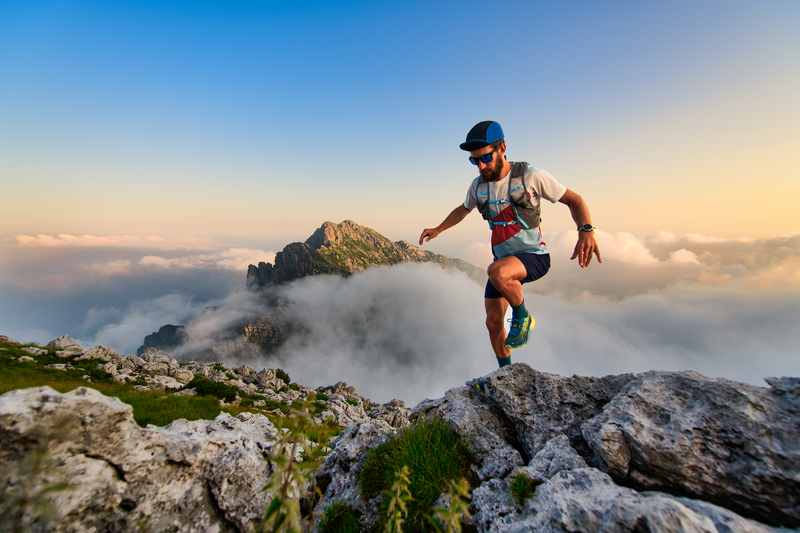
95% of researchers rate our articles as excellent or good
Learn more about the work of our research integrity team to safeguard the quality of each article we publish.
Find out more
REVIEW article
Front. Control Eng. , 11 October 2024
Sec. Control and Automation Systems
Volume 5 - 2024 | https://doi.org/10.3389/fcteg.2024.1452442
This article is part of the Research Topic Advances in Vagus Nerve Stimulation: Biophysiological Modeling and Organ-Specific Therapeutic Strategies View all articles
Targeted plasticity therapy (TPT) utilizes vagus nerve stimulation (VNS) to promote improvements in function following neurological injury and disease. During TPT, a brief burst of VNS induces neuromodulator release, which when paired with relevant behavioral events can influence functionally relevant neuroplasticity. Functional improvements following TPT are therefore in part mediated by neuromodulator signaling. Unfortunately, comorbidities associated with neurological disease often result in altered cognitive states that can influence neuromodulator signaling, potentially impeding neuroplasticity induced by TPT. Aside from altered cognitive states, cardiorespiratory rhythms also affect neuromodulator signaling, due to the vagus nerve’s role in relaying visceral sensory information from the cardiovascular and respiratory systems. Moreover, precise VNS delivery during specific periods of the cardiorespiratory rhythms may further improve TPT. Ultimately, understanding the impact of patient-specific states on neuromodulator signaling may likely facilitate optimized VNS delivery, paving the way for personalized neuromodulation during TPT. Overall, this review explores challenges and considerations for developing advanced TPT paradigms, focusing on altered cognitive states and cardiorespiratory rhythms. We specifically discuss the possible impact of these cognitive states and autonomic rhythms on neuromodulator signaling and subsequent neuroplasticity. Altered cognitive states (arousal deficits or pain) could affect VNS intensity, while cardiorespiratory rhythms may further inform optimized timing of VNS. We propose that understanding these interactions will lead to the development of personalized state dependent VNS paradigms for TPT.
Vagus nerve activity influences central neuromodulator signaling via connections with subcortical structures regulating the release of acetylcholine, norepinephrine, serotonin, and dopamine (Rodenkirch et al., 2022; Hays et al., 2013). This influence impacts synaptic transmission, neuronal excitability, and network activity throughout the nervous system (Lee and Dan, 2012; Brzosko et al., 2019). VNS can activate these neuroanatomical pathways, electrically facilitating neuromodulator release and neuroplasticity. TPT leverages these processes by pairing relevant experiences with VNS in a temporally precise manner, further enhancing activity-dependent neuroplasticity in the central nervous system (Hays, 2016). TPT has been successfully employed across a variety of applications, including stroke (Dawson et al., 2021; Meyers et al., 2018; Badran et al., 2023; Redgrave et al., 2018; Francisco et al., 2023; Khodaparast et al., 2014; Hays et al., 2014), spinal cord injury (Ganzer et al., 2018; Darrow et al., 2020), peripheral nerve injury (Meyers et al., 2019; Darrow et al., 2021; Ruiz et al., 2023a), tinnitus (Engineer et al., 2011; Borland et al., 2016), post-traumatic stress disorder (Noble et al., 2019), as well as learning and memory (Bowles et al., 2022; Altidor et al., 2021; Olsen et al., 2023).
Importantly, the vagus nerve is primarily a conduit for sensory information from end organs to subcortical and cortical areas, facilitating the processing and integration of information related to autonomic function. At the cervical level, ∼80% of its fibers are afferent and relay information from organs to the brain, while the remaining ∼20% are efferent fibers dedicated to organ control. Given these complex interactions between the organs, the vagus nerve, and the central nervous system, at least two major factors emerge that significantly affect overall neuromodulator signaling: 1) altered cognitive states and 2) ongoing autonomic rhythms. While developments in VNS have led to FDA-approved therapies to treat epilepsy, obesity, stroke, and depression, the responder rates in clinical trials have varied significantly compared to preclinical studies (Dickie et al., 2019; Dawson et al., 2021; Morris et al., 1999; Tyler et al., 2017). This could, in part, be due to variability in patient-specific physiology impacting VNS-induced neuromodulator release.
Recent developments in bioelectronic medicines have interestingly begun using stimulation paradigms based on specific physiological states, cardiorespiratory rhythms, and altered cognitive states, representing a possible avenue for optimizing VNS paradigms and TPT. Studies are now exploring the use of closed-loop adaptive neuromodulation systems to optimally deliver stimulation based on the sleep-wake cycle in patients with Parkinson’s disease (Gilron et al., 2021). Algorithms that consider patient-specific circadian rhythms are also now being utilized to optimize adaptive stimulation systems for treating epilepsy (Fleming et al., 2022). Brain-computer interfaces rely on neural signals to decode intent and re-establish control of the impaired end effector (Ethier et al., 2015). These neural signals are often influenced by altered states, such as shifts in attention or arousal (Hennig et al., 2021), motivation (Kleih-Dahms et al., 2021), and neuropathic pain (Vuckovic et al., 2015). Therefore, identifying the influence of non-motor cognitive states in neural decoding could further improve the performance of BCI-FES systems for movement control (Gallego et al., 2022). Moreover, regulating arousal levels via neurofeedback may also improve BCI performance, highlighting the importance of cognitive states (Faller et al., 2019). Overall, evidence suggests that personalized optimization of stimulus programming in neuromodulation systems can importantly improve patient outcomes (Moro et al., 2006). For example, a recent clinical study found that personalized chronic adaptive deep brain stimulation (DBS) outperformed conventional DBS for improving motor symptoms (Oehrn et al., 2024). Optimizing stimulus programming has also been useful in managing speech impairments following Parkinson’s disease (Swinnen et al., 2024). Furthermore, appropriately timed stimulation synchronized with physiological states affects both functional outcome and neuroplasticity. For example, phase specific thalamic DBS can suppress essential tremor significantly better than traditional high-frequency thalamic DBS (Cagnan et al., 2017). Phase-specific stimulation synchronous with cortical beta oscillations can also lead to bidirectional synaptic plasticity (Zanos et al., 2018), similar to studies using electroencephalography (EEG) guided transcranial magnetic stimulation for learning and memory (Taylor et al., 2008) or movement disorders (Wendt et al., 2022). Recent studies are now using closed-loop neuromodulation approaches for individuals with treatment-resistant depression, utilizing recordings from the amygdala and spectral power in the gamma frequency as an indicator of their cognitive state (Scangos et al., 2021). Lastly, cardiovascular rhythms and state dependent stimulation is now being incorporated into adaptive cardiac pacemakers that adjust stimulation based on exertional state and demand (Świerżyńska et al., 2023).
Taken together, these studies outline a framework for possibly developing a state dependent VNS paradigm for TPT to maximize plasticity and function recovery, considering the influence of altered cognitive states and cardiorespiratory rhythms. Patient-specific cognitive states, such as arousal and pain perception changes, could ultimately influence VNS parameter selection. In addition, cardiorespiratory rhythms may significantly impact the VNS timing needed for optimized TPT. Overall, this review aims to summarize and discuss the influence of altered cognitive states and cardiorespiratory rhythms on neuromodulator release, potentially impacting neuroplasticity associated with TPT. The content throughout aims to be hypothesis generating, hopefully paving the way for the development of personalized VNS and optimized TPT.
VNS promotes neuromodulator release that significantly affects neuronal excitability, enhancing signal-to-noise ratios and salient events, as well as influencing synaptic modifications in the brain, thereby regulating several aspects of neuroplasticity (Pedrosa and Clopath, 2017; Hirata et al., 2006). Neuroplasticity represents a critical substrate of learning and memory (Serradj et al., 2023), recovery of motor function after neurological injury (Nandakumar et al., 2023; Mohammed and Hollis 2018; Ward et al., 2003), and several other processes. Subcortical structures, namely, the nucleus basalis of Meynert (NB), the locus coeruleus (LC), the dorsal raphe nucleus (DRN), and the ventral tegmental area (VTA), serve as principal sources of key neuromodulators involved in neuroplasticity, including acetylcholine, norepinephrine, serotonin, and dopamine respectively (for an extensive review refer to (Gu, 2002)). Dysregulation of these neuromodulators has been associated with deficits in learning, impaired memory consolidation, and behavioral dysfunction following neurological injury (Li and Hollis, 2023; Ramanathan et al., 2015; Conner et al., 2010; Monk and Hussain Shuler, 2019; Wachtel et al., 1975).
VNS engages these neuromodulatory transmission systems via the nucleus tractus solitarius (NTS) in the brainstem, subsequently promoting neuronal activation in the NB (Bowles et al., 2022; Collins et al., 2021; Mridha et al., 2021), the LC (Hulsey et al., 2016b), the DRN (Manta et al., 2012; 2013; Dorr and Guy, 2006), and the VTA (Brougher et al., 2021; Fernandes et al., 2020) (Figure 1A). Even a brief burst of VNS releases neuromodulators diffusely throughout the cortex, causing widespread changes in cortical excitability (Collins et al., 2021). Moreover, this neuromodulator release triggered by VNS is essential for TPT-induced neuroplasticity (Kilgard and Merzenich, 1998; Hulsey et al., 2016a; Ramanathan et al., 2009). A reduction in neuromodulator signaling impairs TPT-induced neuroplasticity, while enhancement facilitates neuroplasticity and behavioral outcomes (Hulsey et al., 2016a; Hulsey et al., 2019; Nichols et al., 2011; Meyers et al., 2019; Bowles et al., 2022).
