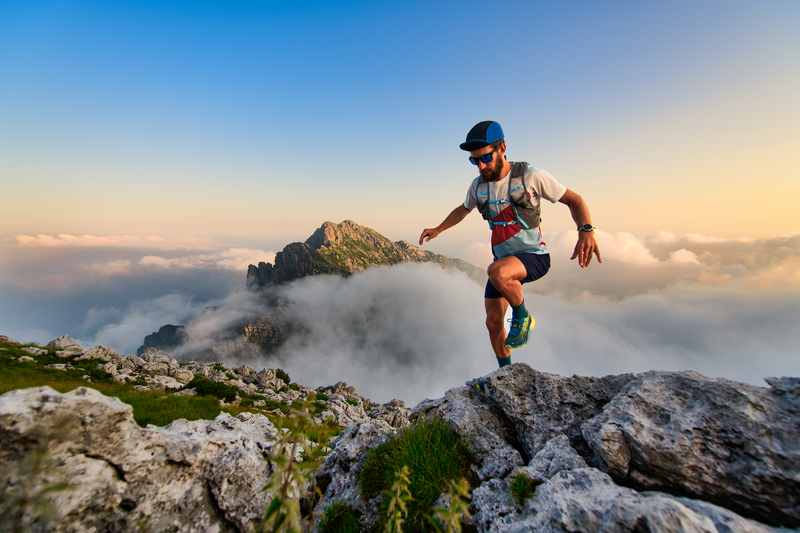
95% of researchers rate our articles as excellent or good
Learn more about the work of our research integrity team to safeguard the quality of each article we publish.
Find out more
ORIGINAL RESEARCH article
Front. Catal. , 30 June 2022
Sec. Biocatalysis
Volume 2 - 2022 | https://doi.org/10.3389/fctls.2022.926316
This article is part of the Research Topic Reductions and Oxidations Catalyzed by Alcohol Dehydrogenases: Useful Biocatalytic Reactions in Organic Synthesis View all 3 articles
Non-enantioselective alcohol dehydrogenases (ADHs) are rarely found in the biocatalysis portfolio. Generally, highly enantioselective ADHs are sought for. Using such ADHs for the oxidation of racemic alcohols generally results in a kinetic resolution of the starting material, which is unfavourable if the ketone represents the product of interest. In the current contribution we report the ADH from Sphingobium yanoikuyae (SyADH) as non-enantioselective ADH for the complete oxidation or rac-heptan-2-ol (representing further 2-alkanols).
Alcohol dehydrogenases are increasingly used as catalysts for the production of fine chemicals and active pharmaceutical intermediates (Huisman et al., 2010; Hollmann et al., 2021). Particularly, enantioselective reduction of prochiral ketones has been in focus during the past decades. Use of ADHs in oxidative direction is far less developed mostly as the oxidation of a sp3-hybridised alcohol carbon to a sp2-hybridised ketone goes hand in hand with the loss of chirality. Therefore, it is not astonishing that most ADH-catalysed oxidation reactions aim at the kinetic resolution of racemic secondary alcohols (Puetz et al., 2020). As a consequence, the scientific literature and the majority of commercially available ADHs deal with highly enantioselective catalysts.
In some cases, however, not the chiral alcohol but rather the ketone represents the desired product (Hochgürtel et al., 2003; Foley and Waldmann, 2022). Heptane-2-one for example, an interesting compound for the flavour industry for its fruity smell and is FDA-listed by as “food additive permitted for direct addition to food for human consumption” (OSHA, 2022). In these cases, highly enantioselective ADHs are not the catalysts of choice as alcohol oxidations mediated by those will yield only 50% of the theoretical yield. To overcome this limitation some chemoenzymatic approaches have been reported (Kedziora et al., 2014; Diaz-Rodriguez et al., 2015; Martinez-Montero et al., 2017; González-Granda et al., 2020). Also, co-application of two enantiocomplementary ADHs may be a practical solution. Most elegantly, however, non-stereoselective ADHs would be useful for the complete oxidation of racemic secondary alcohols to the corresponding ketones. Reports on such ADHs are scarce (Cappaert and Larroche, 2004; Lavandera et al., 2008; Musa et al., 2015; Montgomery et al., 2017). We therefore decided to evaluate the ADH from Sphingobium yanoikuyae (SyADH) (Lavandera et al., 2008) first reported by Kroutil and co-workers as non-enantioselective ADH for the complete oxidation of a range of racemic alcohols such as 2-alkanols or phenyl ethanol derivatives (Lavandera et al., 2008).
Often, ADH-catalysed oxidation reactions are performed using acetone as sacrificial electron acceptor thereby representing a biocatalytic variant of the well-known Oppenauer oxidation (Oppenauer, 1937; Puetz et al., 2020). As in this type of reactions alcohol and ketone starting materials are converted into ketone and alcohol products, respectively, the overall chemical composition of the reactants marginally changes. Consequently, these transformations are plagued by poor thermodynamic driving force and resulting unfavourable equilibria. Shifting the equilibrium by using an excess of acetone is one possibility, which however also generates additional wastes from non-reacted cosubstrate, which is not desirable from an environmental or economic point of view (Bisogno et al., 2010; Ni et al., 2014). We therefore also evaluated if ß-ketoesters may serve as ‘smart cosubstrates’ for the transformation of interest. The resulting ß-hydroxyesters may be expected to be stabilised via intramolecular hydrogen bonds lowering the Gibbs free energy of the coproduct and thereby shifting the equilibrium (Lavandera et al., 2008).
