- 1Department of Electrical and Electronic Engineering, Yonsei University, Seoul, South Korea
- 2Departments of Molecular and Cellular Biology and Applied Mathematics and Biomedical Engineering, University of Arizona, Tucson, AZ, United States
Human-Biology Interaction (HBI) is a field that aims to provide first-hand experience with living matter and the modern life-sciences to the lay public. Advances in optical, bioengineering, and digital technologies as well as interaction design now also enable real and direct experiences at the microscale, such as with living cells and molecules, motivating the sub-field of “micro-HBI.” This is distinct from simulating any biological processes. There is a significant need for HBI as new educational modalities are required to enable all strata of society to become informed about new technologies and biology in general, as we face challenges like global pandemics, environmental loss, and species extinctions. Here we review this field in order to provide a jump-off point for future work and to bring stakeholder from different disciplines together. By now, the field has explored and demonstrated many such interactive systems, the use of different microorganisms, new interaction design principles, and versatile applications, such as museum exhibits, biotic games, educational cloud labs, citizen science platforms, and hands-on do-it-yourself (DIY) Bio maker activities. We close with key open questions for the field to move forward.
Introduction and Concepts
Humans exist in a close relationship with nature, and various inter-species interactions with macroscopic animals and plants have long enriched our lives. Meanwhile, modern life sciences and biotechnology are dramatically transforming our society—similar to the impact of information technology over the past few decades (Riedel-Kruse et al., 2011). These advancements provide new opportunities for humans to access living systems such as cells at the microscopic scale. Moreover, such first-hand experiences are also needed to inform the general public about biotechnology, life-sciences, medicine and ecology, and the current COVID-19 pandemic or loss of terrestrial biodiversity (Riedel-Kruse et al., 2011; Lockee, 2021). Hence Human-Biology Interaction (HBI) (Figure 1A) has emerged as a new interdisciplinary field (Lee et al., 2015a), conceptually related to Human-Computer Interaction (HCI), which aims to provide the general public with first-hand experiences of living matter (Figure 1B). While interactions with macroscopic living organisms (e.g., plants and animals) is often straightforward and requires minimal technology, interactions between humans and microscopic living matter such as protists, bacteria, viruses and DNA is accomplished with the help of modern optical, biological and digital technologies that facilitate inter-scale and cross-modality interactions. These conceptual and technical aspects merit particular attention and have therefore motivated the sub-field of “micro-HBI.” Note that these works are distinctly different from simulations and virtual realities (Loparev et al., 2017), as the presence and interaction of truly living biological material is at its core (Riedel-Kruse et al., 2011), while concepts of augmented and mixed reality are incorporated (Lam et al., 2020).

Figure 1. Overview of key concepts of micro-HBI: (A) Interactive Microbiology: Human actions affect microbiological systems such as cells, and their responses are delivered back to humans; these interactions typically require mediation through digital, optical and bioengineering technologies (Riedel-Kruse et al., 2011; Gerber et al., 2016b). (B) Example of an interactive installation that allows a human to interact in real-time with the living microorganism Euglena gracilis by projecting the human body outline as light into the microscope and eliciting a photophobic Euglena response (red arrow), and all of which is then projected onto a larger screen to provide interactive feedback to the human (Lam et al., 2019; Lee et al., 2020). (C) Concept of the biotic processing unit (BPU) (Gerber et al., 2016b) that provides well-defined digital interaction with the microbiological system, i.e., a biological system (Euglena cell) is housed and can be stimulated and measured. Via a control computer, a user interface enables real-time interaction between human and microbiological systems; interaction design guidelines are therefore important to provide a robust and desired user experience (Gerber et al., 2016b).
The early works in the domain stem from a longstanding BioArt tradition, where many artworks were created from the artist's interaction with microscopic biological matter like bacteria (Osthoff, 2001) or cultured cells (Bakkum et al., 2007). In addition, in the HCI field, the concept of living media (Cheok et al., 2008) was introduced to augment digital systems by using biological matter as a user-interface modality. Then, direct and real-time interactions between human users and microorganisms and/or biochemical processes were proposed and also demonstrated in the form of biotic games for entertainment and education (Riedel-Kruse et al., 2011). In the meantime, citizen science platforms such as EteRNA enabled playful interactions with RNA folding questions while also providing experimental feedback within a few days (Lee et al., 2014; Koepnick et al., 2019). During the development of micro-HBI, the framework of biotic processing units (BPU) (Figure 1C) enabled more flexible and robust engineering and design for exploring various applications such as museum exhibits (Lee et al., 2015a) and cloud labs (Hossain et al., 2015), eventually leading to a first set of best practices regarding HBI design, technology and ethics (Harvey et al., 2014; Gerber et al., 2016b).
Since then, the field has been growing rapidly with an increasing number of stakeholders from various disciplines. HBI systems for various purposes have been pursued including, but not limited to, education (Hossain et al., 2016; Washington et al., 2019), entertainment (Kim et al., 2018a; van Eck and Lamers, 2018), art and installations (Kuznetsov et al., 2018; Lam et al., 2019; Lee et al., 2020) and as a new modality for interaction (Alistar and Pevere, 2020; Merritt et al., 2020; Pataranutaporn et al., 2020; Ofer et al., 2021). In addition, dedicated user studies for HBI have been undertaken (Hossain et al., 2017b; Lam et al., 2019), providing more insight into the design of micro-HBI systems. We also witnessed a number of papers proposing related and overlapping concepts and terminology (some supported by practical work, while others were rather conceptual), for example, “Internet of Biotic Things (IoBT)” (Kim et al., 2018b), “Living Bits” (Pataranutaporn et al., 2020), “BioBits” (Huang et al., 2018; Stark et al., 2018), interactive biodesign (Gough et al., 2021), empathetic living media (Cheok et al., 2008) and living media interfaces (Merritt et al., 2020).
