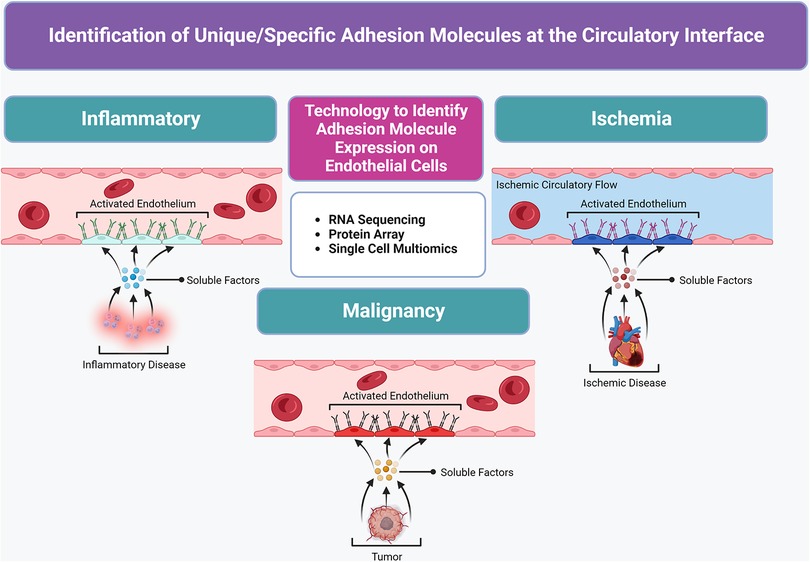
Figure 2. Molecular profiling advancements used to identify unique/special target(s) at the circulatory system interface in diseased tissues to create a “roadmap” for the targeted delivery of therapeutic MSCs. Unique cytokine/chemokine milieu in various diseased tissue states stimulate local vasculature to express a panel of elevated or specific adhesion molecules in activated endothelium.
3. Physical methods of guiding cell engraftment
With the advent of novel molecular and synthetic platforms, new avenues of manipulating the cell surface to change migratory patterns of cells in circulation have become available to researchers. Such methods offer the ability to physically alter or decorate the cell surface with specific proteins without aberrant changes to intrinsic MSC properties that can occur through genetic manipulation or downstream cell signaling (7). Nanocarriers utilizing adhesion moieties or cell membrane components have emerged as a versatile, safe method to achieve the delivery of drug and cell therapeutic payloads to specific tissue sites expressing the cognate receptor for proteins on a modified nanocarrier (5, 17–19). For instance, E-selectin, an inducible CAM with pro-angiogenic signaling effects, along with its ligands, including CD44, CD62 and E-selectin ligand (ESL), have been shown to be upregulated on vasculature in ischemic tissue (20, 21). Liu et al. (5) demonstrated that systemic administration of pre-modified MSCs on which cell surfaces were coated with E-selectin-conjugated dendrimer nanocarriers directed MSC homing to skin wound sites or injured cornea mediated by enhanced interaction of E-selectin/ligands. This resulted in increased tissue repair in an ischemic diabetic wound healing model. This physical cell modification led to efficient trafficking of MSCs to wound areas without indiscriminate distribution to other organs even when administered systemically (5).
Through an extension of the beneficial angiogenic effects of the numerous cell signaling and paracrine factors secreted by MSCs, some groups have created nanocells by combining nanocarrier/membrane derivatives with the MSC secretome. By exploiting the overexpression of prostaglandin E2 (PGE2) receptors in ischemic myocardium, Su et al. (22) designed a platelet-inspired nanocell (PINC) containing PGE2-platelet membranes and cellular signaling factors harvested from the cardiac stem cell (CSC) secretome. Intravenous administration of PGE2-PINCs achieved targeted delivery of the CSC-derived therapeutic payload to the injured myocardium in a murine model of myocardial ischemia and reperfusion injury. This therapy significantly mitigated ischemia and reperfusion injury in myocardium as evidenced by preserved cardiac ejection fraction (EF), increased cycling of cardiomyocytes, activation of endogenous CSCs, and enhanced angiogenesis in cardiac tissue in vivo compared to control PINC treatment lacking PGE2-platelet membranes (22). This work demonstrates a new method to direct the MSC therapeutic payload to target sites.
