- 1College of Life Sciences and Bioengineering, School of Physical Science and Engineering, Beijing Jiaotong University, Beijing, China
- 2Department of Nephrology, China-Japan Friendship Hospital, Beijing, China
Cardiovascular disease remains one of the leading causes of death in China, with increasingly serious negative effects on people and society. Despite significant advances in preventing and treating cardiovascular diseases, such as atrial fibrillation/flutter and heart failure over the last few years, much more remains to be done. Therefore, developing innovative methods for identifying and managing cardiovascular disorders is critical. Nanomaterials provide multiple benefits in biomedicine, primarily better catalytic activity, drug loading, targeting, and imaging. Biomimetic materials and nanoparticles are specially combined to synthesize biomimetic nanoparticles that successfully reduce the nanoparticles’ toxicity and immunogenicity while enhancing histocompatibility. Additionally, the biological targeting capability of nanoparticles facilitates the diagnosis and therapy of cardiovascular disease. Nowadays, nanomedicine still faces numerous challenges, which necessitates creating nanoparticles that are highly selective, toxic-free, and better clinically applicable. This study reviews the scientific accomplishments in this field over the past few years covering the classification, applications, and prospects of noble metal biomimetic nanozymes and biomimetic nanocarriers.
1. Introduction
Nanomaterials refer to materials with a single unit size of between 1 and 1,000 nanometers, having been widely studied and applied in many fields (1). Nanomaterials exhibit the major advantages of better drug loading, targeting, and imaging in biomedicine. However, disadvantages such as partial cytotoxicity and immunogenicity displayed by various nanomaterials have wider clinical applications (2, 3).
The term “nanomedicine” introduced by Drexler et al. has been used to describe any nanomaterial for diagnostic or therapeutic applications. Nanoparticles, the most common form of nanomedicines, are classified according to their composition into lipid nanoparticles, protein nanoparticles, inorganic/metallic nanoparticles, and drug nanocrystals. Based on their basic properties, nanoparticles form a class of materials with unique physical and chemical properties finding a wide range of applications (4).
Biomimetic materials and nanomaterials are the two components that make up biomimetic nanomaterials. Biomimetic nanoparticles with specific biological features are synthesized by carefully combining nanoparticles with biomimetic materials (such as cell membranes, lipoproteins, viruses, etc.). This way, the original nanoparticles’ toxicity and immunogenicity are successfully decreased. However, the nanoparticles should also have better histocompatibility and biological targeting, thus allowing for improved disease detection and treatment (5–7).
Inflammation, the most frequent pathogenic process in the human body, is the immune system’s protective response to damage-induced agents in body tissue (8). Inflammation-associated disorders are categorized into acute and chronic inflammatory types, where trauma, infection, and acute myocardial infarction are examples of the former. In contrast, atherosclerosis, arthritis, and tumor immunity belong to the latter (9, 10).
Despite significant advances in the prevention and treatment of cardiovascular disease over the last few years, much more remains to be done. Therefore, it is critical to developing innovative methods for identifying and managing cardiovascular disorders (11, 12). Biomimetic nanoparticles are a class of potential drugs for treating cardiovascular disease. Nanomedicine-based drug delivery systems should possess superior physical and chemical properties, including tiny size, good selectivity, and high tissue permeability. Consequently, biomimetic nanomaterials have increasingly gained popularity among researchers in recent years (13). This review focuses on the latest developments of biomimetic nanomaterials and how they can be applied to the diagnosis and treatment of cardiovascular disease, particularly the repair of heart damage caused by AMI.
2. Noble metal biomimetic nanozymes
Nanozymes, a recently emerging synthetic enzyme that combines the properties of nanomaterials and enzymes, represent a promising alternative to naturally occurring enzymes. Noble metal-based nanozymes, a crucial part of nanozyme materials, have attracted great attention owing to their various advantages.
2.1. Development of noble metal-based nanozymes
“Nanozymes” are nanomaterials with enzymatic catalytic activity, which display efficient enzyme-like catalytic activity and the characteristics of nanomaterials. In addition, nanozymes have incomparable advantages over natural enzymes, such as low-cost preparation, high stability, and mass production. Nanomaterials have long been considered a class of chemically inert substances with no biological effects. Gao et al. (14) researchers discovered that magnetite (Fe3O4) nanoparticles demonstrate horseradish peroxidase (HRP)-like activity. Since then, many laboratories worldwide have also successfully reported on some nanomaterials with the properties of enzymes (15–17).
Nanozymes, a class of nanomaterials with enzymatic catalytic properties, open more prospects for treating cardiovascular diseases (18). In the past few years, the rapid advances in nanotechnology, bioengineering, enzymatic, and structure have been encouraging researchers to simulate new enzymatic activities and modulate nanoenzyme activities by preparing high-performance nanomaterials (19). Significant progress has also been achieved in elucidating catalytic mechanisms and exploring potential application areas. So far, more than 200 laboratories worldwide have explored nanozymes, and the research of nanozymes has been expanded into many fields, gradually spawning a new field of study (20). Compared with natural enzymes, the various advantages of nanozymes potentiate a wider range of applications. As an essential part of nanoenzyme materials, noble metal-based nanozymes have attracted much attention due to their high biocompatibility, simple synthesis, and easy modification (21). They have been studied and applied in multiple fields for bioactive molecular detection, disease diagnosis, treatment, antibacterial, etc (22).
Noble metal-based nanoparticles mainly include gold (Au), silver (Ag), platinum group metals—platinum (Pt), palladium (Pd), ruthenium (Ru), iridium (Ir), rhodium (Rh), osmium (Os), eight rare metal elements, etc (23). A noble metal is a vital catalyst material with superb optical, electrical, thermal, magnetic, and catalytic properties of the surface. It has favorable adsorption of reactants at moderate strength, contributing to the formation of intermediate “active compounds” (24). The most common uses for noble metals include medical treatment, biosensing, and catalysis (25, 26) (Figure 1).
2.2. Classification of noble metal-based nanozymes
Generally, enzymes can be classified according to their mechanisms: oxidoreductases, transferases, hydrolases, lyases, isomerases, ligases, and translocases. Most of the reported nanozymes can mimic the activities of oxidoreductases such as peroxidase (POD), oxidase (OXD), catalase (CAT), and superoxide dismutase (SOD); some nanozymes show a catalytic ability similar to hydrolases or the others.
Noble metals (such as Au, Ag, Pd, and Pt) exhibit varying enzyme-like activities under different conditions (27), with noble metal materials utilizing the uncoordinated atoms on the surface as the enzymatic reaction center. Under different conditions, noble metals can exhibit various enzymatic activities similar to OXD, POD, CAT, and SOD (28).
A small reserve of noble metals on the earth limits their applications as essential catalyst materials. To improve the utilization rate of noble metals and reduce their costs, the syntheses of non-noble metals with noble metals and non-noble metals with non-noble metals have been developed using doping methods (29, 30). Compared with single metals, bimetallic nanomaterials possess unique electronic structures and more favorable properties due to the synergistic effect of the two metals. The application of nanoalloys can reduce the usage of noble metals and enhance the catalytic activity of created nanomaterials (31). In recent years, the design and synthesis of bimetallic materials based on the elemental composition, microstructure, and other factors have been instrumental in substituting for noble metals and enhancing catalytic activity and selectivity (32).
The preparation of ultra-small nanozymes with simple structures and multi-enzyme-mimicking properties holds great potential for their applications in ROS scavenging (33–35). Meanwhile, the inherent ultra-small property of noble metal-based nanozymes is greatly helpful to alleviate the organism’s toxicity (36).
2.2.1. Peroxidase mimetic activity
Peroxidases (PODs) typically catalyze substrates to oxidate when H2O2 or organic peroxides are consumed. Most natural peroxidases are heme proteins that activate H2O2 and generate high-valent intermediate species capable of extracting electrons from different substrates (37). The abundance of iron in heme proteins has contributed to the finding of many POD-mimicking iron-based nanomaterials (38, 39).
Innovative work by Gao et al. (14) showed that Fe3O4 nanoparticles intrinsically catalyze classical POD substrates, including 3,3,5,5-tetramethylbenzidine (TMB), Diaminobenzidine (DAB), and o-phenylenediamine (OPD) (40). Similar to these nanoparticles, other nanomaterials containing iron can also be used as POD mimetics. In addition, nanomaterials containing other transition metals, including Au (41), Ag (42), Pt (43), Pd (44), Ir (45), and Ru (46), can also serve as PODs (47).
Nanozyme studies on gold nanostructures or their mixtures with other metals are popular as they are easy to synthesize and modify on the surface, highly stable, and excellent in peroxidase activity (48). Many studies followed the first report of peroxidase activity in gold nanoparticles in 2004 to evaluate the peroxidase activity when the morphology was changed and they were hybridized with different metals (49).
2.2.2. Oxidase mimetic activity
OXD usually participates in redox reactions at the expense of the molecular oxygen (O2), which is reduced to H2O2 or H2O. Comotti et al. reported that small-sized gold nanoparticles (AuNPs) could promote the glucose to converse into the gluconate, which behaves similarly, to glucose oxidase (GOx) (50). Since then, ultra-small nanomaterials based on noble metals, such as Au (51, 52), Ag (53), Pt (54), and Ir (55) have been demonstrated to be capable of OXD simulation. Gold nanoparticles (AuNPs) are effective nanomaterial-based enzyme mimics (nanozymes) for enzymatic reactions under mild conditions. AuNPs are one of the most widely studied nanoparticles and are highly inert nanomaterials (56). Recently, they presented surprisingly enzyme-mimicking properties under different surface modifications. Since the catalytic activity of NPs depends on their shape, size, and surface modification, the correlation between the structure and the performance contributes to the design of more efficient catalytic systems (57).
2.2.3. Catalase mimetic activity
Catalase is a typical biocatalyst in almost all living systems that encourages H2O2 to break down into H2O and O2. Up to now, several noble metal nanoparticles have been demonstrated to have catalytic activity (58). Fan et al. (59) successfully synthesized highly stable platinum nanoparticles (Pt-Ft) sized between 1 and 2 nm using apoFt as the nucleation substrate. Pt-Ft was demonstrated to exhibit both catalytic and peroxidase activities by directly measuring the product of its catalytic reactions. With hydrogen peroxide as a substrate, oxygen bubbles were observed after the decomposition of hydrogen peroxide by Pt-Ft. Positively charged gold nanoparticles that possess intrinsic peroxidase-like activity can catalyze the oxidation of the peroxidase substrate 3,3,5,5-tetramethylbenzidine (TMB) by H2O2 to produce a blue color in aqueous solution, thus working as a simple method for the colorimetric detection of H2O2 and glucose (60).
2.2.4. Superoxide dismutase mimetic activity
The superoxide radical (O2–) breakdown into O2 and H2O2 is catalyzed by SOD, which serves as a key antioxidant for the organism to defend against oxidative stress. Superoxide dismutase (SOD) is a crucial and naturally occurring enzyme for cells to defend against oxidation. It can catalyze the superoxide to break down, producing oxygen and hydrogen oxide. SOD-like activities can be seen in variable degrees in nanomaterials made of gold (61), platinum (62), palladium (63), ruthenium (64), and other noble metals. Wu et al. found that palladium nanoparticles could scavenge superoxide and display SOD-like activity, making them potential antioxidant defense in biological systems (65). Pt NPs with SOD-like activity was discovered by Miyamoto et al. and employed in antioxidant therapy in Caenorhabditis. elegans. The findings demonstrated that Pt NPs might prolong the life of C. elegans as they scavenged paraquat-induced H2O2 and superoxide anion O2 (66). The excellent SOD-like enzyme activity of Noble metal-based nanozymes has great potential in the application of antioxidant therapy.