Due to the interdisciplinary nature of the field, the growth has often been horizontal rather than building upon each other. The field is becoming rich in concepts and speculative designs, making it difficult to obtain a comprehensive view of what has already been previously accomplished. In addition, advances in enabling technologies, design methodologies based on thorough user studies, and practical applications and use-cases are comparably overlooked. In this short review, we aim to outline the key concepts of micro-HBI, and we introduce and highlight some noteworthy work in the field as identified through an in-depth literature search. To move the field forward, we focus this review on the enabling technologies, design insights and ethical guidelines, ultimately suggesting future directions.
Notable prior work
To list and introduce notable prior works in micro-HBI, we categorized them based on the application types and the end goals (Figure 2). We identified at least four major (partially overlapping) applications areas, i.e., education and scientific inquiry, art and play, human-computer interactions, and living and programmable materials.
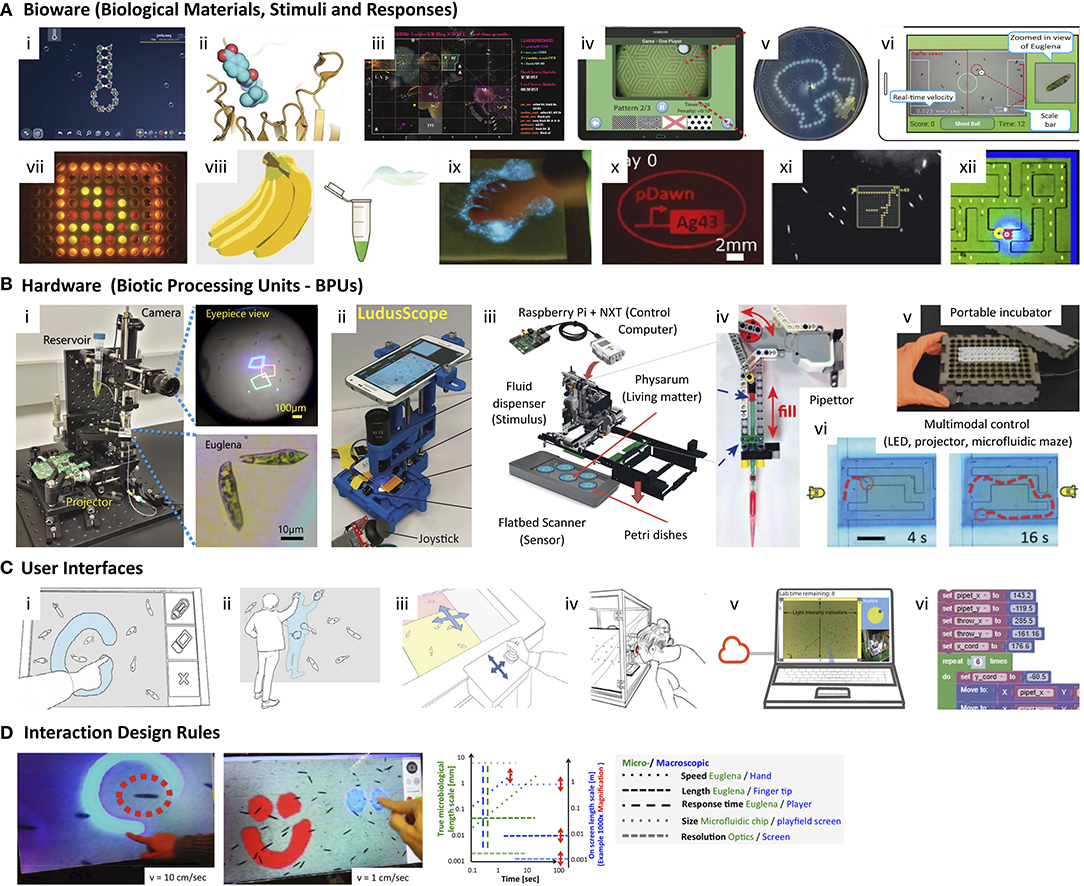
Figure 2. Key aspects of micro-HBI and illustrative examples: (A) Bioware (Biological materials including stimuli and responses): (i) RNA folding (EteRNA) (Lee et al., 2014); (ii) Protein folding (Foldit) (Eiben et al., 2012); (iii) Fungi growth (Mould Rush) (Kim et al., 2019); (iv) Euglena (swarm) photo-bioconvection (Biographer) (Gerber et al., 2016a); (v) Physarum chemotaxis (Turn-based cloud lab) (Hossain et al., 2015); (vi) Euglena (multicell) phototaxis (LudusScope) (Kim et al., 2016); (vii) In vitro fluorescent protein expression (BioBits) (Stark et al., 2018); (viii) Chemical detection through smell and fluorescence (BioBits) (Huang et al., 2018); (ix) Mechanical fluorescent activation with dinoflagellates (Hopscotch game) (Ofer et al., 2021); (x) Bacterial deposition via light (Biofilm Lithography) (Jin and Riedel-Kruse, 2018); (xi) Paramecium galvanotaxis (biotic games) (Riedel-Kruse et al., 2011); (xii) Euglena (single cell) phototaxis (microfluidic Pac-Man maze) (Lam et al., 2020). (B) Hardware (“biotic processing units”-BPUs): (i) Light-projector and optics microscopy (Lee et al., 2015a); (ii) LEDs and low-cost smartphone microscope (Kim et al., 2016); (iii) LEGO pipetting robot and flatbed scanner (Hossain et al., 2015); (iv) LEGO pipettor (Gerber et al., 2017); (v) Portable incubator/plate reader (Stark et al., 2018); (vi) Multimodal swarm programming (LED, projector, microfluidic maze) (Lam et al., 2017). (C) User Interfaces: (i) Touchscreen (Lam et al., 2019); (ii) Full-body interaction (Lam et al., 2019); (iii) Microscope operation (Lam et al., 2019); (iv) Passive observation (Lam et al., 2019); (v) Web-based user interface for cloud lab (Hossain et al., 2016); (vi) Direct programming interface for liquid handler control (Gome et al., 2019). (D) Interaction Design Rules: Specific design rules need to be followed to match the time and length scales of the macroscopic human actions with the microscopic biological responses (Gerber et al., 2016b).