Some have even investigated the application of physical devices to guide stem cell transplantation in difficult locations. For instance, the retention of stem cells to the heart is often impeded due to venous washout resulting from forceful cardiac contraction even when delivered via direct intracoronary injection (23). Hydrogel and microgel formulations as well as direct cell coating are applications that have been extensively investigated in murine and porcine models of myocardial ischemia to overcome challenges in cell retention. Utilizing an alginate scaffold to aid the local implantation of MSCs in a porcine model of MI, Panda et al. (24) demonstrated substantially preserved cell retentiona as well as improved cardiac impulse condution. Others such as Peng et al. (25) have utilized a gelatin methacrylate cell-coating system on murine-derived MSCs locally inoculated to the heart after induction of MI. Cell coating with gelatin methacrylate offered a less bulky matrix compared to conventional hydrogels for MSCs to more directly interact with diseased tissue sites, which resulted in preserved cardiac function, reduced scar size, and augmented angiogenesis compared to control treatments (25). While biomaterials such as MSC-loaded scaffolds and devices hold promise, they still incur postoperative complications associated with implantation of medical devices such as fibrosis and adhesions that can complicate procedures later in life (23). To overcome these challenges, Cheng et al. (26) investigated the use of CSCs labeled with iron microspheres. After ligation of the left anterior descending artery to simulate myocardial ischemia in rats, administration of iron labeled-CSCs via left ventricular injection occurred and was followed by the placement of an external magnet outside the heart for 10 min. Overall, rats exposed to the external magnet demonstrated substantially augmented rates of stem cell engraftment in ischemic heart tissue and significantly reduced migration of therapeutic cells to the lungs. These effects persisted up to 3 weeks after injection and resulted in enhanced therapeutic benefits including higher ventricular EF and attenuated left ventricular remodeling compared to rats not exposed to the external magnet device during stem cell administration (26). Similarly, Shen et al. (27) utilized rat MSCs labeled with superparamagnetic oxide nanoparticles (SPIOs) administered into the left ventricle of recipient rats after induction of myocardial ischemia and placed magnets of varying intensity at the cardiac site. They importantly found that cellular engraftment was significantly correlated with higher magnetic intensity; however, the highest magnetic intensity resulted in microembolization and undermined the clinical benefits of cell therapy. Ultimately, these works serve as examples of physical devices to potentiate cell delivery and retention at target disease tissues without the risks associated with the implantation of long term hardware. While physical alterations such as these hold significant potential to improve targeted cell delivery for cardiovascular applications, future studies will need to consider and rigorously test the safety and toxicity associated with nanocarriers and other synthetic platforms (5, 17). Furthermore, physical devices to guide stem cell engraftment are a unique way to enhance cell transplantation but will need to optimize the least invasive methods possible of device utilization as well as consider their cost and scalability for clinical applications (26, 28).
4. Genetic manipulations to direct cell migration
While physical methods of manipulating the cell surface of MSCs can augment their migratory ability, newer methods of genetic manipulation have emerged as a promising method to overexpress cell-cell binding proteins for delivery and further potentiate the innate properties and therapeutic effects of MSCs (29, 30). For example, colony-stimulating factor 2 (CSF2) is a signaling chemokine that along with its cognate receptor pair CSF2RB modulates leukocyte production and proliferation as well as MSC recruitment and post-myocardial infarction (MI) cardiac remodeling (31). However, expression of CSF2RB is very low in unmodified MSCs (32, 33). To overcome this, Qi et al. (32) utilized an adenovirus vector to induce overexpression of CSF2RB in adipose-derived MSCs (AD-MSCs) prior to intravenous delivery in murine subjects after myocardial ischemia and reperfusion injury. Compared to unmodified AD-MSCs, CSF2RB-modified AD-MSCs became imbued with a cardioprotective phenotype that resulted in markedly preserved ventricular EF and cardiac contractility in injured mice. Moreover, genetic modification with CSF2RB augmented AD-MSCs’ proangiogenic properties and inhibited cell apoptosis in addition to increasing their migratory capacity through increased phosphorylation of STAT5 within the canonical JAK-STAT5 signaling pathway. Modification of MSCs in this way to improve their migratory capacity after intravenous administration can also allow for repeated rounds of noninvasive treatment with cell delivery, thereby overcoming traditional drawbacks associated with invasive intramyocardial injections (34, 35). In a similar fashion, Yan et al. (36) implemented an adenoviral vector to induce overexpression of N-cadherin, a transmembrane cell-cell adhesion protein, in murine derived AD-MSCs. Cells harboring abundant cell surface N-cadherin levels demonstrated improved adhesion on to cardiomyocytes and paracrine effects on angiogenesis mediated by subsequent upregulation of matrix metalloproteinases (MMPs)-10 and -13 as well as hepatocyte growth factor (HGF). Furthermore, this cell therapy resulted in enhanced engraftment at ischemic myocardium and attenuated fibrosis after cardiac ischemia in vivo.
Parallel applications of genetic programming of MSCs have also been undertaken for peripheral vascular diseases such as chronic limb-threatening ischemia (CLTI) in recent years. Hematopoietic stem cells have previously been shown to express a CD44 glycoform known as hematopoietic cell E-/l-selectin ligand (HCELL), which functions as a highly potent ESL to improve their recruitment within bone marrow compartments (19). Modification of cell surface CD44 on stem cells into HCELL has been previously shown to enhance the tropism of these cells to bone, which can also provide a “roadmap” or “GPS” for targeted cell delivery to areas with heightened ESL expression such as in ischemic vasculature (18, 37, 38). To exploit this relationship with E-selectin, a CAM capable of inducing postnatal neovascularization, in ischemic tissue, Quiroz et al. (30) demonstrated a novel application of murine bone marrow-derived MSCs (BM-MSCs) for CLTI after inducing the overexpression of cell surface membrane-bound E-selectin via an adenoviral vector. By simulating limb ischemia via surgical ligation of the femoral artery, mice receiving local therapy with E-selectin-modified BM-MSCs demonstrated rescued revascularization, muscle fiber integrity, and ambulatory capacity on treadmill testing compared to control BM-MSC treatment (30). Interestingly, genetic modification of BM-MSCs with E-selectin also enhanced the angiogenic profile of these cells in vitro through the upregulation of nine proangiogenic genes such as Cxcl2, which among many functions also contributes to cell engraftment (30). Taken together, these studies show hope for augmenting not only cellular delivery and engraftment but also the cell potency of MSCs for future cardiovascular and peripheral disease applications. As researchers continue to explore such options for gene editing, they should consider the associated risks of these approaches such as aberrant insertional mutagenesis and chromosomal instability (39). Although viral vectors have been used in previous clinical applications, adverse immune reactions can occur with their use and impede the stability of gene expression (40, 41).