Figure 1. Mechanisms of TPT-induced neuroplasticity mediated by neuromodulator signaling. (A) TPT commonly involves implanting a VNS cuff (iVNS) at the cervical level along with an implantable pulse generator (IPG), stimulating the ear non-invasively targeting the auricular branch of the vagus (TaVNS), or stimulating the neck non-invasively targeting the cervical vagus (TcVNS). Stimulating the vagus nerve and its branches can activate the nucleus tractus solitarius (NTS) in the brainstem, which has extensive projections to other key brainstem nuclei facilitating neuromodulator release, including the locus coeruleus (LC) for norepinephrine release, the nucleus basalis of Meynert (NB) for acetylcholine release, the dorsal raphe nucleus (DRN) for serotonin release, and the ventral tegmental area (VTA) for dopamine release. Overall, VNS leads to the release of these neuromodulators across multiple brain areas. The vagus nerve also transmits information from the cardiovascular and respiratory tissues to the NTS and higher brain order structures, thereby possibly affecting VNS-induced neuromodulator signaling. (B) TPT for upper limb rehabilitation commonly uses a brief burst of iVNS (0.8 mA, 30 Hz, 100 µs pulse width, and a 0.5 s train duration) that can be paired with successful movements to improve motor performance (e.g., where the achieved force exceeds a given threshold). (C) Coincident activity of presynaptic and postsynaptic neurons during rehabilitation creates a transient synaptic tag, commonly referred to as the synaptic eligibility trace. These traces can be converted to long-term changes in synaptic strength mediated by VNS-induced neuromodulator release local to the synapse (please note the specific time scales in panel C; red dashed lines (B, C): VNS thresholds during successful force events).
TPT commonly uses a brief burst of VNS (e.g., 0.5 s in duration). Importantly, these brief bursts of stimulation alone are insufficient to strengthen synapses and facilitate TPT-induced neuroplasticity, as they must be paired with a given event in a temporally precise manner to promote enhanced function (Hays, 2016; Hays et al., 2014). Aside from TPT, other applications of VNS commonly use longer stimulation trains that can facilitate some degree of neuroplasticity (Furmaga et al., 2012; Biggio et al., 2009; Olsen et al., 2022). Typical plasticity mechanisms follow Hebbian rules requiring the coincident activity of presynaptic and postsynaptic neurons, with the temporal differences in spiking dictating synaptic strengthening via long-term potentiation (LTP) or weakening via long-term depression (LTD), known as spike timing dependent plasticity (STDP) (Urbin et al., 2017; Kleim and Jones, 2008; Ziemann et al., 2004). These synaptic modifications are often transient, and the rules of STDP can be dramatically influenced by the presence of neuromodulators (Brzosko et al., 2019; Pawlak et al., 2010; Gerstner et al., 2018). Recent studies suggest that most synaptic plasticity is heterotypic, involving the reinforcement of neuromodulators seconds after Hebbian conditioning leveraging the synaptic eligibility trace (He et al., 2015; Bailey et al., 2000; Froemke et al., 2007) (Figures 1B,C). Neuromodulator release associated with TPT generally acts as a reinforcer (Bowles et al., 2022) when paired with behavioral events and modifies the STDP window further affecting neuroplasticity (Hays et al., 2013). There are a number of possible behavioral events for triggering VNS during TPT, ultimately depending on the therapeutic application (e.g., VNS paired with a motor event during upper limb rehabilitation, VNS paired with a somatosensory event during tactile rehabilitation, or VNS paired with an auditory event during therapy for tinnitus).
Importantly, VNS activates the NTS, a critical structure receiving ongoing neural signaling from the visceral tissues (e.g., neural signals from the cardiovascular and respiratory systems). Hence, this visceral input likely affects VNS-induced neuronal transmission within the NTS (Beaumont et al., 2017) and subsequent downstream neuromodulator release. Overall, these intricate brain-body interactions and the role of visceral input affecting TPT-induced neuroplasticity are incompletely understood. This emphasizes the need for further inquiry, particularly given the vagus nerve’s connection to the viscera, the brainstem, and higher-order structures.
The stimulation dose is a critical concept when considering neuromodulator release and overall neuromodulatory signaling. The stimulation dose for VNS involves a number of factors governing the amount of electrical energy being applied to the vagus (Peterchev et al., 2012). Stimulation dose can be adjusted by varying the amplitude of stimulation, location, modality of stimulation, waveform characteristics (pulse width, frequency, or inter-trial interval), stimulation timing, and the overall therapy duration. Overall, TPT has used several VNS approaches, including invasive VNS at the cervical level using an implantable cuff (iVNS) (Khodaparast et al., 2014; Borland et al., 2016; Noble et al., 2017; Ganzer et al., 2018; Meyers et al., 2018; Meyers et al., 2019; Darrow et al., 2020; Tseng et al., 2020; Dawson et al., 2021), noninvasive trans-auricular stimulation (TaVNS) targeting the auricular branch of the vagus nerve located on the ear (Redgrave et al., 2018; Badran et al., 2023; Chen et al., 2023; Rufener et al., 2023; Thompson et al., 2021), and transcutaneous VNS at the cervical level (TcVNS) (Gurel et al., 2020; Jigo et al., 2024; McIntire et al., 2021). Each stimulation approach could differentially engage important neuromodulatory systems and hence likely requires unique stimulation dosing strategies. iVNS, TaVNS, and TcVNS are exclusively used in this section to better differentiate the various VNS approaches.
Parametric characterization of the optimal stimulation dose for TPT has been most extensively studied in animal models using iVNS. Increasing the stimulus intensity and pulse width leads to increased activity in the noradrenergic LC (Hulsey et al., 2016a), as well as increased cholinergic transmission from NB to the cortex (Mridha et al., 2021). However, increasing the pulse frequency alters the timing, but not the total amount, of VNS-driven neuronal activity in the LC (Hulsey et al., 2016a) and cholinergic activity from NB (Mridha et al., 2021). Moreover, extensive characterization of plasticity as a function of iVNS dose has revealed a non-monotonic inverted U relationship between frequency, stimulus intensity, and plasticity across several preclinical TPT studies (Hays et al., 2023; Borland et al., 2016; Buell et al., 2019; Souza et al., 2021b; Hays et al., 2023; Loerwald et al., 2018). Additionally, the number of overall VNS event pairings (Hays et al., 2014), as well as the interval between VNS event pairings within sessions (Borland et al., 2018) and across sessions/weeks (Ruiz et al., 2023b), influences neuroplasticity and recovery. An optimal stimulation dose, usually consisting of a specific set of VNS parameters (0.8 mA, 30 Hz, 100 µs pulse width, 0.5 s train duration, and biphasic pulses), generally enhances neuroplasticity and improvements following TPT across a variety of applications (Hays et al., 2023).
There is now increasing interest in using noninvasive TaVNS for TPT, also known to activate subcortical structures such as the NTS and LC (Frangos et al., 2015; Sclocco et al., 2019). TPT paradigms that utilize TaVNS are now being applied to stroke rehabilitation (Badran et al., 2023; Redgrave et al., 2018; Wu et al., 2020) and have shown promise in smaller cohorts of patients. More extensive large-scale clinical trials can be pursued to assess whether TaVNS is a reliable means of providing TPT and enhancing recovery. TPT using noninvasive TcVNS at the cervical level has also shown promise (Jigo et al., 2024; McIntire et al., 2021). Overall, new data indicates that the effects of TaVNS outcomes may be variable compared to iVNS (Thompson et al., 2021; Verma et al., 2021; Badran et al., 2018; Warren et al., 2019; Keute et al., 2019; Gadeyne et al., 2022). This variability could partly be due to differences in fiber recruitment and subsequent activation of the associated neuromodulatory centers, which often results in differential brain network modulation when comparing iVNS and TaVNS (Schuerman et al., 2021). Moreover, these noninvasive studies are commonly done in human subjects, where the variability across investigations could be due to several factors, including patient-specific pathophysiology. Further assessing both TaVNS and TcVNS clinically, and its specific mechanisms preclinically, should allow for a sufficient characterization of the stimulation parameter space and optimization of outcomes as needed following non-invasive TPT.
Overall, there are a number of aspects to consider when optimizing the stimulation dose for TPT. Regardless, the possible effects of altered cognitive states and cardiorespiratory rhythms on TPT-induced plasticity and recovery remain poorly understood. Both of these processes affect neuromodulator signaling. Altered cognitive states may likely affect several required stimulation dose components, including stimulus intensity, pulse width, and duration, while cardiorespiratory rhythms may ultimately impact the timing required for optimized VNS delivery.
While the stimulation dose for maximizing plasticity is robust and generalizable in preclinical models (Hays et al., 2023; Souza et al., 2021a; Tyler et al., 2017), the responder rates in clinical trials have varied (e.g., 47% in the VNS-REHAB pivotal trial for stroke and 50% using VNS paired with tone therapy for tinnitus). This emphasizes a need to possibly optimize the stimulation dose further and specifically consider patient-specific states for improving responder rates and recovery.
Patients with neurological injuries or diseases often have comorbidities, leading to altered cognitive states such as enhanced pain perception, arousal deficits, and lack of motivation. For instance, spinal cord injuries may result in neuropathic pain (Henderson et al., 2011; Blumenthal et al., 2021), while parkinsonism can alter arousal levels (Verma et al., 2024) and induce sudden daytime sleepiness (Verma et al., 2023). Stroke patients also often exhibit apathy (Marin and Wilkosz, 2005). These physiological changes impact neuromodulator transmission and cortical excitability and can therefore potentially modify the effects of TPT.
Recent preclinical studies have highlighted that TPT may fail to improve motor recovery in the presence of neuropathic pain (Adcock et al., 2022). In this study, neuropathic pain may have also contributed to the animal’s overall behavioral engagement in the task. Regardless, pain alters cholinergic signaling in the basal forebrain, potentially affecting TPT-induced neuroplasticity (Oswald et al., 2022; Paquette et al., 2019; Piché et al., 2010). Moreover, neuropathic pain can have effects on noradrenergic signaling in the LC, manifesting as an exacerbation of noradrenergic transmission when co-occurring with chronic depression (Bravo et al., 2014). This suggests that considering the subject’s pain perception may be crucial when optimizing the stimulation dose for TPT. Scoring methodologies, such as a visual analog scale for pain perception, can quantify the patient’s pain perception level (Bodian et al., 2001). Machine learning algorithms can likely use these features to modify stimulus intensity adaptively based on the patient’s pain state, optimizing VNS-mediated neuromodulator release.
