- 1Faculty of Environment, Science and Economy, University of Exeter, Exeter, United Kingdom
- 2Laboratoire de Météorologie Dynamique, Institut Pierre-Simon Laplace, CNRS, École Normale Supérieure, Université PSL, Sorbonne Université, École Polytechnique, Paris, France
- 3Center for Amazonian Sustainability, University of São Paulo, São Paulo, Brazil
- 4Institute of Atmospheric Physics, Chinese Academy of Sciences, Beijing, China
- 5Met Office Hadley Centre, Exeter, United Kingdom
- 6School of Geographical Sciences, University of Bristol, Bristol, United Kingdom
- 7Japan Agency for Marine-Earth Science and Technology (JAMSTEC), Yokohama, Japan
- 8Advanced Institute for Marine Ecosystem Change, Tohoku University, Sendai, Japan
- 9Department of Geography, University of Nevada Reno, Reno, NV, United States
- 10Past Global Changes (PAGES), Bern, Switzerland
- 11Department of Geosciences, Geo- and Environmental Research Center, University of Tübingen, Tübingen, Germany
- 12Department of Environmental Sciences, Informatics and Statistics, Ca’ Foscari University of Venice, Venice, Italy
- 13MARUM, Center for Marine Environmental Sciences, University of Bremen, Bremen, Germany
- 14School of Earth and Environment, University of Leeds, Leeds, United Kingdom
- 15International Institute for Applied Systems Analysis, Laxenburg, Austria
- 16CNRM, Université de Toulouse, Météo-France, CNRS, Toulouse, France
- 17World Climate Research Programme Secretariat, WMO, Geneva, Switzerland
Anthropogenic carbon dioxide (CO2) emissions are the main driver of climate change, with global warming increasing almost linearly with cumulative CO2 emissions. Hence, future warming will primarily result from future emissions of CO2 with contributions from other greenhouse gases (mostly CH4 and N2O) and aerosols. Climate projections of the 21st century, such as those assessed by the IPCC, are provided from comprehensive climate models, also called Earth System models, driven by scenarios of the 21st century evolution of emissions from those climate forcers. While it seems now inevitable that the world will reach 1.5°C of warming above pre-industrial levels by the early 2030s, the extent to which we exceed this warming level and how quickly we may be able to reduce temperatures again depends strongly on global activity taken now to limit emissions. In this paper, we review the current understanding on Earth system changes under two highly contrasted possible future worlds. We first focus on high-end scenarios, where anthropogenic emissions continue to increase over the course of the 21st century, leading to large warming levels, associated impacts on all components of the Earth System, and increased risks of triggering tipping points. We then assess low-end scenarios, where anthropogenic emissions rapidly decline, reaching net zero and potentially becoming net negative before the end of the 21st century. Such “overshoot” scenarios lead to a peak in global warming followed by a slow decline in global temperature, with some degree of reversibility in the global carbon cycle and key Earth system components. We also review paleoclimatic information relevant to these two contrasting future worlds. Paleoclimate evidence for geo-biosphere interactions shows that stabilizing feedbacks operate on millennial or longer timescales, whereas destabilizing feedbacks and tipping cascades occurred also on shorter timescales.
1 Introduction
Over millions of years, the Earth has experienced large changes in its global climate, driven by changes in natural forcings, changes in the planet’s orbital configuration relative to the sun (and associated changes in solar radiation), and changes in geologic activity (and associated changes in atmospheric composition). These initial changes would generally trigger further changes in vegetation cover, ice sheet extent and the global carbon cycle that would amplify (or slow down) the initial perturbation. This is well recorded in past reconstructions of the Earth’s climate and atmospheric composition.
From the Paleocene (~60 million years ago) to the Pleistocene (~2.3 million years ago) the Earth’s climate transitioned profoundly from a hothouse to a coolhouse. This period is characterised by a decline from high atmospheric CO2 (1,000–2,000 ppm) to much lower CO₂ concentrations (250–400 ppm) in 50 million years. Planetary cooling co-occurred with the glaciation of Antarctica ~34 million years ago at the Eocene–Oligocene transition (Lear et al., 2008). Recent work identified an associated major change in ocean circulation (Coxall et al., 2018), opening up a possibility for cascading tipping points during that climate transition (Dekker et al., 2018; Wunderling et al., 2024). These could include transitions in the water and carbon cycles, via changes in ocean circulation and carbon storage, continental weathering, terrestrial biomes and carbon storage (Ladant et al., 2018). These transition periods of Earth climate are of high interest to understand the future Earth response as it represents one of the few documented paleo periods where CO₂ declined and hence provides insights on how the Earth’s climate would respond to declining CO₂ emissions.
During the Pleistocene, the Earth experienced alternances of colder glacial and warmer interglacial periods, transitioning between them by small changes in orbital forcing, amplified by ice sheet extensions, and biogeochemical feedbacks resulting in lower concentrations of the main greenhouse gases (CO₂, CH4, N2O) during glacial than during interglacial periods. Since the beginning of the Holocene, 11,700 years ago, the global mean temperature of the Earth has been more stable than in the Glacial at the global and regional scale (Rehfeld et al., 2018). The atmospheric composition of the main greenhouse gases was around 280 ppm for CO₂, 700 ppb for CH4 and 270 ppb for N2O towards the preindustrial end of the Holocene (Canadell et al., 2021).
From the beginning of the Industrial period, around 1750, the increasing use of fossil fuel resources as the main source of energy and the human appropriation of land for croplands and pastures led to rapid increases in greenhouse gases concentrations, with current levels and rates of changes unprecedented over at least 800,000 years (Masson-Delmotte, 2021).
Since 1750, about 710 GtC (2,600 GtCO2) have been emitted into the atmosphere from human activities, primarily from fossil fuel burning (69%), and also from land use and land cover change (31%) (Friedlingstein et al., 2023). About 45% of anthropogenic CO₂ emissions remain in the atmosphere, leading to the observed increase in atmospheric CO₂ concentration, reaching 419 ppm in 2023 (Friedlingstein et al., 2023). However, a large fraction of the CO₂ emissions (about 55%) is absorbed by the oceans and land ecosystems. These land and ocean carbon sinks are primarily driven by an atmospheric CO₂ concentration increase, with climate change being understood to reduce the strength of these carbon sinks. Over the same historical period, atmospheric CH4 more than doubled, from around 700 ppb in 1750 to about 1,920 ppb in 2023 (Lan et al., 2022), primarily from anthropogenic emissions from agriculture, waste management, and fossil-fuel-related activities (Saunois et al., 2020), but also potential global warming induced increase in natural sources (Canadell et al., 2021; Feng et al., 2022).
As assessed by the Sixth Assessment Report (AR6) of the Intergovernmental Panel on Climate Change (IPCC), each of the last four decades has been successively warmer than any decade that preceded it since 1850. The global surface temperature was 1.19 [1.06 to 1.30]°C higher in 2014–2023 than the 1850–1900 reference period (Forster et al., 2024), with 2023 being the warmest year on record, close to 1.5°C warmer than 1,850–1,900 (Copernicus, 2023).
In this paper, we review the current understanding on future Earth system changes under two highly contrasted possible worlds: high-end scenarios, with further increase in anthropogenic emissions over the course of the 21st century; and low-end scenarios, where anthropogenic emissions rapidly reaching net zero and potentially becoming net negative before the end of the 21st century.
2 Future climate scenarios
2.1 Design of emissions scenarios
Future scenarios assessed by climate science are not predictions of how society will evolve but provide a range of possible futures. It is a societal choice which pathway we try to follow. Climate science and impacts modelling allow us to see the implications of these choices with the aim to guide us to a sustainable 21st century and beyond. Emissions scenarios are often produced using Integrated Assessment Models (IAMs). IAMs are typically optimization models that have mathematical representations of the global economy, energy systems and land-use and agricultural sectors, solving a global system, maximising economic welfare or minimising system costs subject to constraints. Inputs to IAMs are provided by exogenous socioeconomic narratives, such as the Shared Socioeconomic Pathways (SSPs), which specify regional-level population, Gross Domestic Product (GDP), urbanisation, and education pathways to 2,100 (Riahi et al., 2017). Constraints implemented in IAMs may include climate constraints, such as a total allowable cumulative carbon budget acting as a proxy for temperature or year-2100 radiative forcing. An end-of-century radiative forcing constraint is the basis of the well-known Representative Concentration Pathways (RCPs) for the Couple Model Intercomparison Project Phase 5 (CMIP5) (Taylor et al., 2012) and SSP-RCPs for CMIP Phase 6 (CMIP6) (Eyring et al., 2016), examples being RCP4.5 and SSP2-4.5 where the year-2100 radiative forcing is limited to 4.5 W m−2 (O’Neill et al., 2016). Land use patterns provided by IAMs can also provide boundary conditions for Earth System Models (ESMs) that have a dynamic representation of vegetation cover distribution.