The integration of real biological experiments and modeling for educational and scientific inquiry purposes was achieved with real-time interactive and turn-based cloud labs (Hossain et al., 2015, 2016). These systems were accessed in K-12 and university class-room settings as well as through massive open online courses (MOOCs) (Hossain et al., 2016, 2017a; Hossain and Riedel-Kruse, 2018) for their educational outcomes. In hands-on settings, such activities can also be viewed as augmented, interactive microscopy (Kim et al., 2016), contrasting the traditional passive (including the more recent virtual) educational microscopy (Wilson et al., 2016). The citizen science games such as EteRNA and Foldit enabled folding predictions of RNA and protein molecules with real experimental feedback (Lee et al., 2014; Das et al., 2019). Do-it-yourself biology (DIY bio), synthetic biology such as “BioBits” and low-cost equipment provide accessible platforms for education, engineering biological matter and artistic expression (Kuznetsov et al., 2012; Huang et al., 2018; Stark et al., 2018).
Various artistic and playful applications have been demonstrated and others have been proposed (“conceptual design”), many of which have also overlapped the same system or even within the same activity: Open-ended play and rule-based play (Riedel-Kruse et al., 2011; Lee et al., 2015a; Kim et al., 2016; Lam et al., 2020), museum or public installations (Lee et al., 2015a, 2020; Lam et al., 2019), bioart (Kuznetsov et al., 2018), and sonification of microbiological behavior (Riedel-Kruse et al., 2011).
In the HCI field, many digital systems also continued to employ microbes as a part of the interface media (Alistar and Pevere, 2020; Merritt et al., 2020). In these examples, the major purpose of living media was to reinforce psychological factors and enrich the human user's experience. Cheok et al. (2008) showed that living matter can promote human empathy during engagement with digital systems, and Alistar and Pevere (2020) proposed using bacteria as tangible media to deliver information using human senses such as smell and touch. Beyond the interface media, efforts to develop digital systems have focused on the inter-species interaction between humans and microbes for ecological, educational and entertainment purposes (Chen et al., 2021; Ofer et al., 2021). These systems take the non-anthropocentric design approach that includes microbial life as an important stakeholder in the system rather than as a component of the system to be controlled and utilized (Lee et al., 2020).
Many other applications have been considered, such as interactive biocomputation, swarm robotics (Lam et al., 2017; Washington et al., 2019) and sensing, which further connect to the fields of hybrid living and living smart materials (Pataranutaporn and Lyle, 2018; Smith et al., 2020) and where self-assembly (Glass and Riedel-Kruse, 2018) or biofilm lithography (Jin and Riedel-Kruse, 2018) of adhesive cells may provide micro-LEGO bricks. Additionally, a wide range of more macroscopic applications exist such as wearables and clothing (Yao et al., 2015; Nguyen et al., 2021) as well as hybrid living materials (Smith et al., 2020). Other speculative future applications include food and health applications (Huang et al., 2018; Stark et al., 2018).
Microbiology, Technologies, and Interfaces
The design of interactive microbiology systems needs to focus on at least three major components, i.e., the biological subject matter (“bioware”) (Figure 2A), the biotic processing units that both maintain and interface with the biological matters (“hardware”) (Figure 2B), and the interaction modalities and interfaces for humans (“user interface”) (Figure 2C).
Different types of microscopic materials and stimulus modalities (“bioware,” Figure 2A) have been demonstrated single-celled organisms such as Euglena (Hossain et al., 2016), paramecia (Riedel-Kruse et al., 2011), dinoflagellates (Ofer et al., 2021) and bacteria (Alistar and Pevere, 2020; Chen et al., 2021), multicelled organisms such as the slimemold physarum (Hossain et al., 2015), molecules like RNA and DNA (Stojanovic and Stefanovic, 2003; Riedel-Kruse et al., 2011; Lee et al., 2014), cell collectives (Riedel-Kruse et al., 2011; Gerber et al., 2016a), and fungi (Kim et al., 2019) have been incorporated, and others such as viruses (Kim, 2021) have been proposed. Stimulus modalities included chemicals (Riedel-Kruse et al., 2011; Hossain et al., 2015), light (Lee et al., 2015a; Hossain et al., 2016), and electric fields (Bakkum et al., 2007; Riedel-Kruse et al., 2011), and mechanical mechanisms (Ofer et al., 2021). Euglena gracilis (Figures 1B,C) has been proven to be particularly amenable for real-time HBI as it is easy to purchase and culture long term. Furthermore, it has a very robust directional negative phototaxis movement (swimming speed of ~50 μm/s, reaction time of ~0.5 s) as well as many other interesting responses to various light stimuli (Gerber et al., 2016a; Lam et al., 2017; Tsang et al., 2018), its comparably large (length of ~50 μm) and colorful characteristics facilitate an easy and pleasant microscopy experience, and it is already in wide educational use (Oswald and Kwiatkowski, 2011). When choosing biological materials to work with, a number of considerations should be followed (Gerber et al., 2016b), especially regarding the desired response type, response time and response robustness for the desired interactivity; the particular application, e.g., education or art, also further defines these choices. As a stimulus, light is particularly advantageous given its speed and ease of control (Lam et al., 2017). While genetically engineered or “synthetic organisms” have not yet been appreciably deployed toward HBI (Huang et al., 2018), they hold promise for tailoring desired interactions even further.