Unless stated otherwise, chemicals, reagents and solvents were purchased from commercial sources (Alfa Aesar, Sigma-Aldrich, Merck) in the highest purity grade available and were used without further purification. Commercial alcohol dehydrogenases (ketoreductases) were supplied by Codexis (Codex® KRED Screening Kit, https://www.codexis-estore.com/product-page/codex-ketoreductase-kred-screening-kit).
The syadh gene was expressed in E. coli BL21 (DE3) or C43 (DE3) from the published vector pEG53-syadh (Lavandera et al., 2008; de Miranda et al., 2015)or the newly designed vector pET24b-syadh (provided by BaseClear B.V., Leiden, Netherlands). In comparison to the literature-described construct, the syadh gene sequence in pET24b-syadh is not codon-optimised for expression in E. coli and encodes the native gene sequence (Gene accession number: EU427523). Additionally, both gene-constructs differ by the C-terminal Strep-tag, which is not present in the newly designed vector (Kulig, 2013). The gene sequence of syadh in pET24b-syadh is provided in the supplementary information.
400 ml buffered TB-medium or autoinduction medium (24 g×L−1 yeast extract, 12 g×L−1 peptone, 15 mg×L−1 d-glucose, 220 mg×L−1 lactose, 6.3 g×L−1 glycerol, 90 mM KPi, pH 7) supplemented with 50 μg/ml kanamycin was inoculated with the recombinant E. coli BL21(DE3) or C43(DE3) strains to an OD600 = 0.05 and grown at 37°C and 180 rpm. When an OD600 of around 1 was reached, expression was induced with 0.1–1.0 mM IPTG (TB-medium cultures) and bacterial cultures were incubated at 20°C and 140 rpm for at least 20 h. Cells were harvested by centrifugation (17,500 × g for 30 min at 4°C), washed with 50 mM potassium phosphate (KPi) buffer pH 7 and stored either in falcon tubes at −20°C or crystallisation bowls at −80°C. Cells stored in crystallisation bowls were lyophilised at 0.1 mbar and −58°C, transferred to a 50 ml falcon tube and then stored at −20°C.
In order to obtain SyADH in form of cell-free extracts, 2–3 g of “wet” non freeze-dried cells were resuspended in around 15 ml 50 mM KPi, pH 7 containing 0.1 mM PMSF (phenylmethylsulfonyl fluoride). Cells were disrupted in 2 consecutive cycles at 1.5 kbar in a high pressure homogenisator (Multi Shot Cell Disruption System). Cell debris was removed by centrifugation (47.850 × g, 30 min, 4°C) and the soluble fraction (cell-free extract) was collected.
ADH-activity was measured in a continuous photometric assay monitoring NADPH formation at 340 nm (ε340 = 6.22 mM−1 cm−1) in presence of rac-heptan-2-ol as substrate. Reaction mixtures contained 50 mM KPi (pH 7), 10 mM substrate in 5% (v/v) DMSO and 50 µL of cell-free extract in appropriate dilution. After incubation for 1 min at 25°C, the reaction was started by adding 0.5 mM NADP+. The increase of absorption caused by NADPH formation was tracked for 120 s at 25°C in a photometer (Cary 60 spectrophotometer, Agilent). The initial slope (ΔA340/min) between 20–60 s was linear and used to calculate the activity, which is expressed in U/gCDW (activity per g cell dry weight) or U/mg (specific activity). 1 U is defined as the amount of enzyme which is needed to convert 1 µmol substrate in 1 min under assay conditions. All measurements were done in triplicates. In order to refer the ADH activity to the cell dry weight (CDW), 3 × 200 μL cell suspension were transferred to 1.5 ml reaction tubes before cell disruption and centrifuged for 2 min at 13.200 × g. The supernatant was discarded and the cells dried at 60°C for at least 48 h before weighing.
The alcohol substrate was dissolved in acetone and added to a reaction mixture of 50 mM potassium phosphate buffer KPi (pH 7) containing either NAD+ or NADP+ and the corresponding ADH (cell-free extracts or lyophilised cells of E. coli C43(DE3) pET24b-syadh). Lyophilised E. coli C43(DE3) pET24d (EV) cells (20 mg × mL−1) were used as a negative control experiment, under otherwise identical conditions. The reaction mixture (total volume of 500 µL) was incubated in a thermostated orbital shaker at 30°C and 600 rpm shaking frequency. The reactions were stopped at different time points by addition of 500 µL ethyl acetate containing 1-decanol (5 mM) as internal standard. The organic layer was separated and analysed by GC-FID. All reactions were performed in technical duplicates.
Lyophilised cells of E. coli C43 (DE3) pET24b-syadh were rehydrated in 1M KPi (pH 7, 10% v/v) containing 1 mM NADP+. Then, rac-heptan-2-ol and ethyl acetoacetate in organic solvent (90% v/v) were added. Reaction mixtures (total volume of 500 µL) were incubated in an orbital shaker at 30°C and 600 rpm shaking frequency. Reactions were stopped at different time points; an aliquot of the organic phase was dissolved in n-heptane containing tridecane (10 mM) as an internal standard and samples were analysed by GC-FID. Experiments were performed in technical duplicates.