Arousal state can also influence learning (Song, 2019), recovery of function after injury (Goldfine and Schiff, 2011), and performance (Yerkes and Dodson, 1908). It is well known that alterations in neuromodulator signaling mediate arousal’s impact on behavior (Joshi et al., 2016; Reimer et al., 2016; Murphy et al., 2014) and cortical excitability, both of which are essential for synaptic plasticity (Lee and Dan, 2012). Recent studies indicate that VNS modulates pupil dilation, a biomarker of arousal, primarily through noradrenergic signaling in the LC and cholinergic signaling in the NB (Mridha et al., 2021; Collins et al., 2021). Moreover, the basal arousal state before VNS delivery differentially impacts cortical excitability and behavioral responses in preclinical models (Collins et al., 2021). Hence, correlates of neuromodulator signaling assessed using pupillometry can possibly be used to measure basal arousal state and inform the efficacy of VNS delivery. Measuring the high-frequency to low-frequency activity ratios derived from EEG is another possible avenue for assessing arousal levels (Putman et al., 2014). In addition, the P300 signal derived from EEG can also be utilized to quantify correlates of attention and noradrenergic signaling (D’Agostini et al., 2023; Gurtubay et al., 2023; Riccio et al., 2013). While these findings are promising, they await testing in human subjects to understand the complex interaction between arousal states and responses to VNS.
Conventionally, when examining the relationship between arousal and behavior, the Yerke-Dodson law (Yerkes and Dodson, 1908) reveals a non-monotonic inverted U relationship, with moderate arousal being the most optimal and a hyper-aroused state (e.g., stress or pain) being detrimental to performance. Interestingly, it has been hypothesized that hyperarousal resulting from a higher stimulation dose could impede TPT (Hays et al., 2023), potentially explaining the inverted U relationship between stimulation dose and TPT. The relationship between neuromodulator signaling and stimulation parameter optimization has also been modeled previously (Buell et al., 2019). Based on these findings, arousal is likely to significantly modify neuromodulator signaling. Overall, these findings suggest that the arousal levels during TPT sessions likely influences the relationship between stimulation dose, plasticity, and performance. Overall, further studies are needed to elucidate the intricate relationship between arousal states and performance during TPT.
In addition to arousal deficits, diminished motivation is a common comorbidity following brain injury, such as stroke (Marin and Wilkosz, 2005; Verrienti et al., 2023). Lack of motivation adversely impacts rehabilitation therapy efficacy (Yoshida et al., 2023), as well as BCI performance (Kleih-Dahms et al., 2021). This is partly due to impaired endogenous dopaminergic engagement, where the energetic cost of effort often outweighs the reward value (Neuser et al., 2020). Notably, VNS therapy can improve the motivation to work for rewards (Neuser et al., 2020). However, to optimize therapy outcomes, it is necessary to understand how this improvement in motivation interacts with and affects functional outcomes in VNS-based TPT studies.
Medication taken by patients that affects neuromodulator signaling likely also affects VNS mediated neuromodulator release and may limit TPT efficacy (for a more detailed review, refer to (Hays et al., 2023)). In a recent preclinical study, preliminary findings support using beta blockers to alter the inverted U relationship between stimulus intensity and neuroplasticity (Neifert et al., 2023). Moreover, alpha-blockers also attenuated activity-dependent plasticity during rehabilitation (Sawaki et al., 2003). These studies highlight the need to carefully consider the pharmacological agents administered when designing therapeutic strategies that rely on neuromodulator systems such as TPT. In summary, there is a critical need to further assess how altered cognitive states discussed above may interact with VNS-induced neuromodulator signaling, TPT, and recovery.
In addition to cognitive states, cardiorespiratory rhythms also robustly modulate neuromodulator signaling. Sensory afferents from the vagus nerve terminate within the NTS, carrying information related to a number of visceral processes including cardiovascular and respiratory states (e.g., via baroreceptors and pulmonary stretch receptors). Cardiorespiratory afferents arise from several locations, including mechanoreceptors signaling changes in blood pressure and pulmonary stretch (primarily located in the aortic arch, the carotid sinus, and the pulmonary tissues), as well as peripheral chemoreceptors signaling changes in blood gas content (primarily located in the carotid body and aortic body) (Zyuzin and Jendzjowsky, 2022). These systems interact with each other and modulate overall NTS activity (Spyer, 1982; Rogers et al., 1993; Hayward and Felder, 1995; Zoccal et al., 2014; Mifflin, 1993; Beaumont et al., 2017). Hence, it’s likely that neuromodulator release induced by VNS may be differentially modulated across the ongoing phases of the cardiovascular (systole or diastole) and respiratory rhythms (inspiration or expiration).
Neural activity in the LC is differentially modulated during ongoing phases of the cardiovascular and respiratory rhythms (Biancardi et al., 2014; Biancardi et al., 2008; Iwamoto et al., 2023; Magalhães et al., 2018; Morilak et al., 1986; Patrone et al., 2014; Carvalho et al., 2017). For example, neurons in the LC are more likely to be activated during the diastolic phase rather than the systolic phase of the cardiovascular rhythm (Morilak et al., 1986; Morilak et al., 1987). Respiratory rhythms also entrain the neuronal activity of LC, making it more responsive to specific phases of the respiratory cycle (Magalhães et al., 2018; Huangfu et al., 1992; Iwamoto et al., 2023). This was recently assessed using fMRI, demonstrating a greater activation of the LC when VNS was delivered during exhalation compared to inhalation (Sclocco et al., 2019). Overall, studies have used VNS delivered during expiration demonstrating that the NTS may be more sensitive to afferent input during this phase of the respiratory cycle (Garcia et al., 2017; Sclocco et al., 2019). Neuronal activity in the LC also processes information related to blood oxygenation. In fact, studies have shown that the firing frequency of LC is heightened during conditions of hypoxia and hypercapnia (Magalhães et al., 2018; Biancardi et al., 2008). Hence, conditions of altered chemoreceptor signaling (Plataki et al., 2013) may potentially impact neuromodulator signaling in the LC during TPT.
Aside from subcortical structures, cortical excitability in response to sensory stimuli is also preferentially modulated depending on the phase of the cardiovascular cycle (Park et al., 2018; Gray et al., 2010). A recent study demonstrated that both somatosensory evoked potentials and pain perception were higher during the diastolic phase than the systolic phase of the cardiovascular rhythm (Al et al., 2020). Interestingly, this phenomenon also exists across different modalities of sensory stimuli (for an extensive review, refer to Critchley and Garfinkel (2015)).
Lastly, cardiovascular measures such as heart rate variability have been used as a marker of sympathetic and parasympathetic balance (Bravi et al., 2013), usually quantified as the ratio of low frequency (0.04–0.15 Hz) to high frequency (0.15–0.4 Hz) activity. Overall, these measures are sensitive to recording length, signal processing method, breathing, body position, and emotional state (Shaffer and Ginsberg, 2017). Longer recording times pose an additional challenge for precise spectral estimation, making it possibly suboptimal for adaptive TPT paradigms requiring shorter timescales (i.e., seconds). Additionally, recent studies demonstrate that direct recordings of cardiac vagal activity correlate with a subset of heart rate variability parameters (Laborde et al., 2017; Marmerstein et al., 2021; Billman, 2013). Nevertheless, HRV could be useful for quantifying arousal levels (Huo et al., 2023).
Taken together, these studies demonstrate the extensive influence of cardiovascular and respiratory factors on neuromodulator signaling and the sensitivity of inputs originating from both VNS and other sensory stimuli (both factors critically involved in several forms of TPT). Given the impact of cardiorespiratory rhythms on neuromodulator signaling, it is tempting to speculate that VNS during diastole may better facilitate neuromodulator release. Additionally, VNS during expiration may also be beneficial in driving neuroplasticity. Appropriately timing VNS during TPT, considering both the targeted event and the optimal phase(s) of the cardiorespiratory rhythms, may likely further enhance neuroplasticity and behavioral outcomes after TPT. Regardless, extensive work is needed to address these hypotheses.
Altered cognitive states and cardiorespiratory rhythms affect neuromodulator tone, potentially significantly impacting TPT. To assess the possibility of optimizing TPT for personalized use, it is important to consider not only the individual impacts of each state and autonomic rhythm on neuromodulator signaling, but also their complex interactions that could ultimately affect plasticity (Mylavarapu et al., 2023). Given the nature of these interactions, machine learning frameworks may be needed to both fine-tune VNS parameters and further identify relationships between signals (Ganzer et al., 2022; Ganzer and Sharma, 2019). Overall, the performance of any state dependent TPT system is dependent on several factors, including system controller inputs and overall system design. Table 1 summarizes a number of existing neuromodulation devices and their associated stimulation control schemes. These systems have the following stimulation control schemes: 1) open-loop control consisting of a static control algorithm, where stimulation parameters are fixed regardless of the given state; 2) closed-loop feedforward control triggering stimulation delivery via a change in a monitored variable alone; 3) closed-loop feedback control monitoring a variable that needs to be modified in order to alleviate symptoms, therefore optimizing the stimulation dose in an adaptive and closed-loop manner.
Table 1. Overview of selected neuromodulation devices and their control system attributes. Abbreviations: iVNS (implanted vagus nerve stimulation), TPT (targeted plasticity therapy), ECG (electrocardiography), DBS (deep brain stimulation), ECoG (electrocorticography), SCS (spinal cord stimulation), ECAP (evoked compound action potential), LFP (Local field potential), and TcVNS (transcutaneous vagus nerve stimulation).
A state dependent TPT system (schematized in Figure 2) can possibly leverage a number of technology attributes listed in Table 1. As previously discussed, altered cognitive states and cardiorespiratory rhythms may affect neuromodulator signaling and ultimately TPT efficacy. Fortunately, most of these factors, with the exception of pain, have viable biomarkers that can be used for decoding (Figure 2). Spectral analysis of electrocorticography (ECoG) signals have been integrated into responsive neurostimulation systems for epilepsy (Table 1). Similar to ECoG, EEG is also a valuable electrophysiological signal and can be used to assess a patient’s arousal levels and attention (Figure 2A; e.g., via a decrease in the ratio of high-frequency to low-frequency spectral power in the EEG). Additionally, a correlate of basal neuromodulator signaling can be extracted from pupillometry as a complimentary feature for arousal, with additional potential use as a biomarker of VNS-induced neuromodulator release (Figure 2A). For measuring the phases of ongoing cardiovascular and respiratory rhythms, wearable sensors can be utilized similar to other existing technologies (Figure 2B; e.g., via electrocardiography and pneumography) (Bayoumy et. al., 2021). Functional improvements over longer time scales may also be used to potentially adjust stimulation dose and enhance outcomes (Mylavarapu et al., 2023; Epperson et al, 2024), as further recovery of function may require modification of stimulation parameters across time during TPT. Overall, these are a number of hypothesis generating considerations and challenges related to state dependent TPT that require further investigation.