Previously, physical climate models were always run with pre-calculated concentrations of greenhouse gases (GHGs) in future scenarios. However, the development of ESMs, that fully resolve the physical climate system and its coupling with land and ocean carbon cycles, allows for a more realistic forcing with CO2 emissions (Friedlingstein et al., 2006; Hajima et al., 2024). For CMIP Phase 7 (CMIP7), there is a desire that CO₂ emissions-driven becomes the default configuration (Sanderson et al., 2023). For the IPCC AR6, around a dozen ESMs resolved land and ocean carbon cycles and could be driven by CO2 emissions (Arora et al., 2020). These models are incredibly useful for determining the Earth system response to increasing CO₂ emissions, but also the Earth System response to zero or negative emissions (Jones et al., 2016; MacDougall et al., 2020). A few models now have methane emissions-driven capability (Folberth et al., 2022), but other greenhouse gases must be provided with pre-calculated concentrations (Smith and Gasser, 2022). Hence, most climate model experiments that investigate future scenarios, as assessed by IPCC, are run with prescribed concentrations for all GHGs, even in those ESMs that have emissions-driven capability (Sanderson et al., 2023). The GHG concentrations for input into ESMs are calculated using simpler climate models (Meinshausen et al., 2011; Smith et al., 2018) from the IAM-simulated GHGs future emissions pathways.
2.2 How can we define high-end or low-end scenarios?
In the early days of scenario-building such as those from the Special Report on Emissions Scenarios (SRES) (Nakicenovic et al., 2000), “business as usual” tended to denote pathways with constant or increasing greenhouse gas emissions in the future leading to several degrees Celsius of warming in 2100. This continued with the development of the RCPs, where RCP8.5, the highest emissions scenario used for ESMs in CMIP5 and AR5, was described as “business as usual” by its developers (Riahi et al., 2011). Although CO₂ emissions globally are not yet declining, a shift away from coal in favour of gas, and rapid scale up of renewable energy globally, has slowed the growth of CO2 emissions. To track the highest SSP-RCP emissions scenario prepared for CMIP6 and assessed in the IPCC AR6, SSP5-8.5, would require a large-scale reversal back to coal power, which appears to be unlikely. It has been argued that emissions scenarios such as SSP5-8.5 no longer represent “business as usual” (Hausfather and Peters, 2020), as they would now require an unprecedented acceleration in fossil fuel production. In addition, the SSP5-85 frames an amount of CO2 emitted by human activities by the end of the century that exceeds the amount of fossil fuel reserves as assessed by IPCC AR6 (Canadell et al., 2021). Nevertheless, climate outcomes that are representative of these very high emissions scenarios could still occur, should carbon cycle feedback be strong, climate sensitivity high, or some Earth system tipping thresholds crossed (Sarofim et al., 2023).
Low-end scenarios have typically become more ambitious throughout time. Early scenarios such as the SRES sets did not create pathways that were expected to limit global mean surface temperature to below 2°C. Increasingly, scientific and policy discourse was coalescing on 2°C as a warming level that should be avoided to prevent the most catastrophic impacts of climate change (Jaeger and Jaeger, 2011), and therefore a renewed focus on plausible low-end scenarios resulted in IAM groups investigating these trajectories. In time for the IPCC Fifth Assessment Report (AR5), several such scenarios were developed, which for the first time seriously considered the necessary net negative CO2 emissions in the second half of the 21st century. This includes RCP2.6, a scenario for Earth System models that limited warming to 2°C with net negative CO2 emissions around 2070 (van Vuuren et al., 2011). Risk assessment of climate impacts still requires knowledge of plausible low-likelihood high-impact outcomes, and this includes higher than expected climate forcing from human activity (Wood et al., 2023).
The 26th Conference of Parties (COP26), held in Paris in 2015, advocated for a more stringent warming limit, with “holding the increase in the global average temperature to well below 2°C above pre-industrial levels and pursuing efforts to limit the temperature increase to 1.5°C above pre-industrial levels.” New scenarios were required that implemented even deeper mitigation, requiring CO2 emissions reductions of 50% by 2030 relative to 2010, net zero emissions by 2050, and strong action on short-lived climate forcers such as methane and black carbon. In some circumstances, the definition of 1.5°C-consistent allows for a small, temporary overshoot of this warming level, acknowledging the geophysical and socioeconomic difficulties in avoiding peak warming exceeding 1.5°C (Dvorak et al., 2022). The latest scenario designed for CMIP6, SSP1-1.9, is one such representative of this set (O’Neill et al., 2016). Such scenarios typically require large amounts (and rapid deployment) of carbon dioxide removal (CDR) technologies such as direct air capture and bioenergy with carbon capture and storage, but their plausibility at the scales required has been questioned (Anderson and Peters, 2016; Fuss et al., 2018; Smith et al., 2016). IAMs and ESMs are increasingly including specific CDR processes in order to assess their implications, side-effects and co-benefits (Keller et al., 2018; Merfort et al., 2023). Earth system modelling can help establish both the demand for CDR and also the feasibility of supply (Jones, 2022).
2.3 Limitations in scenario design
Scenarios such as the SSP-RCPs describe storylines and mitigation pathways of how society may develop over the course of the 21st century. It is not possible for any scenario to describe the exact emissions and land use pathway that the world will take in the future. There are many unknown unknowns in both the human system and the climate system, and on how society will respond to climate change. Furthermore, the IAMs that are used to construct the scenarios do not include climate change impacts on the rest of the human-Earth system. This is by design in the SSPs but may lead to pathways that while feasible in an IAM may be implausible in the real world. For example, high-end scenarios such as SSP5-8.5 project a warming in excess of 4°C in 2100 relative to pre-industrial (Tebaldi et al., 2021), but continues to grow the economy at 3% per year (Riahi et al., 2017), thus neglecting any negative impacts of climate change on human systems (e.g., health, economy, agriculture, etc.) (Woodard et al., 2019). For low-end scenarios, the feasibility concerns revolve around the plausibility of the rate of emissions reduction that could be achieved in the coming decades and on the rate of deployment and scale of negative emissions technologies (Fuss et al., 2018; Heck et al., 2018; Séférian et al., 2018).
3 Earth system response to high-end scenarios
3.1 Overall climate response
Climate change is primarily driven by anthropogenic CO2 emissions. An advance for the IPCC AR5 was the realisation that global warming is largely scenario independent and approximately linearly related to the total cumulative emissions of CO₂ (Allen et al., 2009; Gregory et al., 2009; Matthews et al., 2009). Other non-CO2 emissions affect the warming, and can contribute to both rapid mitigation efforts and Earth system feedbacks, but as a good approximation the degree of warming we may expect in the future is principally proportional to the total amount of CO2 we emit. This linearity is perhaps surprising and reasons for it are still not fully understood as they include inter-related processes, such as ocean heat and carbon uptake, but also unrelated processes, such as changes in radiative forcing from CO₂ vs. changes in atmospheric CO₂ airborne fraction (Goodwin et al., 2014; MacDougall and Friedlingstein, 2015).
When analysing high-end emissions scenarios, one can approximate the degree of warming expected from the knowledge of the cumulative carbon emissions. The relationship between them is characterised by a metric known as TCRE—the Transient Climate Response to cumulative CO2 Emissions. IPCC AR6 assessed the most likely value of TCRE as 0.45°C per 1,000 GtCO2, with a likely range of 0.27–0.63°C. Given current CO₂ emissions of around 40 GtCO2 per year, the CO₂-induced current warming is expected to be around 0.18°C per decade (neglecting natural climate variability), slightly below the observed rate of warming of about 0.26°C per decade given the warming contribution of non-CO2 greenhouse gases (Forster et al., 2024). By 2100, the high emissions scenarios SSP3-7.0 and SSP5-8.5 have cumulative CO2 emissions, from the year 1850, of 8,120 GtCO2 and 10,590 GtCO2, leading to a warming of 3.7 and 4.8°C, assuming the TCRE central estimate, similar to the IPCC assessed warming (3.6 and 4.4°C respectively). Table 1 shows the approximate expected warming from CO₂ emissions and TCRE along with the more comprehensively assessed estimate from IPCC. The level of agreement is better than the uncertainty in TCRE. This implies that TCRE can be used as a simple and rapid technique to estimate the level of global warming for any scenario by converting from cumulative emissions to a temperature change.

Table 1. Global surface warming and one-sigma range achieved by 2100 and 2300 for three SSP scenarios using the simple TCRE approach, compared with the IPCC AR6 assessed warming (data from Table 4.5 and Tables 4.9 of IPCC AR6 WG1).
3.2 Global carbon cycle response
IPCC AR6 showed that the fraction of emissions absorbed by land and ocean reservoirs decreases for higher levels of emissions. Both land and ocean continue to take up carbon, but the efficiency of the sinks (defined as ratio of emissions taken up) declines. Land and ocean absorb about 70% of anthropogenic CO₂ emissions under the low-end SSP1-1.9 scenario, but less than 40% under the high-end SSP5-8.5 scenario. This decline in carbon sink efficiency is partly due to feedbacks from the climate which inhibits the sink strength, and partly due to the change in rate of anthropogenic emissions (Canadell et al., 2021).
3.2.1 Land carbon cycle response
The land carbon sink is primarily driven by the atmospheric CO₂ increase. Fast processes include vegetation gross primary productivity which respond to elevated CO2 via enhanced photosynthesis and improved water use efficiency. However, productivity and allocation of carbon to long-lived biomass (e.g., wood) can be limited due to nutrient availability. Higher CO2 levels increases the risk of insufficient nutrients to support the allocation of carbon to biomass (Zaehle et al., 2014). While the short-term (5–10 years) response of vegetation to elevated CO2 can be assessed using Free-Air CO2 Enrichment (FACE) experiments (Norby et al., 2005), the long term accumulation of carbon in biomass and soil is still largely uncertain (Liddicoat et al., 2021; Varney et al., 2023a,b). Climate change leads to a reduction of the land sink (relative to a counterfactual with CO₂ increase but no climate change). Warming and/or increased aridity reduces plant photosynthesis, especially in tropical forests, directly reducing the ecosystem’s sink potential. Climate change also leads to a loss of soil carbon due to reduced soil input and enhanced soil decomposition, although soil processes remain poorly represented in models (Varney et al., 2023a,b).
