- 1USM-ALPS Cardiac Research Laboratory, Advanced Medical and Dental Institute, Universiti Sains Malaysia, Penang, Malaysia
- 2Henan Key Laboratory of Cardiac Remodeling and Transplantation, Zhengzhou Seventh People's Hospital, China
- 3Henan Key Laboratory of Medical Tissue Regeneration, Xinxiang Medical University, China
- 4Celestialab Sdn Bhd, Kuala Lumpur, Malaysia
- 5Department of Human Anatomy, Faculty of Medicine and Health Sciences, Universiti Putra Malaysia, Serdang, Malaysia
Myocardial infarction is the most common cause of heart failure, one of the most fatal non-communicable diseases worldwide. The disease could potentially be treated if the dead, ischemic heart tissues are regenerated and replaced with viable and functional cardiomyocytes. Pluripotent stem cells have proven the ability to derive specific and functional cardiomyocytes in large quantities for therapy. To test the remuscularization hypothesis, the strategy to model the disease in animals must resemble the pathophysiological conditions of myocardial infarction as in humans, to enable thorough testing of the safety and efficacy of the cardiomyocyte therapy before embarking on human trials. Rigorous experiments and in vivo findings using large mammals are increasingly important to simulate clinical reality and increase translatability into clinical practice. Hence, this review focus on large animal models which have been used in cardiac remuscularization studies using cardiomyocytes derived from human pluripotent stem cells. The commonly used methodologies in developing the myocardial infarction model, the choice of animal species, the pre-operative antiarrhythmics prophylaxis, the choice of perioperative sedative, anaesthesia and analgesia, the immunosuppressive strategies in allowing xenotransplantation, the source of cells, number and delivery method are discussed.
1. Introduction
Heart failure (HF) is a disease in which the ventricular filling and/or myocardial contraction are compromised due to irreversible pathological modelling in the cardiac structure and function (1–3). Ischemic heart disease is the most common cause of HF. More than 26 million people are living with a failing heart worldwide (4), and 1 in every 8 reported deaths was due to HF (5). This prevalence is expected to increase by 46% in 2030 (6, 7). The most common cause of HF is ischemic heart disease. Myocardial ischemia as a result of the blockage in the coronary artery can affect myocardial contractility, and electrical conduction, and alters cardiac energetics (8). Pathological hypertrophic remodelling of the left ventricle, fatal cardiac tachyarrhythmia (9) followed by a cascade of secondary damage including inflammatory reaction, myocardial cell rupture and fibrotic scarring could collectively cause an abrupt decrease in left ventricular ejection fraction (LVEF) and failure. Despite technological advancement and all the current pharmacological-based treatment options, effective therapy that could prevent damaged hearts from remodelling and failure is still lacking. Heart transplantation is the only cure for end-stage heart failure, but the therapy is challenged by the lack of donor and graft rejection.
Stem cells have been the research interest and hope to remuscularize the weakening, injured myocardium and reverse cardiac remodelling. Compelling evidence has shown that pluripotent stem cells (hPSCs), such as embryonic stem cells (ESCs) or induced pluripotent stem cells (hiPSCs) offer the indefinite source of cardiomyocytes and are the only cells which can be scaled up to produce a clinical relevant number for remuscularizing injured myocardium. Studies have shown that intramyocardially injected hPSCs-cardiomyocytes engrafted, integrated synchronously with the host myocardium, regenerated the remodelled, thin myocardium and improved cardiac function. Whilst the clinical benefits of other adult stem cells such as mesenchymal stromal cells or cardiac-derived progenitor cells in cardiac regeneration are widely acknowledged (10, 11), the extent of remuscularization in the injured hearts was generally far more encouraging using hPSC-cardiomyocytes, as demonstrated in the past studies (12–15).
To establish a suitable disease model for reliable experimentation, investigators need to take into account the animal species of choice, the subject availability, the cost and the similarity/difference between the human subject as well as the methods to induce myocardial injury that produces the appropriate pathological microenvironment mimicking clinical conditions which is suitable for testing therapeutic remuscularization intervention. Table 1 summarizes the different considerations in the use of small animals (mice, rats) and large animals (pigs, dogs, monkeys) for modelling ischemic heart disease.
2. Cardiac remuscularization study using large animals – the importance and rationale
An ideal disease model should mimic the pathophysiology in humans in order to more accurately and reproducibly examine any novel therapy that would successfully translate into clinical applications. In preclinical research, any new therapy would first be tested in small animal models. This is because small animals such as rodents have a short life cycle, hence requiring low maintenance cost and high availability are instrumental in producing statistically meaningful analysis within a comparatively shorter time over the use of large animals (Table 1). An early rat HF model was established by Pfeffer et al. using coronary artery ligation (16). The groundbreaking study dated back to 1979 also served as an important foundation for the development of the successfully translated drug Captopril, the angiotensin-converting enzyme that profoundly improved heart function and survival in post-myocardial infarction (MI) patients (17).
Nonetheless, the differences in heart anatomy, size, hemodynamic characteristics and responses to drugs or treatment between small animal models and humans are likely the cause of failed translation to clinical trials. These differences could also explain the rather disappointing clinical results observed in most major human stem cell therapy trials, despite the overwhelmingly positive outcome and optimism reported in laboratory small animal studies. In 2016, Zwetsloot et al. presented a systematic review and meta-analysis of preclinical studies involving cardiac stem cell treatment in MI animal models (18). They concluded that the magnitude of effects of CSC treatment in the small animal MI model was found to be greater than that of large animals. Moreover, the recent incidence of cardiac arrhythmias reported from non-human primate and pig studies following human cardiomyocyte transplantation was not previously observed in small animals possibly due to their high heart rate (13, 19, 20). Hence, small animal studies may offer important preliminary insights about the tested treatment, but a reassessment of its therapeutic efficacy must be performed on large animals whose systems are physiologically and anatomically more resembles humans prior to starting clinical trials. This is also in line with the recommendations by the transnational alliance for regenerative therapies in cardiovascular syndromes (TACTICS) international group that large mammals should be used as confirmatory studies in view of their resemblance to human disease (21).
3. Strategies to induce myocardial infarction in large animals
Many strategies have been introduced to create MI and HF models in large animals, with the primary objective to occlude major coronary vessels and induce ischemic injury. These include invasive thoracotomy-enabled permanent left anterior descending (LAD) coronary ligation, reversible LAD coronary artery ligation ischemic reperfusion-induced myocardial injury, coronary micro-embolism, hydraulic occluder or ameriod constrictors, or less-invasive percutaneous transluminal coronary angioplasty (PTCA) balloon occlusion-reperfusion of the coronary artery (Table 2). Nevertheless, the most common strategies employed in recent preclinical remuscularization studies using large animals were mainly the thoracotomy/permanent LAD ligation (24) or ischemic reperfusion (30) and PTCA-assisted balloon occlusion/reperfusion of LAD contrary artery (20). Unlike others, these methods allow occlusion to take place at the specific location of the LAD coronary arteries and produce predictable, consistent infarct size which is pivotal for remuscularization study. Notably, the mortality of the LAD occlusion method in large animals is considerably high as they are prone to surgical-induced trauma, high risk of bleeding and developing fatal ventricular fibrillation following MI (35).
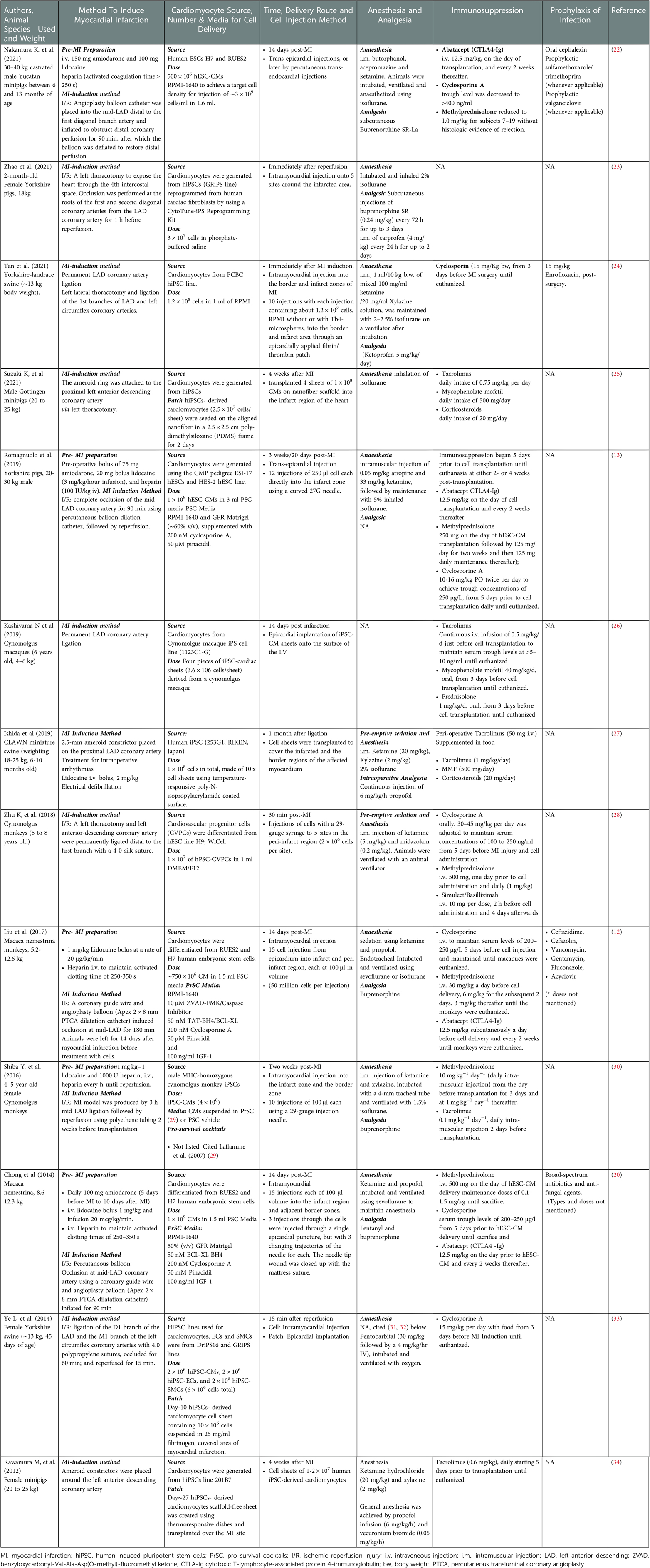
Table 2. Summary of the methodologies used in in vivo cardiac remuscularization studies using large animals.