Lyophilised E. coli C43 (DE3) pET24b-syadh cells (5 mg×mL−1) were pre-incubated (30°C, 230 rpm) for 72 h in a biphasic system (total volume 10 ml) comprising of 1M KPi (pH 7) and n-heptane in a 1:9 phase ratio. Optionally, rac-heptan-2-ol (100 mM), ethyl acetoacetate (100 mM) or 2-heptanone (100 mM) were supplemented as an additive. After pre-incubation, the remaining components were added (final concentrations of reagents in reaction mixture: rac-heptan-2-ol (100 mM), ethyl acetoacetate (100 mM, 1 equiv.), NADP+ (0.5 mM)) and the reaction mixture was stirred for additional 24 h (30°C, 230 rpm). After 24 h, an aliquot of organic phase was dissolved in n-heptane containing tridecane (10 mM) as an internal standard and samples were analysed by GC-FID.
Achiral GC measurements were performed on a gas chromatograph Shimadzu GC-2014 equipped with flame-ionization detector (FID), a split–splitless injector and the following columns:
CP-Sil 5CB (25 m × 0.25 mm × 1.2 µm, Agilent).
CP-Wax 52 CB (25 m × 0.25 mm × 1.2 µm, Agilent).
Chiral GC analyses were performed on a gas chromatograph Shimadzu GC-2010 plus equipped with flame-ionization detector (FID), a split–splitless injector and a HYDRODEX-β-TBDM column (50 m × 0.25 mm × 0.15 µm, Macherey-Nagel). The absolute configuration of the two heptan-2-ol enantiomers was not determined in course of this study.
In the first set of experiments we evaluated a range of commercially available alcohol dehydrogenases (ADHs) for the non-selective oxidation of rac-heptan-2-ol (Figure 1). The enzyme preparations were used as supplied, supplemented with the appropriate nicotinamide cofactor and incubated in the presence of acetone (10% v/v to drive the ADH-catalysed oxidation reaction). From this selection, no ADH appeared particularly suitable for the purpose of this study as in no case conversion significantly above 50% was observed indicating either very low enzyme activity and/or high enantioselectivity towards one heptan-2-ol enantiomer.
FIGURE 1. Screening of commercial alcohol dehydrogenases for oxidation of rac-heptan-2-ol. Reaction conditions: [rac-heptan-2-ol] = 10 mM, [acetone] = 10% v/v, [NAD+] = 0.5 mM or [NADP+] = 0.5 mM, [ADH] = 1 mg×mL−1, [KPi, pH 7] = 50 mM, total volume: 500 μL, T = 30°C, reaction time: 24 h, 600 rpm. Data are expressed as the mean ± SD of the two independent experiments performed in technical duplicates. ADHs derived from a ketoreductase screening kit from Codexis. For the NAD+-dependent ADHs Rh001, Rh004, Rh014, RhOC and YglC NAD+ was applied as cofactor whereas for the NADP+-dependent ADHs YdlC, YprC, GREc NADP+was used. Since for the ADHs Krh1, Krh2A, Krh2B, Krh4, Krh8, Krh9, Krh10, STAc and SPBc no cofactor preference was provided, a mixture of both NAD+ and NADP+ was used for the screening.
Therefore, we drew our attention to the ADH from Sphingobium yanoikuyae (SyADH) that had previously been introduced by Kroutil and coworkers as a non-selective oxidation catalyst for racemic alcohols(Lavandera et al., 2008).
The expression of the syadh gene in E. coli BL21 (DE3) was performed according to previous literature procedures(Kulig et al., 2012; de Miranda et al., 2015; Montgomery et al., 2017; Younes et al., 2017). Protein production was evaluated by SDS-PAGE (Supplementary Figure S1) and a photometric activity assay, following NADPH-formation in the presence of rac-heptan-2-ol. SDS-PAGE analysis suggested that the protein was not produced in high amounts, resulting also in overall low activities in respective cell-free extracts (0.8 × 10−3 U×mg−1). Consequently, we optimised the expression of the syadh gene by sequentially investigating the influence of the expression host, the gene sequence and the inductor concentration (Supplementary Table S1).
The change of the expression host from E. coli BL21(DE3) to E. coli C43(DE3) had a positive effect on SyADH-activity because a roughly five times higher activity was detected in lysates of E.coli C43(DE3) pEG53-syadh (3.9 × 10−3 U×mg−1). The higher ADH activity in E.coli C43(DE3) compared to BL21(DE3) might be explained by the capability of this strain to be less sensitive towards high amounts of recombinant protein, which is the reason why this strain can be used as alternative for genes that are more difficult to express (Miroux and Walker, 1996). Because the overall amount of target protein in cell lysates was still low (Supplementary Figure S1), we turned our attention to a different gene variant of SyADH, which was expressed from the vector pET24b-syadh. The ADH-construct on this vector does not encode for any additional tags and differs further by its gene sequence from the literature-published construct pEG53-syadh (native instead of codon-optimised gene sequence).