Figure 2. System design considerations for state dependent TPT. The following information is applied to upper limb TPT as an example. (A) Altered states such as pain and arousal deficits can alter neuromodulator signaling. Increased pain perception may require reducing VNS intensity (e.g., via amplitude or pulse width) characterized by negative weights (W1 < 0), while arousal deficits may require compensation (W2) by increasing stimulus intensity to achieve the desired neuromodulator signaling. Pupillometry can also be used to extract an indirect fast measure of neuromodulator signaling (W3), with force feedback during upper limb rehabilitation measuring a slower and long-term biomarker of functional improvement (W4), all allowing for further adjustment of VNS parameters and possible optimization of recovery. (B) The specific timing of VNS during TPT may also play a significant role in improving neuroplasticity and recovery of function. For example, force feedback during upper limb TPT can be used as a fast read-out of successful movement trials. TPT during diastole (W5 = 1, if diastole) may further improve neuromodulator signaling. VNS during the expiratory phase of the respiratory cycle (W6 = 1, if expiration) may also lead to optimized neuromodulator release and, hence, enhanced neuroplasticity. Overall, this a priori knowledge can be used to modify the given weights of the schematized control system with further fine-tuning via adaptive machine-learning algorithms.
Simple or complex stimulation control algorithms can be used for decoding and triggering optimized VNS. Ultimately, advanced machine learning techniques may be required to effectively integrate these multifactorial inputs and enhance VNS control (Ganzer et al., 2022; Ganzer and Sharma, 2019). Bayesian optimization represents one option. This approach iteratively refreshes assessment of the response surface by utilizing a probabilistic model that effectively converges on ideal settings, while maintaining a balance between exploration and exploitation (Shahriari et al., 2016). Bayesian optimization has also been extensively used in neurostimulation (Choinière et al., 2024). Furthermore, bayesian optimization may be especially effective for individualizing therapy, as it can adapt to each patient’s unique physiological profile. In addition, deep neural networks and ensemble learning are likely viable options for optimizing VNS control. Deep learning algorithms can handle large datasets that may be required for deciphering the complex relationship between state dependent signals and their role in optimized neuroplasticity. In the context of movement disorders, these models can discern subtle changes in the states mentioned above, potentially maximizing the effectiveness of VNS during TPT. Furthermore, these models may also be able to relate physiological states to optimized VNS control in ways that are not self-evident to humans. Lastly, ensemble learning can combine decisions from multiple models, where simple and complex algorithms are being used in combination (Dietterich, 2000) (e.g., complex arousal state detection using EEG combined with simpler cardiac cycle detection using electrocardiography). Ultimately, future studies will need to consider the combinatorial characterization of physiological states and autonomic rhythms on neuromodulator signaling, plasticity, and functional improvement during TPT, paving the way towards state dependent VNS and enhanced TPT.
BN: Conceptualization, Formal Analysis, Investigation, Methodology, Project administration, Validation, Visualization, Writing–original draft, Writing–review and editing. RM: Conceptualization, Investigation, Methodology, Visualization, Writing–original draft, Writing–review and editing. RH: Conceptualization, Formal Analysis, Investigation, Validation, Writing–original draft, Writing–review and editing. EA: Methodology, Resources, Validation, Writing–original draft, Writing–review and editing. ZY: Resources, Validation, Writing–original draft, Writing–review and editing. CH: Resources, Validation, Writing–original draft, Writing–review and editing. DM: Writing–original draft, Writing–review and editing. VK: Resources, Validation, Writing–original draft, Writing–review and editing. PG: Conceptualization, Funding acquisition, Investigation, Methodology, Project administration, Resources, Supervision, Validation, Writing–original draft, Writing–review and editing.
The author(s) declare that financial support was received for the research, authorship, and/or publication of this article. 1R01NS131493-01 (NIH NINDS grant)
We thank Dr. Eric Meyers for providing feedback on manuscript.
The authors declare that the research was conducted in the absence of any commercial or financial relationships that could be construed as a potential conflict of interest.
All claims expressed in this article are solely those of the authors and do not necessarily represent those of their affiliated organizations, or those of the publisher, the editors and the reviewers. Any product that may be evaluated in this article, or claim that may be made by its manufacturer, is not guaranteed or endorsed by the publisher.
Adcock, K. S., Danaphongse, T., Jacob, S., Rallapalli, H., Torres, M., Haider, Z., et al. (2022). Vagus nerve stimulation does not improve recovery of forelimb motor or somatosensory function in a model of neuropathic pain. Sci. Rep. 12 (1), 9696. doi:10.1038/s41598-022-13621-3
Al, E., Iliopoulos, F., Forschack, N., Nierhaus, T., Grund, M., Motyka, P., et al. (2020). Heart-brain interactions shape somatosensory perception and evoked potentials. PNAS 117 (19), 10575–10584. doi:10.1073/pnas.1915629117
Altidor, L. K. P., Bruner, M. M., Deslauriers, J. F., Garman, T. S., Ramirez, S., Dirr, E. W., et al. (2021). Acute vagus nerve stimulation enhances reversal learning in rats. Neurobiol. Learn. Mem. 184 (October), 107498. doi:10.1016/j.nlm.2021.107498
Badran, B. W., Dowdle, L. T., Mithoefer, O. J., LaBate, N. T., Coatsworth, J., Brown, J. C., et al. (2018). Neurophysiologic effects of transcutaneous auricular vagus nerve stimulation (TaVNS) via electrical stimulation of the tragus: a concurrent TaVNS/fmri study and review. Brain Stimul. 11 (3), 492–500. doi:10.1016/j.brs.2017.12.009
Badran, B. W., Peng, X., Baker-Vogel, B., Hutchison, S., Finetto, P., Kelly, R., et al. (2023). Motor activated auricular vagus nerve stimulation as a potential neuromodulation approach for post-stroke motor rehabilitation: a pilot study. Neurorehabilitation Neural Repair 37 (6), 374–383. doi:10.1177/15459683231173357
Bailey, C. H., Giustetto, M., Huang, Y. Y., Hawkins, R. D., and Kandel, E. R. (2000). Is heterosynaptic modulation essential for stabilizing hebbian plasiticity and memory. Nat. Rev. Neurosci. 1 (1), 11–20. doi:10.1038/35036191
Bayoumy, K., Gaber, M., Elshafeey, A., Omar, M., Dineen, E. H., Marvel, F. A., et al. (2021). Smart wearable devices in cardiovascular care: where we are and how to move forward. Nat. Rev. Cardiol. 18, 581–599. doi:10.1038/s41569-021-00522-7
Beaumont, E., Campbell, R. P., Andresen, M. C., Scofield, S., Singh, K., Libbus, I., et al. (2017). Cervical vagus nerve stimulation augments spontaneous discharge in second-and higher-order sensory neurons in the rat nucleus of the solitary tract. Am. J. Physiol. Heart Circ. Physiol. 313, 354–367. doi:10.1152/ajpheart.00070.2017
Biancardi, V., Bícego, K. C., Almeida, M. C., and Gargaglioni, L. H. (2008). Locus coeruleus noradrenergic neurons and CO2 drive to breathing. Pflügers Archiv - Eur. J. Physiology 455 (6), 1119–1128. doi:10.1007/s00424-007-0338-8
Biancardi, V., Bícego, K. C., and Gargaglioni, L. H. (2014). ATP in the locus coeruleus as a modulator of cardiorespiratory control in unanaesthetized male rats. Exp. Physiol. 99 (1), 232–247. doi:10.1113/expphysiol.2013.074195
Biggio, F., Gorini, G., Utzeri, C., Olla, P., Marrosu, F., Mocchetti, I., et al. (2009). Chronic vagus nerve stimulation induces neuronal plasticity in the rat Hippocampus. Int. J. Neuropsychopharmacol. 12 (9), 1209–1221. doi:10.1017/S1461145709000200
Billman, G. E. (2013). The LF/HF ratio does not accurately measure cardiac sympatho-vagal balance. Front. Physiology 4, 26. doi:10.3389/fphys.2013.00026
Blumenthal, G. H., Nandakumar, B., Schnider, A. K., Detloff, M. R., Ricard, J., Bethea, J. R., et al. (2021). Modelling at-level allodynia after mid-thoracic contusion in the rat. Eur. J. Pain 25, 801–816. doi:10.1002/ejp.1711
Bodian, C. A., Freedman, G., Hossain, S., Eisenkraft, J. B., and Beilin, Y. (2001). The visual analog scale for pain. Anesthesiology 95, 1356–1361. doi:10.1097/00000542-200112000-00013
Borland, M. S., Engineer, C. T., Vrana, W. A., Moreno, N. A., Engineer, N. D., Vanneste, S., et al. (2018). The interval between VNS-tone pairings determines the extent of cortical map plasticity. Neuroscience 369 (January), 76–86. doi:10.1016/j.neuroscience.2017.11.004
Borland, M. S., Vrana, W. A., Moreno, N. A., Fogarty, E. A., Buell, E. P., Sharma, P., et al. (2016). Cortical map plasticity as a function of vagus nerve stimulation intensity. Brain Stimul. 9 (1), 117–123. doi:10.1016/j.brs.2015.08.018
Bowles, S., Hickman, J., Peng, X., Ryan Williamson, W., Huang, R., Washington, K., et al. (2022). Vagus nerve stimulation drives selective circuit modulation through cholinergic reinforcement. Neuron 110 (17), 2867–2885.e7. doi:10.1016/j.neuron.2022.06.017
Bravi, A., Green, G., Herry, C., Wright, H. E., Longtin, A., Kenny, G. P., et al. (2013). Do physiological and pathological stresses produce different changes in heart rate variability? Front. Physiology 4 (JUL), 197. doi:10.3389/fphys.2013.00197
Bravo, L., Torres-Sanchez, S., Alba-Delgado, C., Mico, J. A., and Berrocoso, E. (2014). Pain exacerbates chronic mild stress-induced changes in noradrenergic transmission in rats. Eur. Neuropsychopharmacol. 24 (6), 996–1003. doi:10.1016/j.euroneuro.2014.01.011
Brougher, J., Aziz, U., Adari, N., Chaturvedi, M., Jules, A., Shah, I., et al. (2021). Self-administration of right vagus nerve stimulation activates midbrain dopaminergic nuclei. Front. Neurosci. 15 (December), 782786. doi:10.3389/fnins.2021.782786