2.2.5. Ultra-small noble metal-based nanozymes
The size and morphology of nanozymes play a crucial role in determining enzyme-like activity because they are closely related to the specific surface area of nanozymes. Exposing more active sites due to their higher surface-area-to-volume ratio, smaller-sized nanomaterials have been shown to have better catalytic activity by numerous studies. Noble metal nanomaterials have such potential properties as easy synthesis, ultra-small size, easy surface modification, and specific physicochemical properties (67). Smaller nanozymes, however, can occasionally lose their enzymatic activity. In contrast to 2.9 nm Pt covered by cytosine-rich oligonucleotides, 1.8 nm Pt nanozymes covered by guanine-rich oligonucleotides had weaker peroxidase-like activity. The cause is that 2.9 nm Pt contains more metallic Pt0 than 1.8 nm Pt (68). In conclusion, the catalytic activity of ultra-small noble metal-based nanozymes is related to the surface area and metal valence.
2.2.6. Bimetallic biomimetic nanozymes
The activity of peroxidase nanozymes based on AgM bimetallic alloys can be fine-tuned by gradually changing the ratio of the two metals (69). This is due to alloying, an enzymatic activity dependent on the compositions as the electronic structure changes. Growing with active nanomaterials or doping with another element is a cost-effective way to tune the activity (70).
Growing with less active nanomaterials such as Au and Ag together as well as with more active ones such as Pt and Ir can enhance the activity of enzymes but also effectively utilize these noble metals (71). Its catalytic effectiveness will be at least 20 times greater than that of Pd cubes and 400 times greater than that of HRP, respectively, after multiple atomic Ir layers are coated on Pd cubes (72).
One of the challenges in nanozyme-based nanotechnology is to be multifunctional in one material. Wu’s group synthesized a high-efficiency Au@Pt multifunctional nanozyme to detect H2O2 based on a seed-mediated method. This structure possesses both plasmonic activities from the Au core and enzymatic activity from the Pt shell, thus shortening the detection time and increasing the sensitivity by 1–2 orders of magnitude (73).
To further promote the activity, the less active core is selectively etched after the highly active core grows. For example, after the Pd core is etched, the Pd-Pt core-framework nanodendrites are transferred into the Pt hollow nanodendrites, accompanied by more active sites. Besides, high-refractive-index facets are exposed to enhance peroxidase-like activity (74).
Doping is another effective strategy to modulate enzyme activities benefiting from electronic structural changes. It has been demonstrated through PdO doping that creating heterojunctions on Co3O4 nanoparticles could quantitatively tune the band gap and Fermi level to investigate how metal oxide nanoparticles’ semiconductor properties affect cellular redox homeostasis and potential hazards (75).
2.3. Application
After explorations by researchers, the enzyme-like activity of noble metal-based nanozyme materials has been continuously improved. In some aspects, it has been able to replace biological enzymes for practical purposes (Table 1).
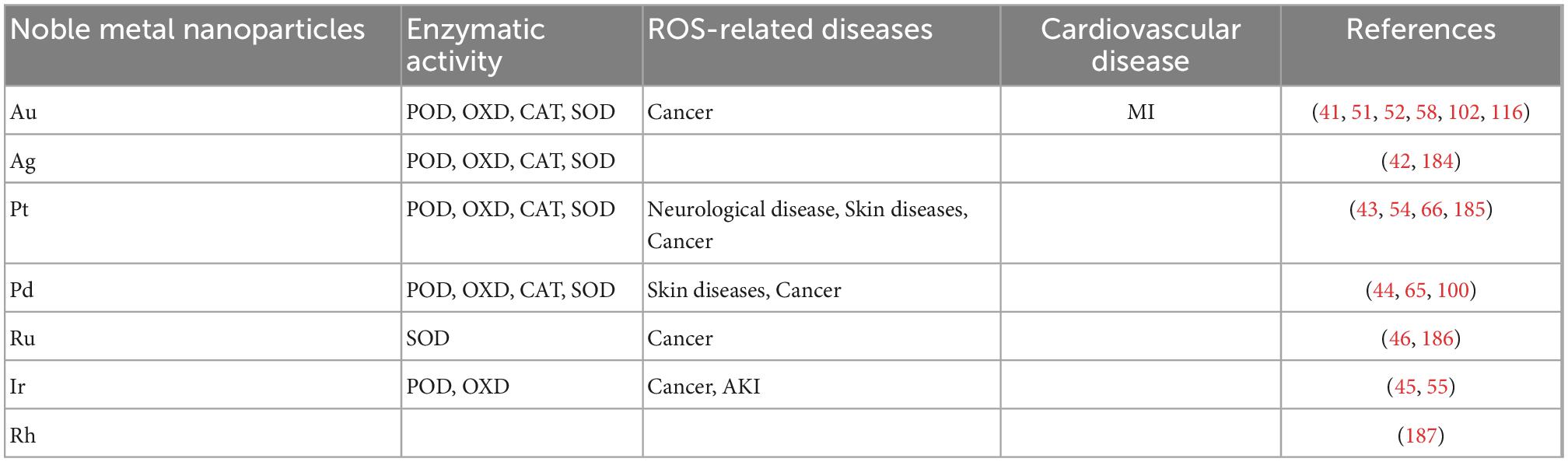
Table 1. The enzymatic mimetic activities of different precious metals and their specific therapeutic applications in several major ROS-related diseases and cardiovascular diseases.
It is well known that reactive oxygen species (ROS), such as superoxide anion (O2–), hydroxyl radical (⋅OH), and hydrogen peroxide (H2O2) are by-products of cellular metabolism (76, 77). Under normal circumstances, producing and removing ROS in the body are balanced dynamically. Low doses of ROS play an essential role in signal transduction, cell proliferation, and defense against pathogen invasion (78, 79). However, abnormally high ROS causes oxidative stress and disturbs the body’s redox equilibrium, significantly impairing the structure and function of the intracellular macromolecule (80, 81). Moreover, studies have shown that neurodegenerative diseases, cancers, diabetes, atherosclerosis, arthritis, and kidney diseases are all related to ROS accumulation (82–85). Given the anti-oxidative and pro-oxidative activities of nano-enzymes and their unique advantages, constructing nano-enzyme-based therapeutic systems and applying them in biomedicine, such as anti-inflammatory, cancer, and especially cardiovascular diseases, are discussed in the article.
2.3.1. Biological detection
The HRP, as a natural enzyme, is often used to detect the content of H2O2. Nanozymes with peroxidase-like activity can substitute HRP. Studies have proved that nanozymes are more efficient and stable in detecting H2O2 (86, 87). The content of substrates can be reflected by that of H2O2, generated by the reaction between the substrates and corresponding enzymes. So far, glucose, lactic acid (88), choline (89), cholesterol (90), and other substances have been detected precisely. Gold nanoparticles have been on the cutting edge of sensing and detection technologies for simple synthesis, surface functionalization, and superior optical and electronic properties (91). Huang’s group directly grew AuNPs on two-dimensional MOF nanosheets to prepare AuNPs/Cu-TCPP (M) (TCPP = tetrakis (4-carboxyphenyl) porphyrin. M = Fe, Co) composite was used to detect glucose based on the chromogenic method (92). To better understand the enzymatic activities of AuNPs, Zhao et al. (93) prepared a three-in-one functional nanoplatform (sensing, self-assembly, and cascade catalysis). They found that glucose could be detected when only AuNPs were used.
Wu et al. (94) achieved strong electrochemiluminescence (ECL) emission by using one type of the G-quadruplex selective iridium (III) complex as an efficient ECL signal probe. Based on the typical sandwich immunoreaction between the cardiac troponin-I antigen (cTnI) and its corresponding antibody, the iridium (III) complex was introduced according to its specific interaction with G-quadruplex DNA to modify on the surface of negatively charged gold nanoparticles (AuNPs). This induced a strengthened increased ECL signal proportional to cTnI concentration. Based on this, cTnI could be detected quantitatively ranging from 5.0 fg/mL to 100 ng/mL, with a detection limit of 1.67 fg/mL. Moreover, the proposed immunosensor successfully detected cTnI between healthy individuals and AMI patients in human serums. This suggested it was a potential candidate to be applied in the early diagnosis of AMI.
2.3.2. Disease treatment
Similar to the application mechanism of nanozymes in the field of antibacterial, how to regulate the ROS level in organisms can also be used to treat diseases. Evidence suggests that ROS levels are closely related to the aging of the skin and other organs. Multiple ROS-resistant and repair systems of complex organisms can mitigate oxidative damage to tissues. Superoxide free radicals in living organisms have solid oxidizing properties, and their excessive levels can damage various tissues, causing diseases. Superoxide dismutase (SOD) is crucial to the antioxidant system. It can decompose superoxide radicals into H2O2, which is further decomposed into H2O and O2 after being catalyzed (95).
2.3.2.1. Treatment of ROS-related diseases
Noble metal-based nanozymes can scavenge ROS and be used to treat ROS-related diseases, such as in antioxidant therapy (96, 97). In recent investigations, biorthogonal enzymes have been utilized to replace ROS to transform pharmacological precursors into hazardous medicines to treat cancer. These techniques have explored new ways to treat diseases with nanozymes (95).
The POD-like activity, photosensitization properties, and localized photothermal effect of noble metal-based nanozymes can be used to alleviate hypoxia at tumor sites and kill tumor cells photothermally and photodynamically (98–101). Therefore, noble metal-based nanozymes are a class of potential anti-tumor therapeutic materials. Gao et al. encapsulated polylactic acid with doxorubicin (DOX). Gold nanoparticles were grown on the surface of glycolic acid copolymer (PLGA). Then the surface was PEGylated to prepare multifunctional hybrid nanostructures with drug loading, CAT-like activity, photoacoustic imaging, and photothermal effect. The photothermal conversion efficiency of the obtained PLGA/DOX@PDA-Au-PEG hybrid structure was as high as 69.0% and a strong photoacoustic signal was produced. Under an 808 nm laser irradiation, the hybrid nanostructures enhanced ROS production, effectively alleviating the hypoxic condition at the tumor site. The release of DOX and the increase of ROS concentration enhanced the effect of chemotherapy/photothermal combined therapy on tumors (102).
2.3.2.2. Cardiovascular disease treatment
Cardiovascular disease (CVD) is the leading cause of death worldwide, with a poor prognosis. Nanoscience involves the cross-integration of different sciences, including physics, materials science, engineering, and biomedicine; it sets the path to transform nanotechnology into clinical research. Given the unique physical, chemical, and biological properties of nanomaterials and nanostructures combined with the recent development of biomedical devices, contrast agents, and so forth, nanotechnology has great potential in cardiovascular regenerative medicine.
2.3.2.2.1 Diagnosis and treatment of coronary atherosclerosis
Despite prevention measures, early identification of atherosclerosis, the common pathophysiological process that causes underlying cardiovascular disease, is difficult to achieve, with overt coronary artery disease or myocardial infarction considered the most commonly initial clinical manifestation. A novel approach to the detection, prevention, and treatment of atherosclerosis is presented by nanoparticles. Concerning the diagnosis of atherosclerosis, new multifunctional nanoparticles with integrated diagnostic and therapeutic capabilities are promising (103). Metal nanoparticles have been used in studies for the specific imaging or treatment of atherosclerosis. CD163 refers to a membrane receptor from the macrophage lineage. Studies on atherosclerosis have revealed higher levels of CD163 expression in inflammation-related locations, indicating the presence of intraplaque hemorrhagic sites or asymptomatic plaques. Consequently, the imaging of CD163-expressing macrophages wokrs as a novel method for the detection of atherosclerotic plaques. For the precise detection of CD163 by MRI, Carlos et al. created a targeted probe based on gold-coated iron oxide nanoparticles vectorized with an anti-CD163 antibody (104).