5. Chemical methods to potential cell delivery
Direct chemical modification of the cell surface can also yield improvements in cell trafficking. Although AD-MSCs offer advantages as a readily accessible tissue source of MSCs with potent paracrine and tissue reparative functions, their homing potential for cardiovascular purposes is significantly limited in unmodified cells (42–44). To overcome this challenge and allow targeting to denuded endothelial sites after vascular injury, Yan et al. (45) altered AD-MSCs utilizing a polyethylene glycol-conjugated derivative linker to facilitate the binding of P-selectin binding peptide (PBP) to the cell surface. After both wire and balloon injury to femoral artery sites in rats to simulate injury from percutaneous interventions observed in human patients, PBP-modified AD-MSCs targeted in significantly greater quantities to vascular injury sites after intraarterial injection and shielded injury sites from pathologic platelet and leukocyte adhesion. In turn, this substantially augmented vascular repair, limited pathologic neointimal hyperplasia, and augmented endothelial cell proliferation compared to unmodified AD-MSCs (45).
Chemical modification has similarly been applied to fuse components of other cell types with natural vascular homing abilities to MSCs. For instance, Tang et al. (46) harvested nanovesicles from platelets and fused them to the cell membrane of CSCs using polyethylene glycol. By exploiting the natural ability of platelet membranes in localizing to ischemic vascular sites and denuded endothelium, platelet-nanovesicle-fused CSCs augmented migration and retention to ischemic myocardium after intravenous delivery in a porcine model of cardiac ischemia and reperfusion (46). Moreover, this modified cell therapy yielded myocyte proliferation and angiogenesis in cardiac sites to a degree far greater than administering unmodified CSCs. As newer pharmacologic and chemical synthetic mechanisms continue to be developed, investigators will have a greater armamentarium of methods to modify MSCs for cardiovascular applications than ever before. However, limitations associated with chemical priming and modification strategies should be taken into account. For example, chemical agents can produce off-target, unintended effects on MSCs and result in transient expression of the molecular target (47). Additionally, scalability and manufacturing of sufficient quantities of MSCs using costly chemical strategies can pose a significant challenge in future clinical applications (48).
6. Host factors affecting modification strategies
While physical, chemical, and genetic modification of MSCs hold significant promise to augment their targeted delivery to disease tissues, the host milieu to which cells are delivered plays an equally important role in determining their therapeutic efficacy. Immunocombatibility between donor and recipients can reduce effectiveness of MSCs. MSCs typically exist as immune privileged cells due to their low expression of HLA-I and MHC-I (4). However, all cell modification strategies and culture methods can cause increased expression of immunogenic molecules. Furthermore, the inflammatory environment in recipient tissue such as interferon gamma can yield heightened MHC-II expression in donor MSCs, which can result in rejection and thereby necessitate repeated injections to achieve clinical benefits (49). The chemokine expression profile in recipient tissue can also be significantly heterogeneous and may not be compatible with unmodified MSCs. For example, areas of myocardial infarction may typically have high levels of expression of chemokines such as CXCL1, CXCL2, and CCL7; however, the presence of their corresponding receptors such as CCR1 and CXCR2 on native MSCs is low and can impede their delivery to such sites (50). As a result, careful molecular profiling of host disease tissue sites is needed prior to selecting targets to modify in donor MSCs to ensure maximal migratory and therapeutic potential is preserved.
The effect of the disease process within recipient tissue is also a major host factor limiting the efficacy of MSC therapy. For CVD such as myocardial infarction and PVD, significant ischemia and hypoxia in tissue results in a limited nutrient supply and hostile tissue interface for delivered MSCs to survive and proliferate in even after they have been modified. Moreover, patients with these diseases often have major comorbidities such as diabetes mellitus, smoking, and renal disease which can exacerbate ischemia and reduce the functionality of MSCs such as from hyperglycemia and circulation of uncleared uremic breakdown products (10). The utilization of biologic scaffolds and hydrogels can help provide a more hospitable cellular environment to areas of local delivery to improve the survival and retention of cell therapy. However, these methods to implant such scaffolds can require invasive surgical or percutaneous procedures to access cardiac and other internal organ sites thereby increasing the risk of infection and anesthestic complications in a patient population already predisposed to higher rates of complications (10).