In addition, Earth System Models do not properly represent forest disturbances yet, in particular drought and wildfires and their expected increase in frequency and/or intensity in a warming world. Although forests are still the largest carbon sink on land, the vulnerability of tropical forests is already documented and shows a decline in carbon storage (Hubau et al., 2020; Parry et al., 2022). Parry et al. (2022) show how the inclusion of fire in ESMs has led to their ability to represent possible localised tipping points as climate thresholds in tropical forests are reached. Jones et al. (2009) demonstrated that large-scale changes of ecosystems can take many years or decades to manifest and may be committed at certain levels of warming before they are observed. The IPCC assessed loss of ecosystems in the updated “reasons for concern” assessment with large-scale singular events likely to transition to high risks levels above 2°C of global warming. Conversely, long-term vegetation dynamics may also represent a substantial carbon sink missing from many ESMs in high latitudes as northward migration of the tree line allows long-term increases in terrestrial biomass (Pugh et al., 2018).
In high-end emissions scenarios, the thaw of permafrost carbon is significant, with carbon loss from permafrost projected to be 18 [3–41] GtC per 1°C of global warming by 2,100 (Chadburn et al., 2017). While Earth System Models represent some of the physical processes of permafrost thaw (Koven et al., 2013), they still lack a representation of key processes such as abrupt thaw, hillslope collapse or thermokarst lake expansion (Canadell et al., 2021). In addition to the thawing of organic carbon, this would lead to the release of available nitrogen, which may stimulate plant growth and offset some of the carbon loss (Burke et al., 2022).
3.2.2 Ocean carbon cycle response
The ocean absorbs about 25% of the annual anthropogenic CO₂ emissions (Friedlingstein et al., 2023). This sink is driven by several mechanisms known as pumps. The dominant pump is the solubility pump which primarily arises from the chemical and hydrodynamics properties of CO₂ to dissolve into seawater. The large-scale ocean circulation and mixing redistributes the dissolved CO₂ in various ocean basins and across ocean layers. The results of this series of processes generates a difference of partial pressure of CO₂ between the surface ocean and the atmosphere, driving the sign of the ocean physical sink of CO₂, which is the dominant ocean sink over the historical and future period. The second pump is the biological pump which arises from a suite of biological processes such as the primary productivity by phytoplankton, the biological production of calcium carbonate and the sink of biological material to the deep ocean. Although this pump is much smaller than the physical ocean sink, it is more efficient to remove CO₂ from the atmosphere over longer timescales.
Similarly to the land sink, the ocean sink is primarily driven by increasing atmospheric CO₂ which reinforces the difference in partial pressure of CO₂ between the ocean and the atmosphere, strengthening the ocean carbon sink (McKinley et al., 2020). However, warming of the surface ocean reduces the solubility of CO₂ into seawater, thus reducing the carbon sink efficiency. Also, warming reinforces the ocean stratification, slowing down the mixing of water with the deeper ocean and the associated carbon export. In all SSP scenarios the ocean carbon sink persists in the future and grows with increasing atmospheric CO₂ levels, despite the growing negative impact of climate change.
3.3 Ocean acidification
When anthropogenic CO2 dissolves into the ocean, it causes seawater to become more acidic, which then affects organisms especially those that form calcium carbonate shells. The phenomenon is termed ocean acidification, which is progressing steadily on a global scale. Since the late 1980s, the pH of the open ocean surface water has been decreasing at a pace of 0.0166 ± 0.0010 per decade (Ma et al., 2023). Anthropogenic acidification is also found in the deep layers of the ocean, and significant acidification has been detected even at a depth of 1,000 m (Lauvset et al., 2020). The CMIP6 models project that a global average pH decline of 0.44 ± 0.005 from the late 19th century (1870–1899) to the end of the 21st century (2080–2099) under SSP5-8.5, contrasted to 0.16 ± 0.002 for the SSP1-2.6 (Kwiatkowski et al., 2020). If such a large pH decrease is realized in the future, aragonite unsaturation could occur, especially in the polar regions (Figuerola et al., 2021; Yamamoto et al., 2012), with a potential direct impact on aragonite shell-forming organisms. Acidification is also projected to progress in the middle and deep ocean layers, although there are greater uncertainties than in the surface layer due to the contribution of transport processes in ocean circulation (Watanabe and Kawamiya, 2017).
As with the open ocean, coastal areas are also being acidified due to anthropogenic CO2 invasion. In addition, the background biological activity in coastal areas is vigorous, so the amplitude of diurnal and seasonal fluctuations is large. The result is that the pH can be temporarily very low and is more likely to fall below a critical value than in the open ocean (Cornwall et al., 2013). Moreover, relatively small-scale phenomena such as coastal upwelling can cause large pH fluctuations, potentially with a serious impact on fisheries (Feely et al., 2008).
In addition to ocean acidification, consequences of anthropogenic CO2 emissions on the ocean include frequent marine heat waves and oxygen depletion. Although each event affects the marine ecosystem individually, it has been pointed out that strong acidification and oxygen depletion may occur simultaneously in areas where marine heat waves occur (Bindoff et al., 2019; Gruber et al., 2021). Understanding these combined effects, along with a detailed understanding of acidification in mid-deep layers and coastal areas mentioned above, is critically needed.
3.4 Abrupt changes and tipping elements
Although our understanding is that global warming levels increase linearly with emissions, there are likely to be abrupt changes or tipping points in the system, which can happen at local-to-regional scales, not necessarily driving a large acceleration in the rate of climate change, but potentially leading to substantial regional to global impacts (Lenton et al., 2008). In the past transitions occurred at slower rates (see Figure 1) and were driven by biogeochemical feedbacks, paced by evolution and tectonics. The latest assessments suggest that multiple tipping points are at non-negligible levels of risk for global warming as low as 1.5°C (Armstrong McKay et al., 2022). The current rate of radiative forcing increase is about 25 times faster than over the last deglaciation. This implies that slow elements of the Earth system, such as ice sheets, are impacted by high rates that may—or may not—be buffered in the case of an overshoot scenario (Ritchie et al., 2021). At progressively higher warming levels, the likelihood of large-scale singular events to occur increases. Outcomes such as Arctic or Antarctic ice sheets instabilities, an Atlantic Meridional Overturning Circulation (AMOC) collapse, or a dieback of the Amazon Forest are plausible. Still, there is little quantitative understanding of the climate thresholds which may trigger these events. Tipping points may be detected by various techniques, including critical slowing down, with some studies reporting these signals as being detected (Boulton et al., 2022) while other studies reporting no evidence yet (Tao et al., 2023). In the case of the Amazon forest, regional drying and warming, in conjunction with human-land-use/deforestation may push Amazon forest beyond the limits of resilience and lead to a widespread loss of tropical forest (Flores et al., 2024; Lapola et al., 2023). However, how such a threshold may change under elevated CO₂, and associated improved water-use efficiency, remains a critical research gap (Good et al., 2011). As mentioned before, observations are already showing an increase in tree mortality and a decline in the forest carbon sink, which suggests the risk is already present. In the future, projections assessed by IPCC suggest drying in the Amazon forest region—especially during June–July–August, which increases with global temperature and higher scenarios. In such scenarios, tropical forest burned area also increases markedly with up to 30% loss of carbon at 4°C under the highest emissions scenario (Burton et al., 2022).
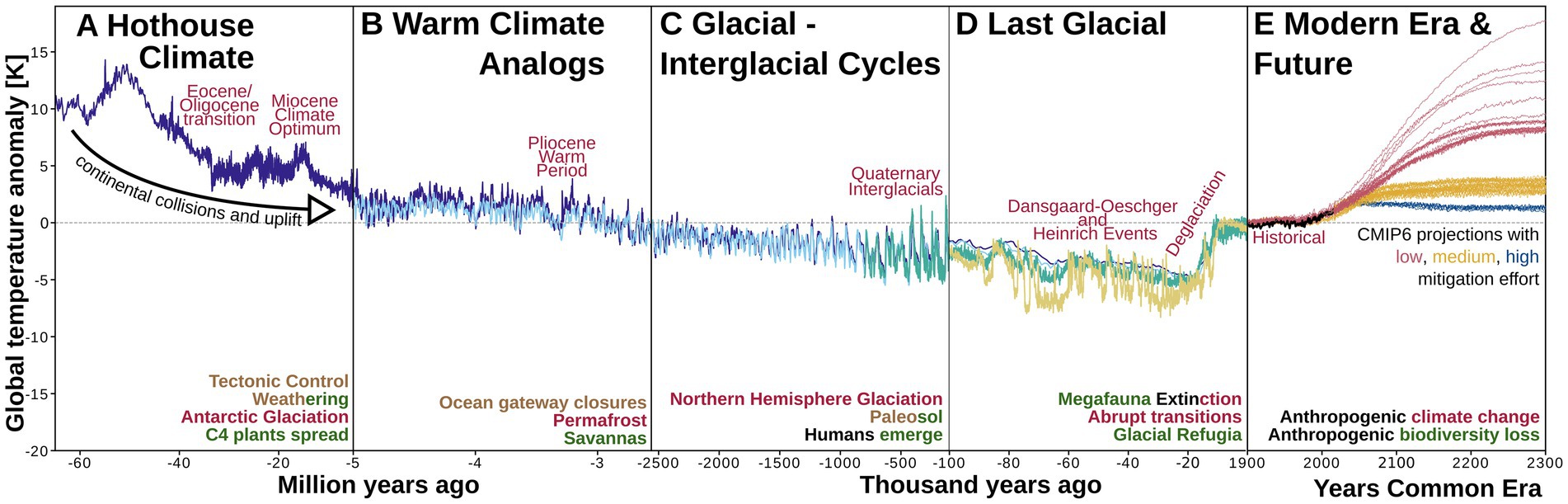
Figure 1. Global mean temperature evolution over the last 60 million years BP to the year 2300 based on paleoclimate reconstructions (panels A, B, C, D), in the context of observations and modelled projections for high (SSP1-1.9), medium (SSP2-45) and low (SSP5-85) mitigation efforts (panel E). Data and calibration details can be found in Rehfeld and Ziegler (2023). Reproduced under CC-By-4.0.