In recent years, inflammatory biomarkers have been demonstrated to act as an effective signal for early diagnosis of atherosclerosis (105). Integrins, macrophage scavenger receptors, VSMCs, and VCAM-1 on endothelial cells are among the most frequently detected biological targets. The VCAM-1 expression is induced early in human atheroma and represents a key element of the inflammation generated during atherosclerosis, contributing to monocyte and lymphocyte recruitment from adventitial vessels and the arterial lumen. The strict temporal and spatial regulation of endothelial adhesion molecules and their critical function in atherosclerosis make them ideal targets for diagnosis (106). Besides, there are many microstructures in late-stage atherosclerotic plaques, such as neovascularization, micro-calcification, and cholesterol crystals, which have become important foci for targeted nanomedicine delivery (107).
It has been demonstrated that atherosclerotic plaque locations display high permeability and retention effect similar to solid tumors. AuNPs drive macrophage depletion in the photothermal therapy (PTT) by converting light energy to heat energy under atherosclerotic conditions. PTT actively makes use of inorganic nanoparticles and spatial near-infrared laser wavelengths to cause intracellular hyperthermia. It is shown that gold nanorods and silicon-gold hybrid nanoparticles function in concert with PTT after the total atherosclerotic volume is reduced (106).
2.3.2.2.2 Nanotherapy for myocardial infarction
In the last few years, nanoparticles such as GNP, carbon dots, and graphene have continued to be key protagonists of different types of sensors (e.g., electrogenerated chemiluminescence sensor) to enable multiple and ultrasensitive detections of relevant cardiac biomarkers such as cTnI, cTnT, myoglobin, and glutathione (108, 109).
During the myocardial damage process, the cardiac troponin I (cTnI) is released into the bloodstream. Early detection of cTnI in the serum of patients with a higher risk of MI can decrease the risk of death. For instance, Guo et al. developed a facile and rapid solution-phase method to detect human cTnI using anti-human cTnI-labeled GNR-based biosensors (110).
Reactive oxygen species are eventually produced due to myocardial ischemia and hypoxia, which cause the pathophysiological circumstances of MI. Decreased glutathione expression is another effect of the steric overproduction of ROS. Therefore, measuring glutathione levels can help diagnose MI and ischemic heart disease. In this context, important to the pathophysiology of MI, glutathione levels are detectable using carbon-dot-based ultrasensitive biosensors. The addition of graphene oxide, mesoporous silica, and silver nanoparticles increased the sensitivity of the carbon dot-based biosensors. Carbon dots and mesoporous silica work well together as supersensing components in biosensors based on conjugated nanomaterials. Therefore, by supplying the required information for future therapy, this notion can aid in the early diagnosis of MI (109).
During the MI, the heart sustains irreparable damage (111). Factors such as the start of a pro-inflammatory cytokine cascade and the production of ROS and RNS contribute to the pathophysiology of TNF-mediated MI (112, 113). Therefore, a key technique for treating MI is effectively decreasing TNF during the inflammatory phase (114, 115). We notice that some novel payloads in NPs-based therapy for MI have been studied. For example, deoxyribozyme-AuNP can silence tumor necrosis factor-α (TNF-α) (116). As a potential treatment for myocardial infarction, they employed gold nanoparticles (AuNPs) functionalized with deoxyribozyme (DNAzyme) to catalytically quiet tumor necrosis factor (TNF) in vivo. They showed a 50% knockdown of TNF-α in primary macrophages, which was not possible with Lipofectamine-based techniques. TNF-α knockdown efficiencies of 50% were achieved following local injection of DNAzyme attached to gold particles (AuNPs) in the rat myocardium, which had strong anti-inflammatory effects and improved immediate cardiac function after MI. These findings are the first evidence to demonstrate the viability of DNAzyme AuNPs conjugates for gene regulation and delivery in vivo. This is important because TNF-α has been associated with numerous inflammatory-mediated illnesses and is a multibillion-dollar treatment target, highlighting the potential effects of DNAzyme-conjugated AuNPs (116).
3. Biomimetic nanocarriers
3.1. Development of biomimetic nanocarriers
Nano-drug carriers can encapsulate water-soluble or poorly soluble drugs. They can also simultaneously encapsulate multiple drugs to co-deliver them. They can achieve sustainability, controllability, intelligence, responsiveness, and so forth. However, it is difficult for them to imitate the natural biological structure effectively (117). Non-biological exogenous factors are inevitably brought into the body when the drug is delivered, decreasing efficacy. On the contrary, biomolecules, such as blood cells, can move freely as the blood is circulated without being opsonized or cleared. Their unique function is to protect the immune system from invading normal cells without being detected (118).
Similarly, drugs are in an environment similar to biological materials before reaching the target site. Therefore, some scholars seek to develop new drug delivery systems of drug carriers according to their biological properties and functions using biomimetic technology. In recent years, there has been continuous research on efficient and specific drug delivery using biological materials such as cell membranes, proteins, viral capsids, and liposomes as carriers (119) (Figure 1).
3.2. Classification of biomimetic nanocarriers
3.2.1. Related delivery systems for proteins, viral capsids, and liposomes
The biomimetic synthesis method using proteins as a template is a new method for nanoparticle preparation. Amino acid residues in protein molecules can interact or coordinate electrostatically with metal ions to form nucleation centers. In the cavity, the nucleation and growth of inorganic nanocrystals are induced by reduction reactions, precipitation reactions, and so forth, thereby forming protein template-based nanoparticles. The method is simple to prepare, with adjustable particle size and good biocompatibility (120).
Some researchers have developed virus-like particles (VLPs) and virions. VLPs are a class of self-assembled particles that mimic the structure of viral capsids (121). The particles’ empty architecture can be filled with a variety of materials including proteins (122), nucleic acid (123), drugs (124), and other nanoparticles (125), and they no longer contain viral genetic material. VLPs and virions can promote cellular uptake and avoid lysosomal phagocytosis, thus achieving targeted delivery. However, biosafety remains a controversial issue (126).
As materials science and nanotechnology keep maturing, many nano-formulations, such as liposomes, micelles, and nanoparticles (NPs), have been developed and used in clinical practice (127). Among them, liposomes are considered an effective drug delivery system (DDS), and over ten liposome drugs have been approved to go on the market (128). Liposomes have many advantages in drug delivery, such as good biocompatibility, biodegradability, tunable size, and surface modification (129). They are often used as embedded hydrophilic or hydrophobic reagents, improving the circulation of drugs in vivo. More importantly, its chemical composition (phospholipids) and lipid bilayer structure resemble biological membranes and are highly compatible with the biological environment. In addition, modifying targeting groups can enhance drug delivery efficiency (130).
Biomimicry, such as liposomes, has been used to construct biofilm models to study the biological functions of living cells (131, 132). However, due to its relatively simple structure, it is difficult to reproduce cell membranes’ complexity accurately.
3.2.2. Cell or cell membrane-based drug delivery systems
In recent years, people have tried to use natural cells or cell-derived vesicles as drug carriers, such as whole cells, extracellular vesicles (EVs), and membrane-encapsulated nanoparticles (133–135). Because they resemble cell membrane structures, they are recognized by the body as “self” components, showing better biocompatibility and lower toxicity. Simple to prepare, this drug delivery system can reduce the loss of membrane proteins and endow the carrier with multiple biological functions and targeting specificity without further modifications. For example, red blood cells (RBCs) are used as carriers to encapsulate small molecule drugs, nucleic acids, proteins, nanoparticles, and so forth in treating systemic diseases due to their long lifespan and good biocompatibility (136, 137). RBCs, stem cells, macrophages, neutrophils, T cells, and NK cells are just a few of the cells that have been employed for drug loading and delivery. In contrast to cell membrane-camouflaged DDSs, recent research suggests that cell-based DDSs can retain all the biological characteristics and functions of native cells, including the long lifespan of erythrocytes (red blood cells, RBCs) in circulation, the inflammatory homing ability of stem cells, macrophages, and neutrophils, and the tumor recognition and killing abilities of T cells and natural killer cells. Consequently, they are able to get beyond the drawbacks of artificial NP carriers (138).
3.2.2.1. Whole cells as drug carriers
The physiological functions of various cells in the human body vary, such as long blood circulation, specific site migration, and crossing physiological barriers. We can select specific cells to deliver drugs using their retained cellular structures and functions. Recently, the design of utilizing whole cells (erythrocytes, stem cells, immune cells, etc.) as drug carriers has become a hotspot (138).
Erythrocytes are the most employed carrier of whole cells. Compared with synthetic drug delivery systems, erythrocytes display the advantages of better biocompatibility and biodegradability with no immunogenicity. Their natural surface protects the loaded drug from being inactivated to prolong circulation time and enhance controllability, which makes the systemic drug delivery systems valuable (139). Various methods have been developed to encapsulate drugs into red blood cells to modify drugs on the surface of red blood cells chemically or physically (140).
Inflammation sites can recruit immune cells (macrophages, lymphocytes, neutrophils, etc.) (141, 142). RNA-loaded liposomes, magnetic nanoparticles, gold nanoshells, and imaging agent-loaded nanoparticles are easily engulfed by monocytes and neutrophils during circulation in the body, and immune cells can selectively cross the blood-brain barrier to allow therapeutic drugs to reach the brain (143–145).
3.2.2.2. Cell membrane-encapsulated nanoparticles as drug carriers
Membrane-based vesicles are natural drug carriers that have been used in various systems. However, due to their preparation method and liposome structure, it is challenging to load hydrophobic agents, co-deliver drugs of different properties, and produce well-controlled release (146). It is well known that polymeric or inorganic nanoparticles can fulfill the above functions, but due to their heterogeneity, they are rapidly cleared by the body. Similar to lipid-encapsulated hybrid nanoparticles, a series of cell membrane-encapsulated nanoparticles have been developed with the advantage of being multi-functional (147).
Since red blood cell membrane (RBC) encapsulation technology was developed in 2011 by Zhang et al. it has been applied to multiple systems for varying purposes (148, 149). The technology adopts a three-step method to prepare novel erythrocyte membrane-encapsulated nanoparticles. First, red blood cells are separated from the whole blood by centrifugation, then hemoglobin is removed with a hypotonic solution. Next, through extrusion molding of a polycarbonate porous membrane (100 to 400 nm), RBCm vesicles with a size of about 100 nm are obtained. The final step is to co-extrude the RBCm vesicles and nanoparticles multiple times to fuse. A lipid bilayer is observed outside the nucleus of the nanoparticles, with a surface potential close to that of RBCm vesicles. Both results confirm the successful encapsulation of the red blood cell membrane.
It is worth noting that the erythrocyte membrane-encapsulated nanoparticles synthesized by this method maintain the original membrane orientation. That is to say, the face is facing outward, and most of the protein content and glycogen density on the membrane surface are similar to those of erythrocyte, capable of stabilizing the physicochemical stability of nanoparticles, thereby prolonging the in vivo circulation time of erythrocyte membrane-coated nanoparticles, which is much longer than that of liposome-based nanoparticles (150). The chemotherapeutic drug doxorubicin DOX nanoparticles can be wrapped around the erythrocyte membrane through chemical coupling or physical encapsulation (151).
Zhang et al. extracted neutrophils (NEs) from mice and loaded paclitaxel PTX liposomes with neutrophil membranes as templates. NE can naturally cross the blood-brain barrier/blood-brain tumor barrier (BBB/BBTB) and glioma sites. Surgical resection of gliomas can release local inflammatory factors, activate NE to migrate to the inflammatory site in the brain, and “amplify the signal,” thereby improving the targeting of brain tumors and significantly inhibiting the recurrence of malignant gliomas after surgery (152, 153).
3.3. Applications
3.3.1. Biomimetic nanomaterials and other inflammation-related diseases
Thamphiwatana et al. (154) obtained the purified macrophage membranes by extracting J774 mouse macrophages through hypotonic lysis, mechanical destruction, and differential centrifugation. They used ultrasound to transform the macrophage membranes into membrane vesicles and mixed them with PLGA. It was discovered that the nanoparticles reduced the inflammation caused by LPS-induced sepsis in mice and increased their chance of survival under the condition.