7. Conclusions and future perspectives
CVD continue to be the leading cause of morbidity and mortality globally and claim the lives of over 17 million people annually (51–53). Current management of CVD includes risk factor modification and preventative strategies such as dietary and lifestyle changes, smoking cessation, medical management of hypertension and cholesterol lipid levels, and surgical revascularization interventions if needed (17, 54, 55). Although these strategies have shown therapeutic efficacy in reducing major cardiovascular events such as heart attack, stroke, acute limb ischemia, sequelae of CLTI, and major limb amputations, significant compliance by patients and caregivers is required and off-target effects from systemic medications can still result in organ dysfunction (17, 54). Given the rising incidence of CVD globally, newer strategies to reduce inflammation and stenosis associated with atherosclerosis progression beyond those imparted by conventional medical and surgical options are needed (52, 53).
MSC-based therapeutics hold substantial potential for tissue and vascular regenerative applications for cardiac and peripheral ischemic disease applications given their natural capacity for immunomodulation, self-renewal, and differentiation. However, delivery of sufficient quantities of cells to target disease sites without invasive intracoronary or direct injection methods remains a substantial challenge (10, 28). Methods to augment targeted cell delivery after systemic injection without indiscriminate trafficking to cell-sink organs such as the lungs and liver are critically needed for patients with these diseases. Recent preclinical advances utilizing physical methods to install adhesion moieties with nanocarriers or device-assisted induction of cell trafficking are avenues with emerging potential. Genetic methods have been widely applied to modify MSCs for other disease applications and are showing promise to enhance MSC phenotype and migratory capacity for cardiovascular applications. Several recent studies have demonstrated chemical modification strategies to influence the trafficking of MSCs to ischemic disease sites.
As investigators begin to translate these and similar preclinical advances into the clinical arena, several important considerations should be taken into account. Modification of MSCs by any strategy should include careful cell phenotypic characterization studies to ensure that innate stem cell properties including multilineage differentiation, self-renewal, and immunodulatory functions are preserved (56, 57). Nonbiologic nanocarrier platforms as well as genetic manipulation strategies utilizing viral vectors should be thoroughly interrogated with regards to their toxicity and safety after systemic administration of cell therapeutics (41, 58–60). Moreover, the cost and scalability utilizing any of these MSC modification domains should be considered prior to initiating preclinical and clinical investigations (48, 61).
Although this review focused primarily on the benefits of targeted MSC therapies, the technologies implemented to modify cells for homing to disease sites are extremely versatile and can be applied to the delivery of pharmacologic agents or additional cell types such as mononuclear cells and others (17, 62). Some investigators have modified mononuclear cells with adenoviral constructs to overexpress proangiogenic factors such as vascular endothelial growth factor (VEGF) and fibroblast growth factor (FGF), which results in their ability to induce endothelial cell proliferation and may represent future treatments for ischemic diseases (63). Moreover, future work may uncover that combinations of these aforementioned physical, genetic, and chemical alteration methods could produce the optimal cell phenotype to achieve localized cell homing to diseased tissue sites. Despite the challenges and limitations that will need to be overcome in translating these scientific advances into actionable clinical therapeutics, targeted cell delivery of MSCs holds tremendous potential and hope for patients with a wide variety of ischemic cardiovascular diseases that fail or are not candidates for other medical and surgical treatment options.
Author contributions
Study Conception and Design: CH, FV, YO, ZJ-L, and OV. Acquisition of Data: CH and ZJ-L. Analysis and Interpretation of Data: CH, FV, YO, ZJ-L, and OV. Drafting of Manuscript: CH. Critical Revision of Manuscript: CH, FV, YO, ZJ-L, and OV. All authors contributed to the article and approved the submitted version.
Funding
This article is supported by grants from the National Institutes of Health R01 HL149452 and R61/R33 HL156152 Catalyze.
Acknowledgments
All figures were created with BioRender.com.
Conflict of interest
ZJ-L and OV declared the following potential conflicts of interest with respect to the research, authorship, and/or publication of this article: the E-selectin gene modification technologies described in referenced articles by ZJ-L and OV were developed in our research laboratory and patented/Licensed by the University of Miami. This technology is currently under pre-clinical development by Ambulero Inc., a new start-up company out of the University of Miami that focuses on developing new vascular treatments for ischemic conditions. Co-authors, ZJ-L and OV, serve as consultants and scientific and medical advisory officers in Ambulero Inc. OV and ZJ-L are co-inventors, co-principal investigators funded by the NIH and are minority share holders in Ambulero Inc.
The remaining authors declare that the research was conducted in the absence of any commercial or financial relationships that could be construed as a potential conflict of interest.
Publisher's note
All claims expressed in this article are solely those of the authors and do not necessarily represent those of their affiliated organizations, or those of the publisher, the editors and the reviewers. Any product that may be evaluated in this article, or claim that may be made by its manufacturer, is not guaranteed or endorsed by the publisher.