Brzosko, Z., Mierau, S. B., and Paulsen, O. (2019). Neuromodulation of spike-timing-dependent plasticity: past, present, and future. Neuron 103, 563–581. doi:10.1016/j.neuron.2019.05.041
Buell, E. P., Borland, M. S., Loerwald, K. W., Chandler, C., Hays, S. A., Engineer, C. T., et al. (2019). Vagus nerve stimulation rate and duration determine whether sensory pairing produces neural plasticity. Neuroscience 406 (May), 290–299. doi:10.1016/j.neuroscience.2019.03.019
Cagnan, H., Pedrosa, D., Little, S., Pogosyan, A., Cheeran, B., Aziz, T., et al. (2017). Stimulating at the right time: phase-specific deep brain stimulation. Brain 140 (1), 132–145. doi:10.1093/brain/aww286
Carvalho, D., Patrone, L. G. A., Marques, D. A., Vicente, M. C., Szawka, R. E., Anselmo-Franci, J. A., et al. (2017). Participation of locus coeruleus in breathing control in female rats. Respir. Physiology and Neurobiol. 245, 29–36. doi:10.1016/j.resp.2017.06.008
Chen, S., Du, M., Wang, Y., Li, Y., Tong, B., Qiu, J., et al. (2023). State of the art: non-invasive electrical stimulation for the treatment of chronic tinnitus. Ther. Adv. Chronic Dis. 14, 204062232211480. doi:10.1177/20406223221148061
Choinière, L., Guay-Hottin, R., Picard, R., Lajoie, G., Bonizzato, M., and Dancause, N. (2024). Gaussian-Process-Based bayesian optimization for neurostimulation interventions in rats. Star. Protoc. 5 (1), 102885. doi:10.1016/j.xpro.2024.102885
Collins, L., Boddington, L., Steffan, P. J., and McCormick, D. (2021). Vagus nerve stimulation induces widespread cortical and behavioral activation. Curr. Biol. 31 (10), 2088–2098.e3. doi:10.1016/j.cub.2021.02.049
Conner, J. M., Kulczycki, M., and Tuszynski, M. H. (2010). Unique contributions of distinct cholinergic projections to motor cortical plasticity and learning. Cereb. Cortex 20 (11), 2739–2748. doi:10.1093/cercor/bhq022
Critchley, H. D., and Garfinkel, S. N. (2015). Interactions between visceral afferent signaling and stimulus processing. Front. Neurosci. 9, 286. doi:10.3389/fnins.2015.00286
D’Agostini, M., Burger, A. M., Jelinčić, V., Leupoldt, A. V., and Diest, I. V. (2023). Effects of transcutaneous auricular vagus nerve stimulation on P300 magnitudes and salivary alpha-amylase during an auditory oddball task. Biol. Psychol. 182 (September), 108646. doi:10.1016/j.biopsycho.2023.108646
Darrow, M. J., Mian, T. M., Torres, M., Haider, Z., Danaphongse, T., Seyedahmadi, A., et al. (2021). The tactile experience paired with vagus nerve stimulation determines the degree of sensory recovery after chronic nerve damage. Behav. Brain Res. 396 (January), 112910. doi:10.1016/j.bbr.2020.112910
Darrow, M. J., Torres, M., Sosa, M. J., Danaphongse, T. T., Haider, Z., Rennaker, R. L., et al. (2020). Vagus nerve stimulation paired with rehabilitative training enhances motor recovery after bilateral spinal cord injury to cervical forelimb motor pools. Neurorehabilitation Neural Repair 34 (3), 200–209. doi:10.1177/1545968319895480
Dawson, J., Liu, C. Y., Francisco, G. E., Cramer, S. C., Wolf, S. L., Dixit, A., et al. (2021). Vagus nerve stimulation paired with rehabilitation for upper limb motor function after ischaemic stroke (VNS-rehab): a randomised, blinded, pivotal, device trial. Lancet 397, 1545–1553. doi:10.1016/S0140-6736(21)00475-X
Dickie, D. A., Jacobson Kimberley, T., Pierce, D., Engineer, N., Brent Tarver, W., and Dawson, J. (2019). An exploratory study of predictors of response to vagus nerve stimulation paired with upper-limb rehabilitation after ischemic stroke. Sci. Rep. 9 (1), 15902. doi:10.1038/s41598-019-52092-x
Dietterich, T. G. (2000). “Ensemble methods in machine learning,” in Lecture notes in computer science (Springer), 1–15. doi:10.1007/3-540-45014-9_1
Dorr, A. E., and Guy, D. (2006). Effect of vagus nerve stimulation on serotonergic and noradrenergic transmission. J. Pharmacol. Exp. Ther. 318 (2), 890–898. doi:10.1124/jpet.106.104166
Engineer, N. D., Riley, J. R., Seale, J. D., Vrana, W. A., Shetake, J. A., Sudanagunta, S. P., et al. (2011). Reversing pathological neural activity using targeted plasticity. Nature 470 (7332), 101–104. doi:10.1038/nature09656
Epperson, J. D., Meyers, E. C., Pruitt, D. T., Wright, J. M., Hudson, R. A., Adehunoluwa, E. A., et al. (2024). Characterization of an algorithm for autonomous, closed-loop neuromodulation during motor rehabilitation. Neurorehabilitation Neural Repair 38 (7), 493–505. doi:10.1177/15459683241252599
Ethier, C., Gallego, J. A., and Miller, L. E. (2015). Brain-controlled neuromuscular stimulation to drive neural plasticity and functional recovery. Curr. Opin. Neurobiol. 33 (August), 95–102. doi:10.1016/j.conb.2015.03.007
Faller, J., Cummings, J., Saproo, S., and Paul, S. (2019). Regulation of arousal via online neurofeedback improves human performance in a demanding sensory-motor task. Proc. Natl. Acad. Sci. 116 (15), 6482–6490. doi:10.1073/pnas.1817207116
Fernandes, A. B., Silva, J. A., Almeida, J., Cui, G., Gerfen, C. R., Costa, R. M., et al. (2020). Postingestive modulation of food seeking depends on vagus-mediated dopamine neuron activity. Neuron 106 (5), 778–788.e6. doi:10.1016/j.neuron.2020.03.009
Fleming, J. E., Kremen, V., Gilron, E., Gregg, N. M., Zamora, M., Dijk, D.-J., et al. (2022). Embedding digital chronotherapy into bioelectronic medicines. IScience 25, 104028. doi:10.1016/j.isci.2022.104028
Francisco, G. E., Engineer, N. D., Dawson, J., Kimberley, T. J., Cramer, S. C., Prudente, C. N., et al. (2023). Vagus nerve stimulation paired with upper-limb rehabilitation after stroke: 2- and 3-year follow-up from the pilot study. Archives Phys. Med. Rehabilitation 104 (8), 1180–1187. doi:10.1016/j.apmr.2023.02.012
Frangos, E., Ellrich, J., and Komisaruk, B. R. (2015). Non-invasive access to the vagus nerve central projections via electrical stimulation of the external ear: FMRI evidence in humans. Brain Stimul. 8 (3), 624–636. doi:10.1016/j.brs.2014.11.018
Froemke, R. C., Merzenich, M. M., and Schreiner, C. E. (2007). A synaptic memory trace for cortical receptive field plasticity. Nature 450 (7168), 425–429. doi:10.1038/nature06289
Furmaga, H., Carreno, F. R., and Frazer, A. (2012). Vagal nerve stimulation rapidly activates brain-derived neurotrophic factor receptor TrkB in rat brain. PLoS ONE 7 (5), e34844. doi:10.1371/journal.pone.0034844
Gadeyne, S., Mertens, A., Carrette, E., Bossche, F. V. D., Paul, B., Raedt, R., et al. (2022). Transcutaneous auricular vagus nerve stimulation cannot modulate the P3b event-related potential in healthy volunteers. Clin. Neurophysiol. 135 (March), 22–29. doi:10.1016/j.clinph.2021.11.079
Gallego, J. A., Makin, T. R., and McDougle, S. D. (2022). Going beyond primary motor cortex to improve brain–computer interfaces. Trends Neurosci. 45, 176–183. doi:10.1016/j.tins.2021.12.006
Ganzer, P. D., Darrow, M. J., Meyers, E. C., Solorzano, B. R., Ruiz, A. D., Robertson, N. M., et al. (2018). Closed-loop neuromodulation restores network connectivity and motor control after spinal cord injury. ELife 7, e32058. doi:10.7554/eLife.32058
Ganzer, P. D., Loeian, M. S., Roof, S. R., Teng, B., Lin, L., Friedenberg, D. A., et al. (2022). Dynamic detection and reversal of myocardial ischemia using an artificially intelligent bioelectronic medicine. Sci. Adv. 8 (1), eabj5473. Epub 2022 Jan 5. PMID: 34985951; PMCID: PMC8730601. doi:10.1126/sciadv.abj5473
Ganzer, P. D., and Sharma, G. (2019). Opportunities and challenges for developing closed-loop bioelectronic medicines. Neural Regen. Res. 14 (1), 46–50. PMID: 30531069; PMCID: PMC6262994. doi:10.4103/1673-5374.243697
Garcia, R. G., Lin, R. L., Lee, J., Kim, J., Barbieri, R., Sclocco, R., et al. (2017). Modulation of brainstem activity and connectivity by respiratory-gated auricular vagal afferent nerve stimulation in migraine patients. Pain 158 (8), 1461–1472. doi:10.1097/j.pain.0000000000000930
Gerstner, W., Lehmann, M., Liakoni, V., Corneil, D., and Brea, J. (2018). Eligibility traces and plasticity on behavioral time scales: experimental support of NeoHebbian three-factor learning rules. Front. Neural Circuits 12, 53. doi:10.3389/fncir.2018.00053
Gilron, R., Little, S., Wilt, R., Perrone, R., Anso, J., and Starr, P. A. (2021). Sleep-aware adaptive deep brain stimulation control: chronic use at home with dual independent linear discriminate detectors. Front. Neurosci. 15 (October), 732499. doi:10.3389/fnins.2021.732499
Goldfine, A. M., and Schiff, N. D. (2011). What is the role of brain mechanisms underlying arousal in recovery of motor function after structural brain injuries? Curr. Opin. Neurology 24, 564–569. doi:10.1097/WCO.0b013e32834cd4f5
Gray, M. A., Minati, L., Paoletti, G., and Critchley, H. D. (2010). Baroreceptor activation attenuates attentional effects on pain-evoked potentials. Pain 151 (3), 853–861. doi:10.1016/j.pain.2010.09.028
Gu, Q. (2002). Neuromodulatory transmitter systems in the cortex and their role in cortical plasticity. Neuroscience 111 (4), 815–835. doi:10.1016/S0306-4522(02)00026-X
Gurel, N. Z., Wittbrodt, M. T., Jung, H., Shandhi, M. H., Driggers, E. G., Ladd, S. L., et al. (2020). Transcutaneous cervical vagal nerve stimulation reduces sympathetic responses to stress in posttraumatic stress disorder: a double-blind, randomized, sham controlled trial. Neurobiol. Stress 13 (November), 100264. doi:10.1016/j.ynstr.2020.100264
Gurtubay, I. G., Perez-Rodriguez, D. R., Fernandez, E., Librero-Lopez, J., Calvo, D., Bermejo, P., et al. (2023). Immediate effects and duration of a short and single application of transcutaneous auricular vagus nerve stimulation on P300 event related potential. Front. Neurosci. 17, 1096865. doi:10.3389/fnins.2023.1096865
Hays, S. A. (2016). Enhancing rehabilitative therapies with vagus nerve stimulation. Neurotherapeutics 13, 382–394. doi:10.1007/s13311-015-0417-z
Hays, S. A., Khodaparast, N., Ruiz, A., Sloan, A. M., Hulsey, D. R., Rennaker, R. L., et al. (2014). The timing and amount of vagus nerve stimulation during rehabilitative training affect poststroke recovery of forelimb strength. NeuroReport 25 (9), 676–682. doi:10.1097/WNR.0000000000000154
Hays, S. A., Rennaker, R. L., and Kilgard, M. P. (2023). How to fail with paired VNS therapy. Brain Stimul. 16, 1252–1258. doi:10.1016/j.brs.2023.08.009