For assessing the efficiency and efficacy of cardiomyocyte therapy on cardiac remuscularization, the animal model must develop clear infarction to create the need for cellular reconstitution. Methods such as coronary microembolism, pacing-induced tachycardia and toxic injury can induce dilated cardiomyopathy even without the presence of clear infarcts, making them less common methods used for the cardiac remuscularization-related study (36).
3.1. Irreversible occlusion of left anterior descending coronary artery
Among the most commonly used methods for modelling MI in large animals is by surgically ligating the LAD coronary artery (37), with or without reperfusion (35). This method requires invasive thoracotomy and complex surgical procedures to minimize unnecessary tissue injury, infection and animal suffering that allow good post-operative recovery. The advantage of this method is the convenience of getting a direct visual of the heart anatomy to identify and choose the site of ligation along the LAD coronary artery since the ligation site determines the resultant infarct size, as well as the mortality rate of the animal (38, 39). In Tan et al. (2021) study, they performed a permanent LAD coronary ligation without reperfusion on Yorkshire-landrace swine (∼13 kg) heart, with the ligature placed at the first branches of LAD and left coronary circumflex (24). This led to an ejection fraction of ∼40% with a scar size of 15% in the swine MI model. Some studies presented rather arbitrary and confusing descriptions in their methodology especially the choice of ligation site, which may explain the inconsistencies that complicate the inter-study analysis (40). In Kashiyama et al. study, they reported using permanent LAD coronary artery ligation in cynomolgus macaques (6 years, 4–6 kg) but without specifying the exact segment of LAD coronary where the ligature was placed (26). In their study, the ejection fraction was found to decrease by 30% in the control macaques.
Noteworthy that in MI models, there are differences in the coronary collateral perfusion network in the animal species of choice which can significantly affect the resultant infarct size and the extent of the ischemic bed. In swine and non-human primates, the coronary arterial architecture with limited collateral vessels is similar to that of humans (41, 42). Therefore, a ligation at a specific coronary segment can produce consistent and predictable infarct size. The only setback in using the swine model is the rapid changes in body weight from juveniles and this may complicate long-term analysis as this alters the baseline of swine cardiac physiology (43). Canine, on the other hand, has an extensive collateral coronary network (44). Placement of the ligature at the similar coronary artery segment may not produce the intended, consistent infarct size (45), because the infarct size decreases as the collateral flow in the canine heart increases (46).
3.2. Gradual LAD coronary occlusion using ameriod constrictor
Ameroid constrictor was first introduced in 1957 (47), consisting of an inner hygroscopic casein ring that, upon fluid absorption, gradually expands against a fixed metal sheath and occludes the vessel where it is placed (48). This method induces chronic progression of coronary artery disease and develops chronic myocardial ischemia in large animals, especially in pigs. LAD occlusion using an ameroid constrictor demonstrated a steady decline in cardiac function from 8 to 12 weeks, with a 50% reduction in the ejection fraction (49), inducing chronic HF in the mini swine model with high reproducibility (50). Ameroid constrictor comes in various sizes to fit the diameter of the vessel of interest which is to be constricted. A study has shown that different device size (tested 2.25, 2.50 and 2.75 mm) has minimal effects on the resultant coronary flow and ischemic area following the constriction in pigs (51). This may not be the case in the canine model as the collateral vessels gradually develop and reduce the regional ischemia and infarct size (51). The modelling of chronic coronary stenosis using ameroid constrictors in large animals is generally less popular due to the laborious animal care and maintenance cost underlying the study.
3.3. Transient LAD occlusion to induce ischemic/reperfusion injury
In clinics, rapid recovery of coronary blood flow through balloon angioplasty is one of the treatment strategies for acute MI. Ischemic/reperfusion (I/R) injury is a paradoxical phenomenon where cellular injury results from the rapid restoration of blood flow after prolonged oxygen deprivation. This is due to the abrupt surge in oxygen level, which increases the production of free radicals and calcium influx that causes mitochondrial damage (52, 53). This is followed by an accelerated inflammatory response which deteriorates cardiac functions and causes irreversible myocardial cell death (54). To model this pathological process in animals, temporary obstruction of LAD flow could be achieved by ligating the LAD coronary artery using a silk suture with the ligature tied against a piece of polyethene tubing parallel to the artery (55). Releasing the polyethene tube would then allow reperfusion and introduce reoxygenation injury. Alternatively, cardiac ischemia-reperfusion injury can also be achieved using a minimally invasive intervention without thoracotomy, by using an inflatable intracoronary balloon catheter (12, 13, 22). Although this method omits thoracotomy and reduces invasive tissue trauma, a specialized skillset and expensive facility are also required in performing the procedure under fluoroscopy.
In the I/R injury model, the length of the induced ischemic time (or the coronary artery occlusion time) also affects the outcome of the infarct size, in addition to the location of LAD coronary artery blockage as previously mentioned. In a recent study by Silvis et al. (2021), they examined the effects of different coronary artery balloon occlusion times (60, 75 and 90 min) and the reperfusion time on the myocardial infarct size in pigs (56). They showed a positive correlation between ischemic time and infarct size determined by the area at risk. However, a longer reperfusion time after the occlusion of 75 min, examined after 1, 3 and 7 days, did not affect or exacerbate the infarct size further. In short, the occlusion time of 30–180 min in duration has proven to cause significant ischemic-induced infarction in a large animal (57, 58). In most recent studies involving cardiac remuscularization study using human cardiomyocytes, the occlusion time used in establishing the I/R MI model were 60 min (swine) (33), 90 min (swine or non-human primates) (13, 22) and 180 min (non-human primates) (30).
3.4. Pre-MI induction preparation to reduce the incidence of fatal arrhythmias
The incidence of arrhythmias is common in MI models, especially the two most commonly used large animal species swine (59) and non-human primates. In the anaesthetized MI porcine model, fatal arrhythmia following coronary occlusion was found to be almost inevitable (38). Approximately 15%–40% of MI models in pigs died of ventricular tachycardias (VT) and ventricular fibrillation (VF) minutes after coronary occlusion (59, 60). Some studies eliminated VF upon onset of MI in pigs by defibrillation at 200 J (56, 61).
3.4.1. Amiodarone
Amiodarone is a class III antiarrhythmic drug and non-competitive beta-blocker agent indicated to treat cardiac dysrhythmias and it has been used as a prophylaxis means to prevent VF during the early onset of MI (62), and to treat VF post-AMI. The study has also shown that amiodarone, in combination with lidocaine the anaesthetics and class 1b antiarrhythmic agent (63, 64), reduced the incidence of fatal arrhythmia in the ovine MI model (65). This combination of prophylaxis regimen has also been adopted by Murry's laboratory in their macaque MI study using human embryonic stem cells-derived cardiomyocytes in 2014 (20). They administered 100 mg amiodarone daily for 5 days prior to MI induction to 10 days after MI induction through the oral route, in addition to using lidocaine bolus at 1 mg/kg and by infusion at 20 μg kg−1 min−1. The same laboratory removed amiodarone from their standard of prophylaxis care in their recent report in 2018 but used it in one animal with refractory VF/VT (bolus intravenous infusion) at 85 min of ischemia and given again in combination with lidocaine (75 mg) and dopamine (10 μg kg−1 min−1) for 24 h after reperfusion due to sinus tachycardia (12). However, they also acknowledged the omission of the amiodarone from their study accounted for a higher incidence of arrhythmias pre-cell injection.
3.4.2. Heparin
Heparin is an anticoagulant with heterogenous size and activity (66).– The primary mechanism of action of heparin is to bind and enhance the activity of antithrombin III and is required for performing percutaneous coronary intervention procedures during I/R modelling in large animals similar to the clinical practice, in order to reduce thrombosis (67, 68). Some studies also infused heparin before introducing I/R injury even in thoracotomy-open chest coronary artery ligation surgery when PCI was not used (30), to maintain a high activated clotting time (∼250–350 s) (69), which were found to inversely correlated with the likelihood of abrupt vessel closure (70, 71).
4. Choice of sedative, anesthesia and analgesia
The surgical procedures required to perform in vivo cardiac remuscularization studies are mostly invasive, some of which require a thoracotomy, percutaneous coronary intervention, and for enabling direct intramyocardial cell injection. Handling large animals to prepare them for these procedures is challenging and inhumane without proper and effective perioperative sedation, general anaesthesia and analgesia. The outcome of infarction in swine was also reported to be affected by the choice of anaesthesia and breed (72). Employing a suitable anaesthetic strategy during MI modelling in the large animal is important for achieving adequate anaesthesia during the induction of the intended pathophysiological changes, as well as securing stable post-surgery hemodynamic and recovery, and reducing the risk of anaesthesia-related mortality due to malignant hyperthermia or complications following long anaesthesia (73). Here, the choice of pre-emptive sedatives, anaesthesia and analgesia used in large animal studies in Table 2 are discussed.
4.1. Pre-emptive sedation and analgesia
Large animals, such as porcine, canine or nonhuman primates require sufficient restraint for any interventions without jeopardizing the safety of the handlers. Ketamine is a widely used intramuscular administered, non-competitive N-Methyl-D-aspartate (NMDA) receptor antagonist and dissociative anaesthetic of which the state of anaesthesia is cataleptic with intense amnesia, analgesia and hypertonus (74). It is a Class III controlled substance governed by the Drug Enforcement Agency (DEA) in the United States, as well as regulated by the local authority of many countries like Malaysia.
Ketamine is more widely used for less painful interventions but offers good restraint in large animals e.g., blood collection. It is a known irritant upon intramuscular injection due to its acidity and has a high risk of developing neuronal damage and loss of sensation in rhesus macaques (75) or muscle damage in marmosets (76). It is also a direct negative inotrope and has the ability to inhibit neuronal and extra-neuronal catecholamine uptake (77). Ketamine provides fast-acting and rapid-onset sedation (78) due to its high hydrosoluble characteristic that allows a rapid increase in bioavailability in the central nervous system (79).
Most procedural sedation and pre-emptive analgesic strategy used in the studies summarized in Table 2 included ketamine prior to the induction of any inhalational anaesthetic agent. However, none of them administered ketamine as monotherapy. Instead, ketamine was combined with other drugs with analgesic effects such as xylazine, midazolam, atropine or propofol. This is because gas anaesthesia alone provides no pain control and can be stressful to the subject upon its withdrawal and recovery. Combinations with xylazine the α2-agonist or other NMDA-antagonist such as diazepam or midazolam can achieve good sedation and excellent analgesia. The combinations also showed to prevent seizures and promote muscle relaxation (80). One of the advantages of using both combo ketamine/xylazine and ketamine/midazolam could abrogate the swallow reflex during endotracheal intubation (81).