References
1. Kean TJ, Lin P, Caplan AI, Dennis JE. MSCs: delivery routes and engraftment, cell-targeting strategies, and immune modulation. Stem Cells Int. (2013) 2013:732742. doi: 10.1155/2013/732742
2. Klepanec A, Mistrik M, Altaner C, Valachovicova M, Olejarova I, Slysko R, et al. No difference in intra-arterial and intramuscular delivery of autologous bone marrow cells in patients with advanced critical limb ischemia. Cell Transplant. (2012) 21(9):1909–18. doi: 10.3727/096368912X636948
3. Luo G, Cheng W, He W, Wang X, Tan J, Fitzgerald M, et al. Promotion of cutaneous wound healing by local application of mesenchymal stem cells derived from human umbilical cord blood. Wound Repair Regen. (2010) 18(5):506–13. doi: 10.1111/j.1524-475X.2010.00616.x
4. Zhou T, Yuan Z, Weng J, Pei D, Du X, He C, et al. Challenges and advances in clinical applications of mesenchymal stromal cells. J Hematol Oncol. (2021) 14(1):24. doi: 10.1186/s13045-021-01037-x
5. Liu Z-J, Daftarian P, Kovalski L, Wang B, Tian R, Castilla DM, et al. Directing and potentiating stem cell-mediated angiogenesis and tissue repair by cell surface E-selectin coating. PLoS One. (2016) 11(4):e0154053. doi: 10.1371/journal.pone.0154053
6. Capilla-González V, López-Beas J, Escacena N, Aguilera Y, de la Cuesta A, Ruiz-Salmerón R, et al. PDGF Restores the defective phenotype of adipose-derived mesenchymal stromal cells from diabetic patients. Mol Ther. (2018) 26(11):2696–709. doi: 10.1016/j.ymthe.2018.08.011
7. Huerta CT, Ortiz YY, Liu ZJ, Velazquez OC. Methods and limitations of augmenting mesenchymal stem cells for therapeutic applications. Adv Wound Care (New Rochelle). (2023) 12(8):467–81. doi: 10.1089/wound.2022.0107
8. Baraniak PR, Cooke MT, Saeed R, Kinney MA, Fridley KM, McDevitt TC. Stiffening of human mesenchymal stem cell spheroid microenvironments induced by incorporation of gelatin microparticles. J Mech Behav Biomed Mater. (2012) 11:63–71. doi: 10.1016/j.jmbbm.2012.02.018
9. Qadura M, Terenzi DC, Verma S, Al-Omran M, Hess DA. Concise review: cell therapy for critical limb ischemia: an integrated review of preclinical and clinical studies. Stem Cells. (2018) 36(2):161–71. doi: 10.1002/stem.2751
10. Huerta CT, Voza FA, Ortiz YY, Liu ZJ, Velazquez OC. Mesenchymal stem cell-based therapy for non-healing wounds due to chronic limb-threatening ischemia: a review of preclinical and clinical studies. Front Cardiovasc Med. (2023) 10:1113982. doi: 10.3389/fcvm.2023.1113982
11. Pober JS. Endothelial activation: intracellular signaling pathways. Arthritis Res. (2002) 4(Suppl 3):S109–16. doi: 10.1186/ar576
12. Vestweber D. Adhesion and signaling molecules controlling the transmigration of leukocytes through endothelium. Immunol Rev. (2007) 218:178–96. doi: 10.1111/j.1600-065X.2007.00533.x
13. Mesri M, Birse C, Heidbrink J, McKinnon K, Brand E, Bermingham CL, et al. Identification and characterization of angiogenesis targets through proteomic profiling of endothelial cells in human cancer tissues. PLoS One. (2013) 8(11):e78885. doi: 10.1371/journal.pone.0078885
14. Appleby SL, Cockshell MP, Pippal JB, Thompson EJ, Barrett JM, Tooley K, et al. Characterization of a distinct population of circulating human non-adherent endothelial forming cells and their recruitment via intercellular adhesion molecule-3. PLoS One. (2012) 7(11):e46996. doi: 10.1371/journal.pone.0046996
15. Sievert W, Tapio S, Breuninger S, Gaipl U, Andratschke N, Trott K-R, et al. Adhesion molecule expression and function of primary endothelial cells in benign and malignant tissues correlates with proliferation. PLoS One. (2014) 9(3):e91808. doi: 10.1371/journal.pone.0091808
16. Fournier AP, Zandee S, Charabati M, Peelen E, Tastet O, Alvarez JI, et al. CLMP Promotes leukocyte migration across brain barriers in multiple sclerosis. Neurol Neuroimmunol Neuroinflamm. (2022) 9(6):e200022. doi: 10.1212/NXI.0000000000200022
17. Choi KA, Kim JH, Ryu K, Kaushik N. Current nanomedicine for targeted vascular disease treatment: trends and perspectives. Int J Mol Sci. (2022) 23(20):12397. doi: 10.3390/ijms232012397
18. Sackstein R. The bone marrow is akin to skin: HCELL and the biology of hematopoietic stem cell homing. J Investig Dermatol Symp Proc. (2004) 9(3):215–23. doi: 10.1111/j.0022-202X.2004.09301.x