Considering the critical role of neutrophils in sepsis-induced liver injury, Xiao et al. explored neutrophil cell membrane-mimicking nanomaterials (NM) as biomimetic nanomaterials for treating the sepsis-related liver injury. In a mouse model with sepsis, NM administration showed good biocompatibility and significantly reduced plasma levels of inflammatory cytokines and biomarkers of liver injury, including aspartate aminotransferase, alanine aminotransferase, and direct bilirubin. Therefore, it provides a promising therapeutic strategy for treating acute liver injury caused by sepsis (155).
Organ transplantation is often the last treatment for end-stage patients with organ diseases. Transplant rejection and inflammatory injury (such as ischemia-reperfusion) account for most failures in organ transplantation (156). Transplant tolerance is present between donors and donors. Progress in similar research has also been made in liver and skin transplantation (157, 158).
Arthritis, a chronic inflammation-related disease, is a common cause of clinical teratogenicity and disability (159). Although progress has been made in studies concerning the clinical application of anti-cytokine biologics, the therapeutic effect on arthritis remains unsatisfactory, especially with the low response rate of rheumatoid arthritis. Biomimetic nanomaterials are also used to treat arthritis (160, 161).
In addition to the diseases mentioned above, many studies have shown the applications of biomimetic nanomaterials in other inflammation-related diseases, such as inflammatory bowel disease, trauma, etc (7).
As a public health disease, COVID-19 is still raging around the world. Pathophysiological studies have shown that virus-induced cytokine release syndrome is the leading cause of death (162, 163). Knowing that the infectivity of COVID-19 depends on its interaction with known or unidentified protein receptors on target cells, Zhang et al. created a cellular nanosponge that primarily impacts host cells as opposed to pathogens (164).
3.3.2. Biomimetic nanomaterials and cardiovascular disease
Cardiovascular disease is a severe and threatening disease as one of the leading causes of death worldwide (165). Common cardiovascular diseases, such as atherosclerosis and valvular heart disease, are chronic systemic inflammatory diseases caused by obesity, autoimmune diseases, and infectious diseases, leading to cardiovascular disease occurrence and exacerbation (166). Biomimetic nanomaterials are also currently used to prevent and treat cardiovascular diseases (167). For instance, Hu et al. obtained a new biomimetic valve by cross-linking erythrocyte membrane-coated drug nanoparticles and an artificial heart valve, which reduces the toxic effect of glutaraldehyde on the heart valve but also considerably improves the tissue of the artificial heart valve (168).
3.3.2.1. Applications in the diagnosis and treatment of atherosclerosis
Atherosclerosis is one of the pathological bases of cardiovascular disease. It is mainly a progressive inflammatory disease involving lipid deposition and massive accumulation of immune cells, eventually forming a fibrous cap on the arterial wall. Among them, dyslipidemia is the most important influencing factor of atherosclerosis. A recent study designed dual-targeted biomimetic nanocomposite particles with a core-shell structure. The research results showed that through a positive feedback loop, the novel multifunctional biomimetic nanoparticles could dynamically enhance the targeting of plaques while achieving the dual effects of inhibiting cholesterol deposition and enhancing efflux. It provides a new treatment to reduce plaque formation and alleviate atherosclerosis (169). Gao et al. and Wang et al. prepared reactive oxygen species responsive nanoparticles (ROS-responsive NPs). They then coated them with the extracted macrophage membrane to obtain the macrophage membrane-coated ROS-responsive nanoparticles. Nanoparticles effectively avoid being cleared by the body’s reticuloendothelial cells, help to target the inflammatory site, realize the sustained release of drugs in the inflammatory site, and reduce the production of reactive oxygen species and the risk of atherosclerotic disease in mice (170, 171). In the sclerosis model, this biomimetic nanomaterial showed a superior therapeutic effect. Based on a similar method, Boada et al. loaded rapamycin on nanoparticles and then coated the nanoparticles with a macrophage membrane. The biomimetic nanomaterials improved the histocompatibility and sustained the release efficiency of the drug. In the mouse model with high-fat diet-induced vasculitis, this newly drug-loaded biomimetic nanoparticle better relieved the vascular inflammation and organ damage to mice (172). Song et al. used PLGA nanoparticles to encapsulate rapamycin and the outer membrane of red blood cells to treat atherosclerosis. They found that red blood cell membranes could reduce the phagocytosis of nanoparticles by macrophages in the blood circulation and enhance their phagocytosis. Aggregation in plaques to achieve sustained release of rapamycin reduces atherosclerotic plaques. Platelet membranes have been used in some studies to modify PLGA rapamycin nanoparticles and obtained similar effects (173).
In addition to reducing the formation of atherosclerotic plaques, the biomimetic nano-delivery system can also be used as an imaging tool to assess the progression of atherosclerosis (174). Zhang et al. took advantage of the natural feature of platelets involved in the disease to extract platelet membranes. Construction of platelet membrane-encapsulated PLGA Nano-Bionic Particles (PNPs). The contrast agent gadolinium is modified on the surface of PNPs to obtain the targeted modal contrast agent GdPNP. MRI analysis showed strong positive contrast 1 h after administration, indicating that Gd-PNP can target the plaques accumulating in the aortic arch in a short time. The formation site enables MRI imaging at the plaque to track the progression of atherosclerosis (175).
3.3.2.2. Role in the repair of cardiac injury
Cardiac damage is mainly due to ischemic necrosis of myocardial cells caused by long-term stenosis or the occlusion of coronary arteries. At the same time, the myocardial ischemia site has vascular endothelial cell damage, collagen exposure, high vascular permeability, and many inflammatory cells, including macrophages. The current clinical drugs for the treatment of myocardial ischemia mainly rely on growth factors, cytokines, and small molecular compounds to improve symptoms and delay cardiac injury. As cell membranes or membrane-like structures have evolved, biomimetic nano-drug delivery systems are expected to achieve efficient “active targeting” of nanoparticles. A platelet-inspired nanocell (PINC) that incorporates prostaglandin E2 (PGE2)-modified platelet membrane and cardiac stromal cell-secreted factors to target the heart after ischemia-reperfusion injury (I/RI) is introduced. The dual roles of targeting and repairing the damaged heart can thus be achieved (176). Studies have confirmed that microvesicles such as exosomes secreted from the iPSC-derived cardiomyocytes exert protective effects by transferring the endogenous molecules to salvage the injured neighboring cells by regulating apoptosis, inflammation, fibrosis, and angiogenesis (177). However, how to efficiently deliver it to the heart remains to be explored. Cardiomyocyte-specific peptides are linked to amp2b-positive exosomal membrane structures, thereby enhancing exosome endocytosis in cardiomyocytes (178). Alternatively, the peptides targeting the heart can be conjugated to exosomes by the linker to make exosomes target the damaged heart more effectively, thereby reducing myocardial fibrosis in the damaged heart. Some of these effects are mediated by the transfer of microRNA (miRNA) to the heart (179).
Huang et al. constructed a long-circulation sustained-release H2S system to explore how it prevented myocardial ischemia-reperfusion (I/R) injury. Red blood cell (RBC) membrane diallyl trisulfide (DATS) supported mesoporous iron oxide nanoparticles (MIONs) (RBC-DATs-MIONs) were highly biocompatible. They prolonged the circulation time and controlled the release of H2S in the plasma and myocardium. They showed excellent therapeutic efficacy in an in vitro hypoxia/reoxygenation and in vivo myocardial I/R model involving multiple mechanisms. For instance, the anti-apoptotic, anti-injury, and antioxidant activity (180).
Su et al. introduced a platelet-excited nanocell (PINC) that incorporates prostaglandin E2 (PGE2) -modified platelet membrane and cardiac stromal cell-secreted factors to target the heart after the I/R injury. In a mouse model of the myocardial I/R injury, intravenous PINCs enhanced cardiac function and attenuated cardiac remodeling. Meanwhile, they improved cardiomyocyte circulation, activated endogenous stem/progenitor cells, and promoted angiogenesis (176).
Lin et al. prepared a biointelligent nanoparticle (MTSNP) with microenvironment targeting and adaptability to repair the ischemic tissue. During the acute phase of ischemia, melatonin is rapidly released from MTSNP. The process scavenged reactive oxygen species, activated melatonin receptor I on MT, and prevented cytochrome C release, activating apoptosis. In the chronic phase, circular DNA could sense the hypoxia and secreted VEGF to revascularize as a response. Importantly, circular DNA might adversely affect receiving feedback from the revascularization and shut down VEGF secretion. The therapeutic potential of MTSNP in myocardial ischemia was verified by various methods (181).
3.3.2.3. Application in other cardiovascular diseases
Thrombosis is involved in the pathophysiological process of diseases, such as atherosclerosis and MI. Nie et al. used platelet nanoparticles extracted from platelets to carry thrombolytic drugs in the targeted thrombolytic therapy (182). Clinically, in-stent restenosis occurs when the platelet aggregates, excessive smooth muscle cell proliferates, and inflammatory cell aggregates. The current research mainly focuses on endothelialization after stent placement. The research is based on constructing liposomes or PLGA nanoparticles to transport proteins or genes such as vascular endothelial growth factor and angiopoietin (183).
4. Conclusion
In conclusion, biomimetic nanomaterials and biomimetic nanodelivery systems are anticipated to become potentially beneficial technologies for disease diagnosis and therapy, enabling nanomedicine to represent significant potential value for medical applications in the diagnosis and treatment of the cardiovascular system. However, its applications still face considerable challenges: biostability, biodistribution, biocompatibility, and biosafety of nanoparticles, for instance. As a result, nanoparticles that are highly selective, toxic-free, and more applicable in therapeutic settings are required. Further optimization is necessary to target biomimetic nanoparticles, high ligand affinities, circulation in vivo, and cytotoxicity. Biomimetic nanomedicine research is still in the early stage until biomimetic nanoparticles that target inflammation are introduced into clinical settings.
Author contributions
All authors participated in the creation of this manuscript, contributed to the article, and approved the submitted version.
Funding
This work was supported by the grants from the National Key R&D Program of China (2017YFC1103601) and the Elite Medical Professionals Project of China-Japan Friendship Hospital (No. ZRJY2021-BJ07).
Conflict of interest
The authors declare that the research was conducted in the absence of any commercial or financial relationships that could be construed as a potential conflict of interest.
Publisher’s note
All claims expressed in this article are solely those of the authors and do not necessarily represent those of their affiliated organizations, or those of the publisher, the editors and the reviewers. Any product that may be evaluated in this article, or claim that may be made by its manufacturer, is not guaranteed or endorsed by the publisher.
References
1. Zhang F, Xia Y, Liu Y, Leng J. Nano/microstructures of shape memory polymers: from materials to applications. Nanoscale Horiz. (2020). 5:1155–73. doi: 10.1039/d0nh00246a
2. Garg D, Sarkar A, Chand P, Bansal P, Gola D, Sharma S, et al. Synthesis of silver nanoparticles utilizing various biological systems: mechanisms and applications-a review. Prog Biomater. (2020) 9:81–95. doi: 10.1007/s40204-020-00135-2
3. Chavoustie SE, Carter BA, Waldbaum AS, Donders GG, Peters KH, Schwebke JR, et al. Two phase 3, double-blind, placebo-controlled studies of the efficacy and safety of astodrimer 1% gel for the treatment of bacterial vaginosis. Eur J Obstet Gynecol Reprod Biol. (2020) 245:13–8. doi: 10.1016/j.ejogrb.2019.11.032
4. Drexler E, Peterson C, Pergamit G. Unbounding the Future: the Nanotechnology Revolution. Fort Mill, SC: Quill Publisher (1991).
5. Yaman S, Chintapula U, Rodriguez E, Ramachandramoorthy H, Nguyen KT. Cell-mediated and cell membrane-coated nanoparticles for drug delivery and cancer therapy. Cancer Drug Resist. (2020) 3:879–911. doi: 10.20517/cdr.2020.55
6. Wolfram J, Zhu M, Yang Y, Shen J, Gentile E, Paolino D, et al. Safety of nanoparticles in medicine. Curr Drug Targets. (2015) 16:1671–81.