Ketamine can cause hypersalivation in large animals and humans (82). This can be addressed by co-administering anticholinergics atropine (82). Furthermore, other combination such as ketamine/propofol, collectively called “Ketofol”, is used for short procedural sedation (12, 20). Propofol is a sedative-hypnotic agent with good sedation and muscle relaxation effects which could address the observed ketamine-induced muscle spasm in rhesus macaques (83). Ketofol has also been found to reduce adverse respiratory events as compared to propofol treatment alone (81), as well as demonstrated neuroprotective and anti-inflammatory responses in mice with toxic status epilepticus (84). However, the combination was also associated with a high incidence of tachycardia (85, 86).
A ketamine/acepromazine/butorphanol procedural analgesia mix was used by Nakamura et al. (2021) in minipigs. Acepromazine is a phenothiazine tranquillizer of which sedation is achieved via inhibiting alpha-adrenergic, dopamine receptors in the central nervous system. It provides only mild to moderate sedation in pigs and requires a normal liver function to be metabolised and excreted through the kidneys. Acepromazine is not by itself an analgesic but it can enhance the effects of analgesic opioids, and in this instance, butorphanol (87). It also prolonged the anaesthetic effects of ketamine and reduced the proportionate dosage of ketamine (88).
4.2. Inhalation anaesthetic
The two most common inhalational anaesthetic agents used in the recent cardiac remuscularization studies in large animals are isoflurane and sevoflurane (Table 2). Isoflurane is a widely chosen inhalational anaesthesia for experimental interventions. However, it is also known for its dose-dependent depression of cardiac performance. This can be resolved by adding nitric oxide to isoflurane anaesthesia to reduce the depressant effects on the heart. Studies have also shown that isoflurane neither contributes to coronary steal at clinically-meaningful concentrations (89) nor causes myocardial ischemia in dogs (90). In fact, isoflurane has been demonstrated to have protective effects against myocardial I/R injury (91). Sevoflurane shares similar characteristics to isoflurane, but it exerts dose-dependent depression of the cardiac functions such as stroke volume, cardiac output or left ventricular contractility without affecting the heart rate (92). Noteworthy, sevoflurane was found to have a 30% lower incidence of VF than isoflurane, as well as comparatively greater hemodynamic stability and lower mortality in a porcine I/R study.
4.3. Post-operation analgesia
Buprenorphine and fentanyl are the two opioid analgesics of choice in large animal studies involving invasive surgery. Buprenorphine is a µ (mu) receptor partial agonist but the binding is strong and hence the effect is long-lasting and able to displace other short-acting pure μ agonists (93). It is also a weak κ (kappa) receptor antagonist, making it a weak inducer of opiate effects. Fentanyl, on the other hand, is short-acting, but a more potent, pure µ-receptor agonist. Both opioids have little effect on the heart (94, 95), but buprenorphine is more favourable than fentanyl because it causes less respiratory depression at high doses with a ceiling effect (96). Whereas fentanyl demonstrated dose-dependent respiratory depression at high doses.
Ketoprofen and carprofen are non-steroidal anti-inflammatory drugs (NSAIDs) drug with analgesic effects and have lower toxicity profiles in animals than other NSAIDs. Both are propionic acid derivatives acting through the inhibition of cyclooxygenase and impedes prostaglandins biosynthesis. These NSAIDs were found effective in postoperative pain relief in dogs (93) but have reported the risk of gastrointestinal complications including stomach ulcers (97). Ketoprofen is also known to affect platelet aggregation and care should be taken in case of gastrointestinal bleeding (98).
5. Cardiomyocyte source, route of delivery and cell number
Successful remuscularization of an injured heart requires promising regeneration of the dead myocardial tissue in order to restore the myocardial muscle density and contractile strength. Many cell candidates have been tested in laboratories or clinics (10), but this review will only discuss the past animal studies that used human cardiomyocytes derived from hiPSCs. Methods and the efficiency of deriving functional cardiomyocytes from human PSCs have been improved substantially, either by using growth factors, small molecules, or combinations of both at a defined culture time (99).
A myriad of methods has been introduced to administer cells into the heart, with an aim to maximize cell homing, retention, engraftment and subsequent survival and function. Previously, cell administration via the systemic intracoronary route in cynomolgus monkeys has proven inefficient with a high incidence of embolism and poor graft survival (100). Local intramyocardial route, however, is the most favourable method to deliver cardiomyocytes, either via trans-endocardial or trans-epicardial injection. The trans-endocardial route is considerably less invasive than other local injection methods as it can be achieved via the percutaneous catheter. The unique challenge in implanting cells into the myocardium is the characteristic of the heart being a constantly contracting organ, which creates the mechanical force that squeezes the injected cells out through the needle track (the “washout” effect) or the broken blood vessels because of direct injection (101, 102).
To achieve high cell retention, epicardial implantation of a cardiac patch may be considered. The only shortcoming of this delivery method is the inevitable, invasive thoracotomy required for the implantation. Recently, a minimally invasive intrapericardial cell injection was proposed (102). The authors performed the procedure through two small incisions (one for insertion of a camera probe and another for a needle with exosomes in hyaluronic acid hydrogel) on the pig chest wall and showed minimal inflammation. They also tested the delivery method using hiPSC-derived cardiac progenitor cells in decellularized porcine heart matrix hydrogel and demonstrated promising cell engraftment on the epicardial surface, minimal immune response as well as the evidence of in vivo cardiomyocytes differentiation of the injected cardiac progenitors. However, this reported benefit was only tested and observed in rat hearts.
Studies have shown that the number of transplanted cardiomyocytes determines the degree of remuscularization in the injured heart (103). The range of cardiomyocyte numbers which were tested in the∼40 g macaque infarcted hearts was between 400 and 1,000 million human induced pluripotent stem cells-derived cardiomyocytes, through intramyocardial injection (12, 20, 30). Assuming the average monkey body weight in those studies was 9 kg, the dose for every kg body weight in a human would be 44–111 million cells or 3–7 billion cells in an adult human with an average body weight of 70 kg. However, these numbers remain inconclusive, as a question was raised about the clinical relevance in proportion to the size of a human heart of which the left ventricle contains only∼5 billion cardiomyocytes (104).
Another common approach in preclinical CM transplantation studies in addition to intramyocardial injection is by epicardial implantation of engineered heart tissue. A recent study by Querdel et al. (2021) showed that EHT made of high cardiomyocyte dose (1.5 × 2.5 cm, 12 × 106 cardiomyocytes) improved heart function in guinea pigs, with in situ time-dependent cardiomyocyte proliferation within the implanted EHT (103). The authors also claimed to have successfully upscaled to generate a 7 × 5 cm human-relevant-sized EHT with 450 million cells for clinical use. In a MI study using Gottingen minipigs (weight 20–25 kg each), Suzuki et al. (2021) transplanted four large, 2.5 × 2.5 cm cardiac tissue made with 2.5 million cardiomyocytes on the aligned nanofibers to the infarcted myocardium (1 billion cells in total, 50 million kg−1 for a 20 kg minipig) (25). They concluded the treatment improved cardiac function and angiogenesis with antifibrotic effects but low engraftment, possibly due to immune rejection.
6. Strategies to overcome xenogenic cell immune rejection and immunosuppression
In most cases, the established MI animal models used for testing the regenerative capability of any cell candidate were from xenogenic sources, e.g., human cardiomyocytes to swine or macaques' hearts. One of the key determinants of successful clinical use of cardiomyocyte therapy is dictated by the degree of engraftment and survival of the transplanted xenogenic cells and this outcome is affected by the immunologic responses of the host recipient upon transplantation. Most of the preclinical cell therapy experimentation involves xenotransplantation (12). Transplantation of non-autologous cells can result in immune reactions that are primarily caused by acute cellular rejection, mainly because of the T cell alloantigen recognition of the major histocompatibility complex (MHC) (105). Some allogenic cell candidates may have the ability to evade immunorecognition and avoid graft rejection, like the mesenchymal stromal cells (MSCs). These cells are known to be immunomodulatory-privileged and can effectively modulate the immune system by inhibiting T cell proliferation or maturation after transplantation (106). This suggests that MSC transplantation might not need immunosuppression even if the cells are of an allogenic source (107). However, such privilege was found to be withheld when the cells were differentiated (108).
The very original concept of the creation of human iPSCs was the possibility to derive them from autologous sources, and offering cell therapy with the patient's own cells for transplant would resolve the problem with immunorejection (109, 110). However, the high cost underlying each iPSC line generation and the significantly longer time (months) required for differentiation and up-scaling may not be feasible for some clinical conditions which in need of immediate treatment. Hence, getting a universal human iPSC line that could serve “off-the-shelves” would make cell therapy more readily available for the use of broader patients.
The idea of cryobanking human induced pluripotent stem cells (iPSCs) generated from HLA homologous donors matching for human leukocyte antigen (HLA)-A, HLA-B, and HLA-DR alleles has been first advocated in the United Kingdom (111). Instead of using autologous cells, matching the compatibility of the allogeneic iPSCs based on these three most notorious triggers of immune rejection would turn the cell lines transplantable for a larger patient population with good graft survival (111, 112). However, this approach may only apply to countries in which the population has low diversity in HLA haplotypes (113). Moreover, the conflicting finding was also observed in iPSC-derived cardiomyocytes which revealed the need for immunosuppressants despite the reduced immune reactivity due to MHC matching (114).
In 2019, Schrepfer's laboratory revealed that generating allogeneic human iPSCs with hypo-immunogenicity is in fact possible (115). These allogeneic human iPSCs inactivated their major histocompatibility complex MHC class I and class II genes (B2M and CIITA, respectively), as well as upregulated the non-MHC ligand CD47 to silence innate immunity. They tested the hypoimmunogenic human iPSCs in allogenic humanized NSG-SGM3 mice and showed the successful formation of teratoma. The iPSC differentiated derivatives, endothelial and cardiomyocytes from the same hypoimmunogenic line also showed similar survival in the mice up to 50 days, confirming engineering process did not compromise the iPSC function and its hypo-immunogenicity. Cowen's laboratory also suggested the removal of CIITA in human PSCs but they proposed selective deletion of HLA-A/-B/-C instead of B2M to preserve the expression of HLA-E and HLA-G, the HLA class Ib molecules that retain the tolerance to natural killer (NK) cells (116). They also introduced the expression of PD-L1 (T cell checkpoint inhibitor) and HLA-G in addition to CD47. In their findings, these modifications were able to be protected from the immunosurveillance of T cells, NK cells and macrophages.