4 Earth system response to low-end scenarios
4.1 Key concepts related to low-end scenarios and climate neutrality
Historically, the family of low-end scenarios emerged in climate assessment at the time of IPCC AR5, where the methodological template of the RCPs accounted for mitigation options in the portfolio of climate solutions (van Vuuren et al., 2011). As such, the introduction of this family of scenarios represented a step change in the provision of future climate projections, contrasting with their predecessors, the SRES scenarios (Nakicenovic et al., 2000).
Contrasting with high-end scenarios, low-end scenarios are defined through several overarching properties that have been assessed in IPCC reports since AR5. The first key property of low-end scenarios is the prominent role of key geophysical constraints, such as the remaining carbon budget. This geophysical quantity is estimated from the total amount of CO2 we can still emit to limit global warming to a certain level while having removed past emissions. The total amount is derived from TCRE. Knowing the current warming level allows to estimate the remaining carbon budget, also accounting for warming from non-CO₂ forcing (Matthews et al., 2020; Rogelj et al., 2016), and the Zero-Emission Commitment (ZEC), i.e., the long-term warming or cooling after CO₂ emissions cease (Jones et al., 2019; MacDougall et al., 2020).
For instance, the IPCC AR6 estimated that the remaining global carbon budget for a 50% chance of staying within 1.5°C was 500 GtCO2 from January 2020 onwards. More recent estimates, accounting for the CO₂ emissions since 2020 and updated estimates of the future warming from non-CO₂ agents, are as low as 200 GtCO2 from January 2024 onwards (Forster et al., 2024). Importantly, this still applies to overshot and CO2 removal, so even if we exceed a target warming level, the concept of a carbon budget helps us plan the scale of action required to return to it.
The fact that low-end scenarios are constrained to a given carbon budget, implies that at a point in time, human-induced CO2 emission will pass from positive to negative. This point in time, when emissions reach “net zero,” represents the second overarching geophysical properties of low-end scenarios. Indeed, the recent IPCC AR6 WGIII report shows that limiting human-caused global warming to a specific level requires limiting cumulative CO2 emissions, i.e., reaching net zero or net negative CO2 emissions, along with strong reductions in other GHG emissions. These low-end scenarios also clarify the difference between net zero CO2 and net zero GHGs, with net zero GHGs (or climate neutrality) requiring all remaining CO2 and non-CO2 GHG emissions to be counterbalanced by durably, ideally permanently, stored CO2 removals.
In the latest IPCC AR6 WGIII scenario database, about 70% of the low-end scenarios halting warming to 2°C deploy net negative CO2 emissions and do not reach net zero GHG emissions by the end of the century.
Beyond “net zero” many scenarios also extend to having globally net-negative emissions of CO2—a case which requires total amounts of CDR to exceed any residual positive emissions from human activity. Net negative emissions are required to enable reductions in global temperature and so-called “overshoot” scenarios. Many scenarios achieving 1.5°C by the end of this century entail periods of overshoot and subsequent net-negative emissions. Different CDR techniques have different consequences, including on land or marine ecosystems, biodiversity, albedo (and regional temperature). Hence we need to consider the concept of “climate neutrality” as well as simply carbon neutrality (Zickfeld et al., 2023).
4.2 Carbon cycle response
Low-end scenarios also helped to challenge an outdated concept where halting global warming only requires stabilised greenhouse gases concentration, not zero emissions. In a geophysical sense, stabilising greenhouse gases concentration would stabilise the radiative forcing from greenhouse gases. This would lead to continuous global warming for centuries because of the slow accumulation of heat in the ocean.
In order to stabilise the climate (i.e., no significant further warming), recent research shows that CO₂ emissions have to cease (MacDougall et al., 2020; Solomon et al., 2009). After CO₂ emissions stop, the ocean and land will continue to absorb the excess CO₂ from the atmosphere, leading to a slow decline in atmospheric CO₂ concentration and, hence, in radiative forcing, which balances the delayed heat uptake by the ocean. The ZEC has been assessed with ESMs in the ZECMIP experiments where ESMs were driven by CO₂ emissions, emitting 1000GtC total before CO₂ emissions were set to zero. 50 years after emissions cease, the simulated ZEC range from −0.36°C to 0.29°C, with a multi-model ensemble mean of −0.07°C, i.e., essentially zero. However, long-term changes in some slow components of the Earth System are expected to continue even when emissions cease, and to be irreversible on human time scales, this is the case for permafrost thaw and carbon release, ice sheets mass loss, and sea level rise.
When CO₂ emissions become negative, via CDR, the land and ocean carbon sinks are expected to further reduce and eventually, potentially turning into CO₂ sources, in particular for the land sinks, driven by the decline in photosynthesis due to decreasing CO₂ concentrations. The ocean on the other hand, is expected to keep absorbing CO₂ for longer (Jones et al., 2016). However, reversing emissions will likely mean reversing global levels of warming. This is quantified by the same TCRE relationship, so every removal of 1,000 GtCO2 will lead to about 0.45°C of cooling. IPCC assesses a level of asymmetry in the CO2 response to negative emissions, but due to the logarithmic dependence of the radiative effect, this means there is likely very little asymmetry in the temperature response. Positive or negative values of the ZEC lead to positive or negative hysteresis behaviour of the global system under negative emissions of CO₂ (Koven et al., 2022).
Although the dominant part of the negative CO2 emissions is assumed to come from the land sector, novel negative CO2 emissions technologies that encompass marine-based CO2 removal are increasingly considered (e.g., Kwiatkowski et al., 2023). Various ocean-based CDR measures have been proposed to mitigate climate change, however, some of the proposed schemes may either promote (nutrient addition) or counteract (alkalinisation) ocean acidification, so it will also be important to also consider CDR from the perspective of ocean acidification.
5 Paleo perspective
5.1 Paleoclimate, CO₂ and sea level
Air bubbles enclosed in ice cores provide direct evidence of past CO2 concentrations (Bereiter et al., 2015; Lüthi et al., 2008). The highest CO2 concentration recorded in polar ice over the past 800,000 years before the industrial era was 300 ppm (Nehrbass-Ahles et al., 2020). Although these values are well below those reached this century, there are at least two periods, the Last Interglacial (LIG, about 125 kyr ago) and Marine Isotope Stage 11 (MIS11, about 400 kyr ago), when sea level likely exceeded current levels (Dutton et al., 2015). Paleo reconstructions of regional sea-level rise rely on evidence for physical, geochemical, and biological features such as coral reefs, shorelines, saltmarshes, or floodmarks that can be dated to the time period of interest. Global sea-level change is derived by combining present and paleo-elevation estimates for regional sea-level with models of glacial isostatic adjustment (Kemp et al., 2015). The first and best studied of these is the LIG when sea level was higher than today, although the exact sea level maximum remains under discussion (Dumitru et al., 2023; Dutton et al., 2015; Dyer et al., 2021). It appears likely that both the Greenland and West Antarctic Ice Sheets were smaller than they are today. The polar warmth experienced in the LIG was comparable to that of the near future. Recent studies suggest that the Greenland Ice Sheet may have lost the ice equivalent to about 3 m of sea level (Sommers et al., 2021), while the West Antarctic Ice Sheet could have lost 4 m of sea level equivalent (Golledge et al., 2021). Insight on sea level can be gained going further back in time to the Pliocene (5 to 3 million years ago), when global mean temperatures was around 4°C higher than today and CO2 concentrations above 400 ppm (Burke et al., 2018). Despite very few sites having preserved direct sea-level proxies, data from coastal caves in Mallorca suggest that sea-level highstand was around ~17 m higher than present in the mid-Pliocene (~3 Ma) and ~ 25 m higher in the Early Pliocene Climatic Optimum (~5 Ma). These estimates agree with those obtained from data and models, that is 15 m on the United States East Coast for the mid-Pliocene (Moucha and Ruetenik, 2017), and 17.5 m from the Patagonian shorelines for the early Pliocene (Hollyday et al., 2023).
5.2 Paleo peatlands and methane
The safe limit for peatland/wetland carbon is first and foremost reliant on having waterlogged soils, but no paleo evidence suggests an upper temperature limit. This is corroborated by modern-day peatlands being present in tropical and temperate areas, with mean annual temperatures above 25°C, wherever moisture is maintained all (or most) year-round. Examples of past time periods with high temperatures leading to unprecedented levels of biomass production exist, i.e., the Carboniferous/Permian periods when the wet early forests (made of spore bearing arborescent plants growing on wet soils) colonised coastal areas of land and stored away all the biomass that we now know as coal seams; this process of carbon burial led to rapid CO2 drawdown (Feulner, 2017). Some of that rapid accumulation of peat deposits during this warm period may have also been related to fungi not having yet evolved to decompose lignin (Dighton, 2007; Hibbett et al., 2016). Paleo evidence of limits for the hydrological cycle, especially in warm conditions, do exist, and they suggest seasonality of rainfall is important, as any period where peat deposits are exposed to oxic conditions will lead to rapid decomposition (Garcin et al., 2022). This all means that high temperatures may not destabilise peatlands directly, especially if plants adapted to warmer conditions are able to colonise them, but hydrological cycle changes leading to more arid or more seasonal hydroclimates would.