7. Wang H, Liu Y, He R, Xu D, Zang J, Weeranoppanant N, et al. Cell membrane biomimetic nanoparticles for inflammation and cancer targeting in drug delivery. Biomater Sci. (2020) 8:552–68. doi: 10.1039/C9BM01392J
8. Bordoni A, Danesi F, Dardevet D, Dupont D, Fernandez AS, Gille D, et al. Dairy products and inflammation: a review of the clinical evidence. Crit Rev Food Sci Nutr. (2017) 57:2497–525. doi: 10.1080/10408398.2014.967385
9. Silvestre-Roig C, Braster Q, Ortega-Gomez A, Soehnlein O. Neutrophils as regulators of cardiovascular inflammation. Nat Rev Cardiol. (2020) 17:327–40. doi: 10.1038/s41569-019-0326-7
10. Adamo L, Rocha-Resende C, Prabhu SD, Mann DL. Publisher correction: reappraising the role of inflammation in heart failure. Nat Rev Cardiol. (2021) 18:735–735. doi: 10.1038/s41569-021-00534-3
11. Truby LK, Rogers JG. Advanced heart failure: epidemiology, diagnosis, and therapeutic approaches. JACC Heart Fail. (2020) 8:523–36. doi: 10.1016/j.jchf.2020.01.014
12. Nonaka M, Hosoda H, Uezono Y. Cancer treatment-related cardiovascular disease: current status and future research priorities. Biochem Pharmacol. (2021) 190:114599. doi: 10.1016/j.bcp.2021.114599
13. Jin K, Luo Z, Zhang B, Pang Z. Biomimetic nanoparticles for inflammation targeting. Acta Pharm Sin B. (2018) 8:23–33. doi: 10.1016/j.apsb.2017.12.002
14. Gao L, Zhuang J, Nie L, Zhang J, Zhang Y, Gu N, et al. Intrinsic peroxidase-like activity of ferromagnetic nanoparticles. Nat Nanotechnol. (2007) 2:577–83. doi: 10.1038/nnano.2007.260
15. Ragg R, Tremel W. Solids go bio: inorganic nanoparticles as enzyme mimics. Eur J Inorg Chem. (2016) 2016:1896–1896. doi: 10.1002/ejic.201600408
16. Jiang B, Liang M. Advances in single-atom nanozymes research. Chinese J Chem. (2021) 39:174–80. doi: 10.1002/cjoc.202000383
17. Wang H, Wan K, Shi X. Recent advances in nanozyme research. Adv Mater. (2019) 31:e1805368. doi: 10.1002/adma.201805368
18. Zhuang J, Zhang X, Liu Q, Zhu M, Huang X. Targeted delivery of nanomedicines for promoting vascular regeneration in ischemic diseases. Theranostics. (2022) 12:6223–41. doi: 10.7150/thno.73421
19. Wang Q, Gao L. Nanozyme-based medicine for enzymatic therapy: progress and challenges. Biomed Mater. (2021) 16:042002. doi: 10.1088/1748-605X/abe7b4
20. Yang B, Chen Y, Shi J. Nanocatalytic medicine. Adv Mater. (2019) 31:e1901778. doi: 10.1002/adma.201901778
21. Li Z, Feng K, Wei Z, Ming M, Ning G, Yu Z. Catalytic mechanism and application of nanozymes. Chin Sci Bull. (2018) 63:2128–39. doi: 10.1360/N972018-00426
22. Dong HJ, Zhang C, Fan YY, Zhang W, Zhang Y. Nanozyme and their ROS regulation effect in cells. Prog Biochem Biophys. (2018) 45:105–17. doi: 10.16476/j.pibb.2017.0460
23. Azharuddin M, Zhu GH, Das D, Ozgur E, Uzun L, Turner AP, et al. A repertoire of biomedical applications of noble metal nanoparticles. Chem Commun. (2019) 55:6964–96. doi: 10.1039/c9cc01741k
24. Klębowski B, Depciuch J, Parlińska-Wojtan M, Baran J. Applications of noble metal-based nanoparticles in medicine. Int J Mol Sci. (2018) 19:4031. doi: 10.3390/ijms19124031
25. He S, Yang L, Lin M, Balasubramanian P, Peng H, Kuang Y, et al. Platinum group element-based nanozymes for biomedical applications: an overview. Biomed Mater. (2021) 16:032001. doi: 10.1088/1748-605X/abc904
26. Doria G, Conde J, Veigas B, Giestas L, Almeida C, Assunção M, et al. Noble metal nanoparticles for biosensing applications. Sensors. (2012) 12:1657–87. doi: 10.3390/s120201657
27. Sharifi M, Faryabi K, Talaei AJ, Shekha MS, Ale-Ebrahim M, Salihi A, et al. Antioxidant properties of gold nanozyme: a review. J Mol Liquids. (2020) 297:112004. doi: 10.1016/j.molliq.2019.112004
28. Zámbó D, Rusch P, Bigall NC. Noble-metal nanorod cryoaerogels with electrocatalytically active surface sites. ACS Appl Mater Interfaces. (2021) 13:57774–85. doi: 10.1021/acsami.1c16424
29. Biswas A, Shukla A, Maiti P. Biomaterials for interfacing cell imaging and drug delivery: an overview. Langmuir. (2019) 35:12285–305. doi: 10.1021/acs.langmuir.9b00419
30. Pei X, Zhang B, Tang J, Liu B, Lai W, Tang D. Sandwich-type immunosensors and immunoassays exploiting nanostructure labels: a review. Anal Chim Acta. (2013) 758:1–18. doi: 10.1016/j.aca.2012.10.060
31. Luo L, Zhu F, Tian R, Li L, Shen S, Yan X, et al. Composition-graded PdxNi1–x nanospheres with Pt monolayer shells as high-performance electrocatalysts for oxygen reduction reaction. ACS Catalysis. (2017) 7:5420–30. doi: 10.1021/acscatal.7b01775
32. Kannan P, Maduraiveeran G. Bimetallic nanomaterials-based electrochemical biosensor platforms for clinical applications. Micromachines. (2021) 13:76. doi: 10.3390/mi13010076
33. Choi HS, Liu W, Misra P, Tanaka E, Zimmer JP, Itty Ipe B, et al. Renal clearance of quantum dots. Nat Biotechnol. (2007) 25:1165–70. doi: 10.1038/nbt1340
34. Loynachan CN, Soleimany AP, Dudani JS, Lin Y, Najer A, Bekdemir A, et al. Renal clearable catalytic gold nanoclusters for in vivo disease monitoring. Nat Nanotechnol. (2019) 14:883–90. doi: 10.1038/s41565-019-0527-6
35. Kang H, Han M, Xue J, Baek Y, Chang J, Hu S, et al. Renal clearable nanochelators for iron overload therapy. Nat Commun. (2019) 10:5134. doi: 10.1038/s41467-019-13143-z
36. Zhao Y, Zhou H, Zhang S, Xu J. The synthesis of metal nanoclusters and their applications in bio-sensing and imaging. Methods Appl Fluoresc. (2019) 8:012001. doi: 10.1088/2050-6120/ab57e7
37. Vlasova I. Peroxidase activity of human hemoproteins: keeping the fire under control. Molecules. (2018) 23:2561. doi: 10.3390/molecules23102561
38. Ricoux R, Sauriat-Dorizon H, Girgenti E, Blanchard D, Mahy JP. Hemoabzymes: towards new biocatalysts for selective oxidations. J Immunol Methods. (2002) 269:39–57. doi: 10.1016/s0022-175900223-5
39. Zandieh M, Liu J. Nanozyme catalytic turnover and self-limited reactions. ACS Nano. (2021) 15:15645–55. doi: 10.1021/acsnano.1c07520
40. Liang M, Yan X. Nanozymes: from new concepts, mechanisms, and standards to applications. Acc Chem Res. (2019) 52:2190–200. doi: 10.1021/acs.accounts.9b00140
41. Navyatha B, Singh S, Nara S. AuPeroxidase nanozymes: promises and applications in biosensing. Biosens Bioelectron. (2021) 175:112882. doi: 10.1016/j.bios.2020.112882
42. Wang D, Zhang B, Ding H, Liu D, Xiang J, Gao XJ, et al. TiO supported single Ag atoms nanozyme for elimination of SARS-CoV2. Nano Today. (2021) 40:101243. doi: 10.1016/j.nantod.2021.101243
43. Jin L, Meng Z, Zhang Y, Cai S, Zhang Z, Li C, et al. Ultrasmall Pt nanoclusters as robust peroxidase mimics for colorimetric detection of glucose in human serum. ACS Appl Mater Interfaces. (2017) 9:10027–33. doi: 10.1021/acsami.7b01616
44. Huang Q, Zhang J, Li W, Fu Y. A heparin-modified palladium nanozyme for photometric determination of protamine. Mikrochim Acta. (2020) 187:226. doi: 10.1007/s00604-020-4208-9
45. Yim G, Kim CY, Kang S, Min D, Kang K, Jang H. Intrinsic peroxidase-mimicking Ir nanoplates for nanozymatic anticancer and antibacterial treatment. ACS Appl Mater Interfaces. (2020) 12:41062–70. doi: 10.1021/acsami.0c10981
46. He L, Li Y, Wu Q, Wang DM, Li CM, Huang CZ, et al. Ru(III)-based metal-organic gels: intrinsic horseradish and NADH peroxidase-mimicking nanozyme. ACS Appl Mater Interfaces. (2019) 11:29158–66. doi: 10.1021/acsami.9b09283
47. Wei Z, Xi Z, Vlasov S, Ayala J, Xia X. Nanocrystals of platinum-group metals as peroxidase mimics for in vitro diagnostics. Chem Commun. (2020) 56:14962–75. doi: 10.1039/d0cc06575g
48. Leifert A, Pan-Bartnek Y, Simon U, Jahnen-Dechent W. Molecularly stabilized ultrasmall gold nanoparticles: synthesis, characterization, and bioactivity. Nanoscale. (2013) 5:6224–42. doi: 10.1039/c3nr00916e
49. Daniel MC, Astruc D. Gold nanoparticles: assembly, supramolecular chemistry, quantum-size-related properties, and applications toward biology, catalysis, and nanotechnology. Chem Rev. (2004) 104:293–346. doi: 10.1021/cr030698
50. Comotti M, Della Pina C, Rossi M. The catalytic activity of “naked” gold particles. Angew Chem Int Ed. (2004) 43:5812–5. doi: 10.1002/anie.200460446
51. Hafez ME, Ma H, Ma W, Long Y. Unveiling the intrinsic catalytic activities of single-gold-nanoparticle-based enzyme mimetics. Angew Chem Int Ed Engl. (2019) 58:6327–32. doi: 10.1002/anie.201901384
52. Luo W, Zhu C, Su S, Li D, He Y, Huang Q, et al. Self-catalyzed, self-limiting growth of glucose oxidase-mimicking gold nanoparticles. ACS Nano. (2010) 4:7451–8. doi: 10.1021/nn102592h
53. Gudkov SV, Serov DA, Astashev ME, Semenova AA, Lisitsyn AB. AgO nanoparticles as a candidate for antimicrobial compounds of the new generation. Pharmaceuticals. (2022) 15:968. doi: 10.3390/ph15080968
54. Yu C, Chen T, Jiang J, Tseng W. Lysozyme-directed synthesis of platinum nanoclusters as a mimic oxidase. Nanoscale. (2014) 6:9618–24. doi: 10.1039/c3nr06896j
55. Cui M, Zhao Y, Wang C, Song Q. The oxidase-like activity of iridium nanoparticles, and their application to colorimetric determination of dissolved oxygen. Microchim Acta. (2017) 184:3113–9.