19. Sackstein R. Fulfilling Koch’s postulates in glycoscience: HCELL, GPS and translational glycobiology. Glycobiology. (2016) 26(6):560–70. doi: 10.1093/glycob/cww026
20. Liu Z-J, Tian R, An W, Zhuge Y, Li Y, Shao H. Identification of E-selectin as a novel target for the regulation of post-natal neovascularization: implications for diabetic wound healing. Ann Surg. (2010) 252(4):625–34. doi: 10.1097/SLA.0b013e3181f5a079
21. Liu ZJ, Tian R, Li Y, Zhang L, Shao H, Yang C, et al. SDF-1α-induced dual pairs of E-selectin/ligand mediate endothelial progenitor cell homing to critical ischemia. Sci Rep. (2016) 6:34416. doi: 10.1038/srep34416
22. Su T, Huang K, Ma H, Liang H, Dinh P-U, Chen J, et al. Platelet-inspired nanocells for targeted heart repair after ischemia/reperfusion injury. Adv Funct Mater. (2019) 29(4):1803567. doi: 10.1002/adfm.201803567
23. Li Z, Hu S, Cheng K. Chemical engineering of cell therapy for heart diseases. Acc Chem Res. (2019) 52(6):1687–96. doi: 10.1021/acs.accounts.9b00137
24. Panda NC, Zuckerman ST, Mesubi OO, Rosenbaum DS, Penn MS, Donahue JK, et al. Improved conduction and increased cell retention in healed MI using mesenchymal stem cells suspended in alginate hydrogel. J Interv Card Electrophysiol. (2014) 41(2):117–27. doi: 10.1007/s10840-014-9940-9
25. Peng H, Chelvarajan L, Donahue R, Gottipati A, Cahall CF, Davis KA, et al. Polymer cell surface coating enhances mesenchymal stem cell retention and cardiac protection. ACS Appl Bio Mater. (2021) 4(2):1655–67. doi: 10.1021/acsabm.0c01473
26. Cheng K, Malliaras K, Li T-S, Sun B, Houde C, Galang G, et al. Magnetic enhancement of cell retention, engraftment, and functional benefit after intracoronary delivery of cardiac-derived stem cells in a rat model of ischemia/reperfusion. Cell Transplant. (2012) 21(6):1121–35. doi: 10.3727/096368911X627381
27. Shen Y, Liu X, Huang Z, Pei N, Xu J, Li Z, et al. Comparison of magnetic intensities for mesenchymal stem cell targeting therapy on ischemic myocardial repair: high magnetic intensity improves cell retention but has no additional functional benefit. Cell Transplant. (2015) 24(10):1981–97. doi: 10.3727/096368914X685302
28. Mingliang R, Bo Z, Zhengguo W. Stem cells for cardiac repair: status, mechanisms, and new strategies. Stem Cells Int. (2011) 2011:310928. doi: 10.4061/2011/310928
29. Suresh SC, Selvaraju V, Thirunavukkarasu M, Goldman JW, Husain A, Alexander Palesty J, et al. Thioredoxin-1 (Trx1) engineered mesenchymal stem cell therapy increased pro-angiogenic factors, reduced fibrosis and improved heart function in the infarcted rat myocardium. Int J Cardiol. (2015) 201:517–28. doi: 10.1016/j.ijcard.2015.08.117
30. Quiroz HJ, Valencia SF, Shao H, Li Y, Ortiz YY, Parikh PP, et al. E-selectin-overexpressing mesenchymal stem cell therapy confers improved reperfusion, repair, and regeneration in a murine critical limb ischemia model. Front Cardiovasc Med. (2021) 8:826687. doi: 10.3389/fcvm.2021.826687
31. Anzai A, Choi JL, He S, Fenn AM, Nairz M, Rattik S, et al. The infarcted myocardium solicits GM-CSF for the detrimental oversupply of inflammatory leukocytes. J Exp Med. (2017) 214(11):3293–310. doi: 10.1084/jem.20170689
32. Qi T, Xu X, Guo Y, Xia Y, Peng L, Li C, et al. CSF2RB overexpression promotes the protective effects of mesenchymal stromal cells against ischemic heart injury. Theranostics. (2023) 13(6):1759–73. doi: 10.7150/thno.81336
33. Park SR, Cho A, Kim JW, Lee HY, Hong IS. A novel endogenous damage signal, CSF-2, activates multiple beneficial functions of adipose tissue-derived mesenchymal stem cells. Mol Ther. (2019) 27(6):1087–100. doi: 10.1016/j.ymthe.2019.03.010
34. Huang A, Liu Y, Qi X, Chen S, Huang H, Zhang J, et al. Intravenously transplanted mesenchymal stromal cells: a new endocrine reservoir for cardioprotection. Stem Cell Res Ther. (2022) 13:253. doi: 10.1186/s13287-022-02922-z
35. Nakamura Y, Kita S, Tanaka Y, Fukuda S, Obata Y, Okita T, et al. Adiponectin stimulates exosome release to enhance mesenchymal stem-cell-driven therapy of heart failure in mice. Mol Ther. (2020) 28(10):2203–19. doi: 10.1016/j.ymthe.2020.06.026