Despite considerable warmth experienced in the Arctic during the LIG (Otto-Bliesner et al., 2021), methane only reached concentrations comparable to pre-industrial level (Köhler et al., 2017; Loulergue et al., 2008). In addition, isotopic measurements of 14CH4 in ice suggests that old carbon reservoirs were not an important component of the methane increase after the last glacial period (Dyonisius et al., 2020). This provides some reassurance that a rapid release of methane from permafrost or marine hydrates has not occurred during warm periods when substantial amounts of permafrost undoubtedly melted.
6 Conclusion
Since the beginning of the industrial revolution, the world has warmed by 1.3°C with impacts detectable all over the world. Climate change will continue in the future until anthropogenic emissions of GHGs are brought to zero. Natural negative feedbacks, such as the land and ocean carbon sinks, cannot counteract all of the anthropogenic forcing; they currently remove about 50% of anthropogenic emissions, but there is a strong consensus that climate change tends to reduce the efficiency of these natural sinks. The planet is on a trajectory to reach 1.5°C in the early 2030s at the current GHG emissions rate. However, our choices will determine the peak level of warming and associated climate impacts. Future warming is primarily driven by future, not past or current, GHG emissions. We assembled paleo information, observations and modelling evidence to assess the consequences of our choices, and the differences between a world with sustained high emissions versus a world with rapid net zero emissions followed by net negative emissions are staggering. Land and ocean ecosystems, as well as human systems, will see severe negative impacts that increase with warming levels. Keeping the planet within safe boundaries requires a rapid, strong and sustained reduction in GHG emissions, reaching net zero as soon as possible. There is urgency to make these decisions now while relatively “low” warming futures are still on the table. This will require considerable changes to our societies and economies, moving away from fossil fuel-based energy towards zero-carbon energy sources across all key sectors (e.g., transport, electricity and heat production, industry and agriculture), while also scaling up carbon dioxide removal to compensate for the hard to abate sectors such as agriculture. Inaction is not an option, the longer we delay, the harder it will be. The evidence base is clear—we need renewed commitment to action now. To quote the United Nations Secretary-General’s special address on climate action on June 5th 2024 “Now is the time to mobilise, now is the time to act, now is the time to deliver. This is our moment of truth” (Guterres, 2024).
Data availability statement
The original contributions presented in the study are included in the article/supplementary material, further inquiries can be directed to the corresponding author.
Author contributions
PF: Writing – original draft, Writing – review & editing. PA: Writing – original draft, Writing – review & editing. AG-S: Writing – original draft, Writing – review & editing. GJ: Writing – original draft, Writing – review & editing. CJ: Writing – original draft, Writing – review & editing. MK: Writing – original draft, Writing – review & editing. JL: Writing – original draft, Writing – review & editing. M-FL: Writing – original draft, Writing – review & editing. KR: Writing – original draft, Writing – review & editing. AR: Writing – original draft, Writing – review & editing. CS: Writing – original draft, Writing – review & editing. RS: Writing – original draft, Writing – review & editing. NW: Writing – original draft, Writing – review & editing. EZ: Writing – original draft, Writing – review & editing.
Funding
The author(s) declare that financial support was received for the research, authorship, and/or publication of this article. CJ was supported by the Joint UK BEIS/Defra Met Office Hadley Centre Climate Programme (GA01101). PF, CJ, and RS received support from the European Union’s Horizon 2020 research and innovation programme under Grant Agreement No. 101003536 (ESM2025—Earth System Models for the Future). CS received support from Horizon Europe under Grant Agreement No. 101081661 (WorldTrans). JL received support from the US National Science Foundation (grants 2142177 and 1802838). KR and EZ acknowledge funding through the Deutsche Forschungsgemeinschaft (STACY, grant no. 395588486) and the Federal Ministry of Education and Research of Germany (PalMod III, grant no. 01LP2311C). PA acknowledges grant 2023/04358-9 from FAPESP—Fundação de Amparo à Pesquisa do Estado de São Paulo. AG-S acknowledges funding from the European Union’s Horizon 2020 research and innovation programme under Grant Agreement No. 865403 (TroPeaCC) and from the Natural Environment Research Council (NERC standard grant number NE/S001166/1).
Acknowledgments
The authors acknowledge the participants of the WCRP Open Science Conference that took place 23–27 October 2023 in Kigali, Rwanda. Part of this study was undertaken by several working groups of the Past Global Changes (PAGES) project, which in turn received support from the Swiss Academy of Sciences and the Chinese Academy of Sciences. MK thanks Masahiko Fujii of the University of Tokyo for sharing recent advances in ocean acidification research.
Conflict of interest
The authors declare that the research was conducted in the absence of any commercial or financial relationships that could be construed as a potential conflict of interest.
Publisher’s note
All claims expressed in this article are solely those of the authors and do not necessarily represent those of their affiliated organizations, or those of the publisher, the editors and the reviewers. Any product that may be evaluated in this article, or claim that may be made by its manufacturer, is not guaranteed or endorsed by the publisher.
References
Allen, M. R., Frame, D. J., Huntingford, C., Jones, C. D., Lowe, J. A., Meinshausen, M., et al. (2009). Warming caused by cumulative carbon emissions towards the trillionth tonne. Nature 458, 1163–1166. doi: 10.1038/nature08019
Anderson, K., and Peters, G. (2016). The trouble with negative emissions. Science 354, 182–183. doi: 10.1126/science.aah4567
Armstrong McKay, D. I., Staal, A., Abrams, J. F., Winkelmann, R., Sakschewski, B., Loriani, S., et al. (2022). Exceeding 1.5°C global warming could trigger multiple climate tipping points. Science 377:eabn7950. doi: 10.1126/science.abn7950
Arora, V. K., Katavouta, A., Williams, R. G., Jones, C. D., Brovkin, V., Friedlingstein, P., et al. (2020). Carbon–concentration and carbon–climate feedbacks in CMIP6 models and their comparison to CMIP5 models. Biogeosciences 17, 4173–4222. doi: 10.5194/bg-17-4173-2020
Bereiter, B., Eggleston, S., Schmitt, J., Nehrbass-Ahles, C., Stocker, T. F., Fischer, H., et al. (2015). Revision of the EPICA dome C CO2 record from 800 to 600 kyr before present. Geophys. Res. Lett. 42, 542–549. doi: 10.1002/2014GL061957
Bindoff, N. L., Cheung, W. W. L., Kairo, J. G., Arístegui, J., Guinder, V. A., Hallberg, R., et al. (2019). “Changing Ocean, Marine ecosystems, and dependent communities” in The ocean and cryosphere in a changing climate: Special report of the intergovernmental panel on climate change. eds. Barange, M., and Seibel B. (Cambridge: Cambridge University Press), 447–588.
Boulton, C. A., Lenton, T. M., and Boers, N. (2022). Pronounced loss of Amazon rainforest resilience since the early 2000s. Nat. Clim. Chang. 12, 271–278. doi: 10.1038/s41558-022-01287-8
Burke, E., Chadburn, S., and Huntingford, C. (2022). Thawing permafrost as a nitrogen Fertiliser: implications for climate feedbacks. Nitrogen 3, 353–375. doi: 10.3390/nitrogen3020023
Burke, K. D., Williams, J. W., Chandler, M. A., Haywood, A. M., Lunt, D. J., and Otto-Bliesner, B. L. (2018). Pliocene and Eocene provide best analogs for near-future climates. Proc. Natl. Acad. Sci. 115, 13288–13293. doi: 10.1073/pnas.1809600115
Burton, C., Kelley, D. I., Jones, C. D., Betts, R. A., Cardoso, M., and Anderson, L. (2022). South American fires and their impacts on ecosystems increase with continued emissions. Clim. Resil. Sustain. 1:e8. doi: 10.1002/cli2.8
Canadell, J. G., Monteiro, P. M. S., Costa, M. H., Cotrim da Cunha, L., Cox, P. M., Eliseev, S., et al. (2021). “Global Carbon and other Biogeochemical Cycles and Feedbacks”, in Climate Change 2021: The Physical Science Basis. Contribution of Working Group I to the Sixth Assessment Report of the Intergovernmental Panel on Climate Change. eds. Masson-Delmotte, V., Zhai, P., Pirani, A., Connors, S. L., Péan, C., Berger, S. United Kingdom and New York, NY, USA: Cambridge University Press, Cambridge, 673–816.
Chadburn, S. E., Burke, E. J., Cox, P. M., Friedlingstein, P., Hugelius, G., and Westermann, S. (2017). An observation-based constraint on permafrost loss as a function of global warming. Nat. Clim. Chang. 7:340. doi: 10.1038/nclimate3262
Copernicus (2023). Copernicus: 2023 is the hottest year on record, with global temperatures close to the 1.5°C limit | Copernicus [WWW Document]. Available at: https://climate.copernicus.eu/copernicus-2023-hottest-year-record (accessed April 16, 2024).