The biological material needs to be housed, maintained, stimulated, and observed (“hardware,” Figure 2B). Therefore, it is necessary to distinguish between hands-on and one-time only activities with logistics similar to classic educational experiments (Gerber et al., 2016a; Kim et al., 2016) and long-term robust activities spanning over days to months that subsequently require a significant degree of robustness and automation (Lee et al., 2015a; Hossain et al., 2016; Lam et al., 2019). In particular, microfluidic technology (Merrin, 2019; Rackus et al., 2019) with various state measurements on the quality of the biological material, feedback to correct for the desired behavior, and parallelization of multiple systems have been proven to be effective over weeks in a demonstrated real-time interactive biology cloud lab (Hossain et al., 2016; Hossain and Riedel-Kruse, 2018). Nevertheless, further improvements are desirable. Overall, advances in open source electronics, such as Arduino and Raspberry Pi and simple webcam microscopes and associated optics provide low-cost and effective technologies for setting up such systems (Hossain et al., 2016; Kim et al., 2016). Microfluidic technologies are not yet as accessible and user-friendly as DIY digital and electronic technologies, although they are steadily improving (Gerber et al., 2015; Rackus et al., 2019). More complex spatio-temporal motion control of cells has been achieved through structured microfluidic chips (such as a Pac-Man maze) and spatial light fields (Lam et al., 2017, 2020). Robotics technology based on LEGO or DIY systems is also enabling (Hossain et al., 2015; Gerber et al., 2017; Gome et al., 2019; Fuhrmann et al., 2021); i.e., turn-based biology cloud labs with activities spanning days have been demonstrated utilizing such robots (Hossain et al., 2015). Ultimately, it would be desirable to establish biotic processing units (BPUs) (Figure 1C) that house microbiological material and enable its stimulation and observation through well-defined, digital interaction channels — in close analogy to electronic central processing units (CPUs) or graphics processing units (GPUs) (Gerber et al., 2016b; Lam et al., 2017; Washington et al., 2019).
Many interface modalities for human users have been demonstrated (“user interface,” Figure 2C). These modalities include touch screens (Lee et al., 2015a,b; Lam et al., 2019), smartphones (Kim et al., 2016), joysticks (Riedel-Kruse et al., 2011; Kim et al., 2016), full-body and motion detection (Lam et al., 2019; Lee et al., 2020), and web-interfaces (Hossain et al., 2015, 2016). Accessibility to different user groups has been achieved through museum installations (Lee et al., 2015a), cloud labs (Hossain et al., 2016), and hands-on DIY approaches (Kim et al., 2016). In these various forms of interaction, the system may guide human users to perform designed activities under predefined rules and purposes (e.g., games, experiments), or the system can also be configured to mediate free-form interactions and open play where users may need to devise their own ways to experience the system (Lee et al., 2015a). Regarding human senses, most interactions utilize natural human visual perceptions, but others have been explored such as smell (Riedel-Kruse et al., 2011; Huang et al., 2018) or sound (indirectly through sonification) (Riedel-Kruse et al., 2011). Different interaction time scales were demonstrated, ranging from real-time interactions (Lee et al., 2015a; Hossain et al., 2016; Kim et al., 2016) to longer timescales, such as the slow biotic game “Mould Rush” (Kim et al., 2018b) and turn-based cloud labs (Hossain et al., 2015).
User Studies, Design Insights, and Best Practices
A growing list of specific interaction design rules have been established (Gerber et al., 2016b; Kim et al., 2020) (Figure 2D). Some of the micro-HBI systems have already been paired with user studies, some even in a controlled fashion. Most of these studies focused on formal and informal education (Kuznetsov et al., 2013; Lee et al., 2015a; Hossain et al., 2016, 2017b; Lam et al., 2019). A few themes and corresponding evidence emerged from these studies: Attitude changes toward science, improved access to biology experimentation for poorer and underprivileged communities through cloud labs, accessibility even for small children due to touchscreens or full-body experiences, and stimulation of prolonged engagement and interactions among multiple users. Users expressed agency and playfulness (“I liked playing around with the online microscope”), and valued the real biology interactions compared to simulations (and the synergistic combination of both) (“using a real microscope is more exciting than using a computer simulation”). A more dedicated comparison museum study (Lam et al., 2019) investigated different interaction modalities with microbial biology, and reported a positive interest in these biological cells due to direct microbial interactions via full-body experiences or touchscreens, whereas the more traditional approach of only controlling the microscope with a joystick even led to loss of interest for some users.
A unique aspect of HBI is the variability (randomness, noise) that exist in the biological matter. Due to this biological variability, not all cells respond identically to the same stimuli, and the responses also vary over time, which can be viewed as glitches in the system but can also serve to entertain and to further convey that this is a real biological system and not a simulation (Kim et al., 2019; Lee et al., 2020). In designing user activities with clear goals (such as a game), striking a balance to control these variations to enable a robust interaction experience while also utilizing them to convey a sense of realness and authenticity to the biological processes is necessary.