Performing expression studies in E. coli C43(DE3), a protein with the correct molecular weight of SyADH accumulated in samples of E. coli C43(DE3)-pET24b-syadh during SDS-PAGE analysis (Supplementary Figure S2). Activities in respective cell lysates confirmed the identity of SyADH and indicated an activity increase of around 30-fold (0.15 U×mg−1) compared to expression titers reached before. The reasons for the higher production of SyADH using the vector pET24b-syadh such as the use of the native instead of the codon-optimised sequence or the possible interference of the affinity tag in the literature-published construct need to be investigated in future studies. A brief investigation on the influence of different inducer concentrations on the expression efficiency showed only a minor influence. Additionally, we tested the so-called auto-induction medium for SyADH production (Studier, 2005). However, the activity yield was approximately 30% lower than using IPTG induction (Supplementary Table S1), which is why we used IPTG induction for the following experiments.
Having SyADH at hand, we performed some initial experiments oxidising rac-heptan-2-ol with lyophilised cells of E. coli C43 (DE3) pET24b-syadh using acetone as cosubstrate (Figure 2). 10 mM rac-heptan-2-ol was completely oxidised to the desired 2-heptanone (98% yield) within 20 min. Higher starting material concentrations took somewhat longer but eventually gave more than 80% conversion within 24 h. Increasing the cosubstrate concentration (acetone) from 5% (v/v) to 10% (v/v) significantly increased the initial rate.
FIGURE 2. SyADH catalysed oxidation of rac-heptan-2-ol (10, 50 and 100 mM) employing acetone as cosubstrate. Reaction conditions: [rac-heptan-2-ol] = 10–100 mM, [acetone] = 5 or 10% v/v, [NADP+] = 1 mM, [E. coli C43(DE3) pET24b-syadh] = 10 mg × mL−1, [KPi, pH 7] = 50 mM, total volume: 500 μL, T = 30°C, 600 rpm. Data are expressed as the mean ± SD of the two independent experiments performed in technical duplicates.
These results already indicated that SyADH exhibited only low enantioselectivity towards rac-heptan-2-ol, nevertheless, we investigated the course of the oxidation reaction with chiral GC (Figure 3). Indeed, the SyADH-catalysed oxidation of rac-heptan-2-ol proceeded as kinetic resolution with one alcohol enantiomer preferentially being oxidised. The enantioselectivity (E), however was rather low (E = 2.3 ± 0.1) and near-full conversion (96%) of the staring material was attained within 24 h.
FIGURE 3. Stereochemical course of the oxidation of rac-heptan-2-ol catalysed by the SyADH-whole cell catalyst. Reaction conditions: [rac-heptan-2-ol] = 50 mM, [acetone] = 10% v/v, [NADP+] = 1 mM, [E. coli C43(DE3) pET24b-syadh] = 10 mg×mL−1, [KPi, pH 7] = 50 mM, total volume: 500 μL, T = 30°C, 600 rpm. 2-heptanol Ent-1 corresponds to the (R)-enantiomer, 2-heptanol Ent-2 corresponds to the (S)-enantiomer.
Substrate loadings in the range of 100 mM are not very attractive envisioning preparative application of the ADH-oxidation technology. In case of rac-heptan-2-ol this corresponds to a maximal product concentration of 11 g × L−1. Consequently, wastes generated include 10 g×L−1 catalysts, 80 g × L−1 acetone/isopropanol and approximately 900 g × L−1 of waste water. Overall, an E-factor (Sheldon, 2017) around 90 kgwaste ×kg−1product can be estimated. Obviously, this is unattractive both from an environmental (Ni et al., 2014; Holtmann and Hollmann, 2022) and economic (Huisman et al., 2010) point of view. We therefore envisioned two improvements of the current setup to reduce the waste formation: 1) increasing the overall reagent loading and 2) reducing the amount of cosubstrate.
To increase the overall payload of hydrophobic starting materials, the two liquid phase system (2LPS) approach is a promising solution. Here, a water-immiscible organic solvent serves as substrate reservoir from which the staring material partitions into the aqueous, biocatalyst-containing, aqueous phase. The reaction product partitions back into the hydrophobic layer. Overall, despite the poor aqueous solubility of the reagents, high reagent payloads can be achieved (van Schie et al., 2021).
The simplest 2LPS approach utilises the staring material itself as hydrophobic organic phase. Indeed, applying rac-heptan-2-ol as a 1:1 (v/v) organic phase to the SyADH-catalysed oxidation reaction resulted in more than 650 mM (17% conversion). As the separation of the starting material and the product was expected to be difficult, we aimed at other hydrophobic co-solvents as carriers for the starting material to achieve full conversion and facile isolation of the product form the non-reactive organic phase. A range of potential hydrophobic, non-reactive organic phases was tested in a phase ratio of 9:1 (organic phase: aqueous phase) (Figure 4). It should be noted that this selection of water-immiscible solvents is far from being exhaustive and that further research may reveal other, more suitable co-solvents (van Schie et al., 2021).
FIGURE 4. The effect of hydrophobicity of organic solvent (expressed as log p values (■)) on the activity of SyADH-catalysed oxidation of rac-heptan-2-ol in biphasic reaction systems. Reaction conditions: [rac-heptan-2-ol]organic phase = 50 mM, [ethyl acetoacetate]organic phase = 100 mM (2 equiv.), [NADP+]aq = 1 mM, [E. coli C43 (DE3) pET24b-syadh]aq = 10 mg×mL−1, [KPi, pH 7] = 1 M (10% v/v), [organic solvent] = 90% v/v, total volume: 500 μL, T = 30°C, reaction time: 24 h, 600 rpm. Data are expressed as the mean ± SD of the two independent experiments performed in technical duplicates.