6.1. Drug-induced immunosuppression
While awaiting to materialize the use of hypoimmunogenic hiPSC lines in clinics, an immunosuppression regimen is needed for any allogenic cell transplantation in the current in vivo large animal studies or in human studies. Immunosuppressive agents are used either alone or in combinations to eliminate the effect caused by host immune rejection following cell transplantation. Calcineurin inhibitors and glucocorticoids are the two common types of immunosuppressive drugs used in allogeneic cell transplantation studies in large animals and using the two in combinations is a more preferred strategy as it yielded superior effects than using a single, individual drug approach.
6.1.1. Calcineurin inhibitor
Calcineurin is calcium and calmodulin-dependent serine/threonine protein phosphatase which activates T cells (117). Calcineurin inhibitor is commonly seen as the choice of immunosuppressive agent in many large animal preclinical studies of cardiac regenerative therapy, such as cyclosporine A (CsA) or tacrolimus (Table 2, Immunosuppression). CsA binds to cyclophilin while tacrolimus binds to immunophilin FK506 binding protein 12 (FKBP12) in the cytoplasm. Both complexes prevent the downstream calcineurin-calmodulin complex-mediated dephosphorylation of nuclear factor of activated T-cells (NFAT) and upregulation of interleukin-2 (IL-2), the key cytokine which activates T cell proliferation. Some studies supported that CsA can enhance the immunosuppressive capability of MSCs, but the evidence is only limited to in vitro observations (118, 119). A study was performed to examine the serum level for animal safety after CsA administration (15 mg−1 day−1, twice daily via oral route) in the I/R pig model (weight ∼33 kg) (120). All readings of the serum levels were found within the reference value, suggesting that the dose is safe to keep CsA serum concentration at 82%. This CsA dosage is still adopted in many recent pig MI studies (24, 33). In non-human primates, CsA was given to maintain a serum trough level of 200–250 μg/L (12, 20). Tacrolimus is also used to replace CsA in case of recurrent rejection (121) and has fewer side effects that are seen in CsA like hypertrichosis and gingival hyperplasia (121).
6.1.2. Steroid
Glucocorticoids are steroid hormones (prednisone and methylprednisolone) that modulate the gene expression of T and B cells, and some other nucleated cells that illicit acute immune rejection. The binding of prednisolone to glucocorticoid receptors on transplanted cells inhibits the downstream nuclear factor-κВ mediated expression of growth factors and secretion of proinflammatory cytokines (122). The binding inhibits the proliferation of several leukocytes including T and B cells, monocytes, macrophages and granulocyte, and made steroids a standard immunosuppressant for heart transplant recipients for induction and maintenance of immunosuppressive state in recipient patients. Long-term consumption of glucocorticoids is also coupled with undesired side effects on the heart as well as other organs (123).
6.1.3. Antibodies
Abatacept is a human cytotoxic T-lymphocyte antigen (CTLA4)-Ig fusion protein which was initially developed as an inhibitor of the CD28/B7 pathway (BMS-188667) (124). The antibody is used to block the interaction of CD80 and CD86 of the antigen-presenting cells with CD28 costimulatory molecule on T cells to prevent its activation. In primates, CTLA4-Ig was tested and found to be effective in suppressing the acute rejection of MHC-mismatched renal allografts (125) and preventing the antibody formation against ovine red blood cells (126) in cynomolgus monkeys.
Basiliximab and daclizumab are chimeric human/murine monoclonal antibodies both targeting the alpha chain of CD25 high-affinity interleukin2 receptor of T cells, and preventing activation of T cells (127). The monoclonal antibody is largely used in renal transplantation with a significantly low acute rejection rate, which also allows rooms to lower the dosage of calcineurin inhibitors or steroids postoperatively.
6.1.4. Mycophenolate mofetil
Mycophenolate mofetil (MMF, or RS-61443), and its hydrolyzed active form mycophenolic acid, inhibits inosine-monophosphate-dehydrogenase isoenzymes I and II, the rate-limiting enzymes crucial in de novo guanosine nucleotide synthesis (128). The inhibition of purine synthesis impedes the proliferation of stimulated T-lymphocytes, as well as the vessel cells such as smooth muscle cells (129), fibroblasts (130) and endothelial cells (131). Unlike other immunosuppressants, MMF can also reduce the prevalence of vascular graft disease, one of the main causes of allograft failure due to the progressive development of intimal hyperplasia. In a swine study, MMF also showed to abrogate cardiac allograft vasculopathy and increased graft survival (132). MMF is known to cause gastrointestinal intolerance or toxicity and this serves as the basis of the MMF dose reduction in patients who underwent allotransplantation (133). Studies have also shown that MMF dose reduction also increased the incidence of sustained rejection (134).
6.1.5. Multiple drug regimen
In most of the cardiomyocyte transplantation studies using large animals, immunosuppression was achieved with multiple drug regimens (MDR) which combine multiple types of immunosuppressants. In a study by Zhu et al. (2018)., the group tested cardiovascular progenitor cell (CVPC) transplantation into an MI cynomolgus monkey model (28). In their study, they found that cyclosporine (30–45 mg/kg) alone could not effectively reduce immune rejection of CVPCs. This outcome was greatly improved by delivery of a multiple-drug regimen (MDR) consisting of cyclosporine (30–45 mg/kg/day), methylprednisolone (1 mg/kg/day with a loading dose of 500 mg), and basiliximab (1.5 mg/kg/day from day 1 till day 4), evident by the presence of the transplanted cells after 28 days of transplant. Yet, the transplanted cells were not detected after 140 days. On the other hand, Murry's laboratory used cyclosporin, methylprednisolone and abatacept in their human cardiomyocytes-to-macaque heart studies (12, 20, 22). They reported no evidence of all rejection with their MDR. In line with the finding, Romagnuolo and colleagues (2019) who employed the same MDR combination also showed minimal cellular rejection based on the grading criteria for human heart allografts (13).
Some interesting modifications in Murry's MDR were noticed comparing their two macaque studies and one study in swine, particularly the dosage of methylprednisolone. In Chong et al. (2014), methylprednisolone was given at 500 mg on the day of cell administration, and the dosage was maintained at 0.1–1.5 mg/kg until the animals were sacrificed. They did not observe any graft rejection. However in Liu's study (2017), methylprednisolone was reduced to 30 mg/kg on the day of cell delivery, and the maintenance dose was adjusted/increased to 6 mg/kg for the subsequent 2 days and 3 mg/kg until the animals were sacrificed. One graft rejection was observed due to interrupted immunosuppression as a result of a damaged intravenous catheter. In their pig study, the dosage of methylprednisolone was, once again, adjusted to 3.0 mg/kg 2 days before transplantation until 2 weeks, down to 1.5 mg/kg for subsequent maintenance. The dosage of methylprednisolone was further reduced to 1.0 mg/kg in some experimental subjects due to complications by porcine cytomegalovirus and pneumocystis pneumonia. The reason behind these substantial changes in methylprednisolone from 2014 was not mentioned, despite the consistent dosage of the other two immunosuppressants Abatacept and cyclosporin used in their macaques' studies. CsA, however, was increased to achieve a serum trough level >400 ng/ml in their recent pig study (22).
7. Discussion
This review provides a comprehensive overview of the animal models for cardiac remuscularization study. Successful establishment of the model would need to be confirmed using multiple analyses and imaging such as echocardiography, magnetic resonance imaging, cardiac pressure-volume loop analysis etc. The choice of anaesthesia, analgesia and antibiotic regimen post-surgery is key to increasing the survival of the animal subjects carrying the injured hearts. Nevertheless, the method of choice is based on the experimental needs and objectives. Transplantation of allogeneic cells would require effective immunosuppression to avoid host-vs.-graft rejection of the cells. While the best regimen has not been concluded, the selection of the immunosuppressive strategy is generally aimed toward achieving low toxicity-related side effects, highly efficient immunosuppression, and a high rate of engraftment and survival of the transplanted cells.
Nonetheless, ongoing concerns regarding the incidence of arrhythmias post-CM transplantation (herein refers to engraftment arrhythmias), were possible due to the presence of the nodal cells within the transplanted cardiomyocytes (12, 13, 30, 135). This problem has become the primary impediment to advancing the therapy to clinical trials. Intensive research has been ongoing to decipher pathways that direct chamber-specific cardiomyocyte differentiation to eliminate the presence of nodal cells in the culture and increase the population of ventricular cardiomyocytes in the subsequently transplanted graft. Alternatively, using a pharmacological approach to mitigate engraftment arrhythmias could also be a viable option (22).
In 2014, the European Society of Cardiology Cellular Biology of the Heart Working Group issued a position paper to urge for improving the preclinical assessment of novel cardioprotective therapies. In the statement, the experts attributed the low translatability of laboratory findings into clinics to the lack of rigorous tests during the preclinical animal study (136). One of the shortcomings was the preference over using reductionist cell or rodent models than employing a more integrative large mammal I/R model which simulates clinical reality. We summarize the methodology from the most recent cardiac remuscularization studies using large animals to provide an overview of the differences in reporting between laboratories, and their strategies in establishing MI models, cell source and delivery, as well as post-operative care analgesia and immunosuppression regimen. To increase the reproducibility and transparency of any future in vivo work, adherent to the Animal Research: Reporting In Vivo Experiments (ARRIVE) guideline is urged to facilitate the minimum information and standard required to be included in reporting and publishing animal research experiments (137).
Author contributions
YY, SKT, ZG and JJT conceived the review outline. YY, FFR and JJT wrote the manuscript. YKY, BS, ZG and JJT reviewed and revised the manuscript. All authors contributed to the article and approved the submitted version.
Funding
ZG and JJT are funded by Zhengzhou Cardiovascular Hospital, Zhengzhou Seventh People's Hospital and the Key Laboratory of Cardiac Function and Structure Function Project Fund (2019KFK001, 304/CIPPT/6501080/A150). JJT is also a recipient of the Fundamental Research Grant Scheme (FRGS/1/2018/STG05/USM/03/3) and USM Research University Grant (1001/CIPPT/8011102).
Acknowledgments
This is a short text to acknowledge the contributions of specific colleagues, institutions, or agencies that aided the efforts of the authors.