HBI also raises potential ethical questions to the designers and the users. These concerns have significant conceptual overlap with other bioethical discussions (Harvey et al., 2014), such as the ethical principles established in the domain of plant/animal interactions (Mancini, 2011; Aspling et al., 2016) and the ethics discussions in the BioArt domain (Stracey, 2009). Related arguments have also been made regarding a non-anthropocentric design approach for human-plant interactions (Fell et al., 2020). The raised concerns often also stem from a misunderstanding of the microscopic biological subject matter (Harvey et al., 2014). Given the non-sentient nature of these microbiological systems, many of these concerns are usually straightforward to address. Nevertheless, these concerns should be taken seriously when designing such systems to engage the public in a positive and supportive manner (Harvey et al., 2014; Merritt et al., 2020; Pataranutaporn et al., 2020; Gough et al., 2021).
Overall, these user studies as well as various exploratory projects revealed a number of prominent features compared to existing modalities to engage with microbiology; in particular, these user studies subsequently revealed a growing set of design rules and general design principles that should be considered when macroscopic humans interact with microscopic living matter (Gerber et al., 2016b; Fell et al., 2020; Merritt et al., 2020; Pataranutaporn et al., 2020; Gough et al., 2021). In addition to more general HCI design principles (Shneiderman, 2016), the following warrant consideration: (i) Biological behavior and responses need to be sufficiently robust, (ii) the interactive time and length scales between microscopic biology and macroscopic humans need to be matched (Figure 2D), (iii) the biological variability should be managed but also embraced as a feature, (iv) the activities should convey that this is real biology and not a simulation, (v) potential safety and ethical aspects need to be addressed, and (iv) the specific audience and application needs to be considered.
Discussion and Open Questions
The field of micro-HBI is now well over a decade old, with the number of contributors from different fields steadily increasing. Significant advancements have been made regarding technology and interaction design. Furthermore, a large application and design space has been explored (Figure 2). These detailed developments are driven for multiple reasons; i.e., technology is advancing (such as DIY microfluidics, electronics and optics), the field has achieved a large number of demonstration and use cases to highlight its versatility, creative potential and overall feasibility have been demonstrated, and the need for society-wide formal and informal education on these subject matters is established. We suggest that the field should now move beyond conceptual papers and speculative design and focus on more practical advancements and real world applications. In particular, recommendations include enabling technology that is practical, robust, and accessible, and that enables versatile design; endeavors aiming to solve practical needs, such as large-scale education, while also including future business models; and actual user studies that are performed to drive design principles. We conclude key focus points that would drive the field forward:
1. Can we have HBI systems that fully automate long-term and robust interactivity with microbes? (Hossain et al., 2016)
2. Can we safely and ethically use synthetic or genetically modified organisms? (Stark et al., 2018)
3. How can we manufacture, deploy and even personalize HBI systems at scale? (Hossain et al., 2017b)
4. Can HBI have the same social and economic impact as predecessor technologies such as electronic video games? (Gerber et al., 2016b)
5. Can HBI make contributions for citizen science? (Lee et al., 2014; Das et al., 2019)
6. Can HBI implement more complex genres of inorganic counterparts, such as real-time strategy games? (Das et al., 2019)
7. Can we have a commercially available (general purpose) platform (“BPU”) that is accessible and upon which others can design, e.g., a mini-game or Tamagotchi-like system? (Gerber et al., 2016b)
8. How can we more effectively bring different fields of expertise together, especially bioengineers, microbiologists, human-computer interaction designers, as well as stakeholders such as educators or the gaming industry? Should there be a dedicated conference on this subject matter?
In conclusion, the argument has been made that micro-HBI might undergo a similar exponential path as its computational counterparts (Gerber et al., 2016b). However, whether that will happen likely significantly depends on standardized and easy-to-use technology of sufficient robustness (e.g., BPU-Figure 1) that would open a large design space, enable killer applications (such as medical diagnostics), and support the engagement and curiosity of scientists/engineers, artists, and the general public.
Author Contributions
Both authors listed have made a substantial, direct, and intellectual contribution to the work and approved it for publication.
Funding
Funding was provided by the College of Science and the MCB Department at the University of Arizona (IR-K), and by the National Research Foundation of Korea (NRF) grant funded by the Korean government (MSIT) (no. 2021R1C1C101290011) (SL).
Conflict of Interest
The authors declare that the research was conducted in the absence of any commercial or financial relationships that could be construed as a potential conflict of interest.
Publisher's Note
All claims expressed in this article are solely those of the authors and do not necessarily represent those of their affiliated organizations, or those of the publisher, the editors and the reviewers. Any product that may be evaluated in this article, or claim that may be made by its manufacturer, is not guaranteed or endorsed by the publisher.
Acknowledgments
We are grateful to A. Hamby and G. Day for feedback and discussion on earlier versions of the manuscript.
References
Alistar, M., and Pevere, M. (2020). Semina aeternitatis: using bacteria for tangible interaction with data, in Extended Abstracts of the 2020 CHI Conference on Human Factors in Computing Systems, (Honolulu, HI), 1–13.
Aspling, F., Wang, J., and Juhlin, O. (2016). Plant-computer interaction, beauty and dissemination, in Proceedings of the Third International Conference on Animal-Computer Interaction, (Milton Keynes), 1–10.
Bakkum, D. J., Gamblen, P. M., Ben-Ary, G., Chao, Z. C., and Potter, S. M. (2007). Meart: the semi-living artist. Front. Neurorobot. 1, 2007. doi: 10.3389/neuro.12.005.2007
Chen, D., Seong, Y. A., Ogura, H., Mitani, Y., and Sekiya, N. (2021). Nukabot: design of care for human-microbe relationships, in Extended Abstracts of the 2021 CHI Conference on Human Factors in Computing Systems, (Yokohama), 1–7.
Cheok, A. D., Kok, R. T., Tan, C., Newton Fernando, O. N., Merritt, T., and Sen, J. Y. P. (2008). Empathetic living media, in Proceedings of the 7th ACM Conference on Designing Interactive Systems, (Cape Town: ACM), 465–473.