Cornwall, C. E., Hepburn, C. D., McGraw, C. M., Currie, K. I., Pilditch, C. A., Hunter, K. A., et al. (2013). Diurnal fluctuations in seawater pH influence the response of a calcifying macroalga to ocean acidification. Proc. R. Soc. B Biol. Sci. 280:20132201. doi: 10.1098/rspb.2013.2201
Coxall, H. K., Huck, C. E., Huber, M., Lear, C. H., Legarda-Lisarri, A., O’Regan, M., et al. (2018). Export of nutrient rich northern component water preceded early Oligocene Antarctic glaciation. Nat. Geosci. 11, 190–196. doi: 10.1038/s41561-018-0069-9
Dekker, M. M., von der Heydt, A. S., and Dijkstra, H. A. (2018). Cascading transitions in the climate system. Earth Syst. Dynam. 9, 1243–1260. doi: 10.5194/esd-9-1243-2018
Dighton, J. (2007). Nutrient cycling by saprotrophic fungi in terrestrial habitats. The Mycota. 4, 287–300. doi: 10.1007/978-3-540-71840-6_16
Dumitru, O. A., Dyer, B., Austermann, J., Sandstrom, M. R., Goldstein, S. L., D’Andrea, W. J., et al. (2023). Last interglacial global mean sea level from high-precision U-series ages of Bahamian fossil coral reefs. Quat. Sci. Rev. 318:108287. doi: 10.1016/j.quascirev.2023.108287
Dutton, A., Carlson, A. E., Long, A. J., Milne, G. A., Clark, P. U., DeConto, R., et al. (2015). Sea-level rise due to polar ice-sheet mass loss during past warm periods. Science 349:aaa4019. doi: 10.1126/science.aaa4019
Dvorak, M. T., Armour, K. C., Frierson, D. M. W., Proistosescu, C., Baker, M. B., and Smith, C. J. (2022). Estimating the timing of geophysical commitment to 1.5 and 2.0 °C of global warming. Nat. Clim. Chang. 12, 547–552. doi: 10.1038/s41558-022-01372-y
Dyer, B., Austermann, J., D’Andrea, W. J., Creel, R. C., Sandstrom, M. R., Cashman, M., et al. (2021). Sea-level trends across the Bahamas constrain peak last interglacial ice melt. Proc. Natl. Acad. Sci. 118:e2026839118. doi: 10.1073/pnas.2026839118
Dyonisius, M. N., Petrenko, V. V., Smith, A. M., Hua, Q., Yang, B., Schmitt, J., et al. (2020). Old carbon reservoirs were not important in the deglacial methane budget. Science 367, 907–910. doi: 10.1126/science.aax0504
Eyring, V., Bony, S., Meehl, G. A., Senior, C. A., Stevens, B., Stouffer, R. J., et al. (2016). Overview of the coupled model Intercomparison project phase 6 (CMIP6) experimental design and organization. Geosci. Model Dev. 9, 1937–1958. doi: 10.5194/gmd-9-1937-2016
Feely, R. A., Sabine, C. L., Hernandez-Ayon, J. M., Ianson, D., and Hales, B. (2008). Evidence for upwelling of corrosive “acidified” water onto the continental shelf. Science 320, 1490–1492. doi: 10.1126/science.1155676
Feng, L., Palmer, P. I., Zhu, S., Parker, R. J., and Liu, Y. (2022). Tropical methane emissions explain large fraction of recent changes in global atmospheric methane growth rate. Nat. Commun. 13:1378. doi: 10.1038/s41467-022-28989-z
Feulner, G. (2017). Formation of most of our coal brought earth close to global glaciation. Proc. Natl. Acad. Sci. 114, 11333–11337. doi: 10.1073/pnas.1712062114
Figuerola, B., Hancock, A. M., Bax, N., Cummings, V. J., Downey, R., Griffiths, H. J., et al. (2021). A review and Meta-analysis of potential impacts of ocean acidification on marine Calcifiers from the Southern Ocean. Front. Mar. Sci. 8. doi: 10.3389/fmars.2021.584445
Flores, B. M., Montoya, E., Sakschewski, B., Nascimento, N., Staal, A., Betts, R. A., et al. (2024). Critical transitions in the Amazon forest system. Nature 626, 555–564. doi: 10.1038/s41586-023-06970-0
Folberth, G. A., Staniaszek, Z., Archibald, A. T., Gedney, N., Griffiths, P. T., Jones, C. D., et al. (2022). Description and evaluation of an emission-driven and fully coupled methane cycle in UKESM1. J. Adv. Model. Earth Syst. 14:e2021MS002982. doi: 10.1029/2021MS002982
Forster, P. M., Smith, C., Walsh, T., Lamb, W. F., Lamboll, R., Hall, B., et al. (2024). Indicators of global climate change 2023: annual update of key indicators of the state of the climate system and human influence. Earth Syst. Sci. Data 16, 2625–2658. doi: 10.5194/essd-16-2625-2024
Friedlingstein, P., Cox, P., Betts, R., Bopp, L., Von Bloh, W., Brovkin, V., et al. (2006). Climate-carbon cycle feedback analysis: results from the C4MIP model intercomparison. J. Clim. 19, 3337–3353. doi: 10.1175/JCLI3800.1
Friedlingstein, P., O’Sullivan, M., Jones, M., Andrew, R., Bakker, D., Hauck, J., et al. (2023). Global Carbon Budget 2023. Earth Syst. Sci. Data 15, 5301–5369. doi: 10.5194/essd-15-5301-2023
Fuss, S., Lamb, W. F., Callaghan, M. W., Hilaire, J., Creutzig, F., Amann, T., et al. (2018). Negative emissions—part 2: costs, potentials and side effects. Environ. Res. Lett. 13:063002. doi: 10.1088/1748-9326/aabf9f
Garcin, Y., Schefuß, E., Dargie, G. C., Hawthorne, D., Lawson, I. T., Sebag, D., et al. (2022). Hydroclimatic vulnerability of peat carbon in the Central Congo Basin. Nature 612, 277–282. doi: 10.1038/s41586-022-05389-3
Golledge, N. R., Clark, P. U., He, F., Dutton, A., Turney, C. S. M., Fogwill, C. J., et al. (2021). Retreat of the Antarctic ice sheet during the last Interglaciation and implications for future change. Geophys. Res. Lett. 48:e2021GL094513. doi: 10.1029/2021GL094513
Good, P., Jones, C., Lowe, J., Betts, R., Booth, B., and Huntingford, C. (2011). Quantifying environmental drivers of future tropical forest extent. J. Clim. 24, 1337–1349. doi: 10.1175/2010JCLI3865.1
Goodwin, P., Williams, R. G., and Ridgwell, A. (2014). Sensitivity of climate to cumulative carbon emissions due to compensation of ocean heat and carbon uptake. Nat. Geosci. 8:29. doi: 10.1038/ngeo2304
Gregory, J. M., Jones, C. D., Cadule, P., and Friedlingstein, P. (2009). Quantifying carbon cycle feedbacks. J. Clim. 22, 5232–5250. doi: 10.1175/2009JCLI2949.1
Gruber, N., Boyd, P. W., Frölicher, T. L., and Vogt, M. (2021). Biogeochemical extremes and compound events in the ocean. Nature 600, 395–407. doi: 10.1038/s41586-021-03981-7
Guterres, A. (2024). Secretary-General’s special address on climate action “a moment of truth.” Avaiilable at: https://www.un.org/sg/en/content/sg/statement/2024-06-05/secretary-generals-special-address-climate-action-moment-of-truth-delivered
Hajima, T., Kawamiya, M., Ito, A., Tachiiri, K., Jones, C., Arora, V., et al. (2024). Consistency of global carbon budget between concentration- and emission-driven historical experiments simulated by CMIP6 earth system models and suggestion for improved simulation of CO2 concentration. EGUsphere, 1–49. doi: 10.5194/egusphere-2024-188
Hausfather, Z., and Peters, G. P. (2020). Emissions – the ‘business as usual’ story is misleading. Nature 577, 618–620. doi: 10.1038/d41586-020-00177-3
Heck, V., Gerten, D., Lucht, W., and Popp, A. (2018). Biomass-based negative emissions difficult to reconcile with planetary boundaries. Nat. Clim. Chang. 8, 151–155. doi: 10.1038/s41558-017-0064-y
Hibbett, D., Blanchette, R., Kenrick, P., and Mills, B. (2016). Climate, decay, and the death of the coal forests. Curr. Biol. 26, R563–R567. doi: 10.1016/j.cub.2016.01.014
Hollyday, A., Austermann, J., Lloyd, A., Hoggard, M., Richards, F., and Rovere, A. (2023). A revised estimate of early Pliocene global mean sea level using geodynamic models of the Patagonian slab window. Geochem. Geophys. Geosyst. 24:e2022GC010648. doi: 10.1029/2022GC010648
Hubau, W., Lewis, S. L., Phillips, O. L., Affum-Baffoe, K., Beeckman, H., Cuní-Sanchez, A., et al. (2020). Asynchronous carbon sink saturation in African and Amazonian tropical forests. Nature 579, 80–87. doi: 10.1038/s41586-020-2035-0
Jaeger, C. C., and Jaeger, J. (2011). Three views of two degrees. Clim. Change Econ. 3, 145–166. doi: 10.1007/s10113-010-0190-9
Jones, C. D. (2022). “Negative emissions: the role and response of the climate system” in Greenhouse gas removal technologies. The Royal Society of Chemistry. eds. M. Bui and N. Mac Dowell, 27–56.