In accordance with the empirical logP 4 rule (set up for whole cell catalysis) (Laane et al., 1987; Villela Filho et al., 2003), we generally observed a correlation between the hydrophobicity of the organic phase applied and the performance of the biocatalyst. Because highest conversions were obtained with n-heptane as organic phase, we used this organic solvent for the following experiments.
Another issue of ADH-catalysed redox reactions is their reversibility and often poor thermodynamic driving force (Kroutil et al., 2004; Puetz et al., 2020). The Free Gibbs Energy of the starting materials and products is very similar, resulting in an equilibrium constant of the overall reaction close to 1. As a matter of fact, additional measures to shift the equilibrium towards full oxidation of the target staring material (e.g. rac-heptan-2-ol) are necessary. Most frequently, large molar excesses of the cosubstrate (acetone) are used for this. This, however, also results in large wastes formed and consequently is questionable from an environmental point of view. Kroutil and co-workers pioneered a smart cosubstrate approach by introducing α-haloketones as cosubstrates (Lavandera et al., 2008). The resulting halohydrin products form intramolecular H-bonds thereby stabilising them, reducing the Free Gibbs Energy and shifting the equilibrium towards the product. α-haloketones and the corresponding halohydrins (despite their versatility as building blocks in organic synthesis) exhibit irritant and lachrymatory properties thereby disfavouring their application for the synthesis of food or cosmetics products. We therefore reasoned that ß-ketoesters such ethyl acetoacetate (Peschko and Stohrer, 2007) may have a comparable effect (Scheme 1).
SCHEME 1. Shifting the equilibrium of ADH-catalysed oxidation reactions using smart cosubstrates. Abbreviations as provided by Codexis were used.
Indeed, using ethyl acetoacetate as smart cosubstrate enabled eliminating molar surpluses of the sacrificial cosubstrate. Already using one equivalent gave near-full conversion within 24 h (Figure 5).
FIGURE 5. The influence of substrate/cosubstrate ratio on the activity of SyADH catalysed oxidation of rac-heptan-2-ol in a biphasic reaction system. Reaction conditions: [rac-heptan-2-ol]org = 50 mM, [Ethyl acetoacetate]org = 50, 100 or 150 mM (1–3 equiv.), [NADP+]aq = 1 mM, [E. coli C43(DE3) pET24b-syadh] = 10 mg×mL−1, [KPi, pH 7] = 1 M (10% v/v), [n-heptane] = 90% v/v, total volume: 500 μL, T = 30°C, 600 rpm. Data are expressed as the mean ± SD of the two independent experiments performed in technical duplicates.
Next to this, we also studied the effect of externally added NADP+. Addition of 0.5 or 1 mM of NADP+ to the aqueous phase increased the conversion from 69% (in the absence of additional NADP+) to more than 86% (in the presence of additional NADP+). The increase in conversion might hint that either the NADP+ amount in lyophilised E. coli cells is limited for achieving higher conversions or that during lyophilisation a part of the intracellular NADP+ was degraded, as it was seen in previous studies (Zehentgruber et al., 2010; Hilberath et al., 2021).
After identification of suitable conditions for the cosubstrates ethyl acetoacetate and NADP+, we aimed at increasing the substrate concentration in the organic phase while simultaneously increasing the ethyl acetoacetate concentration (Figure 6). Very much to our surprise, full conversion could only be achieved with the lowest starting material concentration (100 mM) while e.g. with 500 mM starting material the product yield was approximately on 70%.
FIGURE 6. Effect of different substrate concentrations on the SyADH catalysed oxidation of rac-heptan-2-ol in a biphasic reaction system. Reaction conditions: [rac-heptan-2-ol]org = 100–500 mM, [ethyl acetoacetate]org = 100–500 mM (1 equiv.), [NADP+]aq = 1 mM, [E. coli C43(DE3) pET24b-syadh]aq = 5 mg×mL−1, [KPi, pH 7] = 1 M (10% v/v), [heptane] = 90% v/v, total volume: 500 μL, T = 30°C, 600 rpm.
Product inhibition can only partially explain these findings. The initial rate of the SyADH-catalysed oxidation of rac-heptan-2-ol was inhibited by approx. 50% in the presence of the same concentration of 2-heptanone. Consequently, a decrease in the overall reaction rate was expected but not the complete cease of the reaction (Figure 7).
FIGURE 7. The inhibitory effect of 2-heptanone concentration on the initial rate of SyADH-catalysed oxidation of rac-heptan-2-ol. Activity assay conditions: [rac-heptan-2-ol] = 10 mM, [2-heptanone] = 0–10 mM, [NADP+] = 0.5 mM, [SyADH-cell-free extract] = 2.1 U×mL−1 (100% relative activity), [KPi, pH 7] = 50 mM, [DMSO] = 5% v/v, incubation time = 1 min, T = 25°C total volume: 1000 µL. Data are expressed as the mean ± SD of the three independent experiments.