Conflict of interest
JJT received research funding from ALPS Global Holding and CryoCord Sdn Bhd. SKT is the founder and stockholder of Celestialab Sdn. Bhd and ALPS Global Holding Bhd. Other authors declare the absence of any commercial or financial relationships that could be construed as a potential conflict of interest.
Publisher's note
All claims expressed in this article are solely those of the authors and do not necessarily represent those of their affiliated organizations, or those of the publisher, the editors and the reviewers. Any product that may be evaluated in this article, or claim that may be made by its manufacturer, is not guaranteed or endorsed by the publisher.
References
1. Burchfield JS, Xie M, Hill JA. Pathological ventricular remodeling: mechanisms: part 1 of 2. Circulation. (2013) 128(4):388–400. doi: 10.1161/CIRCULATIONAHA.113.001878
2. Junbo G, Yongjian X, Chen W. Internal medicine. 7th ed. Beijing: People's Medical Publishing House (2014). 942 p.
4. Ferreira JP, Kraus S, Mitchell S, Perel P, Pineiro D, Chioncel O, et al. World heart federation roadmap for heart failure. Glob Heart. (2019) 14(3):197–214. doi: 10.1016/j.gheart.2019.07.004
5. Benjamin EJ, Muntner P, Alonso A, Bittencourt MS, Callaway CW, Carson AP, et al. Heart disease and stroke statistics-2019 update: a report from the American heart association. Circulation. (2019) 139(10):e56–e528. doi: 10.1161/CIR.0000000000000659
6. Heidenreich PA, Albert NM, Allen LA, Bluemke DA, Butler J, Fonarow GC, et al. Forecasting the impact of heart failure in the United States: a policy statement from the American heart association. Circ Heart Fail. (2013) 6(3):606–19. doi: 10.1161/HHF.0b013e318291329a
7. Huffman MD, Berry JD, Ning H, Dyer AR, Garside DB, Cai X, et al. Lifetime risk for heart failure among white and black Americans: cardiovascular lifetime risk pooling project. J Am Coll Cardiol. (2013) 61(14):1510–7. doi: 10.1016/j.jacc.2013.01.022
8. Jessup M, Brozena S. Heart Failure. N Engl J Med. (2003) 348(20):2007–18. doi: 10.1056/NEJMra021498
9. Bloch Thomsen PE, Jons C, Raatikainen MJ, Moerch Joergensen R, Hartikainen J, Virtanen V, et al. Long-Term recording of cardiac arrhythmias with an implantable cardiac monitor in patients with reduced ejection fraction after acute myocardial infarction: the cardiac arrhythmias and risk stratification after acute myocardial infarction (carisma) study. Circulation. (2010) 122(13):1258–64. doi: 10.1161/CIRCULATIONAHA.109.902148
10. Leong YY, Ng WH, Ellison-Hughes GM, Tan JJ. Cardiac stem cells for myocardial regeneration: they are not alone. Front Cardiovasc Med. (2017) 4:47. doi: 10.3389/fcvm.2017.00047
11. Majka M, Sulkowski M, Badyra B, Musialek P. Concise review: mesenchymal stem cells in cardiovascular regeneration: emerging research directions and clinical applications. Stem Cells Transl Med. (2017) 6(10):1859–67. doi: 10.1002/sctm.16-0484
12. Liu YW, Chen B, Yang X, Fugate JA, Kalucki FA, Futakuchi-Tsuchida A, et al. Human embryonic stem cell-derived cardiomyocytes restore function in infarcted hearts of non-human primates. Nat Biotechnol. (2018) 36(7):597–605. doi: 10.1038/nbt.4162
13. Romagnuolo R, Masoudpour H, Porta-Sanchez A, Qiang B, Barry J, Laskary A, et al. Human embryonic stem cell-derived cardiomyocytes regenerate the infarcted pig heart but induce ventricular tachyarrhythmias. Stem Cell Rep. (2019) 12(5):967–81. doi: 10.1016/j.stemcr.2019.04.005
14. Caspi O, Huber I, Kehat I, Habib M, Arbel G, Gepstein A, et al. Transplantation of human embryonic stem cell-derived cardiomyocytes improves myocardial performance in infarcted rat hearts. J Am Coll Cardiol. (2007) 50(19):1884–93. doi: 10.1016/j.jacc.2007.07.054
15. Citro L, Naidu S, Hassan F, Kuppusamy ML, Kuppusamy P, Angelos MG, et al. Comparison of human induced pluripotent stem-cell derived cardiomyocytes with human mesenchymal stem cells following acute myocardial infarction. PLoS One. (2014) 9(12):e116281. doi: 10.1371/journal.pone.0116281
16. Pfeffer MA, Pfeffer JM, Fishbein MC, Fletcher PJ, Spadaro J, Kloner RA, et al. Myocardial infarct size and ventricular function in rats. Circ Res. (1979) 44(4):503–12. doi: 10.1161/01.res.44.4.503
17. Pfeffer MA, Braunwald E, Moye LA, Basta L, Brown EJ Jr., Cuddy TE, et al. Effect of captopril on mortality and morbidity in patients with left ventricular dysfunction after myocardial infarction. Results of the survival and ventricular enlargement trial. The save investigators. N Engl J Med. (1992) 327(10):669–77. doi: 10.1056/NEJM199209033271001
18. Zwetsloot PP, Vegh AM, Jansen of Lorkeers SJ, van Hout GP, Currie GL, Sena ES, et al. Cardiac stem cell treatment in myocardial infarction: a systematic review and meta-analysis of preclinical studies. Circ Res. (2016) 118(8):1223–32. doi: 10.1161/CIRCRESAHA.115.307676
19. Pecha S, Yorgan K, Rohl M, Geertz B, Hansen A, Weinberger F, et al. Human ips cell-derived engineered heart tissue does not affect ventricular arrhythmias in a Guinea pig cryo-injury model. Sci Rep. (2019) 9(1):9831. doi: 10.1038/s41598-019-46409-z
20. Chong JJ, Yang X, Don CW, Minami E, Liu YW, Weyers JJ, et al. Human embryonic-stem-cell-derived cardiomyocytes regenerate non-human primate hearts. Nature. (2014) 510(7504):273–7. doi: 10.1038/nature13233
21. Fernandez-Aviles F, Sanz-Ruiz R, Climent AM, Badimon L, Bolli R, Charron D, et al. Global position paper on cardiovascular regenerative medicine. Eur Heart J. (2017) 38(33):2532–46. doi: 10.1093/eurheartj/ehx248
22. Nakamura K, Neidig LE, Yang X, Weber GJ, El-Nachef D, Tsuchida H, et al. Pharmacologic therapy for engraftment arrhythmia induced by transplantation of human cardiomyocytes. Stem Cell Rep. (2021) 16(10):2473–87. doi: 10.1016/j.stemcr.2021.08.005
23. Zhao M, Nakada Y, Wei Y, Bian W, Chu Y, Borovjagin AV, et al. Cyclin D2 overexpression enhances the efficacy of human induced pluripotent stem cell-derived cardiomyocytes for myocardial repair in a swine model of myocardial infarction. Circulation. (2021) 144(3):210–28. doi: 10.1161/CIRCULATIONAHA.120.049497
24. Tan SH, Loo SJ, Gao Y, Tao ZH, Su LP, Wang CX, et al. Thymosin Beta4 increases cardiac cell proliferation, cell engraftment, and the reparative potency of human induced-pluripotent stem cell-derived cardiomyocytes in a porcine model of acute myocardial infarction. Theranostics. (2021) 11(16):7879–95. doi: 10.7150/thno.56757
25. Suzuki K, Miyagawa S, Liu L, Kawamura T, Li J, Qu X, et al. Therapeutic efficacy of large aligned cardiac tissue derived from induced pluripotent stem cell in a porcine ischemic cardiomyopathy model. J Heart Lung Transplant. (2021) 40(8):767–77. doi: 10.1016/j.healun.2021.04.010
26. Kashiyama N, Miyagawa S, Fukushima S, Kawamura T, Kawamura A, Yoshida S, et al. Mhc-Mismatched allotransplantation of induced pluripotent stem cell-derived cardiomyocyte sheets to improve cardiac function in a primate ischemic cardiomyopathy model. Transplantation. (2019) 103(8):1582–90. doi: 10.1097/TP.0000000000002765
27. Ishida M, Miyagawa S, Saito A, Fukushima S, Harada A, Ito E, et al. Transplantation of human-induced pluripotent stem cell-derived cardiomyocytes is superior to somatic stem cell therapy for restoring cardiac function and oxygen consumption in a porcine model of myocardial infarction. Transplantation. (2019) 103(2):291–8. doi: 10.1097/TP.0000000000002384
28. Zhu K, Wu Q, Ni C, Zhang P, Zhong Z, Wu Y, et al. Lack of remuscularization following transplantation of human embryonic stem cell-derived cardiovascular progenitor cells in infarcted nonhuman primates. Circ Res. (2018) 122(7):958–69. doi: 10.1161/CIRCRESAHA.117.311578
29. Laflamme MA, Chen KY, Naumova AV, Muskheli V, Fugate JA, Dupras SK, et al. Cardiomyocytes derived from human embryonic stem cells in pro-survival factors enhance function of infarcted rat hearts. Nat Biotechnol. (2007) 25(9):1015–24. doi: 10.1038/nbt1327
30. Shiba Y, Gomibuchi T, Seto T, Wada Y, Ichimura H, Tanaka Y, et al. Allogeneic transplantation of ips cell-derived cardiomyocytes regenerates primate hearts. Nature. (2016) 538(7625):388–91. doi: 10.1038/nature19815
31. Xiong Q, Hill KL, Li Q, Suntharalingam P, Mansoor A, Wang X, et al. A fibrin patch-based enhanced delivery of human embryonic stem cell-derived vascular cell transplantation in a porcine model of postinfarction left ventricular remodeling. Stem Cells. (2011) 29(2):367–75. doi: 10.1002/stem.580
32. Zeng L, Hu Q, Wang X, Mansoor A, Lee J, Feygin J, et al. Bioenergetic and functional consequences of bone marrow-derived multipotent progenitor cell transplantation in hearts with postinfarction left ventricular remodeling. Circulation. (2007) 115(14):1866–75. doi: 10.1161/CIRCULATIONAHA.106.659730