Jones, C. D., Ciais, P., Davis, S. J., Friedlingstein, P., Gasser, T., Peters, G. P., et al. (2016). Simulating the earth system response to negative emissions. Environ. Res. Lett. 11. doi: 10.1088/1748-9326/11/9/095012
Jones, C. D., Frölicher, T. L., Koven, C., MacDougall, A. H., Matthews, H. D., Zickfeld, K., et al. (2019). The zero emissions commitment model Intercomparison project (ZECMIP) contribution to C4MIP: quantifying committed climate changes following zero carbon emissions. Geosci. Model Dev. 12, 4375–4385. doi: 10.5194/gmd-12-4375-2019
Jones, C., Lowe, J., Liddicoat, S., and Betts, R. (2009). Committed terrestrial ecosystem changes due to climate change. Nat. Geosci. 2, 484–487. doi: 10.1038/ngeo555
Keller, D. P., Lenton, A., Scott, V., Vaughan, N. E., Bauer, N., Ji, D., et al. (2018). The carbon dioxide removal model Intercomparison project (CDRMIP): rationale and experimental protocol for CMIP6. Geosci. Model Dev. 11, 1133–1160. doi: 10.5194/gmd-11-1133-2018
Kemp, A. C., Dutton, A., and Raymo, M. E. (2015). Paleo constraints on Future Sea-level rise. Curr. Clim. Chang. Rep. 1, 205–215. doi: 10.1007/s40641-015-0014-6
Köhler, P., Nehrbass-Ahles, C., Schmitt, J., Stocker, T. F., and Fischer, H. (2017). A 156 kyr smoothed history of the atmospheric greenhouse gases CO2, CH4, and N2O and their radiative forcing. Earth Syst. Sci. Data 9, 363–387. doi: 10.5194/essd-9-363-2017
Koven, C. D., Arora, V. K., Cadule, P., Fisher, R. A., Jones, C. D., Lawrence, D. M., et al. (2022). Multi-century dynamics of the climate and carbon cycle under both high and net negative emissions scenarios. Earth Syst. Dynam. 13, 885–909. doi: 10.5194/esd-13-885-2022
Koven, C. D., Riley, W. J., and Stern, A. (2013). Analysis of permafrost thermal dynamics and response to climate change in the cmip5 earth system models. J. Clim. 26, 1877–1900. doi: 10.1175/JCLI-D-12-00228.1
Kwiatkowski, L., Berger, M., Bopp, L., Doléac, S., and Ho, D. T. (2023). Contrasting carbon dioxide removal potential and nutrient feedbacks of simulated ocean alkalinity enhancement and macroalgae afforestation. Environ. Res. Lett. 18:124036. doi: 10.1088/1748-9326/ad08f9
Kwiatkowski, L., Torres, O., Bopp, L., Aumont, O., Chamberlain, M., Christian, J. R., et al. (2020). Twenty-first century ocean warming, acidification, deoxygenation, and upper-ocean nutrient and primary production decline from CMIP6 model projections. Biogeosciences 17, 3439–3470. doi: 10.5194/bg-17-3439-2020
Ladant, J.-B., Donnadieu, Y., Bopp, L., Lear, C. H., and Wilson, P. A. (2018). Meridional contrasts in productivity changes driven by the opening of Drake Passage. Paleoceanogr. Paleoclimatol. 33, 302–317. doi: 10.1002/2017PA003211
Lan, X., Thoning, K. W., and Dlugokencky, E. J. (2022). Trends in globally-averaged CH4, N2O, and SF6 determined from NOAA global monitoring laboratory measurements. doi: 10.15138/P8XG-AA10
Lapola, D. M., Pinho, P., Barlow, J., Aragão, L. E. O. C., Berenguer, E., Carmenta, R., et al. (2023). The drivers and impacts of Amazon forest degradation. Science 379:eabp8622. doi: 10.1126/science.abp8622
Lauvset, S. K., Carter, B. R., Pèrez, F. F., Jiang, L.-Q., Feely, R. A., Velo, A., et al. (2020). Processes driving global Interior Ocean pH distribution. Glob. Biogeochem. Cycles 34:e2019GB006229. doi: 10.1029/2019GB006229
Lear, C. H., Bailey, T. R., Pearson, P. N., Coxall, H. K., and Rosenthal, Y. (2008). Cooling and ice growth across the Eocene-Oligocene transition. Geology 36, 251–254. doi: 10.1130/G24584A.1
Lenton, T. M., Held, H., Kriegler, E., Hall, J. W., Lucht, W., Rahmstorf, S., et al. (2008). Tipping elements in the earth’s climate system. Proc. Natl. Acad. Sci. USA 105, 1786–1793. doi: 10.1073/pnas.0705414105
Liddicoat, S. K., Wiltshire, A. J., Jones, C. D., Arora, V. K., Brovkin, V., Cadule, P., et al. (2021). Compatible fossil fuel CO2 emissions in the CMIP6 earth system models’ historical and shared socioeconomic pathway experiments of the twenty-first century. J. Clim. 34, 2853–2875. doi: 10.1175/JCLI-D-19-0991.1
Loulergue, L., Schilt, A., Spahni, R., Masson-Delmotte, V., Blunier, T., Lemieux, B., et al. (2008). Orbital and millennial-scale features of atmospheric ch4 over the past 800,000 years. Nature 453, 383–386. doi: 10.1038/nature06950
Lüthi, D., Le Floch, M., Bereiter, B., Blunier, T., Barnola, J. M., Siegenthaler, U., et al. (2008). High-resolution carbon dioxide concentration record 650,000-800,000 years before present. Nature 453, 379–382. doi: 10.1038/nature06949
Ma, D., Gregor, L., and Gruber, N. (2023). Four decades of trends and drivers of global Surface Ocean acidification. Glob. Biogeochem. Cycles 37:e2023GB007765. doi: 10.1029/2023GB007765
MacDougall, A. H., and Friedlingstein, P. (2015). The origin and limits of the near proportionality between climate warming and cumulative CO2 emissions. J. Clim. 28, 4217–4230. doi: 10.1175/JCLI-D-14-00036.1
MacDougall, A. H., Frölicher, T. L., Jones, C. D., Rogelj, J., Matthews, H. D., Zickfeld, K., et al. (2020). Is there warming in the pipeline? A multi-model analysis of the zero emissions commitment from CO2. Biogeosciences 17, 2987–3016. doi: 10.5194/bg-17-2987-2020
Masson-Delmotte, V. (2021). IPCC, 2021: summary for policymakers. In: Climate change 2021: the physical science basis. Contribution of Working Group I to the Sixth Assessment Report of the Intergovernmental Panel on Climate Change.
Matthews, H. D., Gillett, N. P., Stott, P. A., and Zickfeld, K. (2009). The proportionality of global warming to cumulative carbon emissions. Nature 459, 829–832. doi: 10.1038/nature08047
Matthews, H. D., Tokarska, K. B., Nicholls, Z. R. J., Rogelj, J., Canadell, J. G., Friedlingstein, P., et al. (2020). Opportunities and challenges in using remaining carbon budgets to guide climate policy. Nat. Geosci. 13, 769–779. doi: 10.1038/s41561-020-00663-3
McKinley, G. A., Fay, A. R., Eddebbar, Y. A., Gloege, L., and Lovenduski, N. S. (2020). External forcing explains recent decadal variability of the ocean carbon sink. AGU Adv. 1:e2019AV000149. doi: 10.1029/2019AV000149
Meinshausen, M., Smith, S. J., Calvin, K., Daniel, J. S., Kainuma, M. L. T., Lamarque, J.-F., et al. (2011). The RCP greenhouse gas concentrations and their extensions from 1765 to 2300. Clim. Chang. 109, 213–241. doi: 10.1007/s10584-011-0156-z
Merfort, L., Bauer, N., Humpenöder, F., Klein, D., Strefler, J., Popp, A., et al. (2023). Bioenergy-induced land-use-change emissions with sectorally fragmented policies. Nat. Clim. Chang. 13, 685–692. doi: 10.1038/s41558-023-01697-2
Moucha, R., and Ruetenik, G. A. (2017). Interplay between dynamic topography and flexure along the U.S. Atlantic passive margin: insights from landscape evolution modeling. Glob. Planet. Change 149, 72–78. doi: 10.1016/j.gloplacha.2017.01.004
Nakicenovic, N., Alcamo, J., Davis, G., de Vries, B., Fenhann, J., Gaffin, S., et al. (2000). Special report on emissions scenarios: A special report of working group III of the intergovernmental panel on climate change. Cambridge, UK, 599 pp.