Hays, S. A., Rennaker, R. L., and Kilgard, M. P. (2013). Targeting plasticity with vagus nerve stimulation to treat neurological disease. Prog. Brain Res. 207, 275–299. doi:10.1016/B978-0-444-63327-9.00010-2
Hayward, L. F., and Felder, R. B. (1995). Cardiac rhythmicity among NTS neurons and its relationship to sympathetic outflow in rabbits. Am. J. Physiology-Heart Circulatory Physiology 269 (3), H923–H933. doi:10.1152/ajpheart.1995.269.3.H923
He, K., Huertas, M., Hong, Su Z., Tie, X. X., Hell, J. W., Shouval, H., et al. (2015). Distinct eligibility traces for LTP and LTD in cortical synapses. Neuron 88 (3), 528–538. doi:10.1016/j.neuron.2015.09.037
Henderson, L. A., Gustin, S. M., Macey, P. M., Wrigley, P. J., and Siddall, P. J. (2011). Functional reorganization of the brain in humans following spinal cord injury: evidence for underlying changes in cortical anatomy. J. Neurosci. 31 (7), 2630–2637. doi:10.1523/JNEUROSCI.2717-10.2011
Hennig, J. A., Oby, E. R., Golub, M. D., Bahureksa, L. A., Sadtler, P. T., Quick, K. M., et al. (2021). Learning is shaped by abrupt changes in neural engagement. Nat. Neurosci. 24 (5), 727–736. doi:10.1038/s41593-021-00822-8
Hirata, A., Aguilar, J., and Castro-Alamancos, M. A. (2006). Noradrenergic activation amplifies bottom-up and top-down signal-to-noise ratios in sensory thalamus. J. Neurosci. 26 (16), 4426–4436. doi:10.1523/JNEUROSCI.5298-05.2006
Huangfu, D., Verberne, A. J. M., and Guyenet, P. G. (1992). Rostral ventrolateral medullary neurons projecting to locus coeruleus have cardiorespiratory inputs. Brain Res. 598 (1), 67–75. doi:10.1016/0006-8993(92)90169-A
Hulsey, D. R., Hays, S. A., Khodaparast, N., Ruiz, A., Das, P., Rennaker, R. L., et al. (2016a). Reorganization of motor cortex by vagus nerve stimulation requires cholinergic innervation. Brain Stimul. 9 (2), 174–181. doi:10.1016/j.brs.2015.12.007
Hulsey, D. R., Riley, J. R., Loerwald, K. W., Rennaker Ii, R. L., Kilgard, M. P., and Hays, S. A. (2016b). Title: 1 parametric characterization of neural activity in the locus coeruleus in response to vagus 2 nerve stimulation. Exp. Neurol. 289, 21–30. doi:10.1016/j.expneurol.2016.12.005
Hulsey, D. R., Shedd, C. M., Sarker, S. F., Kilgard, M. P., and Hays, S. A. (2019). Norepinephrine and serotonin are required for vagus nerve stimulation directed cortical plasticity. Exp. Neurol. 320 (October), 112975. doi:10.1016/j.expneurol.2019.112975
Huo, J., Quan, S. F. , Roveda, J., and Li, A. (2023). Coupling analysis of heart rate variability and cortical arousal using a deep learning algorithm. PLoS ONE 18 (4), e0284167. doi:10.1371/journal.pone.0284167
Iwamoto, M., Yonekura, S., Atsumi, N., Hirabayashi, S., Kanazawa, H., and Kuniyoshi, Y. (2023). Respiratory entrainment of the locus coeruleus modulates arousal level to avoid physical risks from external vibration. Sci. Rep. 13 (1), 7069. doi:10.1038/s41598-023-32995-6
Jigo, M., Carmel, J. B., Wang, Q., and Rodenkirch, C. (2024). Transcutaneous cervical vagus nerve stimulation improves sensory performance in humans: a randomized controlled crossover pilot study. Sci. Rep. 14 (1), 3975. doi:10.1038/s41598-024-54026-8
Joshi, S., Yin, L., Kalwani, R. M., and Gold, J. I. (2016). Relationships between pupil diameter and neuronal activity in the locus coeruleus, colliculi, and cingulate cortex. Neuron 89 (1), 221–234. doi:10.1016/j.neuron.2015.11.028
Keute, M., Demirezen, M., Graf, A., Mueller, N. G., and Zaehle, T. (2019). No modulation of pupil size and event-related pupil response by transcutaneous auricular vagus nerve stimulation (TaVNS). Sci. Rep. 9 (1), 11452. doi:10.1038/s41598-019-47961-4
Khodaparast, N., Hays, S. A., Sloan, A. M., Fayyaz, T., Hulsey, D. R., Rennaker, R. L., et al. (2014). Vagus nerve stimulation delivered during motor rehabilitation improves recovery in a rat model of stroke. Neurorehabilitation Neural Repair 28 (7), 698–706. doi:10.1177/1545968314521006
Kilgard, M. P., and Merzenich, M. M. (1998). Cortical map reorganization enabled by nucleus basalis activity. Science 279, 1714–1718. doi:10.1126/science.279.5357.1714
Kleih-Dahms, S. C., Botrel, L., and Kübler, A. (2021). The influence of motivation and emotion on sensorimotor rhythm-based brain–computer interface performance. Psychophysiology 58 (8), e13832. doi:10.1111/psyp.13832
Kleim, J. A., and Jones, T. A. (2008). Principles of experience-dependent neural plasticity: implications for rehabilitation after brain damage. J. Speech, Lang. Hear. Res. 51 (1), 225–239. doi:10.1044/1092-4388(2008/018
Laborde, S., Mosley, E., and Thayer, J. F. (2017). Heart rate variability and cardiac vagal tone in psychophysiological research - recommendations for experiment planning, data analysis, and data reporting. Front. Psychol. 08, 213. doi:10.3389/fpsyg.2017.00213
Lee, S. H., and Dan, Y. (2012). Neuromodulation of brain states. Neuron 76, 209–222. doi:10.1016/j.neuron.2012.09.012
Li, Y., and Hollis, E. (2023). Nicotinic acetylcholine signaling is required for motor learning but not for rehabilitation from spinal cord injury. Neural Regen. Res. 18 (2), 364–367. doi:10.4103/1673-5374.346544
Loerwald, K. W., Borland, M. S., Rennaker, R. L., Hays, S. A., and Kilgard, M. P. (2018). The interaction of pulse width and current intensity on the extent of cortical plasticity evoked by vagus nerve stimulation. Brain Stimul. 11 (2), 271–277. doi:10.1016/j.brs.2017.11.007
Magalhães, K. S., Pedro, F. S., da Silva, M. P., Kuntze, L. B., Paton, J. F. R., Machado, B. H., et al. (2018). Locus coeruleus as a vigilance centre for active inspiration and expiration in rats. Sci. Rep. 8 (1), 15654. doi:10.1038/s41598-018-34047-w
Manta, S., El Mansari, M., and Blier, P. (2012). Novel attempts to optimize vagus nerve stimulation parameters on serotonin neuronal firing activity in the rat brain. Brain Stimul. 5 (3), 422–429. doi:10.1016/j.brs.2011.04.005
Manta, S., El Mansari, M., Debonnel, G., and Blier, P. (2013). Electrophysiological and neurochemical effects of long-term vagus nerve stimulation on the rat monoaminergic systems. Int. J. Neuropsychopharmacol. 16 (2), 459–470. doi:10.1017/S1461145712000387
Marin, R. S., and Wilkosz, P. A. (2005). Disorders of diminished motivation. J. Head Trauma Rehabilitation 20 (4)–388. doi:10.1097/00001199-200507000-00009
Marmerstein, J. T., McCallum, G. A., and Durand, D. M. (2021). Direct measurement of vagal tone in rats does not show correlation to HRV. Sci. Rep. 11 (1), 1210. doi:10.1038/s41598-020-79808-8
McIntire, L. K., Andy McKinley, R., Goodyear, C., McIntire, J. P., and Brown, R. D. (2021). Cervical transcutaneous vagal nerve stimulation (CtVNS) improves human cognitive performance under sleep deprivation stress. Commun. Biol. 4 (1), 634. doi:10.1038/s42003-021-02145-7
Meyers, E. C., Kasliwal, N., Solorzano, B. R., Lai, E., Bendale, G., Berry, A., et al. (2019). Enhancing plasticity in central networks improves motor and sensory recovery after nerve damage. Nat. Commun. 10 (1), 5782. doi:10.1038/s41467-019-13695-0
Meyers, E. C., Solorzano, B. R., James, J., Ganzer, P. D., Lai, E. S., Rennaker, R. L., et al. (2018). Vagus nerve stimulation enhances stable plasticity and generalization of stroke recovery. Stroke 49 (3), 710–717. doi:10.1161/STROKEAHA.117.019202
Mifflin, S. W. (1993). Inhibition of chemoreceptor inputs to nucleus of tractus solitarius neurons during baroreceptor stimulation. Am. J. Physiol. 265, R14–R20. doi:10.1152/ajpregu.1993.265.1.R14
Mohammed, H., and Hollis, E. R. (2018). Cortical reorganization of sensorimotor systems and the role of intracortical circuits after spinal cord injury. Neurotherapeutics 15 (3), 588–603. doi:10.1007/s13311-018-0638-z
Monk, K. J., and Hussain Shuler, M. G. (2019). Are you there, cortex? It’s me, acetylcholine. Neuron 103, 954–956. doi:10.1016/j.neuron.2019.08.039
Morilak, D. A., Fornal, C., and Jacobs, B. L. (1986). Single unit activity of noradrenergic neurons in locus coeruleus and serotonergic neurons in the nucleus raphe dorsalis of freely moving cats in relation to the cardiac cycle. Brain Res. 399 (2), 262–270. doi:10.1016/0006-8993(86)91516-7
Morilak, D. A., Fornal, C. A., and Jacobs, B. L. (1987). Effects of physiological manipulations on locus coeruleus neuronal activity in freely moving cats. II. Cardiovascular challenge. Brain Res. 422 (1), 24–31. doi:10.1016/0006-8993(87)90536-1
Moro, E., Poon, Y.-Y. W., Lozano, A. M., Saint-Cyr, J. A., and Lang, A. E. (2006). Subthalamic nucleus stimulation: improvements in outcome with reprogramming. Archives Neurology 63 (9), 1266–1272. doi:10.1001/archneur.63.9.1266
Morris, G. L., and Wade, M. M.The Vagus Nerve Stimulation Study Group E–E (1999). Long-term treatment with vagus nerve stimulation in patients with refractory epilepsy. Neurology 53 (8), 1731. doi:10.1212/WNL.53.8.1731
Mridha, Z., de Gee, J. W., Shi, Y., Alkashgari, R., Williams, J., Suminski, A., et al. (2021). Graded recruitment of pupil-linked neuromodulation by parametric stimulation of the vagus nerve. Nat. Commun. 12 (1), 1539. doi:10.1038/s41467-021-21730-2
Murphy, P. R., O’Connell, R. G., O’Sullivan, M., Robertson, I. H., and Balsters, J. H. (2014). Pupil diameter covaries with BOLD activity in human locus coeruleus. Hum. Brain Mapp. 35 (8), 4140–4154. doi:10.1002/hbm.22466
Mylavarapu, R. V., Kanumuri, V. V., de Rivero Vaccari, J. P., Misra, A., McMillan, D. W., and Ganzer, P. D. (2023). Importance of timing optimization for closed-loop applications of vagus nerve stimulation. Bioelectron. Med. 9 (1), 8. doi:10.1186/s42234-023-00110-9
Nandakumar, B., Blumenthal, G. H., Disse, G. D., Desmond, P. C., Ebinu, J. O., Ricard, J., et al. (2023). Exercise therapy guides cortical reorganization after midthoracic spinal contusion to enhance control of lower thoracic muscles, supporting functional recovery. Exp. Neurol. 364 (June), 114394. doi:10.1016/j.expneurol.2023.114394
Neifert, N. S., Addo, J. A. A. , Danaphongse, T. T., Pillai, S., Montefalcon, J., Razack, A., Kilgard, M. P., and Hays, S. A. (2023). Beta-adrenergic receptor blockades enable enhanced cortical plasticity at high-intensity vagus nerve stimulation, and provide insight into the mechanism of vns-mediated plasticity. Program no. PSTR280.28. 2023 neuroscience meeting planner. Washington, D.C.: Society for Neuroscience.