33. Ye L, Chang YH, Xiong Q, Zhang P, Zhang L, Somasundaram P, et al. Cardiac repair in a porcine model of acute myocardial infarction with human induced pluripotent stem cell-derived cardiovascular cells. Cell Stem Cell. (2014) 15(6):750–61. doi: 10.1016/j.stem.2014.11.009
34. Kawamura M, Miyagawa S, Miki K, Saito A, Fukushima S, Higuchi T, et al. Feasibility, safety, and therapeutic efficacy of human induced pluripotent stem cell-derived cardiomyocyte sheets in a porcine ischemic cardiomyopathy model. Circulation. (2012) 126(11 Suppl 1):S29–37. doi: 10.1161/CIRCULATIONAHA.111.084343
35. Halkos ME, Zhao ZQ, Kerendi F, Wang NP, Jiang R, Schmarkey LS, et al. Intravenous infusion of mesenchymal stem cells enhances regional perfusion and improves ventricular function in a porcine model of myocardial infarction. Basic Res Cardiol. (2008) 103(6):525–36. doi: 10.1007/s00395-008-0741-0
36. Lindsey ML, Bolli R, Canty JM Jr., Du XJ, Frangogiannis NG, Frantz S, et al. Guidelines for experimental models of myocardial ischemia and infarction. Am J Physiol Heart Circ Physiol. (2018) 314(4):H812–H38. doi: 10.1152/ajpheart.00335.2017
37. Vasudevan P, Gaebel R, Doering P, Mueller P, Lemcke H, Stenzel J, et al. 18f-Fdg Pet-Based imaging of myocardial inflammation predicts a functional outcome following transplantation of mesc-derived cardiac induced cells in a mouse model of myocardial infarction. Cells. (2019) 8(12):1613. doi: 10.3390/cells8121613
38. Lim M, Wang W, Liang L, Han ZB, Li Z, Geng J, et al. Intravenous injection of allogeneic umbilical cord-derived multipotent mesenchymal stromal cells reduces the infarct area and ameliorates cardiac function in a porcine model of acute myocardial infarction. Stem Cell Res Ther. (2018) 9(1):129. doi: 10.1186/s13287-018-0888-z
39. de Jong R, van Hout GP, Houtgraaf JH, Kazemi K, Wallrapp C, Lewis A, et al. Intracoronary infusion of encapsulated glucagon-like peptide-1-eluting mesenchymal stem cells preserves left ventricular function in a porcine model of acute myocardial infarction. Circ Cardiovasc Interv. (2014) 7(5):673–83. doi: 10.1161/CIRCINTERVENTIONS.114.001580
40. Shin HS, Shin HH, Shudo Y. Current Status and limitations of myocardial infarction large animal models in cardiovascular translational research. Front Bioeng Biotechnol. (2021) 9:673683. doi: 10.3389/fbioe.2021.673683
41. Lelovas PP, Kostomitsopoulos NG, Xanthos TT. A comparative anatomic and physiologic overview of the porcine heart. J Am Assoc Lab Anim Sci. (2014) 53(5):432–8. PMID: 25255064
42. Buss DD, Hyde DM, Steffey EP. Coronary collateral development in the rhesus monkey (Macaca Mulatta). Basic Res Cardiol. (1983) 78(5):510–7. doi: 10.1007/BF01906462
43. Tohyama S, Kobayashi E. Age-Appropriateness of porcine models used for cell transplantation. Cell Transplant. (2019) 28(2):224–8. doi: 10.1177/0963689718817477
44. Hearse DJ. Species variation in the coronary collateral circulation during regional myocardial ischaemia: a critical determinant of the rate of evolution and extent of myocardial infarction. Cardiovasc Res. (2000) 45(1):213–9. doi: 10.1016/s0008-6363(99)00331-4
45. Miura T, Downey JM. Collateral perfusion of ischemic myocardium is inversely related to the size of the ischemic zone. Basic Res Cardiol. (1988) 83(2):128–36. doi: 10.1007/BF01907266
46. Flameng W, Schwarz F, Schaper W. Coronary collaterals in the canine heart: development and functional significance. Am Heart J. (1979) 97(1):70–7. doi: 10.1016/0002-8703(79)90116-9
47. Litvak J, Siderides LE, Vineberg AM. The experimental production of coronary artery insufficiency and occlusion. Am Heart J. (1957) 53(4):505–18. doi: 10.1016/0002-8703(57)90359-9
48. Herrmann JL. Do ameroid constrictors reliably occlude porcine coronary arteries? J Surg Res. (2010) 161(1):36–7. doi: 10.1016/j.jss.2009.05.047
49. Wang Y, Li C, Chuo W, Liu Z, Ouyang Y, Li D, et al. Integrated proteomic and metabolomic analysis reveals the nadh-mediated tca cycle and energy metabolism disorders based on a new model of chronic progressive heart failure. Mol Biosyst. (2013) 9(12):3135–45. doi: 10.1039/c3mb70263d
50. Keeran KJ, Jeffries KR, Zetts AD, Taylor J, Kozlov S, Hunt TJ. A chronic cardiac ischemia model in swine using an ameroid constrictor. J Vis Exp. (2017) 128:56190. doi: 10.3791/56190
51. Yajima S, Miyagawa S, Fukushima S, Sakai Y, Isohashi K, Watabe T, et al. A prostacyclin agonist and an omental flap increased myocardial blood flow in a porcine chronic ischemia model. J Thorac Cardiovasc Surg. (2018) 156(1):229–41.e14. doi: 10.1016/j.jtcvs.2018.02.086
52. Kloner RA, Przyklenk K, Whittaker P. Deleterious effects of oxygen radicals in ischemia/reperfusion. Resolved and unresolved issues. Circulation. (1989) 80(5):1115–27. doi: 10.1161/01.cir.80.5.1115
53. Kloner RA, Ellis SG, Lange R, Braunwald E. Studies of experimental coronary artery reperfusion. Effects on infarct size, myocardial function, biochemistry, ultrastructure and microvascular damage. Circulation. (1983) 68(2 Pt 2):I8–15. PMID: 6861331
54. Hu X, Ma R, Lu J, Zhang K, Xu W, Jiang H, et al. Il-23 promotes myocardial I/R injury by increasing the inflammatory responses and oxidative stress reactions. Cell Physiol Biochem. (2016) 38(6):2163–72. doi: 10.1159/000445572
55. Xu Z, McElhanon KE, Beck EX, Weisleder N. A murine model of myocardial ischemia-reperfusion injury. Methods Mol Biol. (2018) 1717:145–53. doi: 10.1007/978-1-4939-7526-6_12
56. Silvis MJM, van Hout GPJ, Fiolet ATL, Dekker M, Bosch L, van Nieuwburg MMJ, et al. Experimental parameters and infarct size in closed chest pig lad ischemia reperfusion models; lessons learned. BMC Cardiovasc Disord. (2021) 21(1):171. doi: 10.1186/s12872-021-01995-7
57. Bikou O, Watanabe S, Hajjar RJ, Ishikawa K. A pig model of myocardial infarction: catheter-based approaches. In: Ishikawa K, editor. Experimental models of cardiovascular diseases: Methods and protocols. New York, NY: Springer New York (2018). p. 281–94.
58. Garcia-Dorado D, Theroux P, Elizaga J, Galinanes M, Solares J, Riesgo M, et al. Myocardial reperfusion in the pig heart model: infarct size and duration of coronary occlusion. Cardiovasc Res. (1987) 21(7):537–44. doi: 10.1093/cvr/21.7.537
59. Koudstaal S, Jansen of Lorkeers S, Gho JM, van Hout GP, Jansen MS, Grundeman PF, et al. Myocardial infarction and functional outcome assessment in pigs. J Vis Exp. (2014) 86:51269. doi: 10.3791/51269
60. Abd-Elmoniem KZ, Tomas MS, Sasano T, Soleimanifard S, Vonken EJ, Youssef A, et al. Assessment of distribution and evolution of mechanical dyssynchrony in a porcine model of myocardial infarction by cardiovascular magnetic resonance. J Cardiovasc Magn Reson. (2012) 14(1):1. doi: 10.1186/1532-429X-14-1
61. Niemann JT, Rosborough JP, Youngquist ST, Shah AP. Transthoracic defibrillation potential gradients in a closed chest porcine model of prolonged spontaneous and electrically induced ventricular fibrillation. Resuscitation. (2010) 81(4):477–80. doi: 10.1016/j.resuscitation.2009.12.027
62. Sattler SM, Lubberding AF, Skibsbye L, Jabbari R, Wakili R, Jespersen T, et al. Amiodarone treatment in the early phase of acute myocardial infarction protects against ventricular fibrillation in a porcine model. J Cardiovasc Transl Res. (2019) 12(4):321–30. doi: 10.1007/s12265-018-9861-6
63. Gianelly R, von der Groeben JO, Spivack AP, Harrison DC. Effect of lidocaine on ventricular arrhythmias in patients with coronary heart disease. N Engl J Med. (1967) 277(23):1215–9. doi: 10.1056/NEJM196712072772301
64. Lee EH, Lee HM, Chung CH, Chin JH, Choi DK, Chung HJ, et al. Impact of intravenous lidocaine on myocardial injury after off-pump coronary artery surgery. Br J Anaesth. (2011) 106(4):487–93. doi: 10.1093/bja/aeq416
65. Li T, Wei X, Watkins AC, Sanchez PG, Wu ZJ, Griffith BP. Prophylactic amiodarone and lidocaine improve survival in an ovine model of large size myocardial infarction. J Surg Res. (2013) 185(1):152–8. doi: 10.1016/j.jss.2013.05.050
66. Hirsh J, Anand SS, Halperin JL, Fuster V, American Heart A. Guide to anticoagulant therapy: heparin: a statement for healthcare professionals from the American heart association. Circulation. (2001) 103(24):2994–3018. doi: 10.1161/01.cir.103.24.2994
67. Pinto DS, Lorenz DP, Murphy SA, Marble SJ, DiBattiste PM, Demopoulos LA, et al. Association of an activated clotting time < or =250 seconds with adverse event rates after percutaneous coronary intervention using tirofiban and heparin (a tactics-timi 18 substudy). Am J Cardiol. (2003) 91(8):976–8. A4. doi: 10.1016/s0002-9149(03)00117-6