Nehrbass-Ahles, C., Shin, J., Schmitt, J., Bereiter, B., Joos, F., Schilt, A., et al. (2020). Abrupt CO2 release to the atmosphere under glacial and early interglacial climate conditions. Science 369, 1000–1005. doi: 10.1126/science.aay8178
Norby, R. J., DeLucia, E. H., Gielen, B., Calfapietra, C., Giardina, C. P., King, J. S., et al. (2005). Forest response to elevated co2 is conserved across a broad range of productivity. Proc. Natl. Acad. Sci. USA 102, 18052–18056. doi: 10.1073/pnas.0509478102
O’Neill, B. C., Tebaldi, C., van Vuuren, D. P., Eyring, V., Friedlingstein, P., Hurtt, G., et al. (2016). The scenario model Intercomparison project (ScenarioMIP) for CMIP6. Geosci. Model Dev. 9, 3461–3482. doi: 10.5194/gmd-9-3461-2016
Otto-Bliesner, B. L., Brady, E. C., Zhao, A., Brierley, C. M., Axford, Y., Capron, E., et al. (2021). Large-scale features of last interglacial climate: results from evaluating the lig127k simulations for the coupled model Intercomparison project (CMIP6)–paleoclimate modeling Intercomparison project (PMIP4). Clim. Past 17, 63–94. doi: 10.5194/cp-17-63-2021
Parry, I. M., Ritchie, P. D. L., and Cox, P. M. (2022). Evidence of localised Amazon rainforest dieback in CMIP6 models. Earth Syst. Dynam. 13, 1667–1675. doi: 10.5194/esd-13-1667-2022
Pugh, T. A. M., Jones, C. D., Huntingford, C., Burton, C., Arneth, A., Brovkin, V., et al. (2018). A large committed long-term sink of carbon due to vegetation dynamics. Earths Future 6, 1413–1432. doi: 10.1029/2018EF000935
Rehfeld, K., Münch, T., Ho, S. L., and Laepple, T. (2018). Global patterns of declining temperature variability from the last glacial maximum to the Holocene. Nature 554, 356–359. doi: 10.1038/nature25454
Rehfeld, K., and Ziegler, E. (2023). Future climate in the context of the earth system evolution over the last 66 million years. doi: 10.6084/m9.figshare.22732775.v1
Riahi, K., Rao, S., Krey, V., Cho, C., Chirkov, V., Fischer, G., et al. (2011). RCP 8.5—A scenario of comparatively high greenhouse gas emissions. Clim. Chang. 109:33. doi: 10.1007/s10584-011-0149-y
Riahi, K., van Vuuren, D. P., Kriegler, E., Edmonds, J., O’Neill, B. C., Fujimori, S., et al. (2017). The shared socioeconomic pathways and their energy, land use, and greenhouse gas emissions implications: an overview. Glob. Environ. Change 42, 153–168. doi: 10.1016/j.gloenvcha.2016.05.009
Ritchie, P. D. L., Clarke, J. J., Cox, P. M., and Huntingford, C. (2021). Overshooting tipping point thresholds in a changing climate. Nature 592, 517–523. doi: 10.1038/s41586-021-03263-2
Rogelj, J., Schaeffer, M., Friedlingstein, P., Gillett, N. P., van Vuuren, D. P., Riahi, K., et al. (2016). Differences between carbon budget estimates unravelled. Nat. Clim. Chang. 6, 245–252. doi: 10.1038/nclimate2868
Sanderson, B. M., Booth, B. B. B., Dunne, J., Eyring, V., Fisher, R. A., Friedlingstein, P., et al. (2023). The need for carbon emissions-driven climate projections in CMIP7. EGUsphere, 1–51. doi: 10.5194/egusphere-2023-2127
Sarofim, M., Smith, C., Malek, P., McDuffie, E., Hartin, C., and Lay, C. (2023). How high is high enough? A multi-million-member ensemble analysis of future climate scenarios and their relevance. doi: 10.21203/rs.3.rs-3158067/v1
Saunois, M., Stavert, A. R., Poulter, B., Bousquet, P., Canadell, J. G., Jackson, R. B., et al. (2020). The global methane budget 2000–2017. Earth Syst. Sci. Data 12, 1561–1623. doi: 10.5194/essd-12-1561-2020
Séférian, R., Rocher, M., Guivarch, C., and Colin, J. (2018). Constraints on biomass energy deployment in mitigation pathways: the case of water scarcity. Environ. Res. Lett. 13:054011. doi: 10.1088/1748-9326/aabcd7
Smith, P., Davis, S. J., Creutzig, F., Fuss, S., Minx, J., Gabrielle, B., et al. (2016). Biophysical and economic limits to negative CO2 emissions. Nat. Clim. Chang. 6, 42–50. doi: 10.1038/nclimate2870
Smith, C. J., Forster, P. M., Allen, M., Leach, N., Millar, R. J., Passerello, G. A., et al. (2018). FAIR v1.3: a simple emissions-based impulse response and carbon cycle model. Geosci. Model Dev. 11, 2273–2297. doi: 10.5194/gmd-11-2273-2018
Smith, C. J., and Gasser, T. (2022). Modeling the non-CO2 contribution to climate change. One Earth 5, 1330–1335. doi: 10.1016/j.oneear.2022.11.007
Solomon, S., Plattner, G. K., Knutti, R., and Friedlingstein, P. (2009). Irreversible climate change due to carbon dioxide emissions. Proc. Natl. Acad. Sci. USA 106, 1704–1709. doi: 10.1073/pnas.0812721106
Sommers, A. N., Otto-Bliesner, B. L., Lipscomb, W. H., Lofverstrom, M., Shafer, S. L., Bartlein, P. J., et al. (2021). Retreat and regrowth of the Greenland ice sheet during the last interglacial as simulated by the CESM2-CISM2 coupled climate–ice sheet model. Paleoceanogr. Paleoclimatol. 36:e2021PA004272. doi: 10.1029/2021PA004272
Tao, S., Wigneron, J.-P., Chave, J., Tang, Z., Wang, Z., Zhu, J., et al. (2023). Little evidence that Amazonian rainforests are approaching a tipping point. Nat. Clim. Chang. 13, 1317–1320. doi: 10.1038/s41558-023-01853-8
Taylor, K. E., Stouffer, R. J., and Meehl, G. A. (2012). A summary of the cmip5 experiment design. Bull. Am. Meteorol. Soc. 93, 485–498. doi: 10.1175/BAMS-D-11-00094.1
Tebaldi, C., Debeire, K., Eyring, V., Fischer, E., Fyfe, J., Friedlingstein, P., et al. (2021). Climate model projections from the scenario model Intercomparison project (ScenarioMIP) of CMIP6. Earth Syst. Dynam. 12, 253–293. doi: 10.5194/esd-12-253-2021
van Vuuren, D. P., Stehfest, E., den Elzen, M., Kram, T., van Vliet, J., Beltran, A. M., et al. (2011). RCP3-PD: exploring the possibilities to limit global mean temperature change to less than 2°C. Clim. Chang. 109, 95–116. doi: 10.1007/s10584-011-0152-3
Varney, R. M., Chadburn, S. E., Burke, E. J., Jones, S., Wiltshire, A. J., and Cox, P. M. (2023a). Simulated responses of soil carbon to climate change in CMIP6 earth system models: the role of false priming. Biogeosciences 20, 3767–3790. doi: 10.5194/bg-20-3767-2023
Varney, R. M., Friedlingstein, P., Chadburn, S. E., Burke, E. J., and Cox, P. M. (2023b). Soil carbon-concentration and carbon-climate feedbacks in CMIP6 earth system models. EGUsphere, 1–21. doi: 10.5194/egusphere-2023-2666
Watanabe, M., and Kawamiya, M. (2017). Remote effects of mixed layer development on ocean acidification in the subsurface layers of the North Pacific. J. Oceanogr. 73, 771–784. doi: 10.1007/s10872-017-0431-3
Wood, R. A., Crucifix, M., Lenton, T. M., Mach, K. J., Moore, C., New, M., et al. (2023). A climate science toolkit for high impact-low likelihood climate risks. Earths Future 11:e2022EF003369. doi: 10.1029/2022EF003369
Woodard, D. L., Davis, S. J., and Randerson, J. T. (2019). Economic carbon cycle feedbacks may offset additional warming from natural feedbacks. Proc. Natl. Acad. Sci. 116, 759–764. doi: 10.1073/pnas.1805187115
Wunderling, N., von der Heydt, A. S., Aksenov, Y., Barker, S., Bastiaansen, R., Brovkin, V., et al. (2024). Climate tipping point interactions and cascades: a review. Earth Syst. Dynam. 15, 41–74. doi: 10.5194/esd-15-41-2024
Yamamoto, A., Kawamiya, M., Ishida, A., Yamanaka, Y., and Watanabe, S. (2012). Impact of rapid sea-ice reduction in the Arctic ocean on the rate of ocean acidification. Biogeosciences 9, 2365–2375. doi: 10.5194/bg-9-2365-2012
Zaehle, S., Medlyn, B. E., De Kauwe, M. G., Walker, A. P., Dietze, M. C., Hickler, T., et al. (2014). Evaluation of 11 terrestrial carbon–nitrogen cycle models against observations from two temperate free-air CO2 enrichment studies. New Phytol. 202, 803–822. doi: 10.1111/nph.12697
Keywords: climate change, earth system, climate projection, carbon cycle, paleoclimate
Citation: Friedlingstein P, Artaxo P, Gallego-Sala AV, Jia G, Jones CD, Kawamiya M, Loisel J, Loutre M-F, Rehfeld K, Rovere A, Smith C, Séférian R, van der Wel N and Ziegler E (2024) Earth system responses to different levels of greenhouse gas emissions mitigation. Front. Clim. 6:1480208. doi: 10.3389/fclim.2024.1480208
Edited by:
Sirkku Juhola, University of Helsinki, FinlandReviewed by:
Lori T. Sentman, Princeton University, United StatesCopyright © 2024 Friedlingstein, Artaxo, Gallego-Sala, Jia, Jones, Kawamiya, Loisel, Loutre, Rehfeld, Rovere, Smith, Séférian, van der Wel and Ziegler. This is an open-access article distributed under the terms of the Creative Commons Attribution License (CC BY). The use, distribution or reproduction in other forums is permitted, provided the original author(s) and the copyright owner(s) are credited and that the original publication in this journal is cited, in accordance with accepted academic practice. No use, distribution or reproduction is permitted which does not comply with these terms.
*Correspondence: Pierre Friedlingstein, cC5mcmllZGxpbmdzdGVpbkBleGV0ZXIuYWMudWs=