Suspiciously, all reactions shown in Figure 6 stopped at approximately the same time (ca. 72 h). We suspected that one of the reaction components may have an inactivating effect on the biocatalyst. Hence, we separately examined the influence of each component in the reaction mixture on the stability of enzyme (Figure 8). After 72 h incubation of SyADH whole cell-biocatalyst in the presence of either n-heptane alone, 2-heptanone or ethyl acetoacetate (dissolved in n-heptane each) the enzyme activity was not significantly impaired. rac-Heptan-2-ol, however, decreased the enzyme stability considerably.
FIGURE 8. Effect of pre-incubation of the SyADH whole cell-biocatalyst in the presence of different reagents on its catalytic activity. Lyophilised cells were incubated for 72 h in (■) n-heptane, (●) rac-heptan-2-ol (in n-heptane), (∆) ethyl acetoacetate (in n-heptane) and (♦) 2-heptanone (in n-heptane), then the remaining components were added. Reaction conditions: [rac-heptan-2-ol] = 100 mM, [Ethyl acetoacetate] = 100 mM (1 equiv.), [NADP+] = 0.5 mM, [E. coli C43(DE3) pET24b-syadh] = 5 mg×mL−1, [KPi, pH 7] = 1 M (10% v/v), [n-heptane] = 90% v/v, total volume: 10 ml, T = 30°C, 230 rpm.
Today, we are lacking a satisfactory explanation for this inhibition/inactivation of SyADH by the starting material rac-heptan-2-ol and further detailed studies will be necessary to shed light on this phenomenon. Limiting the substrate concentration, however, appeared to be a practical approach to increase the life time of SyADH. To test this hypothesis, we performed two experiments limiting the concentration of rac-heptan-2-ol in the reaction mixture. First, a fed-batch-type biotransformation was performed in which rac-heptan-2-ol was added periodically to the reaction mixture (Figure 9). In the second experiment, the organic phase was replaced (as much as possible) after each reaction cycle (Figure 10). In both experiments, the activity of SyADH could be prolonged very significantly for at least 4 days (in case of the fed-batch experiment) or 6 days (in case of replacing the organic phase). In the latter case, the initial rate of rac-heptan-2-ol oxidation slightly decreased from 1.67 mM × h−1 in the first cycle to 1.3 mM×h−1 in the 5th cycle after 7 days.
FIGURE 9. Fed-batch transformation of rac-heptan-2-ol. Reaction conditions: [rac-heptan-2-ol]org = [ethyl acetoacetate]org: added (50 mM each) at t = 0, 22.5, 48, 72, 96 and 120 h, [NADP+]aq = 1 mM, [E. coli C43(DE3) pET24b-syadh]aq = 5 mg×mL−1, [KPi, pH 7] = 1 M (10% v/v), [n-heptane] = 90% v/v, total volume: 500 μL, T = 30°C, 600 rpm.
FIGURE 10. Effect of replacing the organic layer on the SyADH catalysed oxidation of rac-heptan-2-ol in a biphasic reaction system. Reaction conditions: [rac-heptan-2-ol]org = 50 mM, [ethyl acetoacetate]org = 50 mM (1 equiv.), the organic phase was replaced at t = 47, 72, 96 and 135 h, [NADP+]aq = 1 mM, [E. coli C43(DE3) pET24b-syadh]aq = 5 mg×mL−1, [KPi, pH 7] = 1 M (10% v/v), [n-heptane] = 90% v/v, total volume: 500 μL, T = 30°C, 600 rpm.
Overall, these experiments demonstrate that controlling the starting material concentration is an efficient handle to improve SyADH activity.
Overall, in this study we have characterised the ADH from Sphingobium yanoikuyae (SyADH) as non-enantioselective catalyst for the oxidation of a racemic secondary alcohol such as rac-heptan-2-ol. The poor enantioselectivity of SyADH makes it an attractive catalyst if complete transformations of racemic alcohols into the corresponding methyl ketones is desired. Substrate inhibition represents an issue en route to practical application, which, however, can possibly be addressed with a fed-batch strategy.
The raw data supporting the conclusion of this article will be made available by the authors, without undue reservation.
EP designed and carried out most experiments and analysed all the data. TH performed and evaluated experiments with regard to construction of expression vectors, gene expression and activity measurements. KV and FH guided this study and gave advices in the research work. All authors drafted the manuscript and revised the manuscript. All authors read and approved the final manuscript.
The authors declare that the research was conducted in the absence of any commercial or financial relationships that could be construed as a potential conflict of interest.
The handling editor CM declared a past co-authorship with the author FH.
All claims expressed in this article are solely those of the authors and do not necessarily represent those of their affiliated organizations, or those of the publisher, the editors and the reviewers. Any product that may be evaluated in this article, or claim that may be made by its manufacturer, is not guaranteed or endorsed by the publisher.
This research was supported by the Slovak Research and Development Agency under the contract No. APVV-17-0109.