36. Yan W, Lin C, Guo Y, Chen Y, Du Y, Lau WB, et al. N-cadherin overexpression mobilizes the protective effects of mesenchymal stromal cells against ischemic heart injury through a β-catenin–dependent manner. Circ Res. (2020) 126(7):857–74. doi: 10.1161/CIRCRESAHA.119.315806
37. Sackstein R, Merzaban JS, Cain DW, Dagia NM, Spencer JA, Lin CP, et al. Ex vivo glycan engineering of CD44 programs human multipotent mesenchymal stromal cell trafficking to bone. Nat Med. (2008) 14(2):181–7. doi: 10.1038/nm1703
38. Huerta CT, Ortiz YY, Li Y, Ribieras AJ, Voza F, Le N, et al. Novel gene-modified mesenchymal stem cell therapy reverses impaired wound healing in ischemic limbs. Ann Surg. (2023). doi: 10.1097/SLA.0000000000005949. [Epub ahead of print]37334717
39. Cheng S, Nethi SK, Rathi S, Layek B, Prabha S. Engineered mesenchymal stem cells for targeting solid tumors: therapeutic potential beyond regenerative therapy. J Pharmacol Exp Ther. (2019) 370(2):231–41. doi: 10.1124/jpet.119.259796
40. Muhuri M, Maeda Y, Ma H, Ram S, Fitzgerald KA, Tai PW, et al. Overcoming innate immune barriers that impede AAV gene therapy vectors. J Clin Invest. (2021) 131(1):143780. doi: 10.1172/JCI143780
41. Mingozzi F, High KA. Immune responses to AAV vectors: overcoming barriers to successful gene therapy. Blood. (2013) 122(1):23–36. doi: 10.1182/blood-2013-01-306647
42. Jaluvka F, Ihnat P, Madaric J, Vrtkova A, Janosek J, Prochazka V. Current status of cell-based therapy in patients with critical limb ischemia. Int J Mol Sci. (2020) 21(23):E8999. doi: 10.3390/ijms21238999
43. Gentile P. Autologous cellular method using micrografts of human adipose tissue derived follicle stem cells in androgenic alopecia. Int J Mol Sci. (2019) 20(14):E3446. doi: 10.3390/ijms20143446
44. Lee HC, An SG, Lee HW, Park J-S, Cha KS, Hong TJ, et al. Safety and effect of adipose tissue-derived stem cell implantation in patients with critical limb ischemia: a pilot study. Circ J. (2012) 76(7):1750–60. doi: 10.1253/circj.cj-11-1135
45. Yan H, Mi X, Midgley AC, Du X, Huang Z, Wei T, et al. Targeted repair of vascular injury by adipose-derived stem cells modified with P-selectin binding peptide. Adv Sci (Weinh). (2020) 7(11):1903516. doi: 10.1002/advs.201903516
46. Tang J, Su T, Huang K, Dinh P-U, Wang Z, Vandergriff A, et al. Targeted repair of heart injury by stem cells fused with platelet nanovesicles. Nat Biomed Eng. (2018) 2:17–26. doi: 10.1038/s41551-017-0182-x
47. Sobacchi C, Palagano E, Villa A, Menale C. Soluble factors on stage to direct mesenchymal stem cells fate. Front Bioeng Biotechnol. (2017) 5:32. doi: 10.3389/fbioe.2017.00032
48. Guess AJ, Daneault B, Wang R, Bradbury H, La Perle KMD, Fitch J, et al. Safety profile of good manufacturing practice manufactured interferon γ-primed mesenchymal stem/stromal cells for clinical trials. Stem Cells Transl Med. (2017) 6(10):1868–79. doi: 10.1002/sctm.16-0485
49. Barrachina L, Remacha AR, Romero A, Vázquez FJ, Albareda J, Prades M, et al. Priming equine bone marrow-derived mesenchymal stem cells with proinflammatory cytokines: implications in immunomodulation-immunogenicity balance, cell viability, and differentiation potential. Stem Cells Dev. (2017) 26(1):15–24. doi: 10.1089/scd.2016.0209
50. Huang J, Zhang Z, Guo J, Ni A, Deb A, Zhang L, et al. Genetic modification of mesenchymal stem cells overexpressing CCR1 increases cell viability, migration, engraftment and capillary density in the injured myocardium. Circ Res. (2010) 106(11):1753–62. doi: 10.1161/CIRCRESAHA.109.196030
51. Tsao CW, Aday AW, Almarzooq ZI, Alonso A, Beaton AZ, Bittencourt MS, et al. Heart disease and stroke statistics—2022 update: a report from the American heart association. Circulation. (2022) 145(8):e153–639. doi: 10.1161/CIR.0000000000001052
52. Amini M, Zayeri F, Salehi M. Trend analysis of cardiovascular disease mortality, incidence, and mortality-to-incidence ratio: results from global burden of disease study 2017. BMC Public Health. (2021) 21(1):401. doi: 10.1186/s12889-021-10429-0
53. CDC. Heart disease facts|cdc.gov. Centers for disease control and prevention. Published October 14, 2022. Available at: https://www.cdc.gov/heartdisease/facts.htm (Accessed November 11, 2022).