56. Lou-Franco J, Das B, Elliott C, Cao C. Gold nanozymes: from concept to biomedical applications. Nanomicro Lett. (2020) 13:10. doi: 10.1007/s40820-020-00532-z
57. Mikolajczak DJ, Berger AA, Koksch B. Catalytically active peptide-gold nanoparticle conjugates: prospecting for artificial enzymes. Angew Chem Int Ed. (2020) 59:8776–85. doi: 10.1002/anie.201908625
58. Lin Y, Ren J, Qu X. Nano-gold as artificial enzymes: hidden talents. Adv Mater. (2014) 26:4200–17. doi: 10.1002/adma.201400238
59. Fan J, Yin JJ, Ning B, Wu X, Hu Y, Ferrari M, et al. Direct evidence for catalase and peroxidase activities of ferritin-platinum nanoparticles. Biomaterials. (2011) 32:1611–8. doi: 10.1016/j.biomaterials.2010.11.004
60. Jv Y, Li B, Cao R. Positively-charged gold nanoparticles as peroxidase mimic and their application in hydrogen peroxide and glucose detection. Chem Commun. (2010) 46:8017–9. doi: 10.1039/c0cc02698k
61. He W, Zhou YT, Wamer WG, Hu X, Wu X, Zheng Z, et al. Intrinsic catalytic activity of Au nanoparticles with respect to hydrogen peroxide decomposition and superoxide scavenging. Biomaterials. (2013) 34:765–73. doi: 10.1016/j.biomaterials.2012.10.010
62. Zhang L, Laug L, Münchgesang W, Pippel E, Gösele U, Brandsch M, et al. Reducing stress on cells with apoferritin-encapsulated platinum nanoparticles. Nano Lett. (2010) 10:219–23. doi: 10.1021/nl903313r
63. Shen X, Liu W, Gao X, Lu Z, Wu X, Gao X. Mechanisms of oxidase and superoxide dismutation-like activities of gold, silver, platinum, and palladium, and their alloys: a general way to the activation of molecular oxygen. J Am Chem Soc. (2015) 137:15882–91. doi: 10.1021/jacs.5b10346
64. Zhang CX, Lippard SJ. New metal complexes as potential therapeutics. Curr Opin Chem Biol. (2003) 7:481–9. doi: 10.1016/s1367-593100081-4
65. Wen T, He W, Chong Y, Liu Y, Yin J, Wu X. Exploring environment-dependent effects of Pd nanostructures on reactive oxygen species (ROS) using electron spin resonance (ESR) technique: implications for biomedical applications. Phys Chem Chem Phys. (2015) 17:24937–43. doi: 10.1039/C5CP04046A
66. Kajita M, Hikosaka K, Iitsuka M, Kanayama A, Toshima N, Miyamoto Y. Platinum nanoparticle is a useful scavenger of superoxide anion and hydrogen peroxide. Free Radic Res. (2007) 41:615–26. doi: 10.1080/10715760601169679
67. Huang X, Mahmudul HM, Li Z, Deng X, Su X, Xiao Z, et al. Noble metal nanomaterials for the diagnosis and treatment of hematological malignancies. Front Biosci. (2022) 27:40. doi: 10.31083/j.fbl2702040
68. Fu Y, Zhao X, Zhang J, Li W. DNA-based platinum nanozymes for peroxidase mimetics. J Phys Chem C. (2014) 118:18116–25. doi: 10.1021/jp503242e
69. He W, Wu X, Liu J, Hu X, Zhang K, Hou S, et al. Design of AgM bimetallic alloy nanostructures (M = Au, Pd, Pt) with tunable morphology and peroxidase-like activity. Chem Mater. (2010) 22:2988–94. doi: 10.1021/cm100393v
70. Wang C, An C, Qin C, Gomaa H, Deng Q, Wu S, et al. Noble metal-based catalysts with core-shell structure for oxygen reduction reaction: progress and prospective. Nanomaterials. (2022) 12:2480. doi: 10.3390/nano12142480
71. Wang J, Gu H. Novel metal nanomaterials and their catalytic applications. Molecules. (2015) 20:17070–92. doi: 10.3390/molecules200917070
72. Jiang X, Wang H, Wang H, Zhuo Y, Yuan R, Chai Y. Self-enhanced N-(aminobutyl)-N-(ethylisoluminol) derivative-based electrochemiluminescence immunosensor for sensitive laminin detection using PdIr cubes as a mimic peroxidase. Nanoscale. (2016) 8:8017–23. doi: 10.1039/c6nr00229c
73. Wu J, Qin K, Yuan D, Tan J, Qin L, Zhang X, et al. Rational design of Au@Pt multibranched nanostructures as bifunctional nanozymes. ACS Appl Mater Interfaces. (2018) 10:12954–9. doi: 10.1021/acsami.7b17945
74. Zhang X, Mao X, Li S, Dong W, Huang Y. Tuning the oxidase mimics activity of manganese oxides via control of their growth conditions for highly sensitive detection of glutathione. Sens Actuat B Chem. (2018) 258:80–7. doi: 10.1016/j.snb.2017.11.094
75. Zhang H, Pokhrel S, Ji Z, Meng H, Wang X, Lin S, et al. PdO doping tunes band-gap energy levels as well as oxidative stress responses to a Co3O4 p-type semiconductor in cells and the lung. J Am Chem Soc. (2014) 136:6406–20. doi: 10.1021/ja501699e
76. Bensaad K, Cheung EC, Vousden KH. Modulation of intracellular ROS levels by TIGAR controls autophagy. EMBO J. (2009) 28:3015–26. doi: 10.1038/emboj.2009.242
77. Dan Dunn J, Alvarez LA, Zhang X, Soldati T. Reactive oxygen species and mitochondria: a nexus of cellular homeostasis. Redox Biol. (2015) 6:472–85. doi: 10.1016/j.redox.2015.09.005
78. Schieber M, Chandel NS. ROS function in redox signaling and oxidative stress. Curr Biol. (2014) 24:R453–62. doi: 10.1016/j.cub.2014.03.034
79. Dharmaraja AT. Role of reactive oxygen species (ROS) in therapeutics and drug resistance in cancer and bacteria. J Med Chem. (2017) 60:3221–40. doi: 10.1021/acs.jmedchem.6b01243
80. Agita A, Alsagaff MT. Inflammation, immunity, and hypertension. Acta Med Indones. (2017) 49:158–65.
81. Sreedhar A, Aguilera-Aguirre L, Singh KK. Mitochondria in skin health, aging, and disease. Cell Death Dis. (2020) 11:444. doi: 10.1038/s41419-020-2649-z
82. Newsholme P, Haber EP, Hirabara SM, Rebelato EL, Procopio J, Morgan D, et al. Diabetes associated cell stress and dysfunction: role of mitochondrial and non-mitochondrial ROS production and activity. J Physiol. (2007) 583:9–24. doi: 10.1113/jphysiol.2007.135871
83. Bolduc JA, Collins JA, Loeser RF. Reactive oxygen species, aging and articular cartilage homeostasis. Free Radic Biol Med. (2019) 132:73–82. doi: 10.1016/j.freeradbiomed.2018.08.038
84. Aranda-Rivera AK, Cruz-Gregorio A, Aparicio-Trejo OE, Pedraza-Chaverri J. Mitochondrial redox signaling and oxidative stress in kidney diseases. Biomolecules. (2021) 11:1144. doi: 10.3390/biom11081144
85. Hu X, Zhao P, Lu Y, Liu Y. ROS-based nanoparticles for atherosclerosis treatment. Materials. (2021) 14:6921. doi: 10.3390/ma14226921
86. Liu X, Huang D, Lai C, Qin L, Zeng G, Xu P, et al. Peroxidase-like activity of smart nanomaterials and their advanced application in colorimetric glucose biosensors. Small. (2019) 15:e1900133. doi: 10.1002/smll.201900133
87. Wan L, Liu J, Huang X. Novel magnetic nickel telluride nanowires decorated with thorns: synthesis and their intrinsic peroxidase-like activity for detection of glucose. Chem Commun. (2014) 50:13589–91. doi: 10.1039/c4cc06684g
88. Hu A, Liu Y, Deng H, Hong G, Liu A, Lin X, et al. Fluorescent hydrogen peroxide sensor based on cupric oxide nanoparticles and its application for glucose and L-lactate detection. Biosens Bioelectron. (2014) 61:374–8. doi: 10.1016/j.bios.2014.05.048
89. Shadlaghani A, Farzaneh M, Kinser D, Reid RC. Direct electrochemical detection of glutamate, acetylcholine, choline, and adenosine using non-enzymatic electrodes. Sensors. (2019) 19:447. doi: 10.3390/s19030447
90. Kim MI, Cho D, Park HG. Colorimetric quantification of glucose and cholesterol in human blood using a nanocomposite entrapping magnetic nanoparticles and oxidases. J Nanosci Nanotechnol. (2015) 15:7955–61. doi: 10.1166/jnn.2015.11227
91. Tabatabaei MS, Islam R, Ahmed M. Applications of gold nanoparticles in ELISA, PCR, and immuno-PCR assays: a review. Anal Chim Acta. (2021) 1143:250–66. doi: 10.1016/j.aca.2020.08.030
92. Huang Y, Zhao M, Han S, Lai Z, Yang J, Tan C, et al. Growth of Au nanoparticles on 2D metalloporphyrinic metal-organic framework nanosheets used as biomimetic catalysts for cascade reactions. Adv Mater. (2017) 29:1700102. doi: 10.1002/adma.201700102
93. Zhao Y, Huang Y, Zhu H, Zhu Q, Xia Y. Three-in-one: sensing, self-assembly, and cascade catalysis of cyclodextrin modified gold nanoparticles. J Am Chem Soc. (2016) 138:16645–54. doi: 10.1021/jacs.6b07590
94. Wu S, Zou S, Wang S, Li Z, Ma D, Miao X. CTnI diagnosis in myocardial infarction using G-quadruplex selective Ir(III) complex as effective electrochemiluminescence probe. Talanta. (2022) 248:123622. doi: 10.1016/j.talanta.2022.123622
95. Tonga GY, Jeong Y, Duncan B, Mizuhara T, Mout R, Das R, et al. Supramolecular regulation of bioorthogonal catalysis in cells using nanoparticle-embedded transition metal catalysts. Nat Chem. (2015) 7:597–603. doi: 10.1038/nchem.2284
96. Huang Y, Liu C, Pu F, Liu Z, Ren J, Qu X. A GO-Se nanocomposite as an antioxidant nanozyme for cytoprotection. Chem Commun. (2017) 53:3082–5. doi: 10.1039/c7cc00045f
97. Jiao L, Yan H, Wu Y, Gu W, Zhu C, Du D, et al. When nanozymes meet single-atom catalysis. Angewandte Chem Int Ed. (2020) 59:2565–76. doi: 10.1002/anie.201905645
98. Toyokuni S, Okamoto K, Yodoi J, Hiai H. Persistent oxidative stress in cancer. FEBS Lett. (1995) 358:1–3. doi: 10.1016/0014-579301368-B
99. Sun H, Gao N, Dong K, Ren J, Qu X. Graphene quantum dots-band-aids used for wound disinfection. ACS Nano. (2014) 8:6202–10. doi: 10.1021/nn501640q
100. Wen T, Zhang H, Chong Y, Wamer W, Yin J, Wu X. Probing hydroxyl radical generation from H2O2 upon plasmon excitation of gold nanorods using electron spin resonance: molecular oxygen-mediated activation. Nano Res. (2016) 9:1663–73. doi: 10.1007/s12274-016-1060-7
101. Ge C, Wu R, Chong Y, Fang G, Jiang X, Pan Y, et al. Synthesis of Pt hollow nanodendrites with enhanced peroxidase-like activity against bacterial infections: implication for wound healing. Adv Funct Mater. (2018) 28:1801484. doi: 10.1002/adfm.201801484
102. Xi J, Wei G, An L, Xu Z, Xu Z, Fan L, et al. Correction to copper/carbon hybrid nanozyme: tuning catalytic activity by the copper state for antibacterial therapy. Nano Lett. (2020) 20:800. doi: 10.1021/acs.nanolett.9b04878
103. Bejarano J, Navarro-Marquez M, Morales-Zavala F, Morales JO, Garcia-Carvajal I, Araya-Fuentes E, et al. Nanoparticles for diagnosis and therapy of atherosclerosis and myocardial infarction: evolution toward prospective theranostic approaches. Theranostics. (2018) 8:4710–32. doi: 10.7150/thno.26284
104. Tarin C, Carril M, Martin-Ventura JL, Markuerkiaga I, Padro D, Llamas-Granda P, et al. Targeted gold-coated iron oxide nanoparticles for CD163 detection in atherosclerosis by MRI. Sci Rep. (2015) 5:17135. doi: 10.1038/srep17135
105. Pissuwan D, Hattori Y. Detection of adhesion molecules on inflamed macrophages at early-stage using SERS probe gold nanorods. Nanomicro Lett. (2017) 9:8. doi: 10.1007/s40820-016-0111-7
106. Cervadoro A, Palomba R, Vergaro G, Cecchi R, Menichetti L, Decuzzi P, et al. Targeting inflammation with nanosized drug delivery platforms in cardiovascular diseases: immune cell modulation in atherosclerosis. Front Bioeng Biotechnol. (2018) 6:177. doi: 10.3389/fbioe.2018.00177
107. Ou LC, Zhong S, Ou JS, Tian JW. Application of targeted therapy strategies with nanomedicine delivery for atherosclerosis. Acta Pharmacol Sin. (2021) 42:10–7. doi: 10.1038/s41401-020-0436-0