Das, R., Keep, B., Washington, P., and Riedel-Kruse, I. H. (2019). Scientific discovery games for biomedical research. Ann. Rev. Biomed. Data Sci. 2, 253–279. doi: 10.1146/annurev-biodatasci-072018-021139
Eiben, C. B., Siegel, J. B., Bale, J. B., Cooper, S., Khatib, F., Shen, B. W., et al. (2012). Increased diels-alderase activity through backbone remodeling guided by foldit players. Nat. Biotechnol. 30, 190–192. doi: 10.1038/nbt.2109
Fell, J., Greene, T., Wang, J.-C., and Kuo, P.-Y. (2020). Beyond human-centered design: proposing a biocentric view on design research involving vegetal subjects, in Companion Publication of the 2020 ACM Designing Interactive Systems Conference, (Eindhoven), 209–214.
Fuhrmann, T., Ahmed, D. I., Arikson, L., Wirth, M., Miller, M. L., Li, E., et al. (2021). Scientific inquiry in middle schools by combining computational thinking, wet lab experiments, and liquid handling robots, in Interaction Design and Children, (Athens), 444–449.
Gerber, L. C., Calasanz-Kaiser, A., Hyman, L., Voitiuk, K., Patil, U., and Riedel-Kruse, I. H. (2017). Liquid-handling lego robots and experiments for stem education and research. PLoS Biol. 15, e2001413. doi: 10.1371/journal.pbio.2001413
Gerber, L. C., Doshi, M. C., Kim, H., and Riedel-Kruse, I. H. (2016a). Biographr: Science games on a biotic computer, in Proceedings of the First Joint International Conference on Digital Games Research Assotiation and Foundation of Digital Games DiGRA/FDG (Dundee).
Gerber, L. C., Kim, H., and Riedel-Kruse, I. H. (2015). Microfluidic assembly kit based on laser-cut building blocks for education and fast prototyping. Biomicrofluidics 9, 064105. doi: 10.1063/1.4935593
Gerber, L. C., Kim, H., and Riedel-Kruse, I. H. (2016b). Interactive biotechnology: design rules for integrating biological matter into digital games, in DiGRA/FDG (Dundee).
Glass, D. S., and Riedel-Kruse, I. H. (2018). A synthetic bacterial cell-cell adhesion toolbox for programming multicellular morphologies and patterns. Cell 174, 649–658. doi: 10.1016/j.cell.2018.06.041
Gome, G., Waksberg, J., Grishko, A., Wald, I. Y., and Zuckerman, O. (2019). Openlh: open liquid-handling system for creative experimentation with biology, in Proceedings of the Thirteenth International Conference on Tangible, Embedded, and Embodied Interaction, TEI '19 (New York, NY: Association for Computing Machinery), 55–64.
Gough, P., Yoo, S., Tomitsch, M., and Ahmadpour, N. (2021). Applying bioaffordances through an inquiry-based model: a literature review of interactive biodesign. Int. J. Hum. Comput. Interact. 37, 1583–97. doi: 10.1080/10447318.2021.1898846
Harvey, H., Havard, M., Magnus, D., Cho, M. K., and Riedel-Kruse, I. H. (2014). Innocent fun or “microslavery”? an ethical analysis of biotic games. Hastings Center Rep. 44, 38–46. doi: 10.1002/hast.386
Hossain, Z., Bumbacher, E., Blikstein, P., and Riedel-Kruse, I. (2017a). Authentic science inquiry learning at scale enabled by an interactive biology cloud experimentation lab, in Proceedings of the Fourth (2017) ACM Conference on Learning@ Scale, (Cambridge, MA: ACM), 237–240.
Hossain, Z., Bumbacher, E., Brauneis, A., Diaz, M., Saltarelli, A., Blikstein, P., et al. (2017b). Design guidelines and empirical case study for scaling authentic inquiry-based science learning via open online courses and interactive biology cloud labs. Int. J. Artif. Intell. Educ. 28, 478–507. doi: 10.1007/s40593-017-0150-3
Hossain, Z., Bumbacher, E. W., Chung, A. M., Kim, H., Litton, C., Walter, A. D., et al. (2016). Interactive and scalable biology cloud experimentation for scientific inquiry and education. Nat. Biotechnol. 34, 1293–1298. doi: 10.1038/nbt.3747
Hossain, Z., Jin, X., Bumbacher, E. W., Chung, A. M., Koo, S., Shapiro, J. D., et al. (2015). Interactive cloud experimentation for biology: An online education case study, in Proceedings of the 33rd Annual ACM Conference on Human Factors in Computing Systems (Seoul: ACM), 3681–3690.
Hossain, Z., and Riedel-Kruse, I.H. (2018). Life-science experiments online: technological frameworks and educational use cases, in Cyber-Physical Laboratories in Engineering and Science Education, eds M. Auer, A. Azad, A. Edwards, and T. de Jong (Cham: Springer). doi: 10.1007/978-3-319-76935-6_11
Huang, A., Nguyen, P. Q., Stark, J. C., Takahashi, M. K., Donghia, N., Ferrante, T., et al. (2018). Biobits explorer: a modular synthetic biology education kit. Sci. Adv. 4, eaat5105. doi: 10.1126/sciadv.aat5105
Jin, X., and Riedel-Kruse, I. H. (2018). Biofilm lithography enables high-resolution cell patterning via optogenetic adhesin expression. Proc. Natl. Acad. Sci. U.S.A. 115, 3698–3703. doi: 10.1073/pnas.1720676115
Kim, H., Gerber, L. C., Chiu, D., Lee, S. A., Cira, N. J., Xia, S. Y., et al. (2016). Ludusscope: accessible interactive smartphone microscopy for life-science education. PLoS ONE 11, e0162602. doi: 10.1371/journal.pone.0168053
Kim, R. (2021). Virus as quasi-living bio-material for interaction design: Practical, ethical, and philosophical implications, in Extended Abstracts of the 2021 CHI Conference on Human Factors in Computing Systems, 1–7.