54. Stewart J, Manmathan G, Wilkinson P. Primary prevention of cardiovascular disease: a review of contemporary guidance and literature. JRSM Cardiovasc Dis. (2017) 6:2048004016687211. doi: 10.1177/2048004016687211
55. Huerta CT, Orr NT, Tyagi SC, Badia DJ, Richie CD, Endean ED. Direct retrograde bypass is preferable to antegrade bypass for open mesenteric revascularization. Ann Vasc Surg. (2020) 66:263–71. doi: 10.1016/j.avsg.2020.01.019
56. Zhao Y, He J, Qiu T, Zhang H, Liao L, Su X. Epigenetic therapy targeting bone marrow mesenchymal stem cells for age-related bone diseases. Stem Cell Res Ther. (2022) 13:201. doi: 10.1186/s13287-022-02852-w
57. Quiroz HJ, Valencia SF, Liu ZJ, Velazquez OC. Increasing the therapeutic potential of stem cell therapies for critical limb ischemia. HSOA J Stem Cells Res Dev Ther. (2020) 6(1):024. doi: 10.24966/srdt-2060/100024
58. Du Y-Y, Zhou S-H, Zhou T, Su H, Pan H-W, Du W-H, et al. Immuno-inflammatory regulation effect of mesenchymal stem cell transplantation in a rat model of myocardial infarction. Cytotherapy. (2008) 10(5):469–78. doi: 10.1080/14653240802129893
59. Ribieras AJ, Ortiz YY, Li Y, Huerta CT, Le N, Shao H, et al. E-Selectin/AAV2/2 gene therapy alters angiogenesis and inflammatory gene profiles in mouse gangrene model. Front Cardiovasc Med. (2022) 9:929466. doi: 10.3389/fcvm.2022.929466
60. Ribieras AJ, Ortiz YY, Li Y, Le NT, Huerta CT, Voza FA, et al. E-selectin/AAV gene therapy promotes myogenesis and skeletal muscle recovery in a mouse hindlimb ischemia model. Cardiovasc Ther. (2023) 2023:e6679390. doi: 10.1155/2023/6679390
61. Dobrovolskaia MA, Bathe M. Opportunities and challenges for the clinical translation of structured DNA assemblies as gene therapeutic delivery and vaccine vectors. Wiley Interdiscip Rev Nanomed Nanobiotechnol. (2021) 13(1):e1657. doi: 10.1002/wnan.1657
62. Suzuki J, Shimamura M, Suda H, Wakayama K, Kumagai H, Ikeda Y, et al. Current therapies and investigational drugs for peripheral arterial disease. Hypertens Res. (2016) 39(4):183–91. doi: 10.1038/hr.2015.134
Keywords: targeted cell delivery, mesenchymal stem cell, stem cell therapy, cell-based therapy, cardiovascular disease, chronic limb-threatening ischemia, myocardial infarction
Citation: Huerta CT, Voza FA, Ortiz YY, Liu Z-J and Velazquez OC (2023) Targeted cell delivery of mesenchymal stem cell therapy for cardiovascular disease applications: a review of preclinical advancements. Front. Cardiovasc. Med. 10:1236345. doi: 10.3389/fcvm.2023.1236345
Received: 7 June 2023; Accepted: 25 July 2023;
Published: 4 August 2023.
Edited by:
Johnson Rajasingh, University of Tennessee Health Science Center (UTHSC), United StatesReviewed by:
Vincent Salvatore Gallicchio, Clemson University, United StatesSatish Ramalingam, SRM Institute of Science and Technology, India
© 2023 Huerta, Voza, Ortiz, Liu and Velazquez. This is an open-access article distributed under the terms of the Creative Commons Attribution License (CC BY). The use, distribution or reproduction in other forums is permitted, provided the original author(s) and the copyright owner(s) are credited and that the original publication in this journal is cited, in accordance with accepted academic practice. No use, distribution or reproduction is permitted which does not comply with these terms.
*Correspondence: Zhao-Jun Liu emxpdUBtZWQubWlhbWkuZWR1 Omaida C. Velazquez b3ZlbGF6cXVlekBtZWQubWlhbWkuZWR1
Abbreviations AD-MSCs, adipose-derived mesenchymal stem/stromal cells; BM-MSCs, bone marrow-derived MSCs; CVD, cardiovascular diseases; CSC, cardiac stem cell; CAM, cell adhesion molecule; CLTI, chronic limb-threatening ischemia; CSF2, colony-stimulating factor 2; CLMP, coxsackie- and adenovirus receptor-like membrane protein; EF, ejection fraction; ESL, E-selectin ligand; FGF, fibroblast growth factor; HGF, hepatocyte growth factor; HCELL, hematopoietic cell E-/l-selectin ligand; MMPs, matrix metalloproteinases; MSCs, mesenchymal stem/stromal cells; MI, myocardial infarction; PINC, platelet-inspired nanocell; PBP, P-selectin binding peptide; PGE2, prostaglandin E2; SPIOs, superparamagnetic oxide nanoparticles; SDF-1α, stromal cell-derived factor 1 alpha; TGF-β, transforming growth factor beta; VEGF, vascular endothelial growth factor.