68. Niccoli G, Banning AP. Heparin dose during percutaneous coronary intervention: how low dare we go? Heart. (2002) 88(4):331–4. doi: 10.1136/heart.88.4.331
69. Cronin EM, Bogun FM, Maury P, Peichl P, Chen M, Namboodiri N, et al. 2019 Hrs/ehra/aphrs/lahrs expert consensus statement on catheter ablation of ventricular arrhythmias. Europace. (2019) 21(8):1143–4. doi: 10.1093/europace/euz132
70. Narins CR, Hillegass WB, Nelson CL, Tcheng JE, Harrington RA, Phillips HR, et al. Relation between activated clotting time during angioplasty and abrupt closure. Circulation. (1996) 93(4):667–71. doi: 10.1161/01.cir.93.4.667
71. Lincoff AM, Popma JJ, Ellis SG, Hacker JA, Topol EJ. Abrupt vessel closure complicating coronary angioplasty: clinical, angiographic and therapeutic profile. J Am Coll Cardiol. (1992) 19(5):926–35. doi: 10.1016/0735-1097(92)90272-o
72. Solanes N, Bobi J, Arrieta M, Jimenez FR, Palacios C, Rodríguez JJ, et al. An open secret in porcine acute myocardial infarction models: the relevance of anaesthetic regime and breed in ischaemic outcomes. Front Vet Sci. (2022) 9:919454. doi: 10.3389/fvets.2022.919454
73. Cobo AA, Margallo FMS, Díaz CB, Blázquez VB, Bueno IG, Crisóstomo V. Anesthesia protocols used to create ischemia reperfusion myocardial infarcts in swine. J Am Assoc Lab Anim Sci. (2020) 59(5):478–87. doi: 10.30802/aalas-jaalas-19-000137
74. Domino EF, Chodoff P, Corssen G. Pharmacologic effects of ci-581, a new dissociative anesthetic, in man. Clin Pharmacol Ther. (1965) 6:279–91. doi: 10.1002/cpt196563279
75. Brambrink AM, Evers AS, Avidan MS, Farber NB, Smith DJ, Martin LD, et al. Ketamine-Induced neuroapoptosis in the fetal and neonatal rhesus macaque brain. Anesthesiology. (2012) 116(2):372–84. doi: 10.1097/ALN.0b013e318242b2cd
76. Davy CW, Trennery PN, Edmunds JG, Altman JF, Eichler DA. Local myotoxicity of ketamine hydrochloride in the marmoset. Lab Anim. (1987) 21(1):60–7. doi: 10.1258/002367787780740725
77. Page RL 2nd, O'Bryant CL, Cheng D, Dow TJ, Ky B, Stein CM, et al. Drugs that may cause or exacerbate heart failure: a scientific statement from the American heart association. Circulation. (2016) 134(6):e32–69. doi: 10.1161/CIR.0000000000000426
78. Bertrand HG, Ellen YC, O'Keefe S, Flecknell PA. Comparison of the effects of ketamine and fentanyl-midazolam-medetomidine for sedation of rhesus macaques (Macaca Mulatta). BMC Vet Res. (2016) 12(1):93. doi: 10.1186/s12917-016-0721-9
79. Mion G, Villevieille T. Ketamine pharmacology: an update (pharmacodynamics and molecular aspects. Recent Findings). CNS Neurosci Ther. (2013) 19(6):370–80. doi: 10.1111/cns.12099
80. Haskins SC, Klide AM. Precautions when using ketamine for induction of anesthesia. Vet Clin North Am Small Anim Pract. (1992) 22(2):268–9. doi: 10.1016/s0195-5616(92)50605-2
81. Dal T, Sazak H, Tunc M, Sahin S, Yilmaz A. A comparison of ketamine-midazolam and ketamine-propofol combinations used for sedation in the endobronchial ultrasound-guided transbronchial needle aspiration: a prospective, single-blind, randomized study. J Thorac Dis. (2014) 6(6):742–51. doi: 10.3978/j.issn.2072-1439.2014.04.10
82. Kye YC, Rhee JE, Kim K, Kim T, Jo YH, Jeong JH, et al. Clinical effects of adjunctive atropine during ketamine sedation in pediatric emergency patients. Am J Emerg Med. (2012) 30(9):1981–5. doi: 10.1016/j.ajem.2012.04.030
83. Havton LA, Biscola NP, Christe KL, Colman RJ. Ketamine-Induced neuromuscular reactivity is associated with aging in female rhesus macaques. PLoS One. (2020) 15(9):e0236430. doi: 10.1371/journal.pone.0236430
84. Dhote F, Carpentier P, Barbier L, Peinnequin A, Baille V, Pernot F, et al. Combinations of ketamine and atropine are neuroprotective and reduce neuroinflammation after a toxic Status epilepticus in mice. Toxicol Appl Pharmacol. (2012) 259(2):195–209. doi: 10.1016/j.taap.2011.12.024
85. Shi J, Li A, Wei Z, Liu Y, Xing C, Shi H, et al. Ketamine versus ketamine pluses atropine for pediatric sedation: a meta-analysis. Am J Emerg Med. (2018) 36(7):1280–6. doi: 10.1016/j.ajem.2018.04.010
86. Tajoddini S, Motaghi M. Sedative and analgesic effects of propofol–ketamine versus propofol–fentanyl for emergency department procedures. Hong Kong J Emerg Med. (2020) 29(4):212–9. doi: 10.1177/1024907919893466
87. Aarnes TK, Muir WW. Pain assessment and management. In: Peterson ME, Kutzler MA, editors. Small animal pediatrics. Saint louis: W.B. Saunders (2011). p. 220–32.
88. Vogler GA. Anesthesia and analgesia. In: Suckow MA, Weisbroth SH, Franklin CL, editors. The laboratory rat. Burlington: Academic Press (2006). p. 627–64.
89. Priebe HJ, editor. Isoflurane: effects on coronary circulation and clinical implications. In: Reinhart K, Eyrich K, editors. Clinical aspects of O2 transport and tissue oxygenation; (1989); Berlin, Heidelberg: Springer Berlin Heidelberg. Available at: https://doi.org/10.1007/978-3-642-83872-9_29
90. Cheng EY, Kay J, Hoka S, Bosnjak ZJ, Coon RL, Kampine JP. The influence of isoflurane on the vascular reflex response to lung inflation in dogs. Anesthesiology. (1992) 76(6):972–8. doi: 10.1097/00000542-199206000-00017
91. Raphael J, Rivo J, Gozal Y. Isoflurane-Induced myocardial preconditioning is dependent on phosphatidylinositol-3-kinase/akt signalling. Br J Anaesth. (2005) 95(6):756–63. doi: 10.1093/bja/aei264
92. Regueiro-Purrinos M, Fernandez-Vazquez F, de Prado AP, Altonaga JR, Cuellas-Ramon C, Ajenjo-Silverio JM, et al. Ventricular arrhythmias and mortality associated with isoflurane and sevoflurane in a porcine model of myocardial infarction. J Am Assoc Lab Anim Sci. (2011) 50(1):73–8. PMID: 21333167
93. Grisneaux E, Pibarot P, Dupuis J, Blais D. Comparison of ketoprofen and carprofen administered prior to orthopedic surgery for control of postoperative pain in dogs. J Am Vet Med Assoc. (1999) 215(8):1105–10. PMID: 10530322
94. Krantz MJ, Palmer RB, Haigney MCP. Cardiovascular complications of opioid use: jacc state-of-the-art review. J Am Coll Cardiol. (2021) 77(2):205–23. doi: 10.1016/j.jacc.2020.11.002
95. Freye E. Cardiovascular effects of high dosages of fentanyl, meperdine, and naloxone in dogs. Anesth Analg. (1974) 53(1):40–7. doi: 10.1213/00000539-197401000-00010
96. Dahan A, Yassen A, Bijl H, Romberg R, Sarton E, Teppema L, et al. Comparison of the respiratory effects of intravenous buprenorphine and fentanyl in humans and rats. Br J Anaesth. (2005) 94(6):825–34. doi: 10.1093/bja/aei145
97. Shientag LJ, Wheeler SM, Garlick DS, Maranda LS. A therapeutic dose of ketoprofen causes acute gastrointestinal bleeding, erosions, and ulcers in rats. J Am Assoc Lab Anim Sci. (2012) 51(6):832–41. PMID: 23294892
98. Fabian NJ, Moody DE, Averin O, Fang WB, Jamiel M, Fox JG, et al. Pharmacokinetics of single-dose intramuscular and subcutaneous injections of buprenorphine in common marmosets (Callithrix jacchus). J Am Assoc Lab Anim Sci. (2021) 60(5):568–75. doi: 10.30802/AALAS-JAALAS-20-000151
99. Lyra-Leite DM, Gutierrez-Gutierrez O, Wang M, Zhou Y, Cyganek L, Burridge PW. A review of protocols for human ipsc culture, cardiac differentiation, subtype-specification, maturation, and direct reprogramming. STAR Protoc. (2022) 3(3):101560. doi: 10.1016/j.xpro.2022.101560
100. Kobayashi H, Tohyama S, Kanazawa H, Ichimura H, Chino S, Tanaka Y, et al. Intracoronary transplantation of pluripotent stem cell-derived cardiomyocytes: inefficient procedure for cardiac regeneration. J Mol Cell Cardiol. (2022) 174:77–87. doi: 10.1016/j.yjmcc.2022.11.004
101. Teng CJ, Luo J, Chiu RCJ, Shum-Tim D. Massive mechanical loss of microspheres with direct intramyocardial injection in the beating heart: implications for cellular cardiomyoplasty. J Thorac Cardiovasc Surg. (2006) 132(3):628–32. doi: 10.1016/j.jtcvs.2006.05.034
102. van den Akker F, Feyen DAM, van den Hoogen P, van Laake LW, van Eeuwijk ECM, Hoefer I, et al. Intramyocardial stem cell injection: go(ne) with the flow. Eur Heart J. (2016) 38(3):184–6. doi: 10.1093/eurheartj/ehw056
103. Querdel E, Reinsch M, Castro L, Kose D, Bahr A, Reich S, et al. Human engineered heart tissue patches remuscularize the injured heart in a dose-dependent manner. Circulation. (2021) 143(20):1991–2006. doi: 10.1161/CIRCULATIONAHA.120.047904
104. Bolli R, Wysoczynski M. Human embryonic stem cell-derived cardiomyocytes. Circ Res. (2019) 124(8):1157–9. doi: 10.1161/CIRCRESAHA.119.314869
105. Demkes EJ, Rijken S, Szymanski MK, Hoefer IE, Sluijter JPG, de Jager SCA. Requirements for proper immunosuppressive regimens to limit translational failure of cardiac cell therapy in preclinical large animal models. J Cardiovasc Transl Res. (2021) 14(1):88–99. doi: 10.1007/s12265-020-10035-2