Neuser, M. P., Teckentrup, V., Kühnel, A., Hallschmid, M., Walter, M., and Kroemer, N. B. (2020). Vagus nerve stimulation boosts the drive to work for rewards. Nat. Commun. 11 (1), 3555. doi:10.1038/s41467-020-17344-9
Nichols, J. A., Nichols, A. R., Smirnakis, S. M., Engineer, N. D., Kilgard, M. P., and Atzori, M. (2011). Vagus nerve stimulation modulates cortical synchrony and excitability through the activation of muscarinic receptors. Neuroscience 189 (August), 207–214. doi:10.1016/j.neuroscience.2011.05.024
Noble, L. J., Gonzalez, I. J., Meruva, V. B., Callahan, K. A., Belfort, B. D., Ramanathan, K. R., et al. (2017). Effects of vagus nerve stimulation on extinction of conditioned fear and post-traumatic stress disorder symptoms in rats. Transl. Psychiatry 7 (8), e1217. doi:10.1038/TP.2017.191
Noble, L. J., Souza, R. R., and McIntyre, C. K. (2019). Vagus nerve stimulation as a tool for enhancing extinction in exposure-based therapies. Psychopharmacology 236, 355–367. doi:10.1007/s00213-018-4994-5
Oehrn, C. R., Cernera, S., Hammer, L. H., Shcherbakova, M., Yao, J., Hahn, A., et al. (2024). Chronic adaptive deep brain stimulation versus conventional stimulation in Parkinson’s disease: a blinded randomized feasibility trial. Nat. Med. doi:10.1038/s41591-024-03196-z
Olsen, L. K., Moore, R. J., Bechmann, N. A., Ethridge, V. T., Gargas, N. M., Cunningham, S. D., et al. (2022). Vagus nerve stimulation-induced cognitive enhancement: hippocampal neuroplasticity in healthy male rats. Brain Stimul. 15 (5), 1101–1110. doi:10.1016/j.brs.2022.08.001
Olsen, L. K., Solis, E., McIntire, L. K., and Hatcher-Solis, C. N. (2023). Vagus nerve stimulation: mechanisms and factors involved in memory enhancement. Front. Hum. Neurosci. 17, 1152064. doi:10.3389/fnhum.2023.1152064
Oswald, M. J., Han, Y., Li, H., Marashli, S., Oglo, D. N., Ojha, B., et al. (2022). Cholinergic basal forebrain nucleus of Meynert regulates chronic pain-like behavior via modulation of the prelimbic cortex. Nat. Commun. 13 (1), 5014. doi:10.1038/s41467-022-32558-9
Paquette, T., Tokunaga, R., Touj, S., Leblond, H., and Piché, M. (2019). Regulation of cortical blood flow responses by the nucleus basalis of Meynert during nociceptive processing. Neurosci. Res. 149 (December), 22–28. doi:10.1016/j.neures.2019.01.008
Park, H. D., Bernasconi, F., Salomon, R., Tallon-Baudry, C., Spinelli, L., Seeck, M., et al. (2018). Neural sources and underlying mechanisms of neural responses to heartbeats, and their role in bodily self-consciousness: an intracranial EEG study. Cereb. Cortex 28 (7), 2351–2364. doi:10.1093/cercor/bhx136
Patrone, L. G. A., Bícego, K. C., Hartzler, L. K., Putnam, R. W., and Gargaglioni, L. H. (2014). Cardiorespiratory effects of gap junction blockade in the locus coeruleus in unanesthetized adult rats. Respir. Physiology and Neurobiol. 190, 86–95. doi:10.1016/j.resp.2013.09.001
Pawlak, V., Wickens, J. R., Kirkwood, A., and Kerr, J. N. D. (2010). Timing is not everything: neuromodulation opens the STDP gate. Front. Synaptic Neurosci. 2, 146. doi:10.3389/fnsyn.2010.00146
Pedrosa, V., and Clopath, C. (2017). The role of neuromodulators in cortical plasticity. A computational perspective. Front. Synaptic Neurosci. 8 (JAN), 38. doi:10.3389/fnsyn.2016.00038
Peterchev, A. V., Wagner, T. A., Miranda, P. C., Nitsche, M. A., Paulus, W., Lisanby, S. H., et al. (2012). Fundamentals of transcranial electric and magnetic stimulation dose: definition, selection, and reporting practices. Brain Stimul. 5, 435–453. doi:10.1016/j.brs.2011.10.001
Piché, M., Uchida, S., Hara, S., Aikawa, Y., and Hotta, H. (2010). Modulation of somatosensory-evoked cortical blood flow changes by GABAergic inhibition of the nucleus basalis of Meynert in urethane-anaesthetized rats. J. Physiology 588 (12), 2163–2171. doi:10.1113/jphysiol.2010.187633
Plataki, M., Sands, S. A., and Malhotra, A. (2013). Clinical consequences of altered chemoreflex control. Respir. Physiology Neurobiol. 189, 354–363. doi:10.1016/j.resp.2013.04.020
Putman, P., Verkuil, B., Arias-Garcia, E., Pantazi, I., and Schie, C. V. (2014). EEG theta/beta ratio as a potential biomarker for attentional control and resilience against deleterious effects of stress on attention. Cognitive, Affect. and Behav. Neurosci. 14 (2), 782–791. doi:10.3758/s13415-013-0238-7
Ramanathan, D., Tuszynski, M. H., and Conner, J. M. (2009). The basal forebrain cholinergic system is required specifically for behaviorally mediated cortical map plasticity. J. Neurosci. 29 (18), 5992–6000. doi:10.1523/JNEUROSCI.0230-09.2009
Ramanathan, D. S., Conner, J. M., Anilkumar, A. A., and Tuszynski, M. H. (2015). Cholinergic systems are essential for late-stage maturation and refinement of motor cortical circuits. J. Neurophysiology 113 (5), 1585–1597. doi:10.1152/jn.00408.2014
Redgrave, J. N., Moore, L., Oyekunle, T., Ebrahim, M., Falidas, K., Snowdon, N., et al. (2018). Transcutaneous auricular vagus nerve stimulation with concurrent upper limb repetitive task practice for poststroke motor recovery: a pilot study. J. Stroke Cerebrovasc. Dis. 27 (7), 1998–2005. doi:10.1016/j.jstrokecerebrovasdis.2018.02.056
Reimer, J., McGinley, M. J., Liu, Y., Rodenkirch, C., Qi, W., McCormick, D. A., et al. (2016). Pupil fluctuations track rapid changes in adrenergic and cholinergic activity in cortex. Nat. Commun. 7 (November), 13289. doi:10.1038/ncomms13289
Riccio, A., Simione, L., Schettini, F., Pizzimenti, A., Inghilleri, M., Belardinelli, M. O., et al. (2013). Attention and P300-based BCI performance in people with amyotrophic lateral sclerosis. Front. Hum. Neurosci. 7, 732. doi:10.3389/fnhum.2013.00732
Rodenkirch, C., Carmel, J. B., and Wang, Q. (2022). Rapid effects of vagus nerve stimulation on sensory processing through activation of neuromodulatory systems. Front. Neurosci. 16, 922424. doi:10.3389/fnins.2022.922424
Rogers, R. F., Paton, J. F., and Schwaber, J. S. (1993). NTS neuronal responses to arterial pressure and pressure changes in the rat. Am. J. Physiology-Regulatory, Integr. Comp. Physiology 265 (6), R1355–R1368. doi:10.1152/ajpregu.1993.265.6.R1355
Rufener, K. S., Wienke, C., Salanje, A., Haghikia, A., and Zaehle, T. (2023). Effects of transcutaneous auricular vagus nerve stimulation paired with tones on electrophysiological markers of auditory perception. Brain Stimul. 16 (4), 982–989. doi:10.1016/j.brs.2023.06.006
Ruiz, A. D., Malley, K. M., Danaphongse, T. T., Ahmad, F. N., Mota Beltran, C., Rennaker, R. L., et al. (2023b). Effective delivery of vagus nerve stimulation requires many stimulations per session and many sessions per week over many weeks to improve recovery of somatosensation. Neurorehabilitation Neural Repair 37 (9), 652–661. doi:10.1177/15459683231197412
Ruiz, A. D., Malley, K. M., Danaphongse, T. T., Ahmad, F. N., Mota Beltran, C., White, M. L., et al. (2023a). Vagus nerve stimulation must occur during tactile rehabilitation to enhance somatosensory recovery. Neuroscience 532 (November), 79–86. doi:10.1016/j.neuroscience.2023.09.015
Sawaki, L., Werhahn, K. J., Barco, R., Kopylev, L., and Cohen, L. G. (2003). Effect of an α1-adrenergic blocker on plasticity elicited by motor training. Exp. Brain Res. 148 (4), 504–508. doi:10.1007/s00221-002-1328-x
Scangos, K. W., Khambhati, A. N., Daly, P. M., Makhoul, G. S., Sugrue, L. P., Zamanian, H., et al. (2021). Closed-loop neuromodulation in an individual with treatment-resistant depression. Nat. Med. 27 (10), 1696–1700. doi:10.1038/s41591-021-01480-w
Schuerman, W. L., Nourski, K. V., Rhone, A. E., Howard, M. A., Chang, E. F., and Leonard, M. K. (2021). Human intracranial recordings reveal distinct cortical activity patterns during invasive and non-invasive vagus nerve stimulation. Sci. Rep. 11 (1), 22780. doi:10.1038/s41598-021-02307-x
Sclocco, R., Garcia, R. G., Kettner, N. W., Isenburg, K., Fisher, H. P., Hubbard, C. S., et al. (2019). The influence of respiration on brainstem and cardiovagal response to auricular vagus nerve stimulation: a multimodal ultrahigh-field (7T) fmri study. Brain Stimul. 12 (4), 911–921. doi:10.1016/j.brs.2019.02.003
Serradj, N., Marino, F., Moreno-López, Y., Bernstein, A., Sydney, A., Marwa, S., et al. (2023). Task-specific modulation of corticospinal neuron activity during motor learning in mice. Nat. Commun. 14 (1), 2708. doi:10.1038/s41467-023-38418-4
Shaffer, F., and Ginsberg, J. P. (2017). An Overview of heart rate variability metrics and norms. Front. Public Health 5, 258. doi:10.3389/fpubh.2017.00258