108. Zheng F, Nan L. Study of the detection technology of biomarkers of acute myocardial infarction in serum sample by high-throughput suspension array. Genom Appl Biol. (2017) 36:5014–20. doi: 10.13417/j.gab.036.005014
109. Li Z, Zhang J, Li Y, Zhao S, Zhang P, Zhang Y, et al. Carbon dots based photoelectrochemical sensors for ultrasensitive detection of glutathione and its applications in probing of myocardial infarction. Biosens Bioelectron. (2018) 99:251–8. doi: 10.1016/j.bios.2017.07.065
110. Guo Z, Gu C, Fan X, Bian Z, Wu H, Yang D, et al. Fabrication of anti-human cardiac troponin i immunogold nanorods for sensing acute myocardial damage. Nanoscale Res Lett. (2009) 4:1428–33. doi: 10.1007/s11671-009-9415-6
111. Reyes-Retana JA, Duque-Ossa LC. Acute myocardial infarction biosensor: a review from bottom up. Curr Probl Cardiol. (2021) 46:100739. doi: 10.1016/j.cpcardiol.2020.100739
112. Kleinbongard P, Schulz R, Heusch G. TNFα in myocardial ischemia/reperfusion, remodeling and heart failure. Heart Fail Rev. (2011) 16:49–69. doi: 10.1007/s10741-010-9180-8
113. Bugger H, Pfeil K. Mitochondrial ROS in myocardial ischemia reperfusion and remodeling. Biochim Biophys Acta Mol Basis Dis. (2020) 1866:165768. doi: 10.1016/j.bbadis.2020.165768
114. Irwin MW, Mak S, Mann DL, Qu R, Penninger JM, Yan A, et al. Tissue expression and immunolocalization of tumor necrosis factor-alpha in postinfarction dysfunctional myocardium. Circulation. (1999) 99:1492–8. doi: 10.1161/01.cir.99.11.1492
115. Oikonomou E, Leopoulou M, Theofilis P, Antonopoulos AS, Siasos G, Latsios G, et al. A link between inflammation and thrombosis in atherosclerotic cardiovascular diseases: clinical and therapeutic implications. Atherosclerosis. (2020) 309:16–26. doi: 10.1016/j.atherosclerosis.2020.07.027
116. Somasuntharam I, Yehl K, Carroll SL, Maxwell JT, Martinez MD, Che PL, et al. Knockdown of TNF-α by DNAzyme gold nanoparticles as an anti-inflammatory therapy for myocardial infarction. Biomaterials. (2016) 83:12–22. doi: 10.1016/j.biomaterials.2015.12.022
117. Alshawwa SZ, Kassem AA, Farid RM, Mostafa SK, Labib GS. Nanocarrier drug delivery systems: characterization, limitations, future perspectives and implementation of artificial intelligence. Pharmaceutics. (2022) 14:883. doi: 10.3390/pharmaceutics14040883
118. Edgar JY, Wang H. Introduction for design of nanoparticle based drug delivery systems. Curr Pharm Des. (2017) 23:2108–12. doi: 10.2174/1381612822666161025154003
119. Veselov VV, Nosyrev AE, Jicsinszky L, Alyautdin RN, Cravotto G. Targeted delivery methods for anticancer drugs. Cancers. (2022) 14:622. doi: 10.3390/cancers14030622
120. Farjadian F, Ghasemi A, Gohari O, Roointan A, Karimi M, Hamblin MR. Nanopharmaceuticals and nanomedicines currently on the market: challenges and opportunities. Nanomedicine. (2019) 14:93–126. doi: 10.2217/nnm-2018-0120
121. He J, Yu L, Lin X, Liu X, Zhang Y, Yang F, et al. Virus-like particles as nanocarriers for intracellular delivery of biomolecules and compounds. Viruses. (2022) 14:1905. doi: 10.3390/v14091905
122. Mangeot PE, Risson V, Fusil F, Marnef A, Laurent E, Blin J, et al. Genome editing in primary cells and in vivo using viral-derived nanoblades loaded with Cas9-sgRNA ribonucleoproteins. Nat Commun. (2019) 10:45. doi: 10.1038/s41467-018-07845-z
123. Cho CW, Cho YS, Kang BT, Hwang JS, Park SN, Yoon DY. Improvement of gene transfer to cervical cancer cell lines using non-viral agents. Cancer Lett. (2001) 162:75–85. doi: 10.1016/s0304-383500629-7
124. Nooraei S, Bahrulolum H, Hoseini ZS, Katalani C, Hajizade A, Easton AJ, et al. Virus-like particles: preparation, immunogenicity and their roles as nanovaccines and drug nanocarriers. J Nanobiotechnol. (2021) 19:59. doi: 10.1186/s12951-021-00806-7
125. Shen L, Zhou J, Wang Y, Kang N, Ke X, Bi S, et al. Efficient encapsulation of Fe3O4 nanoparticles into genetically engineered hepatitis B core virus-like particles through a specific interaction for potential bioapplications. Small. (2015) 11:1190–6. doi: 10.1002/smll.201401952
126. Segel M, Lash B, Song J, Ladha A, Liu CC, Jin X, et al. Mammalian retrovirus-like protein PEG10 packages its own mRNA and can be pseudotyped for mRNA delivery. Science. (2021) 373:882–9. doi: 10.1126/science.abg6155
127. Farokhzad OC, Langer R. Impact of nanotechnology on drug delivery. ACS Nano. (2009) 3:16–20. doi: 10.1021/nn900002m
128. Allen TM, Cullis PR. Liposomal drug delivery systems: from concept to clinical applications. Adv Drug Deliv Rev. (2013) 65:36–48. doi: 10.1016/j.addr.2012.09.037
129. Sheoran R, Khokra SL, Chawla V, Dureja H. Recent patents, formulation techniques, classification and characterization of liposomes. Recent Pat Nanotechnol. (2019) 13:17–27. doi: 10.2174/1872210513666181127110413
130. Amarandi RM, Ibanescu A, Carasevici E, Marin L, Dragoi B. Liposomal-based formulations: a path from basic research to temozolomide delivery inside glioblastoma tissue. Pharmaceutics. (2022) 14:308. doi: 10.3390/pharmaceutics14020308
131. Xing H, Hwang K, Lu Y. Recent developments of liposomes as nanocarriers for theranostic applications. Theranostics. (2016) 6:1336–52. doi: 10.7150/thno.15464
132. Lu M, Zhao X, Xing H, Xun Z, Yang T, Cai C, et al. Liposome-chaperoned cell-free synthesis for the design of proteoliposomes: Implications for therapeutic delivery. Acta Biomater. (2018) 76:1–20. doi: 10.1016/j.actbio.2018.03.043
133. Dad HA, Gu TW, Zhu AQ, Huang LQ, Peng LH. Plant exosome-like nanovesicles: emerging therapeutics and drug delivery nanoplatforms. Mol Ther. (2021) 29:13–31. doi: 10.1016/j.ymthe.2020.11.030
134. Sun D, Chen J, Wang Y, Ji H, Peng R, Jin L, et al. Advances in refunctionalization of erythrocyte-based nanomedicine for enhancing cancer-targeted drug delivery. Theranostics. (2019) 9:6885–900. doi: 10.7150/thno.36510
135. Barile L, Vassalli G. Exosomes: therapy delivery tools and biomarkers of diseases. Pharmacol Ther. (2017) 174:63–78. doi: 10.1016/j.pharmthera.2017.02.020
136. Elsharkasy OM, Nordin JZ, Hagey DW, de Jong OG, Schiffelers RM, Andaloussi SE, et al. Extracellular vesicles as drug delivery systems: why and how? Adv Drug Deliv Rev. (2020) 159:332–43. doi: 10.1016/j.addr.2020.04.004
137. Rampado R, Caliceti P, Agostini M. Latest advances in biomimetic cell membrane-coated and membrane-derived nanovectors for biomedical applications. Nanomaterials. (2022) 12:1543. doi: 10.3390/nano12091543
138. Yang L, Yang Y, Chen Y, Xu Y, Peng J. Cell-based drug delivery systems and their in vivo fate. Adv Drug Deliv Rev. (2022) 187:114394. doi: 10.1016/j.addr.2022.114394
139. Zhang SQ, Fu Q, Zhang YJ, Pan JX, Zhang L, Zhang ZR, et al. Surface loading of nanoparticles on engineered or natural erythrocytes for prolonged circulation time: strategies and applications. Acta Pharmacol Sin. (2021) 42:1040–54. doi: 10.1038/s41401-020-00606-z
140. Li Y, Raza F, Liu Y, Wei Y, Rong R, Zheng M, et al. Clinical progress and advanced research of red blood cells based drug delivery system. Biomaterials. (2021) 279:121202. doi: 10.1016/j.biomaterials.2021.121202
141. Combes F, Meyer E, Sanders NN. Immune cells as tumor drug delivery vehicles. J Control Release. (2020) 327:70–87. doi: 10.1016/j.jconrel.2020.07.043
142. Wang L, Liu J. Engineered drug-loaded cells and cell derivatives as a delivery platform for cancer immunotherapy. Biomater Sci. (2021) 9:1104–16. doi: 10.1039/d0bm01676d
143. Milman N, Ginini L, Gil Z. Exosomes and their role in tumorigenesis and anticancer drug resistance. Drug Resist Updat. (2019) 45:1–12. doi: 10.1016/j.drup.2019.07.003
144. Dutta B, Barick KC, Hassan PA. Recent advances in active targeting of nanomaterials for anticancer drug delivery. Adv Colloid Interface Sci. (2021) 296:102509. doi: 10.1016/j.cis.2021.102509
145. Chai Z, Ran D, Lu L, Zhan C, Ruan H, Hu X, et al. Ligand-modified cell membrane enables the targeted delivery of drug nanocrystals to glioma. ACS Nano. (2019) 13:5591–601. doi: 10.1021/acsnano.9b00661
146. Tan S, Wu T, Zhang D, Zhang Z. Cell or cell membrane-based drug delivery systems. Theranostics. (2015) 5:863–81. doi: 10.7150/thno.11852
147. Meng X, Wang J, Zhou J, Tian Q, Qie B, Zhou G, et al. Tumor cell membrane-based peptide delivery system targeting the tumor microenvironment for cancer immunotherapy and diagnosis. Acta Biomater. (2021) 127:266–75. doi: 10.1016/j.actbio.2021.03.056
148. Fang RH, Kroll AV, Gao W, Zhang L. Cell membrane coating nanotechnology. Adv Mater. (2018) 30:e1706759. doi: 10.1002/adma.201706759
149. Xia Q, Zhang Y, Li Z, Hou X, Feng N. Red blood cell membrane-camouflaged nanoparticles: a novel drug delivery system for the antitumor application. Acta Pharm Sin B. (2019) 9:675–89. doi: 10.1016/j.apsb.2019.01.011
150. Mehta S, Dumoga S, Malhotra S, Singh N. Comparative analysis of PEG-liposomes and RBCs-derived nanovesicles for anti-tumor therapy. Colloids Surf B Biointerfaces. (2022) 218:112785. doi: 10.1016/j.colsurfb.2022.112785
151. Xiao J, Weng J, Wen F, Ye J. Red blood cell membrane-coated silica nanoparticles codelivering DOX and ICG for effective lung cancer therapy. ACS Omega. (2020) 5:32861–7. doi: 10.1021/acsomega.0c01541
152. Xue J, Zhao Z, Zhang L, Xue L, Shen S, Wen Y, et al. Neutrophil-mediated anticancer drug delivery for suppression of postoperative malignant glioma recurrence. Nat Nanotechnol. (2017) 12:692–700. doi: 10.1038/nnano.2017.54
153. Han L, Jiang C. Evolution of the blood-brain barrier in brain diseases and related systemic nanoscale brain-targeting drug delivery strategies. Acta Pharm Sin B. (2021) 11:2306–25. doi: 10.1016/j.apsb.2020.11.023
154. Thamphiwatana S, Angsantikul P, Escajadillo T, Zhang Q, Olson J, Luk BT, et al. Macrophage-like nanoparticles concurrently absorbing endotoxins and proinflammatory cytokines for sepsis management. Proc Natl Acad Sci U.S.A. (2017) 114:11488–93. doi: 10.1073/pnas.1714267114
155. Xiao Y, Ren C, Chen G, Shang P, Song X, You G, et al. Neutrophil membrane-mimicking nanodecoys with intrinsic anti-inflammatory properties alleviate sepsis-induced acute liver injury and lethality in a mouse endotoxemia model. Mater Today Biol. (2022) 14:100244. doi: 10.1016/j.mtbio.2022.100244
156. Bach JF, Chatenoud L. Organ transplantation and autoimmunity: common mechanisms common therapies. Semin Immunol. (2011) 23:155–6. doi: 10.1016/j.smim.2011.08.001
157. Fisher JD, Acharya AP, Little SR. Micro, and nanoparticle drug delivery systems for preventing allotransplant rejection. Clin Immunol. (2015) 160:24–35. doi: 10.1016/j.clim.2015.04.013
158. Thorp EB, Boada C, Jarbath C, Luo X. Nanoparticle platforms for antigen-specific immune tolerance. Front Immunol. (2020) 11:945. doi: 10.3389/fimmu.2020.00945
159. Giannini D, Antonucci M, Petrelli F, Bilia S, Alunno A, Puxeddu I. One year in review 2020: pathogenesis of rheumatoid arthritis. Clin Exp Rheumatol. (2020) 38:387–97.