106. Di Nicola M, Carlo-Stella C, Magni M, Milanesi M, Longoni PD, Matteucci P, et al. Human bone marrow stromal cells suppress T-lymphocyte proliferation induced by cellular or nonspecific mitogenic stimuli. Blood. (2002) 99(10):3838–43. doi: 10.1182/blood.v99.10.3838
107. William TT, Pendleton JD, Beyer WM, Egalka MC, Guinan EC. Suppression of allogeneic T-cell proliferation by human marrow stromal cells: implications in transplantation. Transplantation. (2003) 75(3):389–97. doi: 10.1097/01.TP.0000045055.63901.A9
108. Huang XP, Sun Z, Miyagi Y, McDonald Kinkaid H, Zhang L, Weisel RD, et al. Differentiation of allogeneic mesenchymal stem cells induces immunogenicity and limits their long-term benefits for myocardial repair. Circulation. (2010) 122(23):2419–29. doi: 10.1161/CIRCULATIONAHA.110.955971
109. Guha P, Morgan JW, Mostoslavsky G, Rodrigues NP, Boyd AS. Lack of immune response to differentiated cells derived from syngeneic induced pluripotent stem cells. Cell Stem Cell. (2013) 12(4):407–12. doi: 10.1016/j.stem.2013.01.006
110. Takahashi K, Tanabe K, Ohnuki M, Narita M, Ichisaka T, Tomoda K, et al. Induction of pluripotent stem cells from adult human fibroblasts by defined factors. Cell. (2007) 131(5):861–72. doi: 10.1016/j.cell.2007.11.019
111. Taylor CJ, Bolton EM, Pocock S, Sharples LD, Pedersen RA, Bradley JA. Banking on human embryonic stem cells: estimating the number of donor cell lines needed for hla matching. Lancet. (2005) 366(9502):2019–25. doi: 10.1016/S0140-6736(05)67813-0
112. Sugita S, Iwasaki Y, Makabe K, Kimura T, Futagami T, Suegami S, et al. Lack of T cell response to ipsc-derived retinal pigment epithelial cells from hla homozygous donors. Stem Cell Rep. (2016) 7(4):619–34. doi: 10.1016/j.stemcr.2016.08.011
113. Yoshida S, Kato TM, Sato Y, Umekage M, Ichisaka T, Tsukahara M, et al. A clinical-grade hla haplobank of human induced pluripotent stem cells matching approximately 40% of the Japanese population. Med (N Y). (2022) 4(1):51–66. doi: 10.1016/j.medj.2022.10.003
114. Kawamura T, Miyagawa S, Fukushima S, Maeda A, Kashiyama N, Kawamura A, et al. Cardiomyocytes derived from mhc-homozygous induced pluripotent stem cells exhibit reduced allogeneic immunogenicity in mhc-matched non-human primates. Stem Cell Rep. (2016) 6(3):312–20. doi: 10.1016/j.stemcr.2016.01.012
115. Deuse T, Hu X, Gravina A, Wang D, Tediashvili G, De C, et al. Hypoimmunogenic derivatives of induced pluripotent stem cells evade immune rejection in fully immunocompetent allogeneic recipients. Nat Biotechnol. (2019) 37(3):252–8. doi: 10.1038/s41587-019-0016-3
116. Han X, Wang M, Duan S, Franco PJ, Kenty JH, Hedrick P, et al. Generation of hypoimmunogenic human pluripotent stem cells. Proc Natl Acad Sci U S A. (2019) 116(21):10441–6. doi: 10.1073/pnas.1902566116
117. Barbarino JM, Staatz CE, Venkataramanan R, Klein TE, Altman RB. Pharmgkb summary: cyclosporine and tacrolimus pathways. Pharmacogenet Genomics. (2013) 23(10):563–85. doi: 10.1097/FPC.0b013e328364db84
118. Maccario R, Moretta A, Cometa A, Montagna D, Comoli P, Locatelli F, et al. Human mesenchymal stem cells and cyclosporin a exert a synergistic suppressive effect on in vitro activation of alloantigen-specific cytotoxic lymphocytes. Biol Blood Marrow Transplant. (2005) 11(12):1031–2. doi: 10.1016/j.bbmt.2005.08.039
119. Buron F, Perrin H, Malcus C, Hequet O, Thaunat O, Kholopp-Sarda MN, et al. Human mesenchymal stem cells and immunosuppressive drug interactions in allogeneic responses: an in vitro study using human cells. Transplant Proc. (2009) 41(8):3347–52. doi: 10.1016/j.transproceed.2009.08.030
120. Jansen Of Lorkeers SJ, Hart E, Tang XL, Chamuleau ME, Doevendans PA, Bolli R, et al. Cyclosporin in cell therapy for cardiac regeneration. J Cardiovasc Transl Res. (2014) 7(5):475–82. doi: 10.1007/s12265-014-9570-8
121. Nivethitha K, Ramesh A, Talwar A, Shenoy N. Rare phenomena of tacrolimus-induced gingival hyperplasia. J Oral Maxillofac Pathol. (2020) 24(2):403. doi: 10.4103/jomfp.JOMFP_50_20
122. Rhen T, Cidlowski JA. Antiinflammatory action of glucocorticoids–new mechanisms for old drugs. N Engl J Med. (2005) 353(16):1711–23. doi: 10.1056/NEJMra050541
123. Oray M, Abu Samra K, Ebrahimiadib N, Meese H, Foster CS. Long-Term Side effects of glucocorticoids. Expert Opin Drug Saf. (2016) 15(4):457–65. doi: 10.1517/14740338.2016.1140743
124. Najafian N, Sayegh MH. Ctla4-Ig: a novel immunosuppressive agent. Expert Opin Investig Drugs. (2000) 9(9):2147–57. doi: 10.1517/13543784.9.9.2147
125. Kirk AD, Harlan DM, Armstrong NN, Davis TA, Dong Y, Gray GS, et al. Ctla4-Ig and anti-Cd40 ligand prevent renal allograft rejection in primates. Proc Natl Acad Sci U S A. (1997) 94(16):8789–94. doi: 10.1073/pnas.94.16.8789
126. Cabrian KM, Berry KK, Shuford WW, Mittler RS, Rodgers JN, Linsley PS. Suppression of T-cell-dependent immune responses in monkeys by Ctla4ig. Transplant Proc. (1996) 28(6):3261–2. PMID: 8962265
127. McKeage K, McCormack PL. Basiliximab: a review of its use as induction therapy in renal transplantation. BioDrugs. (2010) 24(1):55–76. doi: 10.2165/11203990-000000000-00000
128. Allison AC, Eugui EM. Mycophenolate mofetil and its mechanisms of action. Immunopharmacology. (2000) 47(2-3):85–118. doi: 10.1016/s0162-3109(00)00188-0
129. Raisanen-Sokolowski A, Vuoristo P, Myllarniemi M, Yilmaz S, Kallio E, Hayry P. Mycophenolate mofetil (mmf, rs-61443) inhibits inflammation and smooth muscle cell proliferation in rat aortic allografts. Transpl Immunol. (1995) 3(4):342–51. doi: 10.1016/0966-3274(95)80021-2
130. Badid C, Vincent M, McGregor B, Melin M, Hadj-Aissa A, Veysseyre C, et al. Mycophenolate mofetil reduces myofibroblast infiltration and collagen iii deposition in rat remnant kidney. Kidney Int. (2000) 58(1):51–61. doi: 10.1046/j.1523-1755.2000.00140.x
131. Huang Y, Liu Z, Huang H, Liu H, Li L. Effects of mycophenolic acid on endothelial cells. Int Immunopharmacol. (2005) 5(6):1029–39. doi: 10.1016/j.intimp.2005.01.015
132. Schwarze ML, Houser SL, Muniappan A, Allan JS, Menard MT, McMorrow I, et al. Effects of mycophenolate mofetil on cardiac allograft survival and cardiac allograft vasculopathy in miniature swine. Ann Thorac Surg. (2005) 80(5):1787–93. doi: 10.1016/j.athoracsur.2005.04.054
133. Malekinejad H, Cheraghi H, Alizadeh A, Khadem-Ansari MH, Tehrani AA, Varasteh S. Nitric oxide and acute phase proteins are involved in pathogenesis of mycophenolate mofetil-induced gastrointestinal disorders in rats. Transplant Proc. (2011) 43(7):2741–6. doi: 10.1016/j.transproceed.2011.04.016
134. Galiwango PJ, Delgado DH, Yan R, Kozuszko S, Smith R, Rao V, et al. Mycophenolate mofetil dose reduction for gastrointestinal intolerance is associated with increased rates of rejection in heart transplant patients. J Heart Lung Transplant. (2008) 27(1):72–7. doi: 10.1016/j.healun.2007.10.012
135. Anderson ME, Goldhaber J, Houser SR, Puceat M, Sussman MA. Embryonic stem cell-derived cardiac myocytes are not ready for human trials. Circ Res. (2014) 115(3):335–8. doi: 10.1161/CIRCRESAHA.114.304616
136. Lecour S, Botker HE, Condorelli G, Davidson SM, Garcia-Dorado D, Engel FB, et al. Esc working group cellular biology of the heart: position paper: improving the preclinical assessment of novel cardioprotective therapies. Cardiovasc Res. (2014) 104(3):399–411. doi: 10.1093/cvr/cvu225
Keywords: cardiac regeneration, large animal models, pluripotent stem cells (PSC), cardiomyocytes, cardiac remuscularization, myocardial infarction
Citation: Yu Y, Tham SK, Roslan FF, Shaharuddin B, Yong YK, Guo Z and Tan JJ (2023) Large animal models for cardiac remuscularization studies: A methodological review. Front. Cardiovasc. Med. 10:1011880. doi: 10.3389/fcvm.2023.1011880
Received: 4 August 2022; Accepted: 20 February 2023;
Published: 15 March 2023.
Edited by:
Ngan F. Huang, Stanford University, United StatesReviewed by:
Veronica Crisostomo, Jesús Usón Minimally Invasive Surgery Center, SpainThomas E. Sharp III, Louisiana State University, United States
© 2023 Yu, Tham, Roslan, Shaharuddin, Yong, Guo and Tan. This is an open-access article distributed under the terms of the Creative Commons Attribution License (CC BY). The use, distribution or reproduction in other forums is permitted, provided the original author(s) and the copyright owner(s) are credited and that the original publication in this journal is cited, in accordance with accepted academic practice. No use, distribution or reproduction is permitted which does not comply with these terms.
*Correspondence: Jun Jie Tan amp0YW5AdXNtLm15 Zhikun Guo Z3prQHh4bXUuZWR1LmNu
Specialty Section: This article was submitted to Cardiovascular Biologics and Regenerative Medicine, a section of the journal Frontiers in Cardiovascular Medicine