Kim, R., Thomas, S., Dierendonck, R. V., Kaniadakis, A., and Poslad, S. (2018a). Microbial integration on player experience of hybrid bio-digital games, in International Conference on Intelligent Technologies for Interactive Entertainment (Yokohama: Springer), 148–159.
Kim, R., Thomas, S., van Dierendonck, R., Bryan-Kinns, N., and Poslad, S. (2020). Working with nature's lag: Initial design lessons for slow biotic games, in International Conference on the Foundations of Digital Games, (Bugibba), 1–4.
Kim, R., Thomas, S., van Dierendonck, R., and Poslad, S. (2018b). A new mould rush: designing for a slow bio-digital game driven by living micro-organisms, in Proceedings of the 13th International Conference on the Foundations of Digital Games (Malmo), 1–9.
Kim, R., van Dierendonck, R., and Poslad, S. (2019). Moldy ghosts and yeasty invasions: glitches in hybrid bio-digital games, in Extended Abstracts of the 2019 CHI Conference on Human Factors in Computing Systems, (Glasgow), 1–6.
Koepnick, B., Flatten, J., Husain, T., Ford, A., Silva, D.-A., Bick, M. J., et al. (2019). De novo protein design by citizen scientists. Nature 570, 390–394. doi: 10.1038/s41586-019-1274-4
Kuznetsov, S., Barrett, C., Fernando, P., and Fowler, K. (2018). Antibiotic-responsive bioart: exploring diybio as a design studio practice, in Proceedings of the 2018 CHI Conference on Human Factors in Computing Systems (Montreal, QC), 1–14.
Kuznetsov, S., Harrigan-Anderson, W., Faste, H., Hudson, S. E., and Paulos, E. (2013). Community engagements with living sensing systems, in Proceedings of the 9th ACM Conference on Creativity and Cognition, Candamp;C '13 (New York, NY: Association for Computing Machinery), 213–222.
Kuznetsov, S., Taylor, A. S., Regan, T., Villar, N., and Paulos, E. (2012). At the seams: diybio and opportunities for hci, in DIS '12 (New York, NY: Association for Computing Machinery), 258-267.
Lam, A. T., Griffin, J., Loeun, M. A., Cira, N. J., Lee, S. A., and Riedel-Kruse, I. H. (2020). Pac-euglena: a living cellular pac-man meets virtual ghosts, in Proceedings of the 2020 CHI Conference on Human Factors in Computing Systems (Honolulu, HI), 1–13.
Lam, A. T., Ma, J., Barr, C., Lee, S. A., White, A. K., Yu, K., et al. (2019). First-hand, immersive full-body experiences with living cells through interactive museum exhibits. Nat. Biotechnol. 37, 1238–1241. doi: 10.1038/s41587-019-0272-2
Lam, A. T., Samuel-Gama, K. G., Griffin, J., Loeun, M., Gerber, L. C., Hossain, Z., et al. (2017). Device and programming abstractions for spatiotemporal control of active micro-particle swarms. Lab. Chip. 17, 1442–1451. doi: 10.1039/C7LC00131B
Lee, J., Kladwang, W., Lee, M., Cantu, D., Azizyan, M., Kim, H., et al. (2014). Rna design rules from a massive open laboratory. Proc. Natl. Acad. Sci. U.S.A. 111, 2122–2127. doi: 10.1073/pnas.1313039111
Lee, K., Jung, J., and Lee, S. A. (2020). Microaquarium: an immersive and interactive installation with living microorganisms, in Extended Abstracts of the 2020 CHI Conference on Human Factors in Computing Systems, CHI EA '20 (New York, NY: Association for Computing Machinery), 1–4.
Lee, S. A., Bumbacher, E., Chung, A. M., Cira, N., Walker, B., Park, J. Y., et al. (2015a). Trap it!: a playful human-biology interaction for a museum installation, in Proceedings of the 33rd Annual ACM Conference on Human Factors in Computing Systems, (New York, NY: ACM), 2593–2602.
Lee, S. A., Chung, A. M., Cira, N., and Riedel-Kruse, I. H. (2015b). Tangible interactive microbiology for informal science education, in Proceedings of the Ninth International Conference on Tangible, Embedded, and Embodied Interaction (New York, NY: ACM), 273–280.
Lockee, B. B. (2021). Online education in the post-covid era. Nat. Electr. 4, 5–6. doi: 10.1038/s41928-020-00534-0
Loparev, A., Westendorf, L., Flemings, M., Cho, J., Littrell, R., Scholze, A., et al. (2017). Bacpack: exploring the role of tangibles in a museum exhibit for bio-design, in Proceedings of the Eleventh International Conference on Tangible, Embedded, and Embodied Interaction, TEI '17 (New York, NY: Association for Computing Machinery), 111–120.