Shahriari, B., Swersky, K., Wang, Z., Adams, R. P., and Freitas, N. de (2016). Taking the human out of the loop: a review of bayesian optimization. Proc. IEEE 104 (1), 148–175. doi:10.1109/JPROC.2015.2494218
Song, J. H. (2019). The role of attention in motor control and learning. Curr. Opin. Psychol. 29, 261–265. doi:10.1016/j.copsyc.2019.08.002
Souza, R. R., Oleksiak, C. R., Tabet, M. N., Rennaker, R. L., Hays, S. A., Kilgard, M. P., et al. (2021a). Vagus nerve stimulation promotes extinction generalization across sensory modalities. Neurobiol. Learn. Mem. 181 (May), 107425. doi:10.1016/j.nlm.2021.107425
Souza, R. R., Robertson, N. M., McIntyre, C. K., Rennaker, R. L., Hays, S. A., and Kilgard, M. P. (2021b). Vagus nerve stimulation enhances fear extinction as an inverted-U function of stimulation intensity. Exp. Neurol. 341 (July), 113718. doi:10.1016/j.expneurol.2021.113718
Spyer, K. M. (1982). Central nervous integration of cardiovascular control. J. Exp. Biol. 100 (1), 109–128. doi:10.1242/jeb.100.1.109
Świerżyńska, E., Oręziak, A., Główczyńska, R., Rossillo, A., Grabowski, M., Szumowski, Ł., et al. (2023). Rate-responsive cardiac pacing: technological solutions and their applications. Sensors 23, 1427. doi:10.3390/s23031427
Swinnen, B. E. K. S., Lotfalla, V., Scholten, M. N., Prins, R. H. N., Goes, K. M., de Vries, S., et al. (2024). Programming algorithm for the management of speech impairment in subthalamic nucleus deep brain stimulation for Parkinson’s disease. Neuromodulation 27 (3), 528–537. doi:10.1016/J.NEUROM.2023.05.002
Taylor, P. C. J., Vincent, W., and Eimer, M. (2008). Combining TMS and EEG to study cognitive function and cortico-cortico interactions. Behav. Brain Res. 191, 141–147. doi:10.1016/j.bbr.2008.03.033
Thompson, S. L., O’Leary, G. H., Austelle, C. W., Gruber, E., Kahn, A. T., Manett, A. J., et al. (2021). A review of parameter settings for invasive and non-invasive vagus nerve stimulation (VNS) applied in neurological and psychiatric disorders. Front. Neurosci. 15, 709436. doi:10.3389/fnins.2021.709436
Tseng, C. T., Jackson, B., Gaulding, S. J., Hassan, B. S., and Thorn, C. A. (2020). Vagus nerve stimulation promotes cortical reorganization and reduces task-dependent calorie intake in male and female rats. Brain Res. 1748 (December), 147099. doi:10.1016/j.brainres.2020.147099
Tyler, R., Cacace, A., Stocking, C., Tarver, B., Engineer, N., Martin, J., et al. (2017). Vagus nerve stimulation paired with tones for the treatment of tinnitus: a prospective randomized double-blind controlled pilot study in humans. Sci. Rep. 7 (1), 11960. doi:10.1038/s41598-017-12178-w
Urbin, M. A., Ozdemir, R. A., Tazoe, T., and Perez, M. A. (2017). Spike-timing-dependent plasticity in lower-limb motoneurons after human spinal cord injury. J. Neurophysiology 118 (4), 2171–2180. doi:10.1152/jn.00111.2017
Verma, A. K., Nandakumar, B., Acedillo, K., Yu, Y., Marshall, E., Schneck, D., et al. (2024). Slow-wave sleep dysfunction in mild parkinsonism is associated with excessive beta and reduced delta oscillations in motor cortex. Front. Neurosci. 18 (February), 1338624. doi:10.3389/fnins.2024.1338624
Verma, A. K., Ying, Yu, Acosta-Lenis, S. F., Tyler, H., Sanabria, D. E., Molnar, G. F., et al. (2023). Parkinsonian daytime sleep-wake classification using deep brain stimulation lead recordings. Neurobiol. Dis. 176 (January), 105963. doi:10.1016/j.nbd.2022.105963
Verma, N., Mudge, J. D., Kasole, M., Chen, R. C., Blanz, S. L., Trevathan, J. K., et al. (2021). Auricular vagus neuromodulation—a systematic review on quality of evidence and clinical effects. Front. Neurosci. 15, 664740. doi:10.3389/fnins.2021.664740
Verrienti, G., Raccagni, C., Lombardozzi, G., De Bartolo, D., and Iosa, M. (2023). Motivation as a measurable outcome in stroke rehabilitation: a systematic review of the literature. Int. J. Environ. Res. Public Health 20, 4187. doi:10.3390/ijerph20054187
Vuckovic, A., Hasan, M. A., Osuagwu, B., Fraser, M., Allan, D. B., Conway, B. A., et al. (2015). The influence of central neuropathic pain in paraplegic patients on performance of a motor imagery based brain computer interface. Clin. Neurophysiol. 126 (11), 2170–2180. doi:10.1016/j.clinph.2014.12.033
Wachtel, I., Ohno, S., Koo, S., Boyse, G. C., Eichwald, E. A., Silmser, E. J., et al. (1975). “Modulation of visual cortical plasticity by acetylcholine and noradrenaline,” in Immunogenetics. Vol. 257. Editors U. H. Wiberg, and E. Giinther (Company of Biologists).
Ward, N. S., Brown, M. M., Thompson, A. J., and Frackowiak, R. S. J. (2003). Neural correlates of motor recovery after stroke: a longitudinal FMRI study. Brain 126 (11), 2476–2496. doi:10.1093/brain/awg245
Warren, C. M., Tona, K. D., Ouwerkerk, L., van Paridon, J., Poletiek, F., van Steenbergen, H., et al. (2019). The neuromodulatory and hormonal effects of transcutaneous vagus nerve stimulation as evidenced by salivary alpha amylase, salivary cortisol, pupil diameter, and the P3 event-related potential. Brain Stimul. 12 (3), 635–642. doi:10.1016/j.brs.2018.12.224
Wendt, K., Denison, T., Foster, G., Krinke, L., Thomson, A., Wilson, S., et al. (2022). Physiologically informed neuromodulation. J. Neurological Sci. 434 (March), 120121. doi:10.1016/j.jns.2021.120121
Wu, D., Ma, J., Zhang, L., Wang, S., Tan, B., and Jia, G. (2020). Effect and safety of transcutaneous auricular vagus nerve stimulation on recovery of upper limb motor function in subacute ischemic stroke patients: a randomized pilot study. Neural Plast. 2020, 1–9. doi:10.1155/2020/8841752
Yerkes, R. M., and Dodson, J. D. (1908). The relation of strength of stimulus to rapidity of habit-formation. J. Comp. Neurology Psychol. 18 (5), 459–482. doi:10.1002/cne.920180503
Yoshida, T., Otaka, Y., Kitamura, S., Ushizawa, K., Kumagai, M., Jun, Y., et al. (2023). Influence of motivation on rehabilitation outcomes after subacute stroke in convalescent rehabilitation wards. Front. Neurology 14, 1185813. doi:10.3389/fneur.2023.1185813
Zanos, S., Rembado, I., Chen, D., and Fetz, E. E. (2018). Phase-locked stimulation during cortical beta oscillations produces bidirectional synaptic plasticity in awake monkeys. Curr. Biol. 28 (16), 2515–2526.e4. doi:10.1016/j.cub.2018.07.009
Ziemann, U., Iliać, T. V., Pauli, C., Meintzschel, F., and Ruge, D. (2004). Learning modifies subsequent induction of long-term potentiation-like and long-term depression-like plasticity in human motor cortex. J. Neurosci. 24 (7), 1666–1672. doi:10.1523/JNEUROSCI.5016-03.2004
Zoccal, D. B., Werner, I. F., Bassi, M., Colombari, D. S. A., and Colombari, E. (2014). The nucleus of the solitary tract and the coordination of respiratory and sympathetic activities. Front. Physiology 5, 238. doi:10.3389/fphys.2014.00238
Keywords: vagus nerve stimulation, cognitive state, neuromodulator, cardiorespiratory rhythms, targeted plasticity, neuromodulation, personalized therapy, physiological control systems
Citation: Nandakumar B, Mylavarapu RV, Harris R, Albuquerque ER, Yan Z, Herter C, McMillan DW, Kanumuri VV and Ganzer PD (2024) State dependent vagus nerve stimulation for targeted plasticity therapy: challenges and considerations. Front. Control. Eng. 5:1452442. doi: 10.3389/fcteg.2024.1452442
Received: 20 June 2024; Accepted: 20 September 2024;
Published: 11 October 2024.
Edited by:
Gautam Kumar, San Jose State University, United StatesReviewed by:
Victor Pikov, Medipace Inc, United StatesCopyright © 2024 Nandakumar, Mylavarapu, Harris, Albuquerque, Yan, Herter, McMillan, Kanumuri and Ganzer. This is an open-access article distributed under the terms of the Creative Commons Attribution License (CC BY). The use, distribution or reproduction in other forums is permitted, provided the original author(s) and the copyright owner(s) are credited and that the original publication in this journal is cited, in accordance with accepted academic practice. No use, distribution or reproduction is permitted which does not comply with these terms.
*Correspondence: Patrick D. Ganzer, cHhnNDg3QG1pYW1pLmVkdQ==
Disclaimer: All claims expressed in this article are solely those of the authors and do not necessarily represent those of their affiliated organizations, or those of the publisher, the editors and the reviewers. Any product that may be evaluated in this article or claim that may be made by its manufacturer is not guaranteed or endorsed by the publisher.
Research integrity at Frontiers
Learn more about the work of our research integrity team to safeguard the quality of each article we publish.