The Supplementary Material for this article can be found online at: https://www.frontiersin.org/articles/10.3389/fctls.2022.926316/full#supplementary-material
Bisogno, F. R., García-Urdiales, E., Valdés, H., Lavandera, I., Kroutil, W., Suárez, D., et al. (2010). Ketone-Alcohol Hydrogen-Transfer Equilibria: Is the Biooxidation of Halohydrins Blocked? Chem. Eur. J. 16 (36), 11012–11019. doi:10.1002/chem.201001233
Cappaert, L., and Larroche, C. (2004). Oxidation of a Mixture of 2-(R) and 2-(S)-heptanol to 2-heptanone bySaccharomyces Cerevisiaein a Biphasic System. Biocatal. and Biotransformation 22 (4), 291–296. doi:10.1080/10242420400011992
de Miranda, A. S., Simon, R. C., Grischek, B., de Paula, G. C., Horta, B. A. C., de Miranda, L. S. M., et al. (2015). Chiral Chlorohydrins from the Biocatalyzed Reduction of Chloroketones: Chiral Building Blocks for Antiretroviral Drugs. ChemCatChem 7 (6), 984–992. doi:10.1002/cctc.201403023
Diaz-Rodríguez, A., Ríos-Lombardía, N., Sattler, J. H., Lavandera, I., Gotor-Fernández, V., Kroutil, W., et al. (2015). Deracemisation of Profenol Core by Combining laccase/TEMPO-Mediated Oxidation and Alcohol Dehydrogenase-Catalysed Dynamic Kinetic Resolution. Catal. Sci. Technol. 5 (3), 1443–1446. doi:10.1039/C4CY01351D
Foley, D. J., and Waldmann, H. (2022). Ketones as Strategic Building Blocks for the Synthesis of Natural Product-Inspired Compounds. Chem. Soc. Rev 51, 1094–4120. doi:10.1039/D2CS00101B
González-Granda, S., Méndez‐Sánchez, D., Lavandera, I., and Gotor‐Fernández, V. (2020). Laccase‐mediated Oxidations of Propargylic Alcohols. Application in the Deracemization of 1‐arylprop‐2‐yn‐1‐ols in Combination with Alcohol Dehydrogenases. ChemCatChem 12 (2), 520–527. doi:10.1002/cctc.201901543
Hilberath, T., Raffaele, A., Windeln, L. M., and Urlacher, V. B. (2021). Evaluation of P450 Monooxygenase Activity in Lyophilized Recombinant E. coli Cells Compared to Resting Cells. Amb. Expr. 11 (1), 162. doi:10.1186/s13568-021-01319-0
Hochgürtel, M., Biesinger, R., Kroth, H., Piecha, D., Hofmann, M. W., Krause, S., et al. (2003). Ketones as Building Blocks for Dynamic Combinatorial Libraries: Highly Active Neuraminidase Inhibitors Generated via Selection Pressure of the Biological Target. J. Med. Chem. 46 (3), 356–358. doi:10.1021/jm025589m
Hollmann, F., Opperman, D. J., and Paul, C. E. (2021). Biocatalytic Reduction Reactions from a Chemist's Perspective. Angew. Chem. Int. Ed. 60 (11), 5644–5665. doi:10.1002/anie.202001876
Holtmann, D., and Hollmann, F. (2022). Is Water the Best Solvent for Biocatalysis? Mol. Catal. 517, 112035. doi:10.1016/j.mcat.2021.112035
Huisman, G. W., Liang, J., and Krebber, A. (2010). Practical Chiral Alcohol Manufacture Using Ketoreductases. Curr. Opin. in Chem. Biol. 14 (2), 122–129. doi:10.1016/j.cbpa.2009.12.003
Kędziora, K., Díaz-Rodríguez, A., Lavandera, I., Gotor-Fernández, V., and Gotor, V. (2014). Laccase/TEMPO-mediated System for the Thermodynamically Disfavored Oxidation of 2,2-Dihalo-1-Phenylethanol Derivatives. Green Chem. 16 (5), 2448–2453. doi:10.1039/c4gc00066h
Kroutil, W., Mang, H., Edegger, K., and Faber, K. (2004). Recent Advances in the Biocatalytic Reduction of Ketones and Oxidation of Sec -alcohols. Curr. Opin. Chem. Biol. 8 (2), 120–126. doi:10.1016/j.cbpa.2004.02.005
Kulig, J., Simon, R. C., Rose, C. A., Husain, S. M., Häckh, M., Lüdeke, S., et al. (2012). Stereoselective Synthesis of Bulky 1,2-diols with Alcohol Dehydrogenases. Catal. Sci. Technol. 2 (8), 1580–1589. doi:10.1039/C2CY20120H
Kulig, J. (2013). Stereoselective Synthesis of Vicinal Diols with Enzymatic Cascade Reactions. Dusseldorf, Germany: Thesis Heinrich Heine University.