160. Li H, Feng Y, Zheng X, Jia M, Mei Z, Wang Y, et al. M2-type exosomes nanoparticles for rheumatoid arthritis therapy via macrophage re-polarization. J Control Release. (2022) 341:16–30. doi: 10.1016/j.jconrel.2021.11.019
161. Chen J, Zeng S, Xue Q, Hong Y, Liu L, Song L, et al. Photoacoustic image-guided biomimetic nanoparticles targeting rheumatoid arthritis. Proc Natl Acad Sci U.S.A. (2022) 119:e2213373119. doi: 10.1073/pnas.2213373119
162. Weiss C, Carriere M, Fusco L, Capua I, Regla-Nava JA, Pasquali M, et al. Toward nanotechnology-enabled approaches against the COVID-19 pandemic. ACS Nano. (2020) 14:6383–406. doi: 10.1021/acsnano.0c03697
163. Pollard CA, Morran MP, Nestor-Kalinoski AL. The COVID-19 pandemic: a global health crisis. Physiol Genom. (2020) 52:549–57. doi: 10.1152/physiolgenomics.00089.2020
164. Zhang Q, Honko A, Zhou J, Gong H, Downs SN, Vasquez JH, et al. Cellular nanosponges inhibit SARS-CoV-2 infectivity. Nano Lett. (2020) 20:5570–4. doi: 10.1021/acs.nanolett.0c02278
165. Zhao D, Liu J, Wang M, Zhang X, Zhou M. Epidemiology of cardiovascular disease in China: current features and implications. Nat Rev Cardiol. (2019) 16:203–12. doi: 10.1038/s41569-018-0119-4
166. Soliman G. Dietary fiber, atherosclerosis, and cardiovascular disease. Nutrients. (2019) 11:1155. doi: 10.3390/nu11051155
167. Park JH, Dehaini D, Zhou J, Holay M, Fang RH, Zhang L. Biomimetic nanoparticle technology for cardiovascular disease detection and treatment. Nanoscale Horiz. (2020) 5:25–42. doi: 10.1039/c9nh00291j
168. Hu C, Luo R, Wang Y. Heart valves cross-linked with erythrocyte membrane drug-loaded nanoparticles as a biomimetic strategy for anti-coagulation, anti-inflammation, anti-calcification, and endothelialization. ACS Appl Mater Interfaces. (2020) 12:41113–26. doi: 10.1021/acsami.0c12688
169. Jiang C, Qi Z, He W, Li Z, Tang Y, Wang Y, et al. Dynamically enhancing plaque targeting via a positive feedback loop using multifunctional biomimetic nanoparticles for plaque regression. J Control Release. (2019) 308:71–85. doi: 10.1016/j.jconrel.2019.07.007
170. Gao C, Huang Q, Liu C, Kwong CH, Yue L, Wan J, et al. Treatment of atherosclerosis by macrophage-biomimetic nanoparticles via targeted pharmacotherapy and sequestration of proinflammatory cytokines. Nat Commun. (2020) 11:2622. doi: 10.1038/s41467-020-16439-7
171. Wang Y, Zhang K, Li T, Maruf A, Qin X, Luo L, et al. Macrophage membrane functionalized biomimetic nanoparticles for targeted anti-atherosclerosis applications. Theranostics. (2021) 11:164–80. doi: 10.7150/thno.47841
172. Boada C, Zinger A, Tsao C, Zhao P, Martinez JO, Hartman K, et al. Rapamycin-loaded biomimetic nanoparticles reverse vascular inflammation. Circ Res. (2020) 126:25–37. doi: 10.1161/CIRCRESAHA.119.315185
173. Song Y, Huang Z, Liu X, Pang Z, Chen J, Yang H, et al. Platelet membrane-coated nanoparticle-mediated targeting delivery of rapamycin blocks atherosclerotic plaque development and stabilizes plaque in apolipoprotein E-deficient (ApoE) mice. Nanomedicine. (2019) 15:13–24. doi: 10.1016/j.nano.2018.08.002
174. Chen W, Schilperoort M, Cao Y, Shi J, Tabas I, Tao W. Macrophage-targeted nanomedicine for the diagnosis and treatment of atherosclerosis. Nat Rev Cardiol. (2022) 19:228–49. doi: 10.1038/s41569-021-00629-x
175. Wei X, Ying M, Dehaini D, Su Y, Kroll AV, Zhou J, et al. Nanoparticle functionalization with platelet membrane enables multifactored biological targeting and detection of atherosclerosis. ACS Nano. (2018) 12:109–16. doi: 10.1021/acsnano.7b07720
176. Su T, Huang K, Ma H, Liang H, Dinh PU, Chen J, et al. Platelet-inspired nanocells for targeted heart repair after ischemia/reperfusion injury. Adv Funct Mater. (2019) 29:1803567. doi: 10.1002/adfm.201803567
177. Jung JH, Fu X, Yang PC. Exosomes generated from iPSC-derivatives: new direction for stem cell therapy in human heart diseases. Circ Res. (2017) 120:407–17. doi: 10.1161/circresaha.116.309307
178. Mentkowski KI, Lang JK. Exosomes engineered to express a cardiomyocyte binding peptide demonstrate improved cardiac retention in vivo. Sci Rep. (2019) 9:10041. doi: 10.1038/s41598-019-46407-1
179. Davidson SM, Andreadou I, Barile L, Birnbaum Y, Cabrera-Fuentes HA, Cohen MV, et al. Circulating blood cells and extracellular vesicles in acute cardioprotection. Cardiovasc Res. (2019) 115:1156–66. doi: 10.1093/cvr/cvy314
180. Huang K, Wen S, Wang W, Zhou J, Huang J, Wang F, et al. Erythrocyte membrane coated nanoparticle-based control releasing hydrogen sulfide system protects ischemic myocardium. Nanomedicine. (2021) 16:465–80. doi: 10.2217/nnm-2020-0404
181. Lin Y, Liu J, Bai R, Shi J, Zhu X, Liu J, et al. Mitochondria-inspired nanoparticles with microenvironment-adapting capacities for on-demand drug delivery after ischemic injury. ACS Nano. (2020) 14:11846–59. doi: 10.1021/acsnano.0c04727
182. Xu J, Zhang Y, Xu J, Liu G, Di C, Zhao X, et al. Engineered nanoplatelets for targeted delivery of plasminogen activators to reverse thrombus in multiple mouse thrombosis models. Adv Mater. (2020) 32:e1905145. doi: 10.1002/adma.201905145
183. Yin R, Yang D, Wu J. Nanoparticle drug- and gene-eluting stents for the prevention and treatment of coronary restenosis. Theranostics. (2014) 4:175–200. doi: 10.7150/thno.7210
184. Wang G, Jin L, Wu X, Dong Y, Li Z. Label-free colorimetric sensor for mercury(II) and DNA on the basis of mercury(II) switched-on the oxidase-mimicking activity of silver nanoclusters. Anal Chim Acta. (2015) 871:1–8. doi: 10.1016/j.aca.2015.02.027
185. Liu Y, Wu H, Li M, Yin J, Nie Z. pH dependent catalytic activities of platinum nanoparticles with respect to the decomposition of hydrogen peroxide and scavenging of superoxide and singlet oxygen. Nanoscale. (2014) 6:11904–10. doi: 10.1039/c4nr03848g
186. Cao G, Jiang X, Zhang H, Croley T, Yin J. Mimicking horseradish peroxidase and oxidase using ruthenium nanomaterials. RSC Adv. (2017) 7:52210–7. doi: 10.1039/C7RA10370K
Keywords: cardiovascular disease, nanomedicine, nanomaterials, theranostics, heart failure
Citation: Gong Y, Liu H, Ke S, Zhuo L and Wang H (2023) Latest advances in biomimetic nanomaterials for diagnosis and treatment of cardiovascular disease. Front. Cardiovasc. Med. 9:1037741. doi: 10.3389/fcvm.2022.1037741
Received: 06 September 2022; Accepted: 09 December 2022;
Published: 04 January 2023.
Edited by:
Sufang Li, Peking University People’s Hospital, ChinaReviewed by:
Dashuai Zhu, University of North Carolina at Chapel Hill, United StatesGan Chen, Institute of Health Service and Transfusion Medicine, China
Copyright © 2023 Gong, Liu, Ke, Zhuo and Wang. This is an open-access article distributed under the terms of the Creative Commons Attribution License (CC BY). The use, distribution or reproduction in other forums is permitted, provided the original author(s) and the copyright owner(s) are credited and that the original publication in this journal is cited, in accordance with accepted academic practice. No use, distribution or reproduction is permitted which does not comply with these terms.
*Correspondence: Haibin Wang, ✉ d2FuZ2hiQGJqdHUuZWR1LmNu; Li Zhuo, ✉ YmVsaW5kYXpobEBhbGl5dW4uY29t