Mancini, C. (2011). Animal-computer interaction: a manifesto. Interactions 18, 69–73. doi: 10.1145/1978822.1978836
Merrin, J. (2019). Frontiers in microfluidics, a teaching resource review. Bioengineering 6, 109. doi: 10.3390/bioengineering6040109
Merritt, T., Hamidi, F., Alistar, M., and DeMenezes, M. (2020). Living media interfaces: a multi-perspective analysis of biological materials for interaction. Digital Creativity 31, 1–21. doi: 10.1080/14626268.2019.1707231
Nguyen, P. Q., Soenksen, L. R., Donghia, N. M., Angenent-Mari, N. M., de Puig, H., Huang, A., et al. (2021). Wearable materials with embedded synthetic biology sensors for biomolecule detection. Nat. Biotechnol. 39, 1366–1374. doi: 10.1038/s41587-021-00950-3
Ofer, N., Bell, F., and Alistar, M. (2021). Designing direct interactions with bioluminescent algae. Design. Interact. Syst. Conf. 2021, 1230–1241. doi: 10.1145/3461778.3462090
Osthoff, S. (2001). Eduardo Kac”s Genesis: Biotechnology Between the Verbal, the Visual, the Auditory, and the Tactile. Cambridge, MA: MIT Press.
Oswald, C., and Kwiatkowski, S. (2011). Population growth in euglena: a student-designed investigation combining ecology, cell biology, and quantitative analysis. Am. Biol. Teach. 73, 469–473. doi: 10.1525/abt.2011.73.8.8
Pataranutaporn, P., and Lyle, K. (2018). Toward human-magic interaction: interfacing biological, tangible, and cultural technology, in HCI International 2018 - Posters' Extended Abstracts, ed C. Stephanidis (HCI International).
Pataranutaporn, P., Vujic, A., Kong, D. S., Maes, P., and Sra, M. (2020). Living bits: opportunities and challenges for integrating living microorganisms in human-computer interaction, in Proceedings of the Augmented Humans International Conference (Kaiserslautern), 1–12.
Rackus, D. G., Riedel-Kruse, I. H., and Pamme, N. (2019). learning on a chip: microfluidics for formal and informal science education. Biomicrofluidics 13, 041501. doi: 10.1063/1.5096030
Riedel-Kruse, I. H., Chung, A. M., Dura, B., Hamilton, A. L., and Lee, B. C. (2011). Design, engineering and utility of biotic games. Lab. Chip 11, 14–22. doi: 10.1039/C0LC00399A
Shneiderman, B., Plaisant, C., Cohen, M. S., Jacobs, S., Elmqvist, N., and Diakopoulos, N. (2016). Designing the User Interface: Strategies for Effective Human-Computer Interaction. 6th Edn. Availalble online at: https://nsuworks.nova.edu/gscis_facbooks/18
Smith, R. S. H., Bader, C., Sharma, S., Kolb, D., Tang, T.-C., Hosny, A., et al. (2020). Hybrid living materials: digital design and fabrication of 3d multimaterial structures with programmable biohybrid surfaces. Adv. Funct. Mater. 30, 1907401. doi: 10.1002/adfm.201907401
Stark, J. C., Huang, A., Nguyen, P. Q., Dubner, R. S., Hsu, K. J., Ferrante, T. C., et al. (2018). Biobits bright: a fluorescent synthetic biology education kit. Sci. Adv. 4, eaat5107. doi: 10.1126/sciadv.aat5107
Stojanovic, M. N., and Stefanovic, D. (2003). A deoxyribozyme-based molecular automaton. Nat. Biotechnol. 21, 1069–1074. doi: 10.1038/nbt862
Stracey, F. (2009). Bio-art: the ethics behind the aesthetics. Nat. Rev. Mol. Cell Biol. 10, 496. doi: 10.1038/nrm2699
Tsang, A. C., Lam, A. T., and Riedel-Kruse, I. H. (2018). Polygonal motion and adaptable phototaxis via flagellar beat switching in euglena gracilis. bioRxiv 292896. doi: 10.1101/292896
van Eck, W., and Lamers, M. H. (2018). Mapping the field of organism-involved computer games, in Proceedings of the 13th International Conference on the Foundations of Digital Games (Malmo), 1–8.
Washington, P., Samuel-Gama, K. G., Goyal, S., Ramaswami, A., and Riedel-Kruse, I. H. (2019). Interactive programming paradigm for real-time experimentation with remote living matter. Proc. Natl. Acad. Sci. U.S.A. 116, 5411–5419. doi: 10.1073/pnas.1815367116
Wilson, A. B., Taylor, M. A., Klein, B. A., Sugrue, M. K., Whipple, E. C., and Brokaw, J. J. (2016). Meta-analysis and review of learner performance and preference: virtual versus optical microscopy. Med. Educ. 50, 428–440. doi: 10.1111/medu.12944
Keywords: Human-Biology Interaction (HBI), microbiology, cloud labs, biotic games, human-computer interaction design, bioengineering, cells
Citation: Lee SA and Riedel-Kruse IH (2022) Micro-HBI: Human-Biology Interaction With Living Cells, Viruses, and Molecules. Front. Comput. Sci. 4:849887. doi: 10.3389/fcomp.2022.849887
Received: 06 January 2022; Accepted: 06 April 2022;
Published: 16 May 2022.
Edited by:
Stacey Kuznetsov, Arizona State University, United StatesReviewed by:
Raphael Kim, Delft University of Technology, NetherlandsCopyright © 2022 Lee and Riedel-Kruse. This is an open-access article distributed under the terms of the Creative Commons Attribution License (CC BY). The use, distribution or reproduction in other forums is permitted, provided the original author(s) and the copyright owner(s) are credited and that the original publication in this journal is cited, in accordance with accepted academic practice. No use, distribution or reproduction is permitted which does not comply with these terms.
*Correspondence: Seung Ah Lee, c2V1bmdhaGxlZSYjeDAwMDQwO3lvbnNlaS5hYy5rcg==; Ingmar H. Riedel-Kruse, aW5nbWFyJiN4MDAwNDA7YXJpem9uYS5lZHU=
†These authors have contributed equally to this work