Laane, C., Boeren, S., Vos, K., and Veeger, C. (1987). Rules for Optimization of Biocatalysis in Organic Solvents. Biotechnol. Bioeng. 30 (1), 81–87. doi:10.1002/bit.260300112
Lavandera, I., Kern, A., Resch, V., Ferreira-Silva, B., Glieder, A., Fabian, W. M. F., et al. (2008). One-Way Biohydrogen Transfer for Oxidation of Sec-Alcohols. Org. Lett. 10 (11), 2155–2158. doi:10.1021/ol800549f
Martínez-Montero, L., Gotor, V., Gotor-Fernández, V., and Lavandera, I. (2017). Stereoselective Amination of Racemic Sec-Alcohols through Sequential Application of Laccases and Transaminases. Green Chem. 19 (2), 474–480. doi:10.1039/c6gc01981a
Miroux, B., and Walker, J. E. (1996). Over-production of Proteins inEscherichia Coli: Mutant Hosts that Allow Synthesis of Some Membrane Proteins and Globular Proteins at High Levels. J. of Mol. Biol. 260 (3), 289–298. doi:10.1006/jmbi.1996.0399
Montgomery, S. L., Mangas-Sanchez, J., Thompson, M. P., Aleku, G. A., Dominguez, B., and Turner, N. J. (2017). Direct Alkylation of Amines with Primary and Secondary Alcohols through Biocatalytic Hydrogen Borrowing. Angew. Chem. Int. Ed. 56 (35), 10491–10494. doi:10.1002/anie.201705848
Musa, M. M., Patel, J. M., Nealon, C. M., Kim, C. S., Phillips, R. S., and Karume, I. (2015). Thermoanaerobacter Ethanolicus Secondary Alcohol Dehydrogenase Mutants with Improved Racemization Activity. J. of Mol. Catal. B Enzym. 115, 155–159. doi:10.1016/j.molcatb.2015.02.012
Ni, Y., Holtmann, D., and Hollmann, F. (2014). How Green Is Biocatalysis? to Calculate Is to Know. ChemCatChem 6 (4), 930–943. doi:10.1002/cctc.201300976
Oppenauer, R. V. (1937). Eine Methode der Dehydrierung von Sekundären Alkoholen zu Ketonen. I. Zur Herstellung von Sterinketonen und Sexualhormonen. Recl. Trav. Chim. Pays-Bas 56 (2), 137–144. doi:10.1002/recl.19370560206
OSHA (2022). OSHA Occupational Chemical Database. [Online]. Available at: https://www.osha.gov/chemicaldata (Accessed May 10th, 2022).
Peschko, C., and Stohrer, J. (2007). Verfahren zur Oxidation von sekundären Alkoholen durch Enzyme. DE102006009743A1.
Puetz, H., Puchľová, E., Vranková, K., and Hollmann, F. (2020). Biocatalytic Oxidation of Alcohols. Catalysts 10 (9), 952. doi:10.3390/catal10090952
Sheldon, R. A. (2017). The E-Factor 25 Years on: the Rise of Green Chemistry and Sustainability. Green Chem. 19 (1), 18–43. doi:10.1039/C6GC02157C
Studier, F. W. (2005). Protein Production by Auto-Induction in High-Density Shaking Cultures. Protein Expr. and Purif. 41 (1), 207–234. doi:10.1016/j.pep.2005.01.016
van Schie, M. M. C. H., Spöring, J.-D., Bocola, M., Domínguez de María, P., and Rother, D. (2021). Applied Biocatalysis beyond Just Buffers - from Aqueous to Unconventional Media. Options and Guidelines. Green Chem. 23, 3191–3206. doi:10.1039/D1GC00561H
Villela Filho, M., Stillger, T., Müller, M., Liese, A., and Wandrey, C. (2003). Is Log P a Convenient Criterion to Guide the Choice of Solvents for Biphasic Enzymatic Reactions? Angew. Chem. Int. Ed. 42 (26), 2993–2996. doi:10.1002/anie.200351089
Younes, S. H. H., Ni, Y., Schmidt, S., Kroutil, W., and Hollmann, F. (2017). Alcohol Dehydrogenases Catalyze the Reduction of Thioesters. ChemCatChem 9, 1389–1392. doi:10.1002/cctc.201700165
Keywords: alcohol dehydrogenase, deracemisation reactions, oxidation reactions, biocatalysis, Sphingobium yanoikuyae
Citation: Puchľová E, Hilberath T, Vranková K and Hollmann F (2022) Towards Biocatalytic Oxidation of Secondary Alcohols to Carbonyl Products of Relevance for Flavors and Fragrances. Front. Catal. 2:926316. doi: 10.3389/fctls.2022.926316
Received: 22 April 2022; Accepted: 16 May 2022;
Published: 30 June 2022.
Edited by:
Cintia Duarte de Freitas Milagre, Universidade Estadual Paulista, BrazilReviewed by:
Vicente Gotor-Fernández, University of Oviedo, SpainCopyright © 2022 Puchľová, Hilberath, Vranková and Hollmann. This is an open-access article distributed under the terms of the Creative Commons Attribution License (CC BY). The use, distribution or reproduction in other forums is permitted, provided the original author(s) and the copyright owner(s) are credited and that the original publication in this journal is cited, in accordance with accepted academic practice. No use, distribution or reproduction is permitted which does not comply with these terms.
*Correspondence: Kvetoslava Vranková, a3ZldGthLnZyYW5rb3ZhQGF4eGVuY2Uuc2s=; Frank Hollmann, Zi5ob2xsbWFubkB0dWRlbGZ0Lm5s
Disclaimer: All claims expressed in this article are solely those of the authors and do not necessarily represent those of their affiliated organizations, or those of the publisher, the editors and the reviewers. Any product that may be evaluated in this article or claim that may be made by its manufacturer is not guaranteed or endorsed by the publisher.
Research integrity at Frontiers
Learn more about the work of our research integrity team to safeguard the quality of each article we publish.